- 1Alaska Science Center, U.S. Geological Survey, Anchorage, AK, United States
- 2Division of Wildlife Conservation, Alaska Department of Fish and Game, Fairbanks, AK, United States
- 3U.S. Fish & Wildlife Service, Anchorage, AK, United States
- 4Department of Ecology and Conservation Biology, Texas A&M University, College Station, TX, United States
- 5Department of Rangeland, Wildlife and Fisheries Management, Texas A&M University, College Station, TX, United States
Investigators have speculated that the climate-driven “greening of the Arctic” may benefit barren-ground caribou populations, but paradoxically many populations have declined in recent years. This pattern has raised concerns about the influence of summer habitat conditions on caribou demographic rates, and how populations may be impacted in the future. The short Arctic summer provides caribou with important forage resources but is also the time they are exposed to intense harassment by insects, factors which are both being altered by longer, warmer growing seasons. To better understand the effects of summer forage and insect activity on Arctic caribou demographic rates, we investigated the influence of estimated forage biomass, digestible energy (DE), digestible nitrogen (DN), and mosquito activity on the reproductive success and survival of adult females in the Central Arctic Herd on the North Slope of Alaska. We tested the hypotheses that greater early summer DN would increase subsequent reproduction (parturition and late June calving success) while greater biomass and DE would increase adult survival (September–May), and that elevated mosquito activity would reduce both demographic rates. Because the period when abundant forage DN is limited and overlaps with the period of mosquito harassment, we also expected years with low DN and high harassment to synergistically reduce caribou reproductive success. Examining these relationships at the individual-level, using GPS-collared females, and at the population-level, using long-term monitoring data, we generally found support for our expectations. Greater early summer DN was associated with increased subsequent calving success, while greater summer biomass was associated with increased adult survival. Mosquito activity was associated with reductions in adult female parturition, late June calving success, and survival, and in years with low DN, had compounding effects on subsequent late June calving success. Our findings indicate that summer nutrition and mosquito activity collectively influence the demographic rates of Arctic caribou, and may impact the dynamics of populations in the future under changing environmental conditions.
Introduction
As the Arctic warms at three times the global rate (Arctic Monitoring and Assessment Report, 2021) there are growing concerns about the impacts of climate change on the behavior and demography of migratory, barren-ground caribou (Rangifer tarandus) populations (Fauchald et al., 2017; Mallory and Boyce, 2018; Eikelenboom et al., 2021), and the people that rely on them for subsistence (Kenny et al., 2018). The dynamics of large, barren-ground caribou populations are strongly influenced by bottom-up forage conditions (Messier et al., 1988; Mallory et al., 2018), causing investigators to speculate that the climate-driven “greening of the Arctic” will benefit caribou and conspecific reindeer by providing earlier and longer access to summer forage resources (Cebrian et al., 2008; Tveraa et al., 2013). Barren-ground caribou populations fluctuate widely over the span of decades (Bongelli et al., 2020), but in recent years, most populations have declined in association with warmer, more productive growing seasons (Fauchald et al., 2017). The mechanism driving this unexpected relationship is unknown, but the pattern has raised significant questions about the role of summer habitat conditions in influencing caribou population dynamics, and how climate change may affect caribou herds in the future (Fauchald et al., 2017; Mallory and Boyce, 2018).
Arctic caribou exhibit the longest terrestrial migrations on earth to reach their summer ranges (Fancy et al., 1989; Joly et al., 2020b) which provide access to important forage resources (White et al., 1975; Griffith et al., 2002; Johnson et al., 2021). As capital breeders, caribou must maximize their foraging opportunities during the short Arctic summer to regain body stores lost during the previous winter and amass stores for the upcoming winter and reproductive cycle (Barboza and Parker, 2008; Parker et al., 2009; Taillon et al., 2013). Captive studies on caribou have demonstrated that greater consumption of forage biomass and energy enable caribou to increase their mass, fat reserves, and deposition of protein, while sufficient body protein is needed for fetal development and early lactation (Chan-McLeod et al., 1994; Barboza and Parker, 2008; Taillon et al., 2013; Thompson and Barboza, 2013). Arctic caribou subsist on lichen and senesced vascular vegetation most of the year (Russell et al., 1993; Joly et al., 2015), a diet that is generally high in digestible energy (DE) but very low in digestible protein [indexed by digestible nitrogen (DN)]. During the short Arctic summer growing season, recent studies have found that DE appears to be sufficient in caribou forage, while DN is only abundant in newly emergent vegetation, declining below maintenance levels after mid-summer (Barboza et al., 2018; Johnson et al., 2021). Given this constraint, Barboza et al. (2018) hypothesized that caribou may be limited by their ability to store adequate protein when it is available, which could have effects on their subsequent reproductive success. Given the challenge, however, of quantifying forage quality and quantity over such remote and expansive landscapes, little is known about the role of summer nutrition in driving observed variation in the demographic rates of Arctic caribou.
Summer is a critical period for Arctic caribou to acquire nutrients, but it is also the time when they experience intense harassment by insects (Joly et al., 2020a), which constrains their foraging opportunities. During mid-summer when temperatures peak, harassment by mosquitoes (Culicidae) and oestrid flies (Oestridae) strongly influences the behavior of Arctic caribou and reindeer. Harassment has been associated with reduced time spent foraging, increased time spent walking and running, and movements to areas that are cooler, windier, and have less preferred forage (White et al., 1975; Hagemoen and Reimers, 2002; Witter et al., 2012; Ehlers et al., 2021; Johnson et al., 2021). Given these effects, summers with greater insect harassment have been correlated with reduced calf weights and adult body condition (Helle and Tarvainen, 1984; Weladji et al., 2003; Gagnon et al., 2020), presumably due to decreased foraging efficiency and increased energy expenditure. Investigators have suggested that these effects could have demographic consequences (Colman et al., 2003; Weladji et al., 2003), but the relationship between summer insect harassment and caribou demography has been unknown.
While forage availability and insect harassment strongly shape Arctic caribou behavior during the summer, both factors are being altered as a function of changing climate conditions. Earlier phenology and warmer temperatures in the Arctic appear to be increasing the biomass (Elmendorf et al., 2012; Doiron et al., 2014), but potentially reducing the quality, of summer forage for caribou, particularly forage protein (Doiron et al., 2014; Zamin et al., 2017; Johnson et al., 2018; Richert et al., 2021). During such summers, forage protein can be lower and often declines more quickly, truncating the period that abundant protein is available. Longer, warmer summers may also increase the duration and intensity of insect harassment for Arctic caribou (Witter et al., 2012; Culler et al., 2015; Koltz and Culler, 2021), which could further reduce foraging efficiency and increase energy expenditure, especially during the limited period when forage protein is abundant. Understanding the influence of these factors, and how they may interact, is important as investigators have speculated that even small reductions in summer forage opportunities could have cascading effects on caribou body condition, and subsequently, demographic rates (White, 1983).
To examine the influence of summer forage and insect conditions on Arctic caribou demographic rates, we investigated the roles of forage biomass, DE, DN, and an index of mosquito harassment on the reproductive success and survival of adult females in the Central Arctic Herd (CAH) on the North Slope of Alaska. Because caribou are capital breeders, forage resources acquired during the summer months are expected to influence reproductive success in the subsequent year (Taillon et al., 2013). Therefore, we hypothesized that Arctic caribou reproductive success would (1) increase following summers with greater DN, (2) decrease following summers predicted to have greater mosquito activity, and (3) experience compounding effects after years with both lower DN and higher mosquito activity, as the limited period of abundant DN overlaps with the period of mosquito harassment. We also hypothesized that adult survival would (4) increase following summers with higher forage biomass and DE, and (5) decrease following summers predicted to have higher mosquito activity. To evaluate these hypotheses, we paired demographic and habitat-use data from caribou with spatiotemporal estimates of summer forage and mosquito activity (Johnson et al., 2018, 2021). We tested hypotheses at the individual-level, by evaluating the summer habitat use patterns of global positioning system (GPS)-collared females relative to their annual productivity and survival, and at the population-level, by capitalizing on long-term monitoring data to assess herd summer range conditions relative to annual herd demographic rates. This approach enabled us to take advantage of fine-scale habitat conditions experienced by individual GPS-collared females, while also evaluating long-term trends based on monitoring data that included larger samples of females collared with both GPS and vey high frequency (VHF) technology.
Materials and methods
Study system
The summer range of the CAH includes portions of the Arctic Coastal Plain, and the foothills and mountains of the Brooks Range, Alaska, United States (Figure 1). The terrain gradually rises from sea level along the Arctic Ocean to approximately 1,000 m in the mountains. Landcover in the summer range is dominated by wet and dry graminoids, tussock tundra, dwarf shrub vegetation, and thaw lakes (for additional details see Johnson et al., 2018). Summers in this region are short, cool, and moist, while winters are long, cold and dry with annual precipitation averaging 14.5 cm (Deadhorse AP weather station).1 Between 1991 and 2020, the average temperature in July (warmest month) was 8.5°C and in January (coolest month) was −26.1°C.2
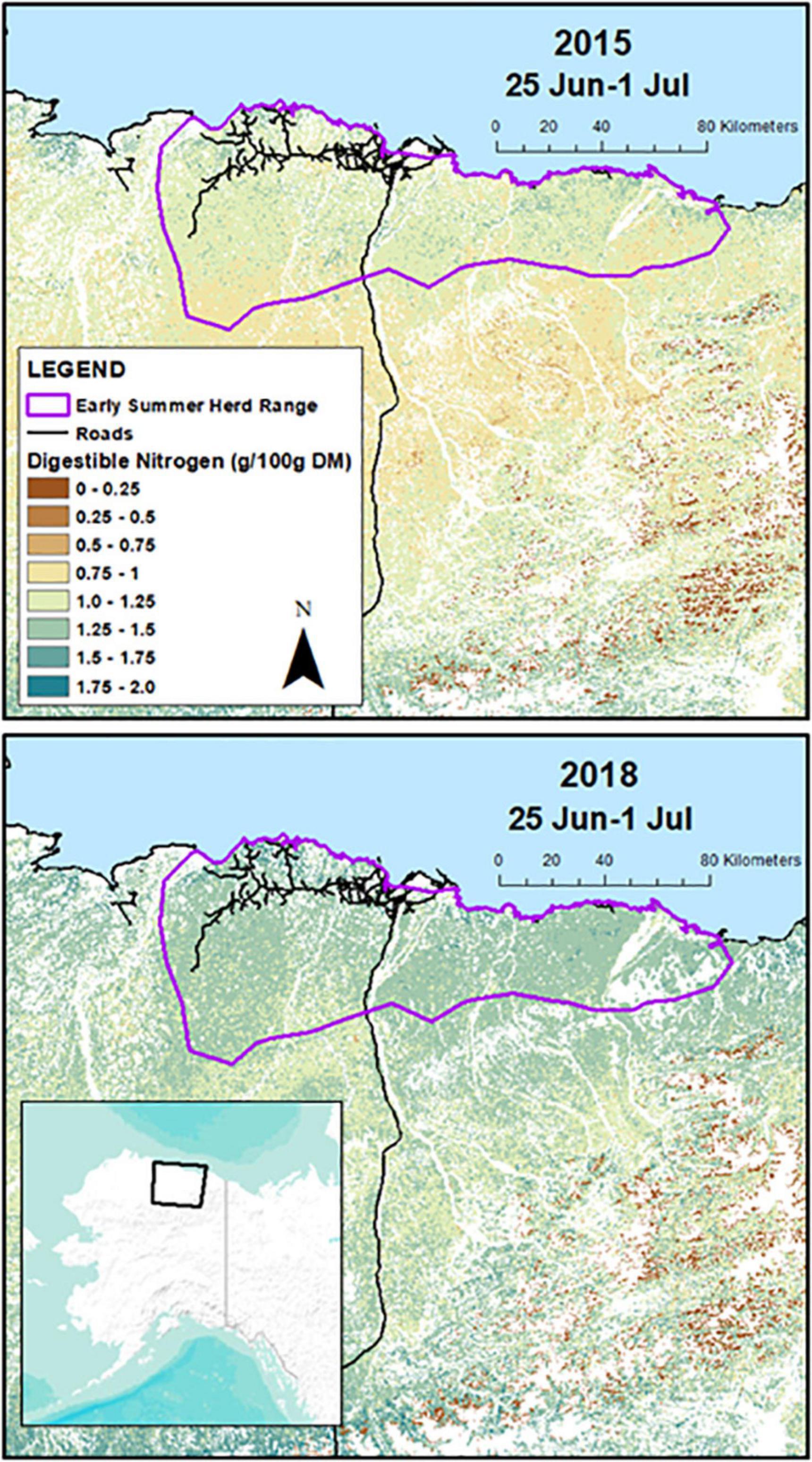
Figure 1. Prediction area for weekly summer forage conditions in the Central Arctic Caribou Herd on the North Slope of Alaska. The top panel depicts estimates of digestible nitrogen 25 June–1 July in 2015, a year which had early spring phenology, and the bottom panel depicts digestible nitrogen the same week in 2018, a year with late spring phenology. The purple line shows the early summer herd range polygon (16 June–15 July) where caribou were located during the period that digestible nitrogen is abundant.
The CAH numbered ∼19,000 caribou when Alaska Department of Fish and Game (ADFG) estimated its population size in 1991 (Lenart, 2021). It increased over the next 2 decades to reach an estimated peak of ∼68,000 caribou in 2010, and then precipitously declined to ∼23,000 in 2016. Since then, the herd appears to be slowly increasing in size, and was most recently estimated at ∼30,000 in 2019 (Supplementary Figure 1).
In May, female CAH caribou migrate north from the Brooks Range to their calving grounds on the central coastal plain. After calving in early June, the CAH continues to move north toward the coast during the post-calving and mosquito harassment periods, and then after mid-summer, shifts south toward the foothills (Johnson et al., 2021). In the fall, the CAH migrates south to winter ranges in the foothills of the Brooks Range, or on the south side of the range (Nicholson et al., 2016), typically wintering in the same general area (Pedersen et al., 2021).
Caribou data
Between 2002 and 2019 ADFG captured and collared adult female CAH caribou (≥3 years old) via helicopter net-gunning. Depending on the year, different numbers of caribou were captured during March–June and fit with either VHF or GPS collars. Caribou GPS collars (Telonics, Lotek) were primarily deployed between 2003–2007 and 2014–2020 (sample sizes in Supplementary Table 1) and were programed to collect a location every 2–12 h, depending on the year and collar type. We excluded GPS locations from the first week post-capture to reduce any capture-related effects in caribou movements. If GPS-collared females left the primary summer range where we estimated forage conditions (Figure 1; described below) during the summer (16 June–31 August), they were excluded from the analyses. Females wearing GPS collars were monitored for survival throughout the year, and were assumed to be dead when a mortality signal was emitted from a collar, or the collar was stationary. Females with GPS collars were used to investigate relationships between individual habitat-use patterns and their annual productivity and survival (being parturient, having a calf at heel in late June, and surviving the remainder of the biological year), while all collared females (wearing GPS and VHF collars) were used to collect annual information on herd productivity (the proportion parturient and the proportion with a calf at heel in late June).
Each year (2002–2020), ADFG conducted two aerial fixed-wing surveys of collared female caribou to quantify reproductive success. During late May and early June (30 May–11 June), the peak of the calving season, they observed each female 1–3 times to determine whether she was parturient (had produced a calf or was likely to). Parturition status was based on the presence of a newborn calf, distended udder, or antlers, a classification approach that has been found to be 97% accurate from fixed wing aircraft (Whitten, 1995). The udder of a caribou distends prior to parturition and remains distended for several days if a calf is lost. Additionally, parturient females typically retain one or both antlers into the calving season while most non-parturient females shed them prior to the calving season (Whitten, 1995). A second aerial survey was then conducted in late June (18–26 June) to determine whether the calves of parturient females survived the neonate period and were still at heel. ADFG assumes there is some error in late June calf survival classifications, which likely increases when caribou are more aggregated, but the error rate is unknown. Late June surveys were not conducted in 2015 or 2016, as caribou were too closely grouped to determine individual calving status.
Because density dependence can influence caribou and reindeer demographic rates (Pachkowski et al., 2013; Peeters et al., 2017), we accounted for the CAH population trend in some of our analyses. Between 1997 and 2019, ADFG conducted an early summer photocensus of CAH during 9 years, which were used to estimate herd abundance (Lenart, 2019, 2021; Supplementary Figure 1). Based on the abundance estimates, we calculated the annual exponential population growth rate (r) between field surveys (assuming r was constant) following the equation ln(NT/N0)/T, where N0 was the previous abundance estimate and NT was the abundance estimate after T years (Mills, 2013).
Characterizing forage and insect conditions
We used spatiotemporal models derived by Johnson et al. (2018, 2021) and data from Adams and Gustine (2018) to predict caribou summer forage biomass (g dry matter [DM]/m2), DN (g/100 g DM) and DE (kJ/g DM; all at 250 m resolution) each week during 16 June–31 August, 2002–2019. Briefly, Johnson et al. (2018, 2021) modeled spatiotemporal variation in field measurements of the quantity and quality of 6 forage species that comprise a majority of the summer diet of caribou on the North Slope (White and Trudell, 1980; Thompson and McCourt, 1981; Russell et al., 1993; Griffith et al., 2002): tussock cottongrass (Eriophorum vaginatum), water sedge (Carex aquatilis), Bigelow’s sedge (Carex bigelowii), Arctic lousewort (Pedicularis spp.), diamond-leaf willow (Salix pulchra), and Richardson’s willow (Salix richardsonii). At field plots, every 2 weeks across the growing season, the aboveground biomass of the current annual growth of each forage species was clipped, dried, and weighed, while representative samples of each species were analyzed for DN and DE. Forage biomass was estimated for each field plot during each sampling occasion as the sum of the biomass estimated for each target species. Nutrient digestibility and DM were estimated from digested residues obtained using an in vitro digestibility method with purified enzymes validated for caribou (VanSomeren et al., 2015). We calculated DN as the product of total nitrogen content (estimated with an elemental analyzer) and nitrogen digestibility, and DE was the product of the gross energy content (determined with an adiabatic bomb calorimeter) and digestible DM. We averaged DN and DE values across the species present on each plot during each sampling occasion.
We then modeled spatial and temporal variation in biomass, DE, and DN using the Normalized Difference Vegetation Index (NDVI) from eMODIS ALASKA data (Jenkerson et al., 2010), days before or after peak NDVI, vegetation type (Boggs et al., 2016), and distance to the coast, and used the models to generate weekly raster predictions of forage conditions across the CAH summer range each year (root mean square error for model predictions of biomass was 8.97 g/m2 DM, for DN was 0.26 g/100 g DM, and for DE was 1.79 kJ/g DM). Forage sampling occurred within four vegetation types (tussock tundra, herbaceous mesic, herbaceous wet, and dwarf shrub), so our predictions were constrained to only those areas (covering 75% of the summer range). Additionally, the models could not predict weekly forage conditions when NDVI values were classified as snow (Jenkerson et al., 2010). Because habitat used by the CAH during the calving season (1–15 June) is often still snow covered (Johnson et al., 2021), our forage predictions commenced at the start of the post-calving period (16 June) and continued through August. An example of weekly predicted DN in late June is depicted in Figure 1, showing the values in a year with early spring phenology (2015) relative to a year with late spring phenology (2018). During summer, forage that provides <1 g N/100 g DM or <9.5 kJ/g DM is likely to impair female caribou, as non-lactating female reindeer were unable to gain mass during summer below these levels (Thompson and Barboza, 2017).
We calculated an index predicting mosquito activity (MI) using the equation from Russell et al. (1993, 2013) that was developed for the neighboring Porcupine caribou herd. This index was based on temperature and wind speed conditions associated with increased mosquito activity in the field, and ranged from 0 to 1 where higher values indicated greater activity. The index equaled 1 when the temperature was ≥18°C and the wind speed was 0 m/s, while it was 0 when the temperature was <6°C or the wind speed was >6 m/s. This index has been highly effective at predicting shifts in caribou behavior (Cameron et al., 1995; Wilson et al., 2012; Johnson et al., 2021), but field personnel have noted that the index can be high even when there is little observed mosquito activity (B. Lenart, personal communication). We calculated MI for each caribou GPS location, and for coordinates within the summer range (see below) based on the date and time using spatial hourly temperature and wind speed data from the Modern-Era Retrospective Analysis for Research and Applications Version 23 (resolution 0.625° × 0.5°; Johnson et al., 2021).
Individual-level analyses of reproductive success and survival
We used data from GPS-collared adult females to examine the influence of an individual’s estimated summer forage and mosquito conditions on their subsequent reproductive success and survival. To quantify a caribou’s exposure to different summer habitat conditions, we first attributed all summer GPS locations with their predicted values of forage biomass, DE, and DN. For each animal-year data set, we then calculated the average biomass and DE at their locations across the summer (16 June–31 August) and average DN during early summer (16 June–15 July), when it typically exceeds the threshold for protein storage (Barboza et al., 2018; Johnson et al., 2021). We also calculated the average MI for GPS locations collected during the mosquito harassment period (25 June–15 July; Johnson et al., 2020).
We then investigated whether estimated summer forage or mosquito conditions (year t) were associated with parturition (classified as being parturient or not, 0/1) or late June calving success (classified as having a calf at heel in late June, 0/1) the following year (year t + 1). Only those females that were alive in the summer of year t and June of t + 1 were included in our analyses. We modeled parturition and late June calving success with generalized linear mixed models using a binomial link function with the “lme4” package (Bates et al., 2015) in R version 4.1.2 (R Core Team, 2021). In all models, we included a random effect for each individual female to account for repeated sampling of some animals. We initially also included a random effect for year, but the coefficient estimates were essentially zero and indicated singular fit, so we excluded this effect (Bates et al., 2015).
For both parturition and late June calving success, we ran several models that enabled us to test our hypotheses about the importance of early summer DN and mosquito season MI, relative to summer DE and summer biomass, on subsequent reproductive success (Table 1 provides the list of models). We also tested for an interaction between early summer DN and MI, given that forage DN only exceeds maintenance levels during the early summer when mosquito harassment is most prevalent (Barboza et al., 2018; Johnson et al., 2021). Additionally, we evaluated global models with and without the interaction term, and null models to confirm that the addition of forage and mosquito covariates improved model fit. Prior to running models, we assessed correlation coefficients (|r| < 0.7; Dormann et al., 2013; Supplementary Table 2) to ensure there were no issues were multicollinearity, and we standardized variables to facilitate convergence (Schielzeth, 2010). We used Akaike’s Information Criterion for small sample sizes (AICc; Burnham and Anderson, 2002) and model weights to rank models. We considered those models within 2 AICc values of our top model to be competitive, as long as they did not include uninformative parameters (i.e., those that include one extra parameter and make little to no improvement in the model log-likelihood but are ranked close to more parsimonious models with lower AIC values; Arnold, 2010; Leroux, 2019). For our top model(s) of each demographic rate, we inspected the residuals using diagnostics from the R package “DHARMa” (Hartig, 2021) and calculated the area under the receiver operating characteristic curve (AUC) as a measure of model performance. We wanted to include the population growth rate as a covariate in all reproductive models, but were missing data for 2019 (there has not been a more recent photocensus to estimate r), which would have excluded 59 observations of parturition and 52 observations of late June calving success. Using individual data from 2003 to 2018, however, we found that a model accounting for the population growth rate did not significantly improve the fit of the null model for either reproductive rate (parturition model with r relative to null model: ΔAICc = 1.28, β = −1.40, SE = 1.68, p = 0.41; late June calving success model with r relative to null model: ΔAICc = 1.51, β = 1.53, SE = 2.06, p = 0.46).
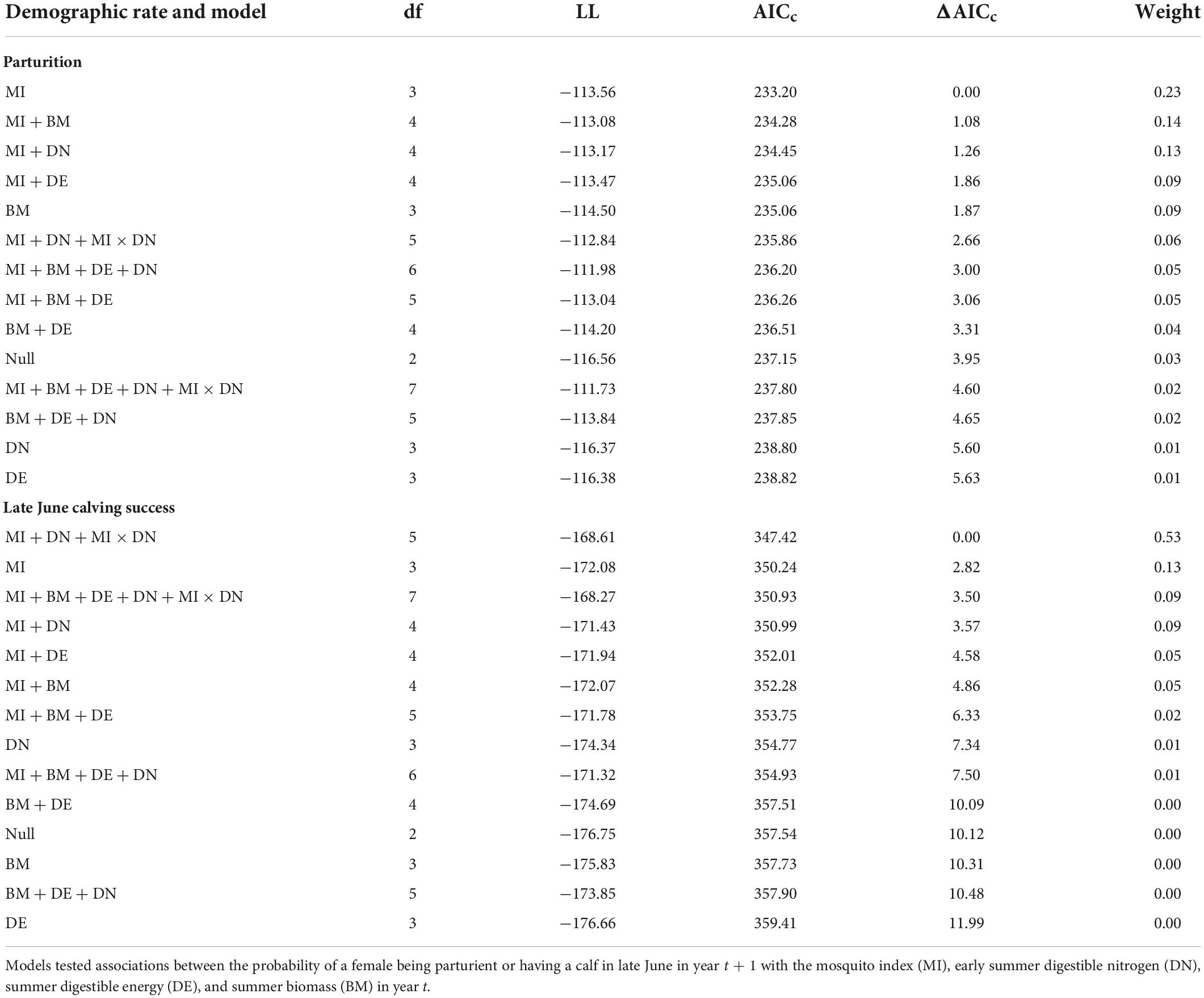
Table 1. Model selection criteria for individual-level models of female caribou parturition and late June calving success in the Central Arctic Herd, Alaska, 2003–2020.
We tested the influence of summer forage and mosquito conditions on individual adult female survival using Cox proportional hazard models (Cox and Oakes, 1984), which allows for staggered entry and right censoring. Consistent with our model set for evaluating reproductive success, we tested the same series of models (Table 3) to examine the influence of summer forage biomass and DE, early summer DN, and MI on adult female survival the remainder of the biological year (1 September–31 May; 9-months). Females that died during the summer (year t; n = 23) were excluded from our analyses, as their summer forage and mosquito metrics were not comparable to those that lived through August. We fit mixed effects Cox models using the R package “coxme” (Therneau, 2020) treating individual female and year as random effects. We used a recurrent study design (Fieberg and DelGiudice, 2009) and censored animals that dropped their collars or experienced collar malfunctions. Prior to running models, we ensured that variables were not collinear (|r| < 0.7). We investigated the proportional hazards assumption in top models by inspecting Schoenfeld residuals with respect to time (Schoenfeld, 1982) and plotted the marginal survival probabilities using the R package “rms” (Harrell, 2021). We did not include the population growth rate as a covariate in our survival analyses, given that we were missing data for 2019 (66 observations). Instead, we tested whether the inclusion of r improved the fit of the null survival model using data from 2003 to 2018, and found that it did not (survival model with r relative to null model: ΔAICc = 0.83, β = −1.16, SE = 1.03, p = 0.26).
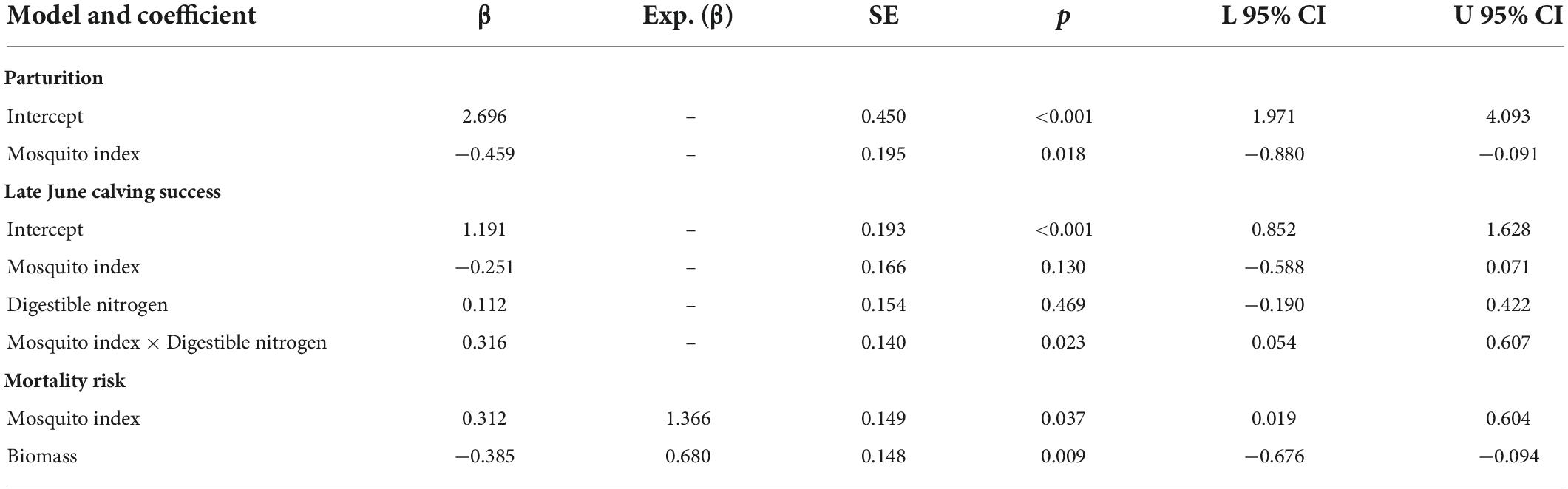
Table 2. Standardized coefficients from the top individual-level models of parturition, late June calving success, and hazards to 9-month survival (September–May) for adult female caribou in the Central Arctic Herd, Alaska, 2003–2020.
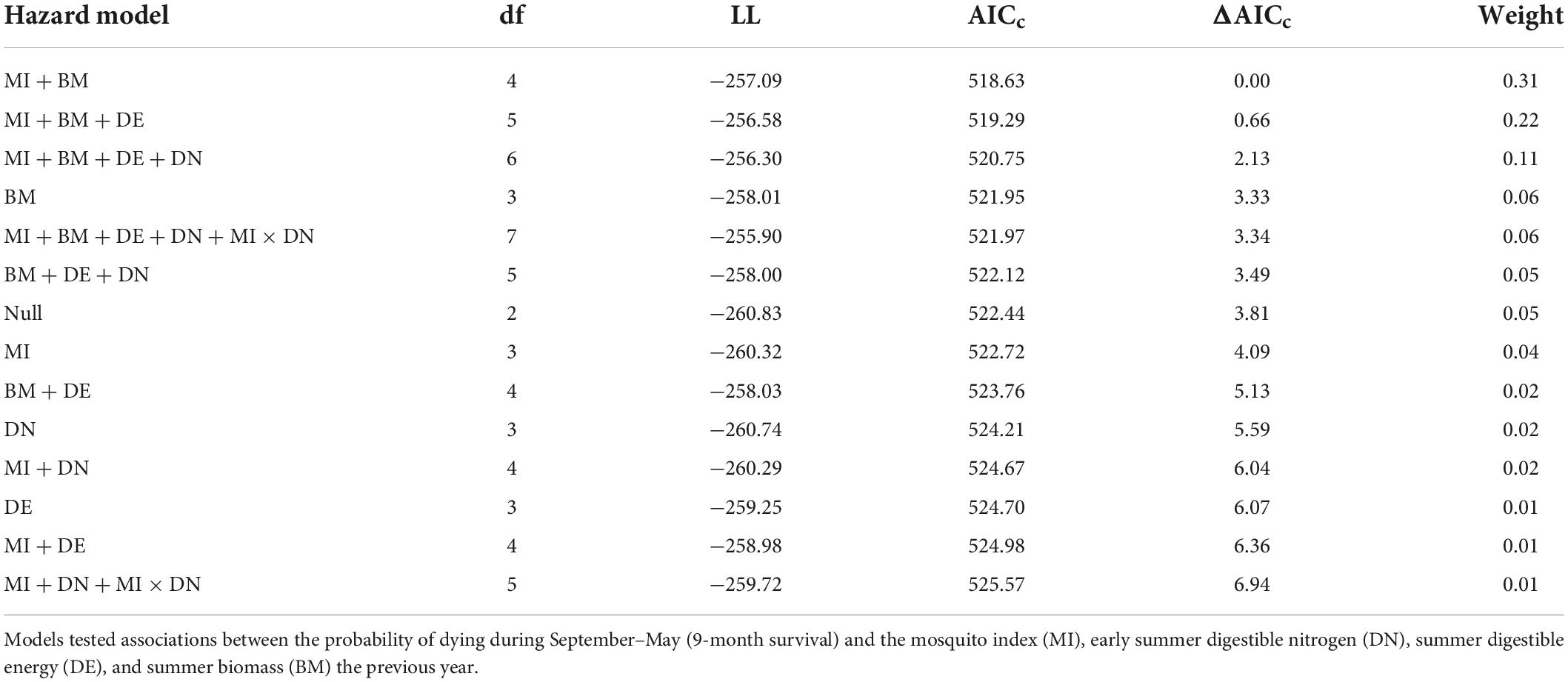
Table 3. Model selection criteria for individual-level models of hazards to survival of adult female caribou in the Central Arctic Herd, Alaska, 2003–2020.
Population-level analyses of reproductive success
Annual monitoring of VHF- and GPS-collared female caribou allowed us to also examine our hypotheses about the influence of estimated summer forage and mosquito conditions on reproductive success at the population-level. We first delineated polygons of three summer seasonal ranges for the CAH population using all GPS collar locations collected across the study period. We calculated the 90% kernel utilization distribution from locations collected during the “early summer” (16 June–15 July), the “mosquito season” (25 June–15 July), and across the “full summer” (16 June–31 August). Kernel distributions were generated based on 80% of the reference bandwidth (Kie et al., 2010) using the R package “adehabitatHR” (Calenge, 2006), and areas within the Arctic Ocean were removed (see an example of the early summer range polygon for the population in Figure 1). Within each of these seasonal range polygons, we randomly generated 1,000 locations. Each year (2002–2019), we attributed the random locations with their daily predicted biomass, DE, and DN values to characterize average population summer forage conditions. We then calculated average annual DN values for the population early summer range, and average annual biomass and DE values for the population full summer range, during the relevant time periods. To assess annual variation in conditions conducive to mosquito activity, we attributed the 1,000 random locations within the population mosquito range with their MI every 2 h (the sampling period of most GPS collars) based on their spatial coordinates, date, and time. We then calculated the annual average MI for the population mosquito range during the mosquito season.
We assessed the influence of forage and mosquito conditions on reproduction at the population-level using annual monitoring data from all CAH collared females (GPS and VHF; Supplementary Table 1). Annual aerial observations of collared females by ADFG were used to estimate the proportion of females ≥4 years old that were parturient and the proportion that had a calf at heel in late June (Lenart, 2020, 2021), metrics which are used in part to index herd performance for management. To test our hypotheses, we used logistic regression models (generalized linear models with a binomial link function) to examine whether the annual number of females that were parturient or had a calf in late June in year t + 1 (relative to those that were surveyed; 2003–2020) were associated with average early summer DN, summer biomass, summer DE, or the mosquito season MI in year t (2002–2019). Given the small sample size (n = 16 years for parturition models and n = 15 years for late June calving success due to missing surveys), we ran separate models for each forage and mosquito variable to assess their support. We also tested models that included MI or the forage variables along with late June calving success from the previous year to account for potential reproductive carryover effects (Cook et al., 2021). To assess whether density effects were evident, we tested whether the estimated annual population growth rate in year t was associated with either annual parturition or late June calving success in year t + 1. Given our small sample sizes, and missing data for 2019, we assessed the relationship between r and the reproductive rates in separate models.
Results
Individual-level analyses of reproductive success and survival
We obtained an average of 895 GPS locations/female/summer which were used to calculate individual average summer forage and mosquito metrics. Estimated summer forage components at caribou locations varied within and across different growing seasons (Figure 2), and the average forage and mosquito metrics for different individuals varied widely within and across years (Supplementary Figure 2). For GPS-collared females, average annual summer biomass at their locations was 36.07 g/m2 DM (SD = 5.66), summer DE was 10.27 kJ/g DM (SD = 0.31), summer DN was 1.03 (SD = 0.10), early summer DN was 1.26 g/100 g DM (SD = 0.06), and the mosquito season MI was 0.16 (SD = 0.07).
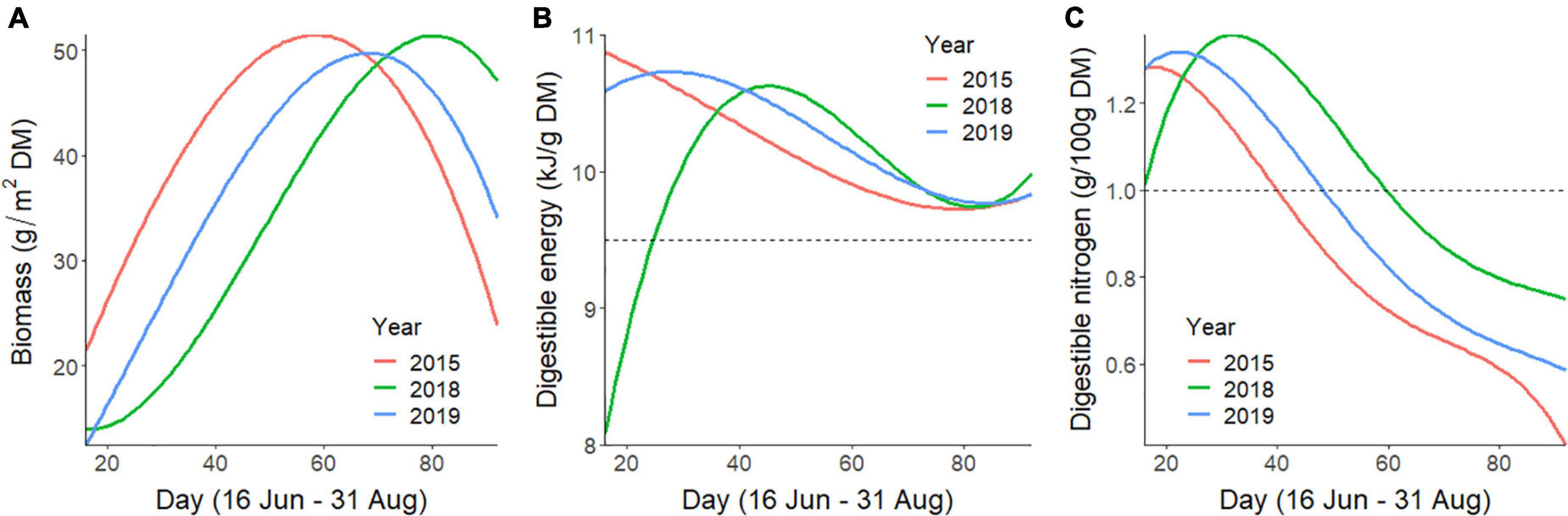
Figure 2. Predicted temporal trends of daily average values of forage (A) biomass, (B) digestible energy, and (C) digestible nitrogen at caribou locations across the summer (16 June–31 August; days 16–92) for the Central Arctic Caribou Herd, Alaska. Average forage values were smoothed and are depicted for 2015, 2018, and 2019, which represent years with early, late, and moderate summer phenology, respectively. The horizontal dotted reference lines for digestible energy and nitrogen indicate thresholds identified for non-lactating, conspecific, female reindeer to gain mass during summer.
We obtained data on parturition from 156 individual caribou, for a total of 344 animal-years, that had accompanying forage and mosquito data the previous summer. We obtained late June calving success data from 145 individual caribou, for a total of 299 animal-years, that had accompanying forage and mosquito data the previous summer. For GPS-collared females, the mean probability of being parturient was 0.89 (SE = 0.02), and the mean probability of having a calf in late June was 0.69 (SE = 0.03). We monitored the survival (1 September–31 May) of 185 adult females, for a total of 416 animal-years, that were GPS collared the previous summer. Over the 9-month monitoring period, the average probability of adult female survival was 0.89 (SE = 0.02).
At the individual-level, the top parturition model included only MI (Table 1). As hypothesized, greater MI in year t was associated with a reduced probability of parturition the subsequent summer (Figure 3; Table 2; unstandardized β = −6.46, SE = 2.74), with greater model uncertainty at higher levels of MI. Four other models were within 2 AICc values of the top model, but three of those included MI with a single forage parameter that was uninformative (Leroux, 2019). The other competitive model included only biomass, but with less than half the weight of the top model (Table 1), having an unexpected relationship that greater forage biomass in year t was associated with a lower probability of parturition the following year (unstandardized β = −0.08, SE = 0.04). The AUC for the top parturition model was 0.95 indicating it performed well.
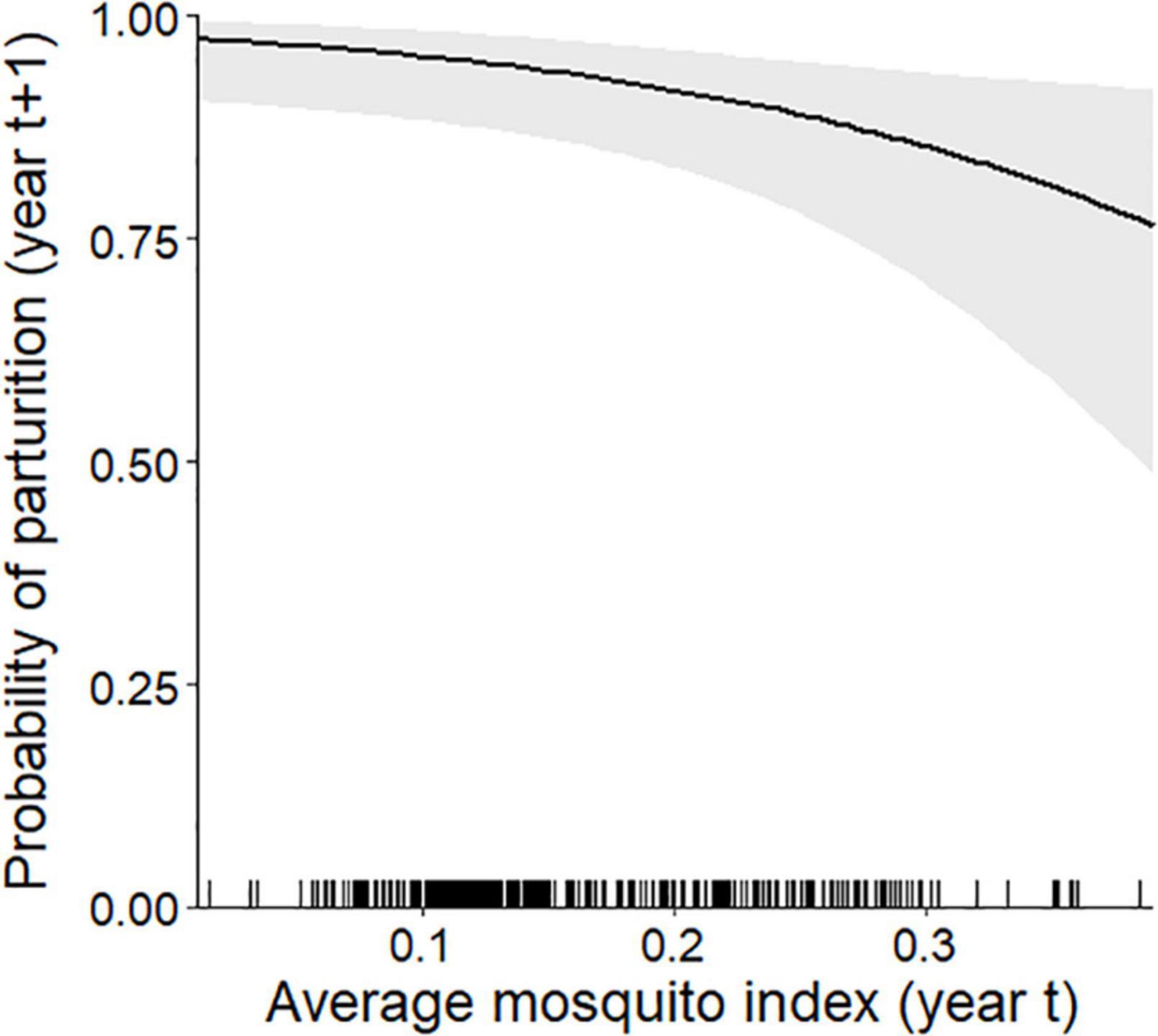
Figure 3. Individual-level probability (and 95% confidence interval) of an adult female caribou being parturient in year t + 1 modeled as a function of the average mosquito index during the mosquito harassment season the previous summer (year t), Central Arctic Herd, Alaska, 2003–2020. The rug plot along the bottom of the figure depicts the distribution of data.
When we assessed the influence of forage and mosquito conditions on the probability of having a calf in late June, we found that the top model included MI, DN, and the interaction between MI and DN (Tables 1, 2); no other models were competitive. As hypothesized, individuals experiencing higher MI had a reduced probability of having a calf the following year (Figure 4A), while those experiencing higher DN had a greater probability of having a calf (Figure 4B). These variables interacted with one another such that greater MI more strongly reduced the probability of late June calving success when forage DN was lower (Figure 4C), with estimates becoming more uncertain at very high levels of MI. The AUC for the top calving model was 0.87 indicating it performed well.
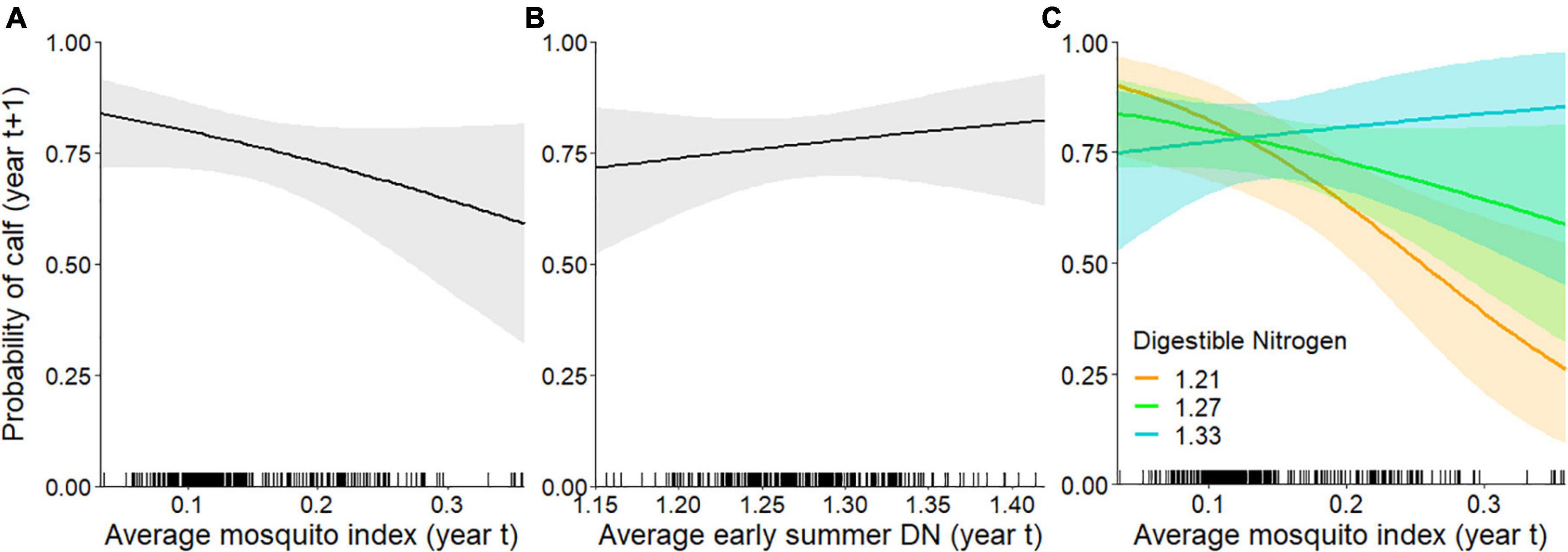
Figure 4. Individual-level probability (and 95% confidence interval) of an adult female caribou having a calf in late June (year t + 1) modeled as a function of the previous summer’s (A) mosquito index during the mosquito harassment season, (B) early summer digestible nitrogen (DN), and (C) the interaction between the mosquito index and DN (DN is depicted at 10, 50, and 90% quantiles of observed values), Central Arctic Herd, Alaska, 2003–2020. The rug plot along the bottom of the figure shows the distribution of data.
The top model of 9-month (September–May) adult female survival included MI and summer biomass (Table 3). Greater MI was associated with reduced adult survival (Figure 5A) and greater biomass was associated with increased survival (Figure 5B; Table 2), with greater uncertainty in model estimates at very high values of MI and very low values of biomass. One other model was within 2 AICc values of the top model (Table 3). It included MI and biomass, along with a positive effect of DE on survival, but this parameter was uninformative (Leroux, 2019). As expected, DN and an interaction between DN and MI were not associated with adult survival (Table 3).
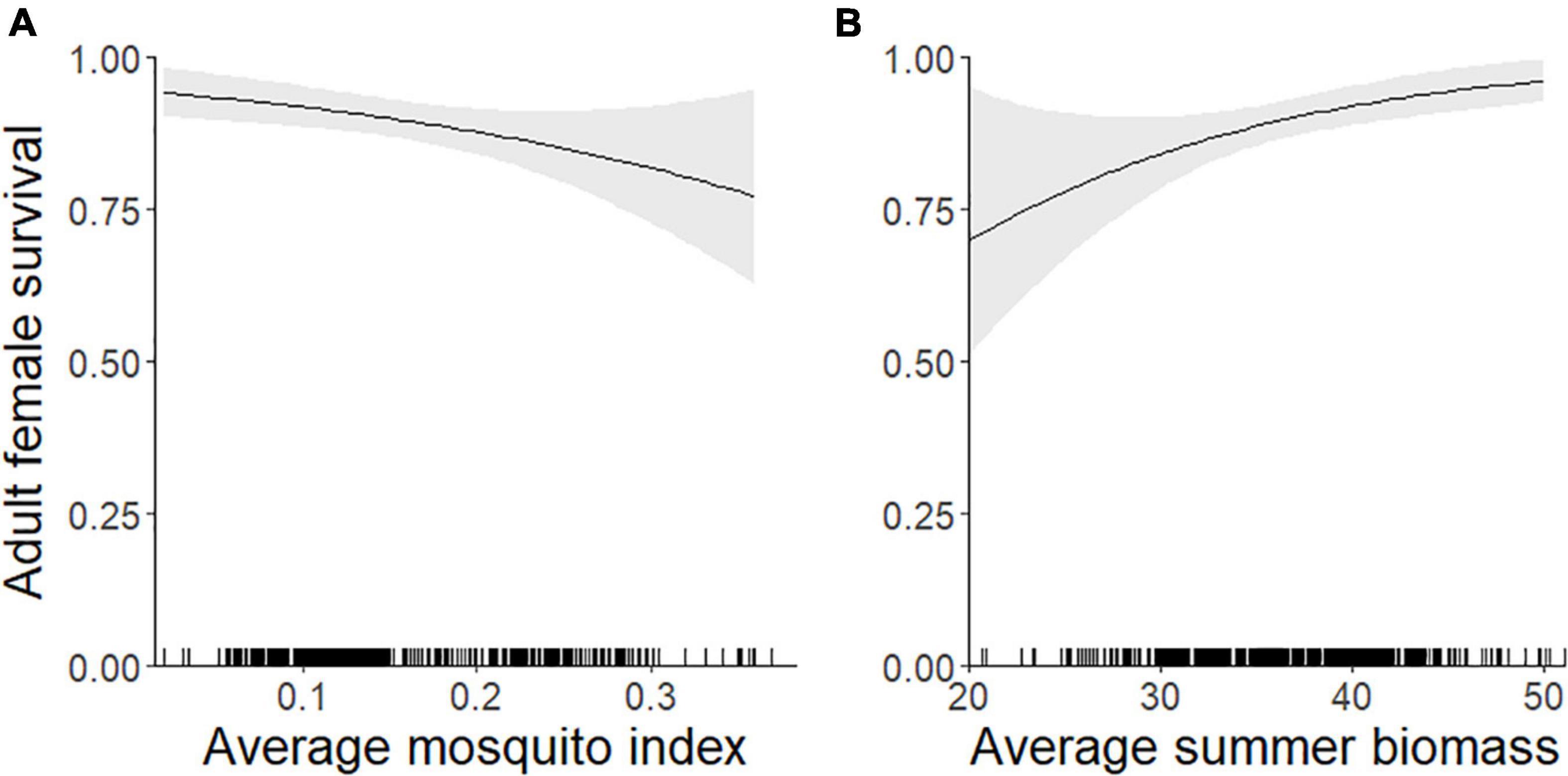
Figure 5. Individual-level probability (and 95% confidence interval) of an adult female caribou surviving 9-months (1 September–31 May) modeled as a function of the previous summer’s (A) mosquito index during the mosquito harassment season, and (B) estimated forage biomass (g/m2), Central Arctic Herd, Alaska, 2003–2020. The rug plot along the bottom of the figure depicts the distribution of data.
Population-level analyses of reproductive success
At the population-level, the average annual proportion of females that were parturient was 0.89, ranging from 0.75 to 0.98 (Figure 6A). The average annual proportion of females observed with calves in late June was 0.74, ranging from 0.52 to 0.91 (Figure 6B). The top model for parturition included MI and previous late June calving success with 56% of the model weight; the second top model included only MI with 37% of the weight (Table 4). Annual parturition rates (year t + 1) were negatively associated with the previous summer’s mosquito season MI (Figure 7A; Table 5), mirroring results from the top model of the individual-level analyses. Parturition rates were also lower when the previous year had high late June calving success (Table 5), suggesting a reproductive carryover effect. The top model of late June calving success included only MI, with 70% of the weight, with lower predicted calving success following summers with greater MI (Figure 7B; Table 5). Models that included a negative relationship with MI or a positive relationship with DN, with or without previous calving success, carried all of the weight in the model set (Table 4). The annual estimated population growth rate was not associated with subsequent reproductive rates (parturition model with r relative to null model: ΔAICc = 2.52, β = 0.47, SE = 1.12, p = 0.67; late June calving success model with r relative to null model: ΔAICc = 2.37, β = 0.67, SE = 1.07, p = 0.53).
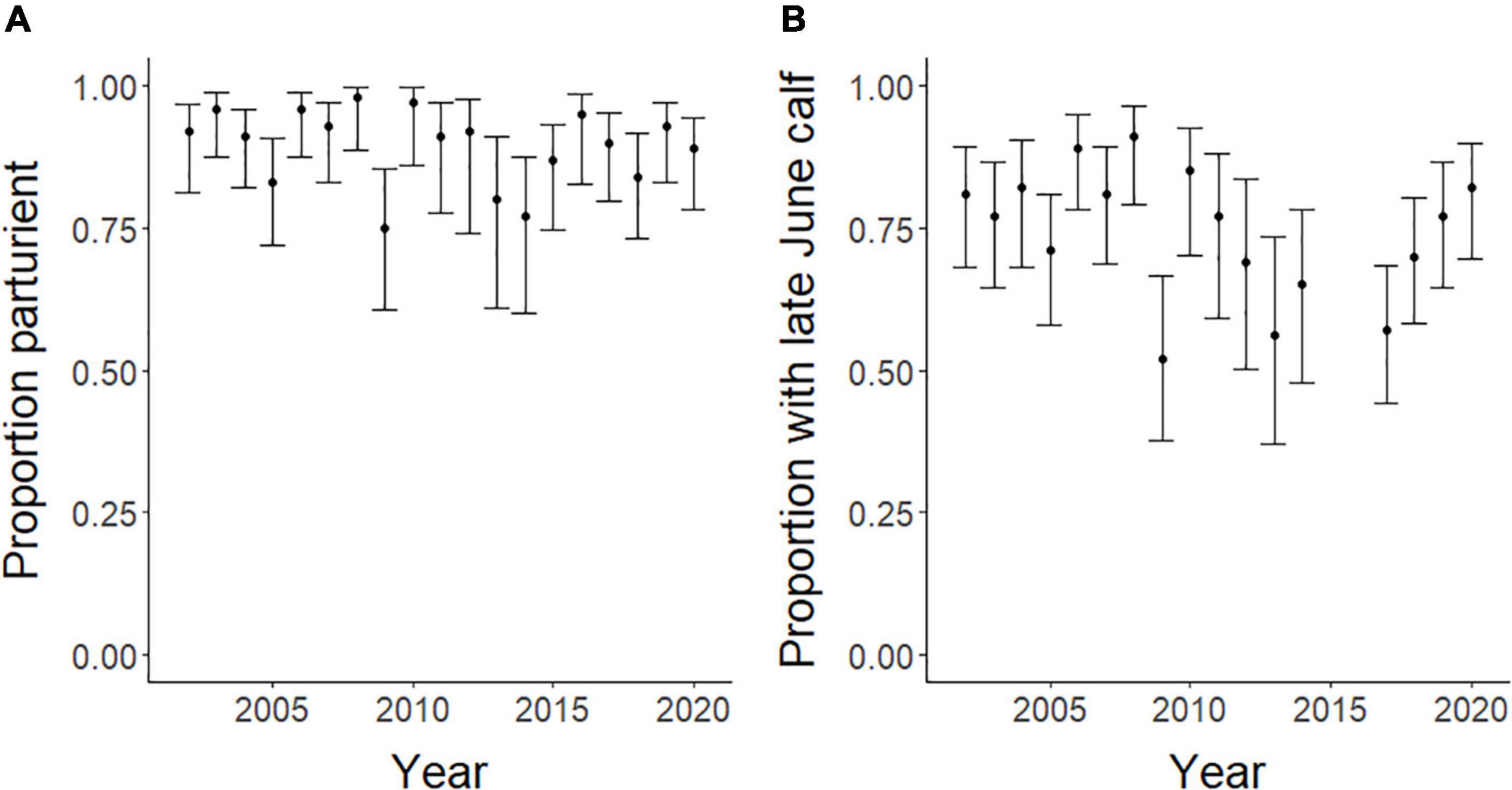
Figure 6. Annual population-level proportion of female caribou that were parturient or had a calf at heel in late June (and 95% confidence intervals) in the Central Arctic Herd, Alaska, 2002–2020.
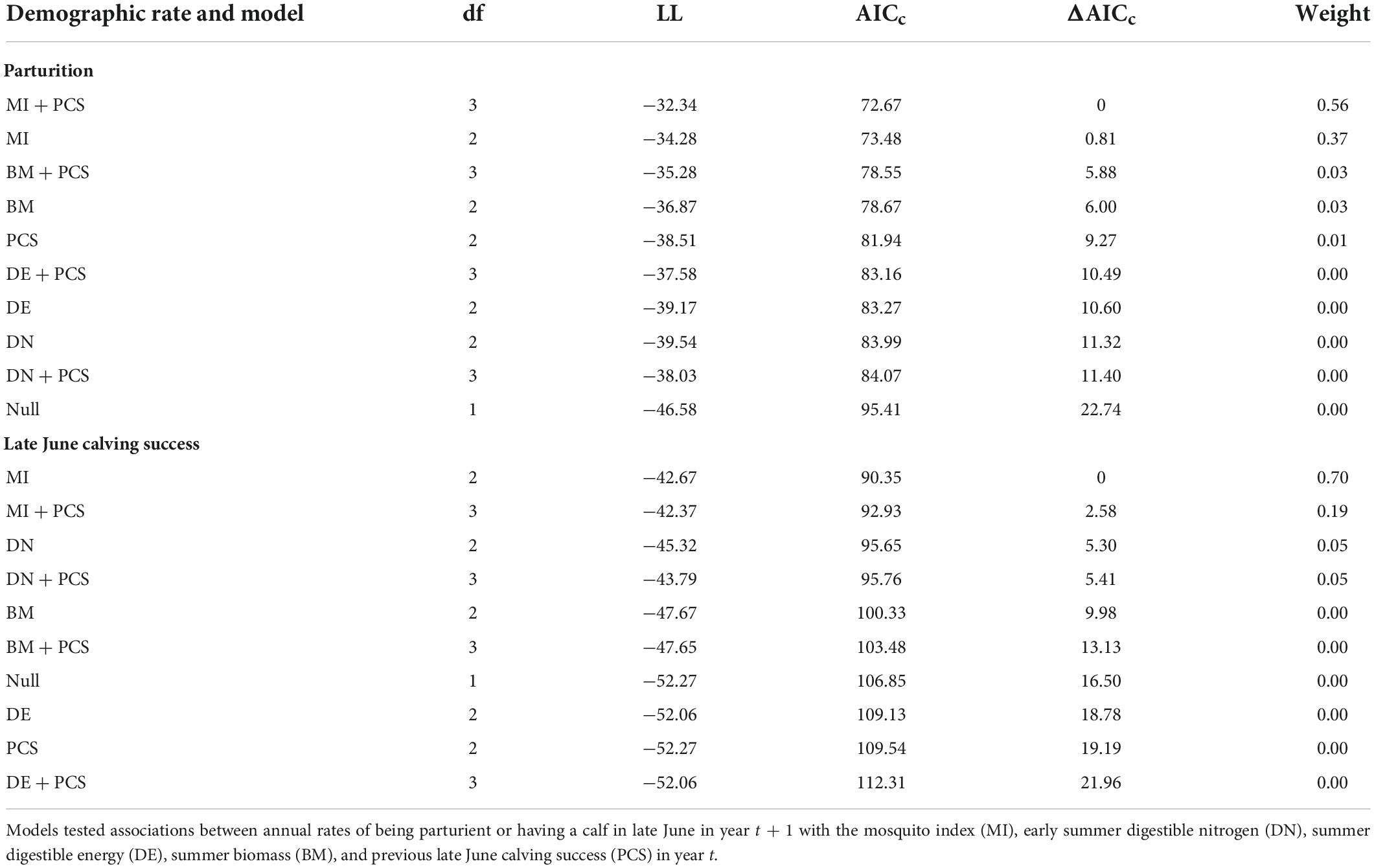
Table 4. Model selection criteria for population-level models of parturition and late June calving success of adult female caribou in the Central Arctic Herd, Alaska, 2002–2020.
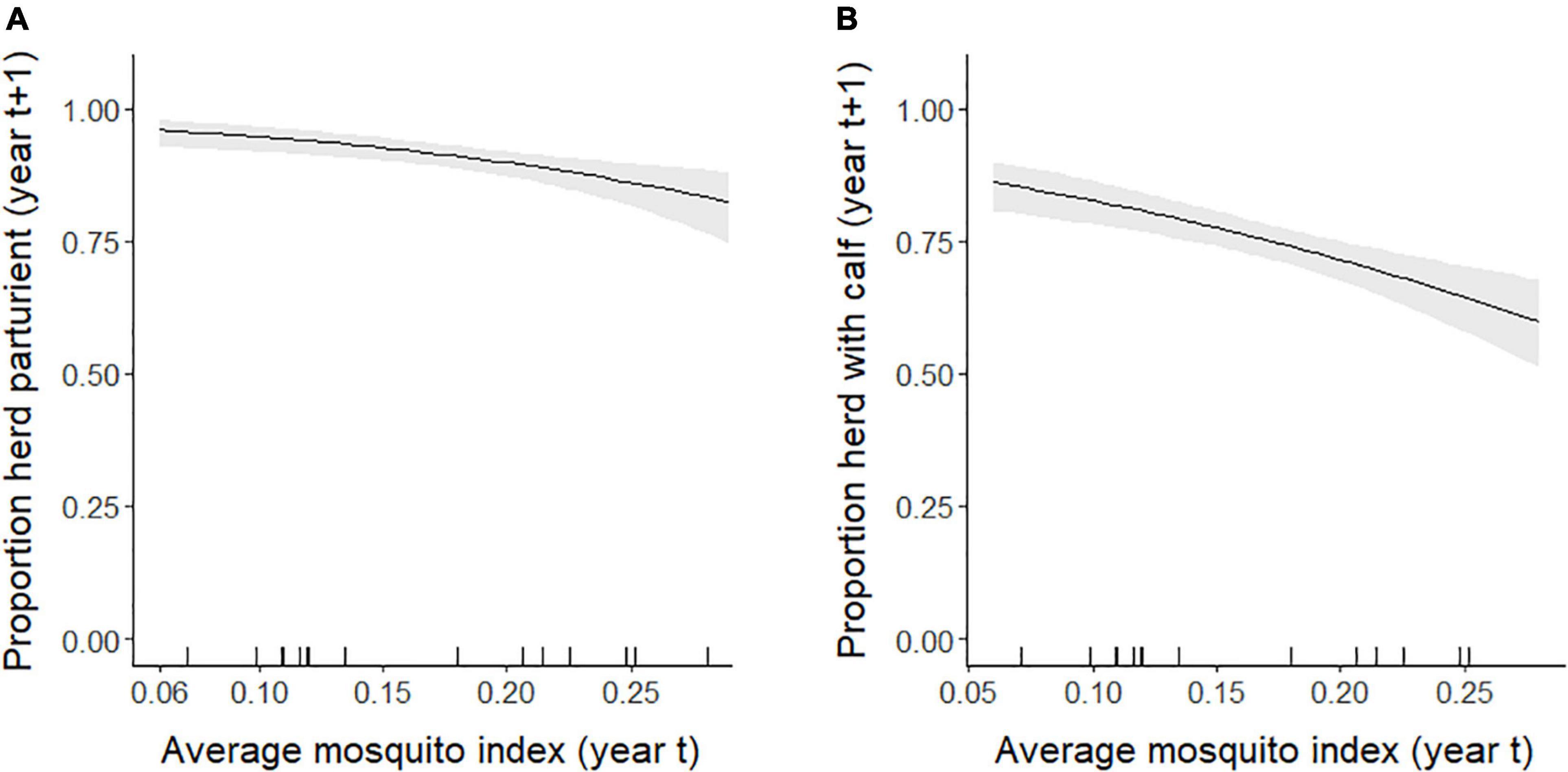
Figure 7. Annual population-level (A) parturition rates (and 95% confidence intervals) of female caribou modeled as a function of the mosquito index during the previous summer, and (B) late June calving success rates (and 95% confidence intervals) modeled as a function of the mosquito index the previous summer, Central Arctic Herd, Alaska, 2002–2020. The rug plot along the bottom of the figure depicts the distribution of data.
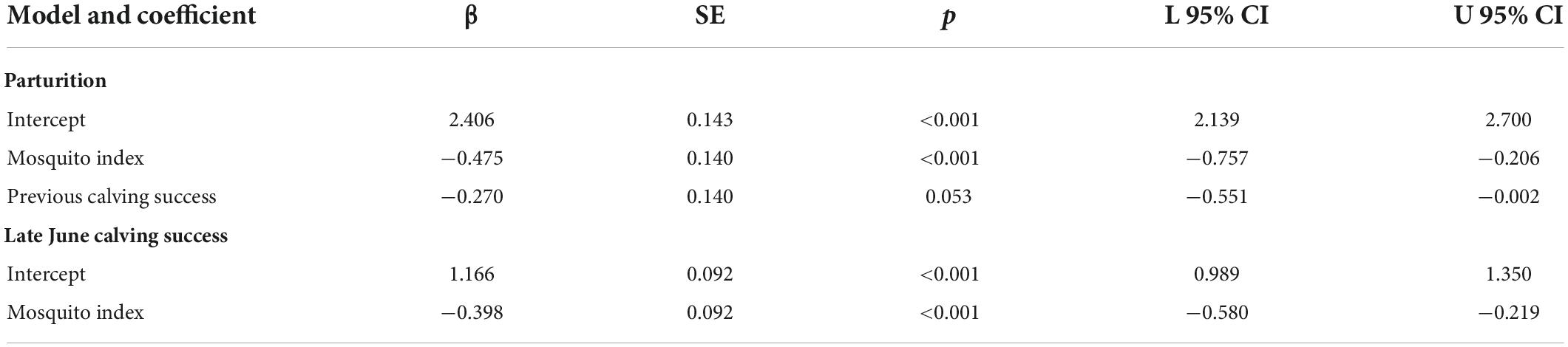
Table 5. Standardized coefficients from the top population-level models of parturition and late June calving success of adult female caribou in the Central Arctic Herd, Alaska, 2002–2020.
Discussion
Our results indicate that summer habitat conditions influence CAH caribou reproductive success and survival, generally supporting our hypotheses. While the value of summer foraging opportunities for caribou has long been recognized (White, 1983), difficulty in assessing nutritional components across expansive Arctic landscapes has hampered our understanding of its impact on caribou demography. We found that greater forage DN was associated with increased subsequent late June calving success, while greater forage biomass was associated with increased September–May adult survival, highlighting the likely differential impacts of forage quality and quantity on caribou demographic rates. Importantly, our findings not only illustrate the relevance of the summer range for providing caribou with nutritious and abundant forage, but also for exposing caribou to mosquito harassment. As predicted, we found that greater MI was associated with reductions in both the reproductive success and survival of adult female caribou, and that summers with both higher MI and lower DN synergistically reduced late June calving success the following year. Given that warmer, longer growing seasons are associated with both higher MI and lower DN (Culler et al., 2015; Zamin et al., 2017; Johnson et al., 2018), these factors may covary and occur more frequently in the future, suggesting that changing climate conditions have the potential to have compounding effects on caribou demographic rates.
As hypothesized, greater mosquito harassment depressed subsequent reproductive success and survival. Indeed, MI was associated with reductions in reproductive success in both our individual- and population-level analyses, and indicated a reduction in September–May adult survival by up to 16% over the observed range of variation. Arctic caribou strongly alter their behavior in response to mosquito harassment, as they increase their energy expenditure, reduce foraging time, and move to barren or snow-covered areas (White et al., 1975; Russell et al., 1993; Johnson et al., 2021). These extreme responses suggest that harassment must be highly consequential to caribou fitness, but the specific effects on caribou demographic rates have been unknown (Joly et al., 2020a). Harassment coincides with the time of year when caribou are in their poorest body condition (Cook et al., 2021) and need to replenish reserves, which may make them particularly vulnerable to insects. Our findings underscore the likely relevance of mosquito harassment for influencing Arctic caribou body condition and demographic rates, and the need to account for this factor in future monitoring and investigations. While we used a temperature- and wind-based index of mosquito activity (Russell et al., 1993, 2013), we suspect that an index incorporating additional variables would have greater accuracy and predictive ability. For example, Witter et al. (2012) found that predictions of mosquito activity were improved by incorporating the cumulative number of growing degree days, which informed the timing of mosquito emergence.
Our individual-level analysis suggested that the probability of having a calf in late June increased following years when caribou had access to greater early summer forage DN. As capital breeders, caribou rely on maternal body stores of nitrogen acquired the previous summer for fetal development and early lactation (Barboza and Parker, 2008; Taillon et al., 2013; Barboza et al., 2020). Because Arctic caribou consume protein poor diets most of the year, primarily consisting of lichen and senesced vegetation (Russell et al., 1993; Joly et al., 2015), we assume that DN stored during the early summer must be conserved for subsequent reproduction, a mechanism supported by our results. Adequate forage DN, however, is only available to the CAH during the first half of the summer (until∼mid–July; Barboza et al., 2018; Johnson et al., 2021), which overlaps with the period of mosquito harassment (∼25 June–15 July). Because higher MI depresses caribou selection for DN (Johnson et al., 2021) we hypothesized that summers with both higher MI and lower DN would have a compounding effect on reproductive success. Individual data on late June calving success supported this expectation, as caribou that experienced both higher MI and lower DN exhibited a decline in their probability of having a calf at heel in the following year. Because available forage DN tends to be depressed during earlier, warmer summers (Zamin et al., 2017), the same conditions that enable earlier, more intense periods of mosquito harassment (Culler et al., 2015), these effects could coincide more frequently in the future. Earlier periods of mosquito harassment also have the potential to overlap with the calving season, when parturient cows and calves have limited mobility and newborns are particularly vulnerable (Culler et al., 2015). Although our hypothesis about the interaction of MI and DN was supported by data on late June calving success, it was not supported for parturition. We suspect this is because the probability of being parturient in the CAH was high (89%), while the probability of having a calf in late June was significantly lower (69%), exhibiting more of the variation in early summer reproductive success. A caribou with low protein stores may birth a calf, but not be able to support it through early lactation (Barboza and Parker, 2008).
While higher forage DN was associated with an increase in subsequent late June calving success, higher forage biomass was associated with an increase in caribou survival, providing support for our hypothesis. Indeed, the September–May survival of adult females was higher following summers with greater forage biomass, which we expect enabled them to gain more mass and fat (Chan-McLeod et al., 1994, 1999; Parker et al., 2009). Greater mass and fat have been associated with increased survival in other caribou and reindeer populations (Parker et al., 2009; Albon et al., 2017), as well as in other large herbivores (Cook et al., 2004; Monteith et al., 2014; Kautz et al., 2020). Surprisingly, however, our individual-level analysis suggested that greater summer biomass may also be negatively associated with subsequent parturition (lowest competitive model with ΔAICc of 1.87). We speculate that greater forage biomass may increase calf survival in the current year (Tveraa et al., 2013), which could have carryover effects on the ability of a female to reproduce in consecutive years (Parker et al., 2009; Newby and DeCesare, 2020; Cook et al., 2021). We did not have adequate data to include the previous year’s calving success in our individual-level models (we were missing data for ∼35% of our observations), but we did include it as a covariate in our population-level models of parturition. We found that previous late June calving success (year t) did indeed reduce parturition in the subsequent year (year t + 1), lending support to our speculation.
Although caribou demographic rates were related to forage DN and biomass, we found no relationships with DE. We had hypothesized that greater DE would increase adult female survival, similar to biomass, as higher DE has been associated with greater mass and fat gain in caribou and reindeer (Chan-McLeod et al., 1994). Given the importance of DE to caribou and other ungulates (Cook et al., 2004; Tollefson et al., 2010; Denryter et al., 2022), we suspect that the lack of relationship between DE and survival was because most forage species within the CAH summer range have DE values exceeding the threshold for energy storage (Barboza et al., 2018; Johnson et al., 2021), so DE does not appear to be limiting. In a post hoc analysis, we assessed associations between CAH demographic rates and early summer DE and biomass, along with early summer DN, as a check that our hypotheses about the relevant periods for different forage components for CAH were appropriate. Our results for parturition and late June calving success were the same; the top model of parturition only included MI, while the top model of late June calving success included MI, early summer DN, and their interaction (Supplementary Table 3). Adult survival exhibited no strong relationships with early summer forage components, as the null was the top model (Supplementary Table 4).
Studies on caribou and other ungulates commonly assess demographic patterns in relation to satellite-derived proxies of vegetation growth, like NDVI (Tveraa et al., 2013; Stoner et al., 2016; Fauchald et al., 2017; Lukacs et al., 2018), but few studies assess direct links to the nutritional components of vegetation that animals select (but see Long et al., 2016; Proffitt et al., 2016). By modeling different forage components at landscape scales, we were able to investigate the separate effects of forage quality and quantity on caribou reproductive success and survival, finding that greater quantity was associated with higher survival while greater quality was associated with higher late June calving success. Such information may be useful in diagnosing the underlying mechanisms of depressed demographic rates in the future. Interestingly, even within the same summer, we observed high variation in the estimated forage and mosquito conditions of individual females depending on their habitat-use patterns (Supplementary Figure 2). This highlights the importance of understanding how variation in habitat use confers animal fitness (Gaillard et al., 2010; DeCesare et al., 2014), especially under changing environmental conditions. Importantly, the habitat-demography relationships we identified also largely mirror our recent findings on how caribou select for forage and mosquito conditions, especially at fine spatial scales. Using resource selection function (RSF) models, Johnson et al. (2021) found that CAH female caribou select areas with higher DN, DE, and biomass, but lower MI, similar conditions associated with increased late June calving success and survival. Collectively, these results suggest that summer habitat selected by the CAH confers fitness benefits (Fretwell and Lucas, 1970), reinforcing the utility of RSFs for identifying habitat conditions important to population performance (Aldridge and Boyce, 2007).
The links we found between the CAH’s demographic rates and their summer range conditions may have implications for caribou populations in the future. Climate change is generally expected to result in earlier, warmer Arctic summers (Lader et al., 2017; Severson et al., 2021) which has the potential to increase the severity and duration of mosquito harassment (Culler et al., 2015; Koltz and Culler, 2021), increase forage biomass (Elmendorf et al., 2012; Doiron et al., 2014), and reduce forage DN (Zamin et al., 2017; Johnson et al., 2018; Richert et al., 2021). These factors are likely to have opposing effects on caribou demographic rates, but their relative magnitudes are unknown. For example, our results suggest that greater mosquito harassment may reduce caribou reproductive success and survival, particularly given the compounding effects of lower DN on late June calving success, but that greater forage biomass may offset declines to survival. As a result, the net effects of these different factors on caribou population growth are currently unclear. Adult female survival is the demographic rate with the highest elasticity in ungulate populations, but because it is often relatively stable, reproductive success, which is more variable, often drives temporal variation in population growth (Gaillard et al., 2000). In practice, the relative influence of these vital rates may depend upon the population trajectory, as ungulate declines may often be driven by depressed adult survival, while stable and increasing populations may be more commonly driven by variation in reproduction (Johnson et al., 2010; Manlik, 2019). Understanding the collective influence of these different summer factors on CAH population performance will require adequate data on additional vital rates (i.e., juvenile survival), information that is currently lacking. Fauchald et al. (2017) found that declines in barren-ground caribou populations occurred during summers with greater vegetation productivity but was uncertain about the mechanism driving this relationship. In our study, individual female caribou that experienced greater summer biomass also experienced greater insect harassment (Pearson’s correlation = 0.43, t = 9.71, p < 0.001) and reduced forage DN (Pearson’s correlation = −0.45, t = −10.21, p < 0.001), conditions that may depress some caribou demographic rates and potentially influence population trends.
While we detected relationships between caribou demographic rates and extrinsic summer habitat conditions, we did not detect clear relationships with the intrinsic condition of the population growth rate or previous late June calving success. Negative density dependence is well recognized to influence the dynamics of caribou and other ungulate populations (Bonenfant et al., 2009; Ahrestani et al., 2013), but despite the variation in population trend during the study (Supplementary Figure 1), we failed to detect an effect of r on reproduction or survival. Caribou abundance was estimated from photocensus counts on their early summer range, but during different times of the year the herd regularly overlaps with animals in the neighboring Teshekpuk and Porcupine caribou herds (Prichard et al., 2020), potentially confounding the relationship with summer density. Additionally, only nine population estimates occurred during the 20-year study period, hampering our ability to assess the influence of annual variation in r. In our population-level models, we tried to account for reproductive carryover effects by including the previous year’s late June calving success. In our top parturition model, we found greater late June calving success in the previous year was associated with reduced parturition in the current year, presumably due to a reproductive carryover effect (Cook et al., 2021), but there was no clear relationship with late June calving success in the current year. We suspect that late June calving success is a poor index of the full reproductive costs of rearing calves until they are weaned, which likely accounts for the mixed responses to this variable.
Our analyses were useful for testing hypotheses about the influence of summer habitat conditions on caribou demographic rates, but have several limitations that are important to acknowledge. For example, our forage models were based on only six key forage species, where their average biomass, DN and DE were estimated for each pixel of the CAH summer range, with associated error (Johnson et al., 2018, 2021). Because caribou are highly selective foragers (Denryter et al., 2017) we expect that their use of different species varies across the summer (Russell et al., 1993; Ehlers et al., 2021), an aspect we had no information on and could not incorporate into our calculations. Additionally, our forage predictions were limited to those landcover types that were sampled in the field, which excluded low shrub and sparsely vegetated areas that are certainly used by caribou in summer. Increasing the precision of our forage models, accounting for temporal variation in plant selection, and obtaining abundance and quality estimates across additional vegetation types, would all improve our forage estimates and strengthen our inferences about their relative importance. While we investigated the influence of conditions conducive to harassment by mosquitos, we did not evaluate the potential effects of harassment by oestrid flies, which occurs during mid- to late-summer. In other caribou and reindeer populations, oestrid flies elicit strong behavioral responses (Hagemoen and Reimers, 2002; Witter et al., 2012) and reduce body condition and pregnancy rates (Hughes et al., 2009). Our weather-based index of oestrid fly harassment, however, has been shown to have little influence on caribou behavior (Johnson et al., 2021), leading us to suspect that the index is inadequate for detecting meaningful variation in fly activity. With a more reliable index, it will be important to assess the relative and collective influence of mosquito and fly harassment on caribou demography in future research.
While our study examined correlations between summer habitat conditions and caribou reproductive and survival rates, it is important to recognize that it does not demonstrate causation, nor does it account for other factors likely to be influential during summer or the remainder of the year. For example, predation, winter nutrition, winter weather, human disturbance, harvest, and other parasites have all been demonstrated or hypothesized to influence demographic rates in other barren-ground caribou populations (Boulanger et al., 2011; Pachkowski et al., 2013; Kaluskar et al., 2019; Plante et al., 2020). Our research provides support for hypotheses about the role of summer conditions in influencing caribou demographic rates but additional research will be needed to comprehensively elucidate the suite of factors potentially influencing CAH demography, their relative effects, and how they may be altered in the future by changing climate conditions.
Despite the limitations, our work adds support to a growing body of evidence emphasizing the importance of summer nutrition for shaping demographic performance in ungulates (Cook et al., 2013; Monteith et al., 2014; Proffitt et al., 2016; Schrempp et al., 2019; Cook et al., 2021, Proffitt et al., 2021), and uniquely illustrates how mosquito harassment, and its interaction with summer forage resources, may also influence demographic rates. Indeed, our findings suggest that summer foraging opportunities for Arctic caribou are a function of both forage availability and accessibility, and to unravel the mechanisms affecting caribou demography both aspects need to be considered. Given that changing climate conditions are altering caribou forage quantity and quality (Zamin et al., 2017; Richert et al., 2021) while also altering insect abundance and phenology (Culler et al., 2015), it will be important to monitor changes in these habitat factors. Such information will be critical for clarifying the influence of changing summer conditions on caribou demographic rates and population trends, and ultimately, to predict how they may be altered in the future.
Data availability statement
The datasets presented in this study can be found in online repositories. The names of the repository/repositories and accession number(s) can be found below: Data used to develop the forage models are available at https://doi.org/10.5066/F7JQ106W. Caribou locations are restricted from public access by Alaska Department of Fish and Game, Alaska Statue 16.05.815. Population-level demographic data, habitat and insect information are available through the sources cited in the text.
Ethics statement
This animal study was reviewed and approved by Alaska Department of Fish and Game, Animal Care and Use Committee.
Author contributions
HJ: conceptualization, data collection, analysis, and writing—original draft. EL, DG, and LA: data collection, funding acquisition, and writing—review and editing. PB: conceptualization and writing—review and editing. All authors contributed to the article and approved the submitted version.
Funding
This project was part of the USGS Changing Arctic Ecosystems Initiative and was supported by funding from the Species and Land Management Programs of the USGS Ecosystem Mission Area and Alaska Department of Fish and Game.
Acknowledgments
We thank ConocoPhillips and Exxon Mobil for providing caribou location data, and C. Swingley and A. Prichard for data processing. We appreciated comments and suggestions from J. Severson, J. Pearce, L. Parrett, J. Caikoski, C. Krenz, and reviewers that greatly improved our analyses and manuscript. We also thank members of the North Slope Borough for their constructive comments on this and other USGS research activities in Arctic Alaska. Any use of trade, firm, or product names is for descriptive purposes only and does not imply endorsement by the U.S. Government.
Conflict of interest
The authors declare that the research was conducted in the absence of any commercial or financial relationships that could be construed as a potential conflict of interest.
Publisher’s note
All claims expressed in this article are solely those of the authors and do not necessarily represent those of their affiliated organizations, or those of the publisher, the editors and the reviewers. Any product that may be evaluated in this article, or claim that may be made by its manufacturer, is not guaranteed or endorsed by the publisher.
Supplementary material
The Supplementary Material for this article can be found online at: https://www.frontiersin.org/articles/10.3389/fevo.2022.899585/full#supplementary-material
Footnotes
- ^ https://akclimate.org/data/precipitation-normals/
- ^ https://akclimate.org/data/air-temperature-normals/
- ^ https://gmao.gsfc.nasa.gov/reanalysis/MERRA-2/
References
Adams, L. G., and Gustine, D. D. (2018). Caribou forage and soil data, North Slope of Alaska, 2011-2014. U.S. Geological Survey data release. Anchorage, AK: U.S. Geological Survey, Alaska Science Center. doi: 10.5066/F7JQ106W
Ahrestani, F. S., Hebblewhite, M., and Post, E. (2013). The importance of observation versus process error in analyses of global ungulate populations. Sci. Rep. 3:3125. doi: 10.1038/srep03125
Albon, S. D., Irvine, R. J., Halvorsen, O., Langvatn, R., Loe, L. E., Ropstad, E., et al. (2017). Contrasting effects of summer and winter warming on body mass explain population dynamics in a food-limited Arctic herbivore. Glob. Change Biol. 23, 1374–1389. doi: 10.1111/gcb.13435
Aldridge, C. L., and Boyce, M. S. (2007). Linking occurrence and fitness to persistence: Habitat-based approach for endangered greater sage-grouse. Ecol. Appl. 17, 508–526. doi: 10.1890/05-1871
Arctic Monitoring and Assessment Report (2021). Arctic climate change update 2021: Key trends and impacts. Summary for policy-makers, Arctic Monitoring and Assessment Programme. Tromsø: Arctic Council.
Arnold, T. W. (2010). Uninformative parameters and model selection using Akaike’s information criterion. J. Wildl. Manage. 74, 1175–1178. doi: 10.1111/j.1937-2817.2010.tb01236.x
Barboza, P. S., and Parker, K. L. (2008). Allocating protein to reproduction in Arctic reindeer and caribou. Physiol. Biochem. Zool. 81, 835–855. doi: 10.1086/590414
Barboza, P. S., Shively, R. D., Gustine, D. D., and Addison, J. A. (2020). Winter is coming: Conserving body protein in female reindeer, caribou, and muskoxen. Front. Ecol. Evol. 8:150. doi: 10.3389/fevo.2020.00150
Barboza, P. S., Van Someren, L. L., Gustine, D. D., and Bret-Harte, M. S. (2018). The nitrogen window for arctic herbivores: Plant phenology and protein gain of migratory caribou (Rangifer tarandus). Ecosphere 9:e02073. doi: 10.1002/ecs2.2073
Bates, D., Maechler, M., Bolker, B., and Walker, S. (2015). Fitting linear mixed-effects models using lme4. J. Stat. Softw. 67, 1–48. doi: 10.18637/jss.v067.i01
Boggs, K., Flagstad, L., Boucher, T., Kuo, T., Fehringer, D., Guyer, S., et al. (2016). Vegetation map and classification: Northern, Western, and Interior Alaska, 2nd Edn. Anchorage, AK: Alaska Center for Conservation Science, University of Alaska Anchorage.
Bonenfant, C., Gaillard, J.-M., Coulson, T., Festa-Bianchet, M., Loison, A., Garel, M., et al. (2009). Empirical evidence of density dependence in populations of large herbivores. Adv. Ecol. Res. 41, 313–357. doi: 10.1016/S0065-2504(09)00405-X
Bongelli, E., Dowsley, M., Velasco-Herrera, V. M., and Taylor, M. (2020). Do North American migratory barren-ground caribou subpopulations cycle? Arctic 73, 326–346. doi: 10.14430/arctic71029
Boulanger, J., Gunn, A., Adamczewski, J., and Croft, B. (2011). A data-driven demographic model to explore the decline of the Bathurst caribou herd. J. Wildl. Manage. 75, 883–896. doi: 10.1002/jwmg.108
Burnham, K. P., and Anderson, D. R. (2002). Model selection and multimodel inference: A practical information-theoretic approach, 2nd Edn. New York, NY: Springer, 488.
Calenge, C. (2006). The package “adehabitat” for the R software: A tool for the analysis of space and habitat use by animals. Ecol. Model. 197, 516–519. doi: 10.1016/j.ecolmodel.2006.03.017
Cameron, R. D., Lenart, E. A., Reed, D. J., Whitten, K. R., and Smith, W. T. (1995). Abundance and movements of caribou in the oilfield complex near Prudhoe Bay, Alaska. Rangifer 15, 3–7. doi: 10.7557/2.15.1.1150
Cebrian, M., Kielland, K., and Finstad, G. (2008). Forage quality and reindeer productivity: Multiplier effects amplified by climate change. Arct. Antarct. Alp. Res. 40, 48–54. doi: 10.1657/1523-0430(06-073)[CEBRIAN]2.0.CO;2
Chan-McLeod, A. C. A., White, R. G., and Holleman, D. F. (1994). Effects of protein and energy intake, body condition, and season on nutrient partitioning and milk production in caribou and reindeer. Can. J. Zool. 72, 938–947. doi: 10.1139/z94-127
Chan-McLeod, A. C. A., White, R. G., and Russell, D. E. (1999). Comparative body composition strategies of breeding and nonbreeding female caribou. Can. J. Zool. 77, 1901–1907. doi: 10.1139/z99-169
Colman, J. E., Pedersen, C., Hjermann, D. Ø., Holand, Ø., Moe, S. R., and Reimers, E. (2003). Do wild reindeer exhibit grazing compensation during insect harassment? J. Wildl. Manage. 67, 11–19. doi: 10.2307/3803056
Cook, J. G., Johnson, B. K., Cook, R. C., Riggs, R. A., Delcurto, T., Bryant, L. D., et al. (2004). Effects of summer-autumn nutrition and parturition date on reproduction and survival of elk. Wildl. Monogr. 155, 1–61.
Cook, J. G., Kelly, A. P., Cook, R. C., Culling, B., Culling, D., McLaren, A., et al. (2021). Seasonal patterns in nutritional condition of caribou (Rangifer tarandus) in the southern Northwest Territories and northeastern British Columbia, Canada. Can. J. Zool. 99, 845–858. doi: 10.1139/cjz-2021-0057
Cook, R. C., Cook, J. G., Vales, D. J., Johnson, B. K., McCorquodale, S. M., Shipley, L. A., et al. (2013). Regional and seasonal patterns of nutritional condition and reproduction in elk. Wildl. Monogr. 184, 1–44. doi: 10.1002/wmon.1008
Culler, L. E., Ayres, M. P., and Virginia, R. A. (2015). In a warmer Arctic, mosquitos avoid increased mortality from predators by growing faster. Proc. R. Soc. B 282:20151549. doi: 10.1098/rspb.2015.1549
DeCesare, N. J., Hebblewhite, M., Bradley, M., Hervieux, D., Neufeld, L., and Musiani, M. (2014). Linking habitat selection and predation risk to spatial variation in survival. J. Anim. Ecol. 83, 343–352. doi: 10.1111/1365-2656.12144
Denryter, K. A., Cook, R. C., Cook, J. G., and Parker, K. L. (2017). Straight from the caribou’s (Rangifer tarandus) mouth: Detailed observations of tame caribou reveal new insights into summer-autumn diets. Can. J. Zool. 95, 81–94. doi: 10.1139/cjz-2016-0114
Denryter, K. A., Cook, R. C., Cook, J. G., and Parker, K. L. (2022). Animal-defined resources reveal nutritional inadequacies for woodland caribou during summer-autumn. J. Wildl. Manage. 86:e22161. doi: 10.1002/jwmg.22161
Doiron, M., Gauthier, G., and Lévesque, E. (2014). Effects of experimental warming on nitrogen concentration and biomass of forage plants for an Arctic herbivore. J. Ecol. 102, 508–517. doi: 10.1002/ece3.2859
Dormann, C. F., Elith, J., Bacher, S., Buchmann, C., Carl, G., Carré, G., et al. (2013). Collinearity: a review of methods to deal with it and a simulation study evaluating their performance. Ecography 36, 27–46.
Ehlers, L., Coulombe, G., Herriges, J., Bentzen, T., Suitor, M., Joly, K., et al. (2021). Critical summer foraging tradeoffs in a subarctic ungulate. Ecol. Evol. 11, 17835–17872. doi: 10.1002/ece3.8349
Eikelenboom, M., Higgins, R. C., John, C., Kerby, J., Forchhammer, M. C., and Post, E. (2021). Contrasting dynamical responses of sympatric caribou and muskoxen to winter weather and earlier spring green-up in the Arctic. Food Webs 27:e00196. doi: 10.1016/j.fooweb.2021.e00196
Elmendorf, S. C., Henry, G. H. R., Hollister, R. D., Björk, R. G., Boulanger-Lapointe, N., Cooper, E. J., et al. (2012). Plot-scale evidence of tundra vegetation change and links to recent summer warming. Nat. Clim. Change 2, 453–457. doi: 10.1038/nclimate1465
Fancy, S. G., Pank, L. F., Whitten, K. R., and Regelin, W. L. (1989). Seasonal movements of caribou in arctic Alaska as determined by satellite. Can. J. Zool. 67, 644–650. doi: 10.1139/z89-093
Fauchald, P., Park, T., Tømmervik, H., Myneni, R., and Hausner, V. H. (2017). Arctic greening from warming promotes declines in caribou populations. Sci. Adv. 3:e1601365. doi: 10.1126/sciadv.1601365
Fieberg, J., and DelGiudice, G. D. (2009). What time is it? Choice of time origin and scale in extended proportional hazards models. Ecology 90, 1687–1697. doi: 10.1890/08-0724.1
Fretwell, S. D., and Lucas, H. L. (1970). On territorial behaviour and other factors influencing habitat distribution in birds. Acta Biotheor. 19, 16–36.
Gagnon, C. A., Hamel, S., Russell, D. E., Powell, T., Andre, J., Svoboda, M. Y., et al. (2020). Merging indigenous and scientific knowledge links climate with the growth of a large migratory caribou population. J. Appl. Ecol. 57, 1644–1655. doi: 10.1111/1365-2664.13558
Gaillard, J.-M., Festa Bianchet, M., Yoccoz, N. G., Loison, A., and Toïgo, C. (2000). Temporal variation in fitness components and population dynamics of large herbivores. Annu. Rev. 31, 367–393. doi: 10.1146/annurev.ecolsys.31.1.367
Gaillard, J.-M., Hebbewhite, M., Loison, A., Fuller, M., Powell, R., Basille, M., et al. (2010). Habitat-performance relationships: Finding the right metric at a given scale. Philos. Trans. R. Soc. B 365, 2255–2265. doi: 10.1098/rstb.2010.0085
Griffith, B. G., Douglas, D. C., Walsh, N. E., Young, D. D., McCabe, T. R., Russell, D. E., et al. (2002). “Section 3—The Porcupine caribou herd,” in Arctic Refuge coastal plain terrestrial wildlife research summaries: USGS Biological Science report 2002–0001, eds D. C. Douglas, P. E. Reynolds, and E. B. Rhode (Reston, VA: USGS), 8–37.
Hagemoen, R. I. M., and Reimers, E. (2002). Reindeer summer activity pattern in relation to weather and insect harassment. J. Anim. Ecol. 71, 883–892. doi: 10.1046/j.1365-2656.2002.00654.x
Harrell, F. E. (2021). Regression modeling strategies. Version 6.2-0. Available online at: https://cran.r-project.org/web/packages/rms/rms.pdf (accessed January 20, 2022).
Hartig, F. (2021). DHARMa: Residual diagnostics for hierarchical (multi-level/mixed) regression models. R package version 0.4.3. Available online at: https://cran.r-project.org/web/packages/DHARMa/index.html (accessed January 20, 2022).
Helle, P., and Tarvainen, L. (1984). Effects of insect harassment on weight gain and survival in reindeer calves. Rangifer 4, 24–27. doi: 10.7557/2.4.1.488
Hughes, J., Albon, S. D., Irvine, R. J., and Woodin, S. (2009). Is there a cost of parasites to caribou? Parasitology 136, 253–265. doi: 10.1017/S0031182008005246
Jenkerson, C. B., Maiersperger, T., and Schmidt, G. L. (2010). eMODIS: A user-friendly data source. U.S. Geological Survey open file report 2010-1055. Reston, VA: U.S. Geological Survey. doi: 10.3133/ofr20101055
Johnson, H. E., Golden, T. S., Adams, L. G., Gustine, D. D., and Lenart, E. A. (2020). Caribou use of habitat near energy development in Arctic Alaska. J. Wildl. Manage. 84, 401–412. doi: 10.1002/jwmg.21809
Johnson, H. E., Golden, T. S., Adams, L. G., Gustine, D. D., Lenart, E. A., and Barboza, P. S. (2021). Dynamic selection for forage quality and quantity in response to phenology and insects in an Arctic ungulate. Ecol. Evol. 11, 11664–11688. doi: 10.1002/ece3.7852
Johnson, H. E., Gustine, D. D., Golden, T. S., Adams, L. G., Parrett, L. S., Lenart, E. A., et al. (2018). NDVI exhibits mixed success in predicting spatiotemporal variation in caribou summer forage quality and quantity. Ecosphere 9:e02461. doi: 10.1002/ecs2.2461
Johnson, H. E., Mills, L. S., Stephenson, T. R., and Wehausen, J. D. (2010). Population-specific vital rate contributions influence management of an endangered ungulate. Ecol. Appl. 20, 1753–1765. doi: 10.1890/09-1107.1
Joly, K., Gurarie, E., Sorum, M. S., Kaczensky, P., Cameron, M. D., Jakes, A. F., et al. (2020b). Longest terrestrial migrations and movements around the world. Sci. Rep. 9:15333. doi: 10.1038/s41598-019-51884-5
Joly, K., Couriot, O., Cameron, M. D., and Gurarie, E. (2020a). Behavioral, physiological, demographic and ecological impacts of hematophagous and endoparasitic insects on an Arctic ungulate. Toxins 12:334. doi: 10.3390/toxins12050334
Joly, K., Wasser, S. K., and Booth, R. (2015). Non-invasive assessment of the interrelationships of diet, pregnancy rate, group composition, and physiological and nutritional stress of barren-ground caribou in later winter. PLoS One 10:e0127586. doi: 10.1371/journal.pone.0127586
Kaluskar, S., Blukacz-Richards, E. A., Johnson, C. A., He, Y., Langlois, A., Kim, D.-K., et al. (2019). Development of a model ensemble to predict Peary caribou populations in the Canadian Arctic Archipelago. Ecosphere 10:e02976. doi: 10.1002/ecs2.2976
Kautz, T. M., Belant, J. L., Beyer, D. E. Jr., Strickland, B. K., and Duquette, J. F. (2020). Influence of body mass and environmental conditions on winter mortality risk of a northern ungulate: Evidence for a late-winter survival bottleneck. Ecol. Evol. 10, 1666–1677. doi: 10.1002/ece3.6026
Kenny, T. A., Fillion, M., Simpkin, S., Wesche, S. D., and Chan, H. M. (2018). Caribou (Rangifer tanandus) and Inuit nutritional security in Canada. EcoHealth 15, 590–607. doi: 10.1007/s10393-018-1348-z
Kie, J. G., Matthiopoulos, J., Fieberg, J., Powell, R. A., Cagnacci, F., Mitchell, M. S., et al. (2010). The home-range concept: Are traditional estimators still relevant with the modern telemetry technology? Philos. Trans. R. Soc. B Biol. Sci. 365, 2221–2231. doi: 10.1098/rstb.2010.0093
Koltz, A. M., and Culler, L. E. (2021). Biting insects in a rapidly changing Arctic. Curr. Opin. Insect Sci. 47, 75–81. doi: 10.1016/j.cois.2021.04.009
Lader, R., Walsh, J. E., Bhatt, U. S., and Bieniek, P. A. (2017). Projections of twenty-first-century climate extremes for Alaska via dynamical downscaling and quantile mapping. J. Appl. Meteorol. Climatol. 56, 2393–2409. doi: 10.1175/JAMC-D-16-0415.1
Lenart, E. A. (2019). Memorandum: 2019 Central Arctic caribou photocensus results. Fairbanks, AK: Alaska Department of Fish and Game, Division of Wildlife Conservation.
Lenart, E. A. (2020). Memorandum: 2020 Central Arctic caribou winter distribution, parturition, postcalving, and fall composition. Fairbanks, AK: Alaska Department of Fish and Game, Division of Wildlife Conservation.
Lenart, E. A. (2021). Central Arctic caribou management report and plan, game management unit 26B: Report period 1 July 2012-30 June 2017, and plan period 1 July 2017-30 June 2022. Juneau, AK: Alaska Department of Fish and Game, Division of Wildlife Conservation.
Leroux, S. J. (2019). On the prevalence of uninformative parameters in statistical models applying model selection in applied ecology. PLoS One 14:e0206711. doi: 10.1371/journal.pone.0206711
Long, R. A., Bowyer, R. T., Porter, W. P., Mathewson, P., Monteith, K. L., Findolt, S. L., et al. (2016). Linking habitat selection to fitness-related traits in herbivores: the role of the energy landscape. Oecologia 181, 709–720.
Lukacs, P. M., Mitchell, M. S., Hebblewhite, M., Johnson, B. K., Johnson, H., Kauffman, M., et al. (2018). Factors influencing elk recruitment across ecotypes in the Western United States. J. Wildl. Manage. 82, 698–710. doi: 10.1002/jwmg.21438
Mallory, C. D., and Boyce, M. S. (2018). Observed and predicted effects of climate change on Arctic caribou and reindeer. Environ. Rev. 26, 13–25. doi: 10.1139/er-2017-0032
Mallory, C. D., Campbell, M. W., and Boyce, M. S. (2018). Climate influences body condition and synchrony of barren-ground caribou abundance in Northern Canada. Polar Biol. 41, 855–864.
Manlik, O. (2019). “The importance of reproduction for the conservation of slow-growing animal populations,” in Reproductive science in animal conservation—progress and prospects, 2nd Edn, eds P. Comizzoli, J. Brown, and W. V. Holt (New York, NY: Springer), 13–39. doi: 10.1007/978-3-030-23633-5_2
Messier, F., Huot, J., le Henaff, D., and Luttich, S. (1988). Demography of the George River caribou herd: Evidence of population regulation by forage exploitation and range expansion. Arctic 41, 279–287. doi: 10.14430/arctic1733
Mills, L. S. (2013). Conservation of wildlife populations: Demography, genetics, and management, 2nd Edn. Malden, MA: Wiley-Blackwell.
Monteith, K. L., Bleich, V. C., Stephenson, T. R., Pierce, B. M., Conner, M. M., Kie, J. G., et al. (2014). Life-history characteristics of mule deer: Effects of nutrition in a variable environment. Wildl. Monogr. 186, 1–62. doi: 10.1002/wmon.1011
Newby, J. R., and DeCesare, N. J. (2020). Multiple nutritional currencies shape pregnancy in a large herbivore. Can. J. Zool. 98, 307–315. doi: 10.1139/cjz-2019-0241
Nicholson, K. L., Arthur, S. M., Horne, J. S., Garton, E. O., and Del Vecchio, P. A. (2016). Modeling caribou movements: Seasonal range and migration routes of the Central Arctic herd. PLoS One 11:e0150333. doi: 10.1371/journal.pone.0150333
Pachkowski, M., Côté, S. D., and Festa-Bianchet, M. (2013). Spring-loaded reproduction: Effects of body condition and population size on fertility in migratory caribou (Rangifer tarandus). Can. J. Zool. 91, 473–479. doi: 10.1139/cjz-2012-0334
Parker, K. L., Barboza, P. S., and Gillingham, M. P. (2009). Nutrition integrates environmental responses of ungulates. Funct. Ecol. 23, 57–69. doi: 10.1111/j.1365-2435.2009.01528.x
Pedersen, S. H., Bentzen, T. W., Reinking, A. K., Liston, G. E., Elder, K., Lenart, E. A., et al. (2021). Quantifying effects of snow depth on caribou winter range selection and movement in Arctic Alaska. Mov. Ecol. 9:48. doi: 10.1186/s40462-021-00276-4
Peeters, B., Veiberg, V., Pedersen, A. Ø., Stien, A., Irvine, R. J., Aanes, R., et al. (2017). Climate and density dependence cause changes in adult sex ratio in a large herbivore. Ecosphere 8:e01699. doi: 10.1002/ecs2.1699
Plante, S., Dussault, C., Richard, J. H., Garel, M., and Côté, S. D. (2020). Untangling effects of human disturbance and natural factors on mortality risk of migratory caribou. Front. Ecol. Evol. 8:154. doi: 10.3389/fevo.2020.00154
Prichard, A. K., Parrett, L. S., Lenart, E. A., Caikoski, J. R., Joly, K., and Person, B. T. (2020). Interchange and overlap among four adjacent Arctic caribou herds. J. Wildl. Manage. 84, 1500–1514. doi: 10.1002/jwmg.21934
Proffitt, K. M., Courtemanch, A. B., Dewey, S. R., Lowrey, B., McWhirter, D. E., Monteith, K. L., et al. (2021). Regional variability in pregnancy and survival rates of Rocky Mountain bighorn sheep. Ecosphere 12:e03410.
Proffitt, K. M., Hebblewhite, M., Peters, W., Hupp, N., and Shamhart, J. (2016). Linking landscape-scale differences in forage to ungulate nutritional ecology. Ecol. Appl. 26, 2156–2174. doi: 10.1002/eap.1370
R Core Team (2021). R: A language and environmental for statistical computing. Vienna: R Foundation for statistical computing.
Richert, J. C., Leffler, A. J., Spalinger, D. E., and Welker, J. M. (2021). Snowier winters extend autumn availability of high-quality forage for caribou in Arctic Alaska. Ecosphere 12:e03617. doi: 10.1002/ecs2.3617
Russell, D. E., Martell, A. M., and Nixon, W. A. (1993). The range ecology of the Porcupine caribou herd in Canada. Rangifer 8, 1–168. doi: 10.7557/2.13.5.1057
Russell, D. E., Whitfield, P. H., Cai, J., Gunn, A., White, R. G., and Poole, K. (2013). CARMA’s MERRA-based caribou range climate database. Rangifer 33, 145–152. doi: 10.7557/2.33.2.2535
Schielzeth, H. (2010). Simple means to improve the interpretability of regression coefficients. Methods Ecol. Evol. 1, 103–113. doi: 10.1016/j.ejrad.2008.03.019
Schoenfeld, D. (1982). Partial residuals for the proportional hazards regression model. Biometrika 69, 239–241. doi: 10.1093/biomet/69.1.239
Schrempp, T. V., Rachlow, J. L., Johnson, T. R., Shipley, L. A., Long, R. A., Aycrigg, J. L., et al. (2019). Linking forest management to moose population trends: the role of the nutritional landscape. PLoS One 14:e0219128. doi: 10.1371/journal.pone.0219128
Severson, J. P., Johnson, H. E., Arthur, S. M., Leacock, W. B., and Suitor, M. J. (2021). Spring phenology drives range shifts in a migratory Arctic ungulate with key implications for the future. Glob. Change Biol. 19, 4546–4563. doi: 10.1111/gcb.15682
Stoner, D. C., Sexton, J. O., Nagol, J., Bernales, H. H., and Edwards, T. C. Jr. (2016). Ungulate reproductive parameters track satellite observations of plant phenology across latitude and climatological regimes. PLoS One 11:e0148780. doi: 10.1371/journal.pone.0148780
Taillon, J., Barboza, P. S., and Côté, S. D. (2013). Nitrogen allocation to offspring and milk production in a capital breeder. Ecology 94, 1815–1827. doi: 10.1890/12-1424.1
Therneau, T. (2020). Mixed effects cox models. Version 2.2-16. Available online at: https://cran.r-project.org/web/packages/coxme/index.html (accessed January 20, 2022).
Thompson, D. C., and McCourt, K. H. (1981). Seasonal diets of the Porcupine caribou herd. Am. Midl. Nat. 105, 70–76. doi: 10.2307/2425011
Thompson, D. P., and Barboza, P. S. (2013). Responses of caribou and reindeer (Rangifer tarandus) to acute food shortages in spring. Can. J. Zool. 91, 610–618. doi: 10.1139/cjz-2013-0047
Thompson, D. P., and Barboza, P. S. (2017). Seasonal energy and protein requirements for Siberian reindeer (Rangifer tarandus). J. Mammal. 98, 1558–1567. doi: 10.1093/jmammal/gyx132
Tollefson, T. N., Shipley, L. A., Woodrow, L. M., Keisler, D. H., and Dasgupta, N. (2010). Influence of summer and autumn nutrition on body condition and reproduction in lactating mule deer. J. Wildl. Manage. 74, 974–986. doi: 10.2193/2008-529
Tveraa, T., Stein, A., Bårdsen, B.-J., and Fauchald, P. (2013). Population densities, vegetation green-up, and plant productivity: Impacts on reproductive success and juvenile body mass in reindeer. PLoS One 8:e56450. doi: 10.1371/journal.pone.0056450
VanSomeren, L. L., Barboza, P. S., Thompson, D. P., and Gustine, D. D. (2015). Monitoring digestibility of forages for herbivores: A new application for an old approach. Can. J. Zool. 93, 187–195. doi: 10.1139/cjz-2014-0207
Weladji, R. B., Holand, Ø., and Almoy, T. (2003). Use of climatic data to assess the effect of insect harassment on the autumn weight of reindeer (Rangifer tarandus) calves. J. Zool. 260, 79–85. doi: 10.1017/S0952836903003510
White, R. G. (1983). Foraging patterns and their multiplier effects on productivity of northern ungulates. Oikos 40, 377–384. doi: 10.2307/3544310
White, R. G., Thompson, B. R., Skoogland, T., Person, S. J., Holleman, D. F., and Luick, J. R. (1975). “Ecology of caribou at Prudhoe Bay, Alaska,” in Ecological investigations of the tundra biome in the Prudhoe Bay region, Alaska. Special Report No. 2, ed. J. Brown (Fairbanks, AK: Biological Papers of the University of Alaska), 150–201.
White, R. G., and Trudell, J. (1980). Habitat preference and forage consumption by reindeer and caribou near Atkasook, Alaska. Arct. Alp. Res. 12, 511–529. doi: 10.2307/1550498
Whitten, K. R. (1995). Antler loss and udder distention in relation to parturition in caribou. J. Wildl. Manage. 59, 273–277. doi: 10.2307/3808940
Wilson, R. R., Prichard, A. K., Parrett, L. S., Person, B. T., Carroll, G. M., Smith, M. A., et al. (2012). Summer resource selection and identification of important habitat prior to industrial development for the Teshekpuk Caribou Herd in northern Alaska. PLoS One 7:e48697. doi: 10.1371/journal.pone.0048697
Witter, L. A., Johnson, C. J., Croft, B., Gunn, A., and Gillingham, M. P. (2012). Behavioural trade-offs in response to external stimuli: Time allocation of an Arctic ungulate during varying intensities of harassment by parasitic flies. J. Anim. Ecol. 81, 284–295. doi: 10.1111/j.1365-2656.2011.01905.x
Keywords: biomass, barren-ground caribou, digestible nitrogen, mosquito harassment, Rangifer tarandus, reproduction, summer nutrition, survival
Citation: Johnson HE, Lenart EA, Gustine DD, Adams LG and Barboza PS (2022) Survival and reproduction in Arctic caribou are associated with summer forage and insect harassment. Front. Ecol. Evol. 10:899585. doi: 10.3389/fevo.2022.899585
Received: 18 March 2022; Accepted: 27 July 2022;
Published: 20 September 2022.
Edited by:
Brett K. Sandercock, Norwegian Institute for Nature Research (NINA), NorwayReviewed by:
Brage Bremset Hansen, Norwegian Institute for Nature Research (NINA), NorwayJohn Cook, National Council for Air and Stream Improvement, Inc. (NCASI), United States
Evelyn Hunter Merrill, University of Alberta, Canada
Copyright © 2022 Johnson, Lenart, Gustine, Adams and Barboza. This is an open-access article distributed under the terms of the Creative Commons Attribution License (CC BY). The use, distribution or reproduction in other forums is permitted, provided the original author(s) and the copyright owner(s) are credited and that the original publication in this journal is cited, in accordance with accepted academic practice. No use, distribution or reproduction is permitted which does not comply with these terms.
*Correspondence: Heather E. Johnson, aGVhdGhlcmpvaG5zb25AdXNncy5nb3Y=