- Mitrani Department of Desert Ecology, Blaustein Institutes for Desert Research, Ben-Gurion University of the Negev, Midreshet Ben-Gurion, Israel
Coexistence theories develop rapidly at the ecology forefront suffering from interdisciplinary gaps and a lack of universality. The modern coexistence theory (MCT) was developed to address these deficiencies by formulating the universal conditions for coexistence. However, despite this theory's mechanistic foundation, initially, it has only rarely been used to determine the exact mechanisms that govern the competitive outcome. Recent theoretical developments have made MCT more accessible to experimentalists, but they can be challenging in practice. We propose that a comprehensive understanding of species co-occurrence patterns in nature can be reached by complementing the phenomenological approach with both the mechanistic view of MCT and coexistence experiments of the type that prevailed from the 1970s to the 2010s, which focused on specific mechanisms (designated the “mechanistic approach”). As a first step in this direction, we conducted a systematic review of the literature from 1967 to 2020, covering mechanistic experiments for invasibility—the criterion for species coexistence—and the best-studied classical coexistence mechanisms, namely, resource-ratio, natural enemy partitioning, frequency-dependent exploitation by generalist enemies, and the storage effect. The goals of the review were to evaluate (i) the percentage of the abovementioned mechanistic experiments that satisfy the theoretical criteria (designated “eligible studies”), (ii) the scope of these eligible studies, and (iii) their level of support for the theoretical predictions, and to identify their (iv) overarching implications and (v) research gaps. Through examination of 2,510 publications, the review reveals that almost 50 years after the theoretical formulations of the above four coexistence mechanisms, we still lack sufficient evidence to reveal the prevalence of coexistence and of each of the coexistence mechanisms, and to assess the dependency of the mechanisms on the natural history of the competing organisms. By highlighting, on the one hand, the overarching implications of the mechanistic approach to coexistence, and on the other hand, current research gaps, and by offering ways to bridge these gaps in the future, we seek to bring the mechanistic approach back to life.
Introduction
One of the major goals in the field of community ecology is to explore the biological mechanisms underlying community patterns. At the heart of the goal is the challenge of understanding the stable coexistence (see Glossary) patterns and mechanisms maintaining the remarkable species diversity found in nature. The root of this interest derives from the contradiction between Gause's law (Gause, 1934), which states that species competing for the same limiting resource cannot persist together indefinitely, and the observations of high diversity of similar species in an apparently limited number of ecological niches in nature (e.g., Hutchinson, 1961). Today, we know that, under certain conditions, stable coexistence is possible, even for organisms exploiting the same resource, and that coexistence is not solely resource based (e.g., Chesson and Kuang, 2008; McPeek, 2012), making the subject even more compelling to researchers (Agrawal et al., 2007; Chesson, 2018; Letten et al., 2018).
The longstanding interest in this subject is reflected in the broad range of theories for coexistence mechanisms that have been developed over the past 50 years and that encompass various research disciplines, organisms, environments, and types of interactions. Importantly, in contrast to many other ecological subjects, interest in coexistence has never ceased (Figure 1). However, the variety of coexistence perspectives both limits the different theories' accessibility to experimentalists (e.g., Wilson et al., 2007) and increases the likelihood that researchers will reinvent the wheel (e.g., compare between Comita et al., 2014; Vage et al., 2018); in addition, their work may suffer from a lack of universality (e.g., compare the approaches taken in the following studies: Chesson, 2000b; Kneitel and Chase, 2004; Letten et al., 2017; Levine et al., 2017; Eidelman et al., 2019; Kotler and Brown, 2020; Luimstra et al., 2020). Consequently, today, there is a deficiency in empirical information regarding coexistence patterns and mechanisms (Figure 1; Letten et al., 2017; Bartomeus and Godoy, 2018; Ellner et al., 2019).
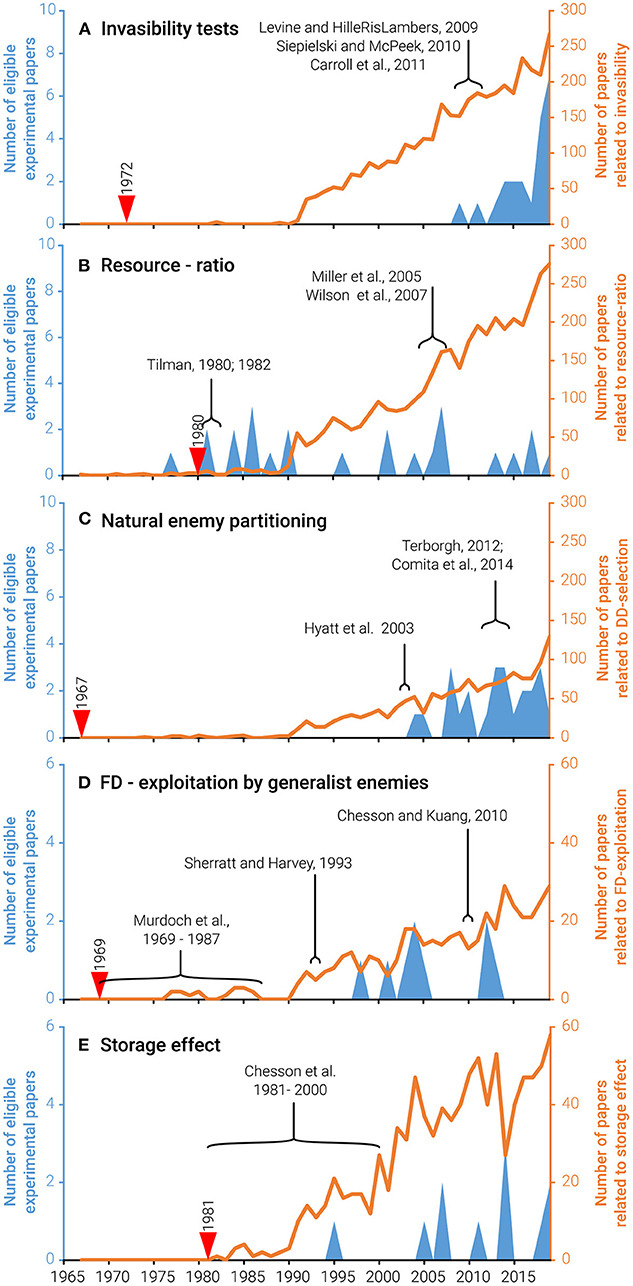
Figure 1. Literature trends, reflecting the lack of experimental coexistence studies. General interest in the coexistence topics that we chose (orange (orange lines, right axis) and the experimental studies that our review selected as eligible (blue areas, left axis) is still increasing. However, for each topic-year combination, the eligible experimental studies constitute less than 2% of the published pool. We chose five core topics, namely, invasibility tests for coexistence (A) and mechanisms allowing invasibility, i.e., resource-ratio (B), natural enemy partitioning (C), frequency-dependent exploitation by a generalist enemy (D), and the storage effect (E). The starting year of each literature survey and the influential papers that were used as a basis for the literature search are indicated by red arrows and elongated black brackets , respectively.
The modern coexistence theory (MCT; see Glossary) was developed to address these deficiencies by formulating the universal conditions for coexistence, i.e., that stabilizing niche differences will offset relative fitness differences (Chesson, 2000b). This theory, which constitutes a common framework for a diverse range of study systems, mechanisms, and topics (Grainger et al., 2019b), has prevailed in coexistence research over the past few years. MCT partitions population growth rates into the different mechanisms that contribute to coexistence (Chesson, 1990, 1994, 2000a). However, following the syntheses by Chesson (2000b) and Chesson and Kuang (2008), MCT's initial works mainly took a phenomenological form, by mapping how species traits and environmental conditions relate to niche and fitness differences, without delving into the details of the underlying mechanisms (e.g., Adler et al., 2007; Mayfield and Levine, 2010; Narwani et al., 2013; Godoy et al., 2014; Kraft et al., 2015; Germain et al., 2016; Saavedra et al., 2017; Ke and Letten, 2018; Ocampo-Ariza et al., 2018; Gallego et al., 2019; Spaak and De Laender, 2020). Accordingly, this phenomenological form of MCT is chiefly concerned with the outcomes of coexistence mechanisms, rather than with the mechanisms themselves. In recent years, the mechanistic basis has continued to develop, providing a general analytical approach to multiple mechanisms (e.g., Chesson, 2008, 2018; Kuang and Chesson, 2010; Ellner et al., 2019) and allowing the assessment of their relative contributions to coexistence in a single studied community (Letten et al., 2018; Grainger et al., 2019a; Hallett et al., 2019; Shoemaker et al., 2020). However, this mechanistic view of MCT is challenging, as it requires measuring and modeling population responses to multiple limiting factors that represent variation in community composition and in the environment (Ellner et al., 2019).
We propose that to extend our understanding of species co-occurrence patterns in nature, it is necessary to restore the coexistence experiments that prevailed from the 1970s to the 2010s, which sought more specific mechanisms. This can be done through both classical coexistence experiments and the mechanistic view of MCT (designated the “mechanistic approach”), in parallel with making progress by applying the phenomenological approach. The mechanistic approach will improve the precision in formulating mechanisms by pinpointing the specific selective factors underlying coexistence (e.g., limiting resources, generalist enemies, or environmental disturbance) and will establish associations between species' traits and interaction outcomes. It will also provide direct proof of cause-and-effect relationships and widen the knowledge of specific systems. As a first step, we conducted a systematic review of the literature from 1967 to 2020, covering mechanistic experiments for invasibility—the criterion for species coexistence—and the best-studied classical coexistence mechanisms, namely, resource-ratio, natural enemy partitioning, frequency-dependent exploitation by generalist enemies, and the storage effect. The review's objectives were to evaluate (i) the percentage of these mechanistic experiments that satisfy the theoretical criteria (designated “eligible studies”), (ii) the scope of these eligible studies, and (iii) their level of support for the theoretical predictions, and to identify their (iv) overarching implications and (v) research gaps. We conclude by identifying the reasons for the research gaps and offer future directions to address the obstacles and bring these classical mechanistic experiments—and others—back to life.
Methods
General Literature Search Strategy
Separate literature surveys were conducted for each coexistence topic in the ISI Web of Knowledge database; only papers published in English were surveyed. For each survey, we first searched for the most influential relevant papers. In some cases, these influential papers were comprehensive reviews, and in others, we selected the original papers that established the theoretical foundation of the mechanism (Supplementary Table S1). Then, we complemented each survey for the missing years that were not covered previously by searching for additional papers that cited the influential papers or that included specific relevant topic terms in the title, the abstract, the author keywords, or the abstract keywords (see Supplementary Table S1 for the exact topic terms). To focus the survey on experimental coexistence studies, we decided that all the selected papers should include, in addition to the specific key terms and citations, the key terms “coexisten*” or “co-existen*,” and “experiment*,” “manipulat*,” or “treatment” (Supplementary Table S1). By using this approach, we might have missed some relevant papers that are not part of this search engine's database or that only included the specific key terms in the paper's body. However, it served to make the literature search uniform among the various tested topics and study systems. We then screened the titles of both the studies that were included in previous reviews and those identified from the database, and we removed irrelevant papers. The remaining papers were carefully checked, and only the papers that satisfied the theoretical criteria (details for each topic are provided below) were regarded as eligible studies (Figure 2 and Supplementary Table S2). Thus, the decision as to whether a paper was considered eligible or not was based on the design of the experiment and not on its results.
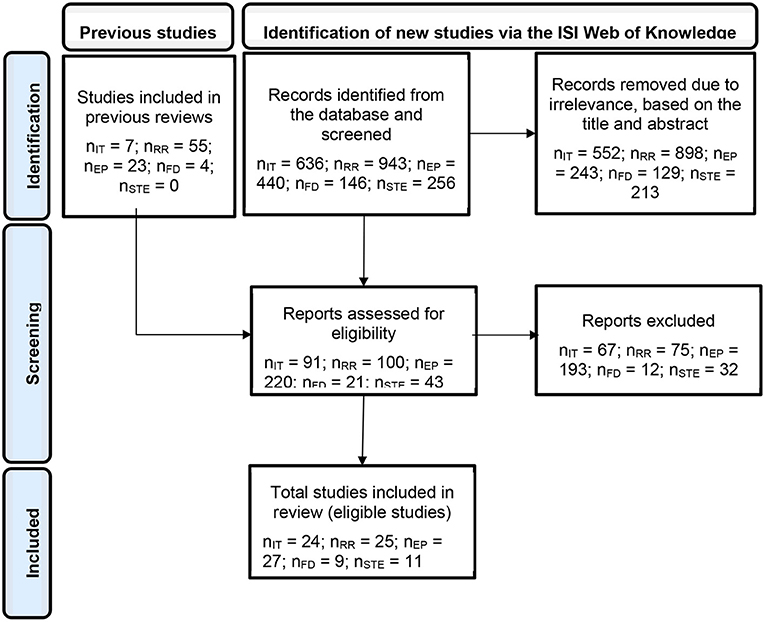
Figure 2. Flow diagram for the systematic review of the five coexistence topics: invasibility tests (IT), resource-ratio (RR), natural enemy partitioning (EP), frequency-dependent exploitation by generalist enemies (FD), and the storage effect (STE). “n” is the sample size.
Initially, the papers related to each coexistence topic were scanned by a single reviewer (coauthors HH, MG, CC, SH, or SC), using the papers' titles and abstracts. Then, coauthor HH, who defined the theoretical criteria for each topic, made decisions regarding papers whose eligibility was in doubt, read the eligible papers, and extracted the required information from these papers. The design and reporting scheme of the surveys conform to PRISMA reporting guidelines (Liberati et al., 2009).
Search Strategy and Selection Criteria for Each Review Topic
To estimate the prevalence of coexistence, we searched for experimental studies that included invasibility tests (see below). To choose the target coexistence mechanisms, we used Chesson's synthesis (2000b), which organizes the multiple classical coexistence theories under a unified framework. We selected theories (i) that deal with local coexistence, (ii) that have well-defined theoretical criteria, (iii) that are relatively simple and intuitive, yet constitute building blocks for more complex models, (iv) that encompass the essence of consumer-resource, enemy-consumer, and environment-consumer interactions, (v) that, to the best of our knowledge, are familiar to experimentalists, and (vi) for which a preliminary literature search detected at least five experimental studies.
Below, we briefly introduce each of the topics and the selection criteria that were applied to regard a paper as eligible for testing each topic. Theoretically, proper tests of coexistence mechanisms should associate the features of the mechanism under test with demonstrated stabilization, such as mutual invasibility or the density and frequency dependence of long-term growth rates. This is because the presence of a required set of conditions, by itself, does not imply that these conditions are the ones that promote coexistence; other stabilizing effects may dominate (Chesson, 2018). However, due to the paucity of studies that have included this association, a proper test of mutual invasibility was a sufficient criterion to select a paper as eligible for this topic, and proper tests for the features of each of the four mechanisms (see below) were sufficient criteria to select a paper as eligible for each one of them. In Supplementary Table S2, we indicated, for each eligible invasibility study, whether it was associated with a specific mechanism, and for the studies designed to test each of the four coexistence mechanisms, we indicated whether the purported mechanism was associated with mutual invasibility tests or any other coexistence proxies.
Three obstacles prevented us from presenting a standard mathematical model for each topic, namely, the models' complexity levels, their multifold techniques [e.g., compare the approaches used by Roughgarden and Feldman (1975), Hutson (1984), and Kuang and Chesson (2010) to investigate the stabilizing effect of the frequency-dependent exploitation by generalist enemies mechanism), and the original absence of a mathematical approach for the natural enemy partitioning mechanism (Janzen, 1970; Connell, 1971)]. Thus, instead, below, we refer the readers to the papers that include the most simple and basic models for each of the topics. To illustrate the experimental design required for testing the various coexistence topics, we refer, in each topic, to the same basic design elements, namely when each species is maintained alone and in a species mixture, at low and high densities (Figures 3A,C). The search methods and terms, the number of papers retrieved in each specific search, and the number of eligible papers from the selected pool are presented in Figure 2 and Supplementary Table S1.
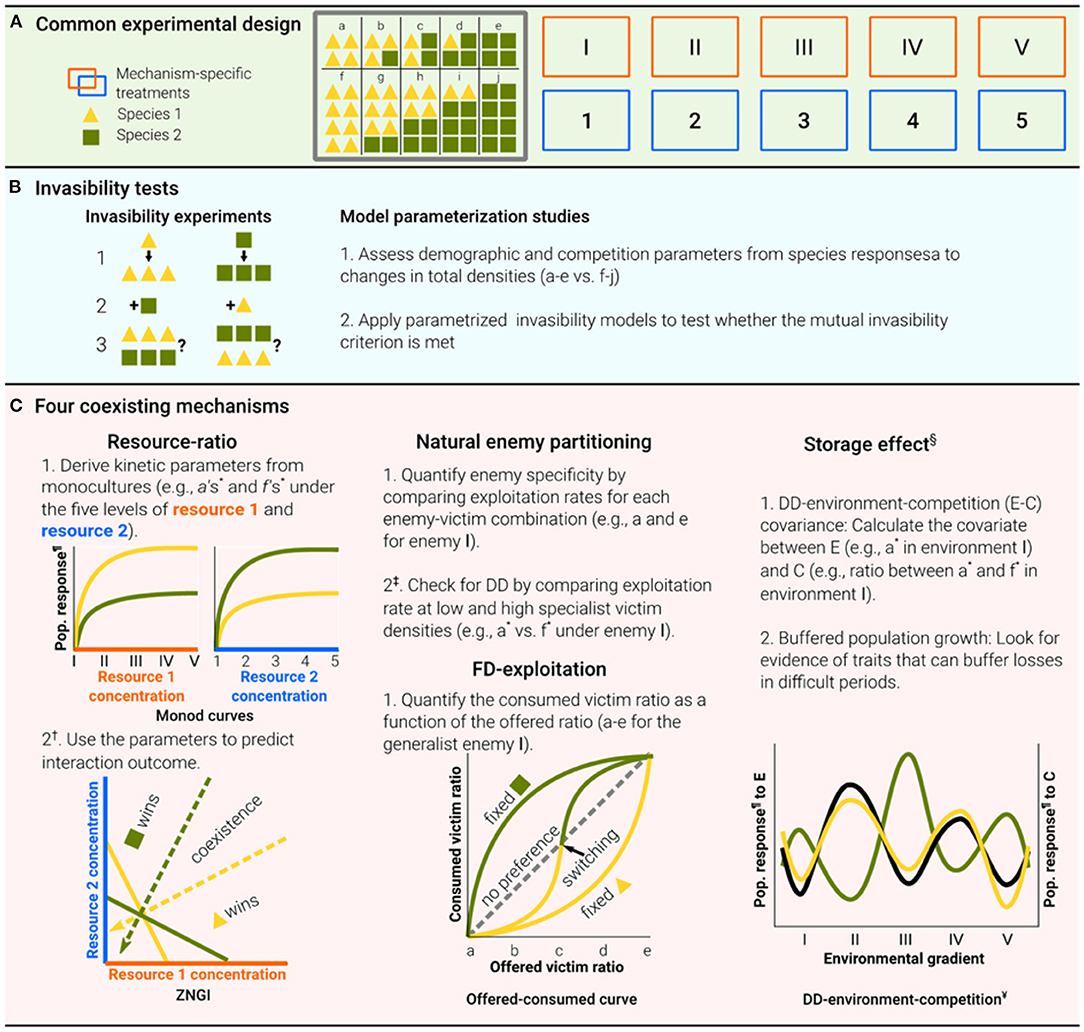
Figure 3. Proposed experimental design to encourage coexistence experiments and bridge the interdisciplinary gap. To facilitate interdisciplinary communication and uniform coexistence studies, we illustrate here how the same basic experimental design can be exploited to test various coexistence mechanisms. All eligible studies in the literature surveys included variations of these designs. Comprehensive experimental designs of all four coexistence mechanisms should assess the population response of the target species when each species is maintained alone (a and f in the matrix) and (or) in a species mixture (b–e and g–j in the matrix), at low (a–e in the matrix) and (or) high (f–j in the matrix) densities (A). However, the experimental conditions required for testing the various mechanisms may differ [the orange and blue rectangles may represent various levels of factors; see (C)]. Invasibility tests can be conducted through either invasibility experiments, in which each species as an invader is introduced at low densities to established residences and its growth rate is estimated, or model parametrization studies (B). The exact experimental design depends on the target mechanism. For example, for the resource-ratio, natural enemy partitioning, frequency-dependent exploitation (FD-exploitation), and storage effect mechanisms, the relevant factors are resource levels, specialist enemy densities, generalist enemy densities, and environmental conditions, respectively (C). *Examples are provided for the yellow species but should also apply to the green species.†Alternatively use the modern coexistence theory (MCT) approach; Figure 3C in Letten et al. (2017). ‡Alternatively use the MCT approach; Figures 5B,C in Ke and Wan (2020). §The first criterion is formulated for the spatial storage effect, while the second is inherent in this mechanism (derives from the dispersal of the population across a heterogeneous environment). However, the same rationale should apply to the temporal storage effect. In this case, the orange and blue rectangles mark time periods, and the second criterion is essential. ¶Population response means the per capita growth rate. However, due to practical difficulties, it can be replaced by any fitness-correlative trait, such as biomass. ¥The yellow and green lines represent the environmental responses of the two species in their resident and invader states, respectively, and the black line represents competition. The storage effect figure is modified from Holt and Chesson (2016).
Invasibility Tests
Mutual invasibility tests are methods that are based on the theoretical distinction between stable coexisting and co-occurring species (see Glossary) and that distinguish between these two types of associations by testing the ability of each species to exhibit a positive growth rate when it is rare in a resident community (see Glossary) that remains at typical steady state abundances., i.e., when it fulfills the invasibility criterion (MacArthur, 1972). These tests can be employed in various communities and environment types, as long as the species are not rare due to Allee or priority effects, the species are not part of an intransitive interaction network, and the conditions do not lead to alternative stable states (reviewed in Grainger et al., 2019b). In the latter cases, alternative criteria should be met for defining stable coexistence (Chesson, 2018, 2020). Siepielski and McPeek (2010) conducted a literature search from 1972 to 2009 that quantified the experimental evidence for invasibility. Here, we complemented this survey by extending it to October 2020 (Supplementary Table S1).
We considered as eligible those studies that included a sufficient test of the mutual invasibility criterion using one of two approaches: model parameterization studies and mutual invasibility experiments (Broekman et al., 2019; Godwin et al., 2020). Model parameterization studies (Glossary) project the long-term community dynamics in invasion models that are built on experimentally verified relationships between species frequencies and their population growth. To adhere to the experimental requirement, we accepted only studies that evaluated the model parameters based on intra- and interspecies competition assays, including density, frequency manipulations, or both. In mutual invasibility experiments, each species, as an invader, is introduced to an established population or community of resident species (see Glossary), and its growth rate is monitored (Figure 3B).
“Resource-Ratio” Mechanism
The “resource-ratio” mechanism is a fluctuation-independent mechanism (see Glossary), which allows species coexistence due to trade-offs in resource requirements and consumption. Coexistence is predicted when each species is a better competitor for a different resource (see the “R* rule prediction” in the Glossary), consuming relatively more of the resource that limits its own growth rate, and when the natural supply of these limiting resources does not disproportionally favor one of the species. In this scenario, each species is limited by changes in intraspecific, rather than interspecific, densities (Tilman, 1982). The idea dates back to the 1970s (e.g., MacArthur, 1972) but was recognized by ecologists some 10 years later, mainly through Tilman's graphical approach (Tilman, 1980, 1982), which formulated the underlying hypotheses (Tilman, 1980). In accordance with this approach, coexistence can be graphically viewed by analyzing how zero net growth isoclines (ZNGIs; see Glossary) of the competing species cross each other in relation to the position of resource supply ratio and consumption vectors (see Glossary; Figure 3C). Subsequent reviews, dating from 1980 to 2003, surveyed experiments testing the theory (Miller et al., 2005; Wilson et al., 2007). We complemented these surveys by extending them to October 2020 (Supplementary Table S1).
We considered as eligible, monoculture experiments generating predictions about species ranks per resource (based on the “R* rule) and assessing the relevant trade-offs for two species competing for two or more resources (Figure 3C). Then, at least one prediction about the community composition had to be tested in a mixed culture. Following Miller et al. (2005), the community composition predictions were that (i) species dominance would change with the resource ratio (Miller's prediction P2), (ii) the number of coexisting species would not outweigh the number of limiting resources (Miller's prediction P3), (iii) resource supply and consumption rates would affect the competition outcome (Miller's predictions P5 and P6, respectively), and (iv) the highest community diversity would occur at intermediate resource ratios (Supplementary Table S2). We also accepted cases where none of the community composition predictions were tested, but invasibility tests were used to confirm the species ranking and tradeoffs (Letten et al., 2017).
“Natural Enemy Partitioning” Mechanism
The “natural enemy partitioning” mechanism is also fluctuation-independent; it assumes that each of the interacting species is controlled by a different enemy and predicts an increase in the enemy's regulation with increased victim (see Glossary) densities. Coexistence is retained when the enemies are specialists to some extent and, thus, attack some species more than others and when their attack rate is density dependent. Under these conditions, the enemies increase the niche differences and decrease the fitness differences between competing victim species (Grover, 1994; Chesson and Kuang, 2008). Janzen (1970) formulated the natural enemy partitioning hypothesis using qualitative graphics. Experimental evidence in plants for the Janzen-Connell hypothesis (see Glossary), which is a special case of the natural enemy partitioning mechanism, has been previously reviewed (Hyatt et al., 2003; Carson et al., 2008; Terborgh, 2012; Comita et al., 2014). We broadened these surveys (from 1967) to include other organisms and complemented them by extending them to October 2020 (Supplementary Table S1).
We accepted only studies that assumed, a priori, that an enemy with some victim preferences exists, and that the presence of such an enemy was experimentally manipulated for at least two victim species. For each of the eligible studies, we recorded whether it also met the other theoretical criteria, i.e., confirms enemy specificity, DD-regulation of the specific victim, and enemy effect on victim interspecific interactions (Figure 3C and Supplementary Table S2).
“Frequency-Dependent Exploitation by Generalist Enemies” Mechanism (Designated FD-Exploitation)
The mechanism of “FD-exploitation” is also fluctuation-independent. The tendency of generalist enemies (see Glossary) to exploit their victims (see Glossary) disproportionately when they are abundant and ignore the victims disproportionately when they are rare allows for recovery of the victim species from low densities, as was illustrated by different mathematical approaches (Roughgarden and Feldman, 1975; Hutson, 1984; Kuang and Chesson, 2010). Since the 1970s, theoretical studies have investigated the effect of FD-exploitation on victim coexistence, and in parallel, experimental studies have tested for FD behaviors (Murdoch, 1969; Lawton et al., 1974; Murdoch and Oaten, 1975; Murdoch et al., 1975; Oaten and Murdoch, 1975; Goss-Custard, 1977; Greenwood and Elton, 1979; Greenwood, 1984; Murdoch and Bence, 1987; Allen, 1988; Sherratt and Harvey, 1993; Kuang and Chesson, 2010).
We conducted a literature survey from 1969 until October 2020 for studies that cited any of the above theoretical and experimental papers or that used relevant keywords (Supplementary Table S1). We accepted studies that examined experimentally whether the exploited proportion of a particular victim changes from “less than expected” to “more than expected” as the frequency of that victim increases (Figure 3C). Because victim coexistence was explicitly tested in only two of the 150 papers identified from previous reviews and the database, a proper test of FD-exploitation was a sufficient criterion to select a paper (Supplementary Table S2).
“Storage Effect” Mechanism
Temporal and spatial environmental variability may promote species coexistence if the perception of better conditions is species-specific and if each species can demographically benefit when conditions are better to a greater extent than they are harmed in poor conditions. The dependence on the environmental variability is termed a “fluctuation-dependent mechanism” in the MCT. Among fluctuation-dependent mechanisms, the storage effect specifically promotes coexistence in cases in which two criteria are satisfied: (i) there is density-dependent environment-competition covariance, so that the resident-state covariance between environment and competition is higher than the covariance of the invader-state, and (ii) there is buffered population growth, so that for each species, growth under favorable conditions compensates for the unfavorable conditions, whereby losses are often temporarily buffered through life history traits (e.g., seed banks) (Chesson, 2008; Holt and Chesson, 2016). Importantly, while species-specific responses to the environment are expected to be associated with the first criterion, provided that competition is an increasing function of environmental responses, by themselves, these responses cannot promote coexistence (Chesson, 2008; Holt and Chesson, 2016). Thus resident-invader covariance differences are key elements in the storage effect.
The first comprehensive mathematical model, which was based on the work of Sale (1977), inspired by a coral reef fish system, and constitutes the foundation for all other related models, dealt with the temporal storage effect (Chesson and Warner, 1981). Shortly thereafter, the concept was broadened to include the spatial storage effect and was fitted to other study systems and environments (Warner and Chesson, 1985; Chesson and Huntly, 1989, 1997; Chesson, 1994). Note that since the spatial storage effect operates through the dispersal of the population across a variable environment, with some dispersal between different environmental patches, the buffered population growth criterion is automatically embedded therein.
We conducted a literature survey from 1981 to October 2020 for studies citing these papers of Chesson et al. or using the relevant keywords presented in Supplementary Table S1. We only accepted studies that aimed to test the two criteria (Figure 3C and Supplementary Table S2).
Synthesis Methods: Data Extraction and Analyses
For each eligible paper, we recorded: (i) the number of target species (i.e., species hypothesized to coexist) used to test the hypothesis (invasibility criterion or specific mechanism), (ii) the type of environment (laboratory, field, or outdoor experimental systems in which the species compositions were determined by the researcher, e.g., microcosm, mesocosm, or macrocosm), (iii) the manipulated factors (factors related to the competitor species, their resources and enemies, and their environmental conditions), (iv) whether a model was embedded in the study, (v) the experimental design, (vi) whether the hypothesis was supported by the experimental results, in which, for the invasibility tests that had an a priori prediction about the likelihood that the target species coexist, we recorded whether the prediction was supported or not, and (vii) whether the purported mechanism was associated with mutual invasibility tests (Supplementary Table S2). Some of the studies conducted multiple experiments with either multiple species combinations or with the same species combination under a variety of conditions. For these, we reported “support” when all multiple experiments supported the hypothesis, “no support” for the opposite case, and “partial support” when some experiments supported and others refuted the hypothesis. Furthermore, to account for the variability within each paper, in addition to the exploration at the study level, we extracted, from each eligible paper, all tests conducted for an interacting species combination and gave it a score of either “0” or “1” if less than 60% or at least 60% of the tests supported the theoretical hypotheses, respectively. For each species combination, we also indicated the organisms' group, habitat type, and size (Figures 4B, 5). Considering the small number of eligible studies detected for each coexistence topic and the clear link between particular mechanisms and study systems (Figures 2, 4, 5 and Supplementary Table S2), below we provide a qualitative summary of the results, without statistical analyses.
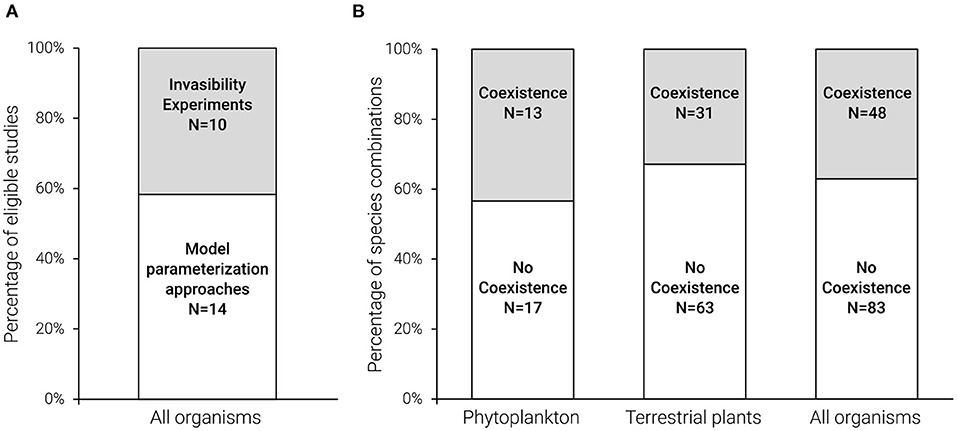
Figure 4. Assessment of coexistence prevalence, illustrating the limited evidence for coexistence in nature. Mutual invasibility tests are methods that are based on the theoretical distinction between stable coexisting and transient co-occurring species, and as such, can be exploited to assess coexistence prevalence in nature. Adequate tests of the mutual invasibility criterion can be conducted either directly by invasibility experiments or indirectly by model parameterization studies (Figure 3B). Our literature survey identified only a few eligible studies based on invasibility tests, and the division of studies between the two methods was similar (A). The two best explored study systems with respect to invasibility tests—phytoplankton and plants (22 and 73% of the 140 tested species combinations, respectively)—provided low (< 44%) support for coexistence (i.e., successful reciprocal invasibility; gray) in species combinations co-occurring in nature (B). Note that the species combinations that do not co-occur in nature are not included in (B).
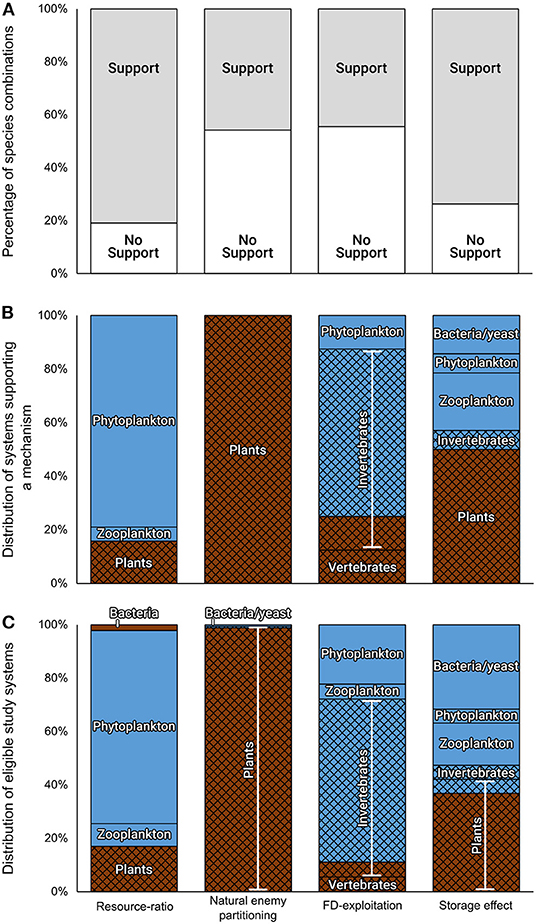
Figure 5. Assessment of the prevalence of the four chosen coexistence mechanisms, illustrating the deficiencies in our current mechanistic knowledge. The experimental support varied among mechanisms, suggesting that their prevalence in nature is different (A). The division of the species combinations that were tested in the eligible studies and support a mechanism, according to the taxonomical groups of the interacting species (white titles), origin (aquatic in blue and terrestrial in brown), and size (microbes on a plain colored background and macroorganisms on a hatched background), demonstrates a link between the coexistence mechanism and the study system (B). However, since the division of supporting evidence among organismal groups was similar to their division among all eligible tested species combinations (C), it was not clear whether these results reflect a tendency of specific groups to be controlled by particular coexistence mechanisms or a bias already introduced during the stage of model-system choice. Taken together, these results suggest that the scarcity of eligible tests and the bias of specific mechanisms toward specific study systems do not permit a comprehensive analysis of the prevalence of the four chosen coexistence mechanisms in nature.
Results
All records initially retrieved by each specific search and records extracted from each eligible paper for each species combination can be found in the Figshare Repository (Hawlena et al., 2022).
The Percentage of Eligible Studies
The initial screening covered 2,510 papers; of these, 89 had been identified by previous reviews and 2,422 were new studies identified through the ISI Web of Knowledge search. Of the initial 2,510 papers screened, 2,035 were excluded after screening of the titles and abstracts, and an additional 379 papers were excluded as their design did not satisfy the theoretical criteria (Figure 2). Among the latter group of ineligible papers, three types of study were rejected because they failed to satisfy the theoretical criteria for invasibility tests: most did not include a manipulation of frequency- or density-dependent interspecific competition (70%); others did not determine mutual invasibility through growth rate assessments (15%), and yet others had not established the resident community before introducing the invader (15%). Thus, in sum, 96% of the papers that incorporated the invasibility terms comprised neither invasibility experiments nor model parameterization studies that evaluate their parameters based on intra- and interspecies competition assays (Figures 1, 2 and Supplementary Table S1). For the resource-ratio mechanism, most studies that failed to satisfy the theoretical criteria included only one resource (54%) or had not calculated R* for all tested species (40%). Others did not include a mixed culture of the species (1%) or did not test any of the predictions about community composition (5%). For the natural enemy partitioning mechanism, most studies that failed to satisfy the theoretical criteria used a generalist predator (36%) or multiple predators that interacted among themselves (28%). The others were observational (8%), did not manipulate the enemy presence (17%) or the densities of the target species (7%), or did not assess the interaction between the victim species (4%). For the FD-exploitation mechanism, most studies that failed to satisfy the theoretical criteria used victim species frequencies of three or less (92%), and one study focused on the predator's perspective using virtual prey (8%). For the storage effect mechanism, most studies that failed to satisfy the theoretical criteria did not test the two storage effect criteria (75%), whereas the others (25%) were observational.
In total, 96 papers were retrieved for detailed evaluation; 14 of them had already been identified by previous reviews (Supplementary Tables S1, S2). The limited number of eligible studies precludes a proper analysis of the effect sizes, and thus, the description of the results provided below is qualitative. The eligible papers and their distribution among categories, with regard to the organisms' group, habitat type, and size, are provided in Figures 4, 5.
The Eligible Studies' Scope and Level of Support for the Theoretical Predictions
Invasibility tests: Of the 24 eligible studies, 42% comprised invasibility experiments, and 58% included model parameterization studies (Figure 4A). Only 50% of these 24 studies associated the mutual invasibility tests with the features of a specific mechanism (column “mechanism-invasibility” in Supplementary Table S2). Importantly, an invasibility-criterion-based examination of the eligible studies that were conducted on species co-occurring in nature revealed that only 37% of the species combinations were predicted to maintain long-term coexistence, suggesting that the current co-occurrence of the other 63% of species combinations may be a transient phenomenon (Figure 4B). The two main tested groups were phytoplankton and terrestrial plants (22 and 73% of the 140 tested species combinations, respectively), and their tendency toward long-term coexistence was similar (Figure 4B).
Four coexistence mechanisms: Support for the resource-ratio (81%, N = 47) and storage effect (74%, N = 19) mechanisms was relatively higher than for the natural enemy partitioning (46%, N = 164) and FD-exploitation (44%, N = 18) mechanisms (Figure 5A). The species combinations that supported each mechanism were not randomly distributed among groups, but were dominated by phytoplankton, terrestrial plants, aquatic invertebrates, and terrestrial plants, for the resource-ratio, natural enemy partitioning, FD-exploitation, and storage effect mechanisms, respectively (Figure 5B). However, the division of supporting evidence among organismal groups was similar to their division among all eligible studies (Figure 5C).
The storage effect mechanism was best associated with the mutual invasibility tests; 73% of its eligible studies included these tests (column “experimental design” in Supplementary Table S2). In contrast, none of the eligible studies of the FD-exploitation mechanism included mutual invasibility tests, while only two studies exploring the resource-ratio mechanism and only one study exploring the natural enemy partitioning mechanism included these tests (Chung and Rudgers, 2016; Letten et al., 2017; column “mechanism-invasibility” in Supplementary Table S2). Studies exploring the three latter mechanisms sometimes used alternative methods to associate the feature of their mechanisms with coexistence proxies. For example, to explore the effect of FD-exploitation on coexistence, Ishii and Shimada (2012) manipulated the attack rate and correlated it with the time until competitive exclusion. Similarly, in all eligible studies of the resource-ratio mechanism, the outcomes of interspecific competition were experimentally tested with respect to the limiting resources and supply rates in question, and they were compared with the corresponding predictions. Finally, in nine of the 27 eligible studies of the natural enemy partitioning mechanism, either the density-dependent effect was associated with an increased species diversity, the enemy effect was associated with the strength of interspecific competition, or the long-term community response was estimated through model trajectories (column “experimental design” in Supplementary Table S2).
The Eligible Studies' Implications
A mechanistic exploration of species coexistence may have overarching implications for our understanding of the structure and function of natural communities. Here, we used the reviewed papers on the five coexistence topics to highlight examples from which insights could be gained by applying a mechanistic approach.
Mutual invasibility tests are derived from the theoretical distinction between stable coexisting and co-occurring species and therefore play an important role in bridging theoretical-experimental gaps. In addition to their direct function in predicting species' long-term coexistence, mutual invasibility tests can improve our ability to determine the spatial scale of coexistence (Hart et al., 2017), assess changes in species dynamics (Stein et al., 2016), and predict where and when biodiversity will persist. Invasibility tests can also be used to investigate evolutionary questions and phenological effects on species coexistence (Hart et al., 2019; Blackford et al., 2020).
Investigating the resource-ratio mechanism adds insights into bottom-up community regulation, highlighting the most important resources that determine the community structure and identifying the species-specific limiting factors. Such information can improve our ability to forecast succession, disturbances, trophic interactions, and global change effects (e.g., Luimstra et al., 2020), and to evaluate the role of resource competition in habitat changes and disease (Bell et al., 1990; Zhang et al., 2015). An exploration of the resource-ratio mechanism can also be used to predict higher-level community patterns, such as the associations between productivity and species diversity (Harpole and Suding, 2011). Insights into top-down community regulation may be drawn from the experimental exploration of the natural enemy partitioning and FD-exploitation mechanisms. A better understanding of the natural enemy partitioning mechanism can improve our ability to predict the changes in community structure and dynamics in the face of specialized pathogens and other invasive enemies and to design potential solutions to regulate them (Liu and Stiling, 2006). A better understanding of the FD-exploitation mechanism can improve our understanding of frequency-dependent evolutionary feedbacks, apparent mutualism and commensalism, and predator coexistence (Gaymer et al., 2001; Clay et al., 2007; Long et al., 2012) and may reveal the causes underlying long-term patterns of population dynamics, such as population cycles (Hanski et al., 1991). Finally, the reviewed papers demonstrate that insights into fluctuation-dependent mechanisms, such as the storage effect, can improve our understanding of community responses to changing conditions, which is fundamental to the study of natural communities. The specific attention given in this mechanism to the potential change in interaction outcomes, under different habitat and temporal conditions, can shed light on various natural phenomena, such as Hutchinson's paradox (Descamps-Julien and Gonzalez, 2005), and human-induced changes, as in the case of invasive species (Epstein et al., 2019).
Research Gaps Reflected in the Eligible Studies
Invasibility Tests
The evaluation of the eligible invasibility tests revealed two main theoretical-experimental gaps. First, to simulate rarity, most invasibility experiments added low invader numbers to established resident communities of the competitor species, thereby increasing the chances for demographic stochasticity that, theoretically, should not exist. Second, most of the invasibility experiments were short-term, in violation of the steady-state assumption. Finally, most (88%) of the studies focused on pairwise interactions, while in nature, most communities are composed of more than two species.
Four Coexistence Mechanisms
An evaluation of the eligible studies of the four coexistence mechanisms revealed a few theoretical-experimental gaps. For the resource-ratio mechanism, the main drawback was the lack of quantification of the consumption vectors, the supply vectors, and the ZNGIs that are necessary for generating quantitative predictions (52% of the studies). For the natural enemy partitioning mechanism, the eligible studies rarely tested all the theoretical criteria, including the enemies' level of preference for victim species, the density-dependent regulation of each victim, and the enemy effect on victim interspecific interactions (only 30% of the eligible studies). For the FD-exploitation mechanism, the eligible studies rarely combined invasibility tests or competition trials with FD experiments (only 22% of the eligible studies). For the storage effect mechanism, the fluctuations in the environment were mostly manipulated for short time periods (days). More generally, the incorporation of outdoor experimental systems, field observations, and field manipulations was limited for the experimental tests of all the mechanisms except the natural enemy partitioning mechanism (4, 27, 44, and 78%, for the resource-ratio, storage effect, FD-exploitation, and natural enemy partitioning mechanisms, respectively), calling into question the relevance of the tests to natural settings. However, probably the most alarming deficiency in the experiments that explore coexistence mechanisms is the lack of association with demonstrated stability.
Discussion
The systematic review of the chosen coexistence topics emphasizes that restoring the mechanistic approach, in parallel with making ongoing progress with the phenomenological approach, is important. Despite the overarching implications and insights that can be gained by such a mechanistic approach, our review suggests that the experimental support for the chosen coexistence topics is lacking to such an extent that today—more than 50 years after the formulation of the theories—we do not have sufficient experimental evidence to assess the prevalence of coexistence, nor that of each of the coexistence mechanisms, or the dependence of the mechanisms on the competing organisms' natural history. Below, we discuss the extent of this deficiency in experimental studies and of the interdisciplinary gap and the reasons for these gaps, and we offer avenues for bridging these gaps. We predict that an integration of phenomenological and mechanistic approaches will broaden our understanding of species occurrence patterns in nature.
Extent of the Deficiency in Experimental Studies and of the Interdisciplinary Gap
In contrast to the growing interest in coexistence and the remarkable progress in the theoretical and phenomenological approach (e.g., Levine et al., 2017; Saavedra et al., 2017; Godoy et al., 2018; Ke and Letten, 2018; Ocampo-Ariza et al., 2018; Gallego et al., 2019; Spaak and De Laender, 2020), our review suggests that experimental support for specific coexistence mechanisms lags behind (Figures 1, 2). The mechanistic approach lags behind to such an extent that we do not have sufficient experimental evidence to assess the prevalence of coexistence for the following reasons: First, while coexistence theory suggests that the invasibility criterion is one of the key methods to distinguish coexistence from co-occurrence, our literature search revealed that most of the papers that incorporated the invasibility terms did not include invasibility tests. Second, the theoretical-experimental gaps (e.g., the occurrence of demographic stochasticity and short experimental periods), the scarcity of tests with a complete design that reflects the natural settings (e.g., multiple species and mutual tests), and the study bias toward terrestrial plants (Figure 4B) restrict our ability to quantify the extent of the experimental support for the invasibility criterion in co-occurring species.
Considering the assessment of each of the coexistence mechanisms' prevalence, support for the resource-ratio and storage effect mechanisms was higher than that for the natural enemy partitioning and FD-exploitation mechanisms (Figure 5A). This difference suggests that the prevalence of a mechanism is not related to the nature of the mechanism's dependence on environmental variation. Instead, the results may reflect a tendency toward lower support for mechanisms mediated by enemies (i.e., top-down control) than for mechanisms mediated by resources (i.e., bottom-up control), reinforcing the conclusion that top-down interactions act mainly as fitness equalizing mechanisms (Bartomeus and Godoy, 2018); hence, without mechanisms that enhance the niche differences between competing species, these interactions are less likely to maintain stable coexistence. However, here too, the theoretical-experimental gaps (e.g., restricting the experiment to short periods, not testing core theoretical predictions, and not associating each mechanism's features with demonstrated stabilization), the scarcity of tests with a complete design that reflects the natural setting (e.g., outdoor experiments, field observations, and field manipulations), and the bias of specific mechanisms toward specific study systems (Figure 5C) did not permit a comprehensive analysis.
Similarly, our review suggests that we do not have sufficient experimental evidence to determine the dependence of the mechanisms on the natural history of the competing organisms, partly due to a linkage between the target mechanism and the study system. In particular, the resource-ratio mechanism was supported mainly in plankton, the natural enemy partitioning mechanism in plant-soil microbial systems, the FD-exploitation mechanism in invertebrates, and the storage effect hypothesis in short-lived organisms (Figure 5B). However, the division of supporting evidence among organismal groups was similar to their division among all eligible studies (Figure 5C). Considering that, in general, studies supporting a theory are more likely to be reported than studies with negative results, we may speculate that the mechanism-system linkage reflects a tendency of specific groups to be controlled by particular coexistence mechanisms. However, an inherent system-specific bias precludes such an explanation, and currently, we cannot reject the alternative explanation that the bias had already been introduced at the stage of model system choice. Although our systematic review used general, rather than system-specific, ecological key terms, it revealed that coexistence experiments are limited to a handful of study systems, mostly annual plants, aquatic invertebrates, and plankton (Figure 5C). This bias may reflect both the research interests of the leading research groups and practical methodological difficulties.
Though we intentionally chose to focus the review on a set of well-known mechanisms, our synthesis suggests that the experimental focus is neither uniformly distributed among mechanisms, nor is it determined by their theoretical coverage or the publication of influential papers (Figure 1). For example, over the years, the resource-ratio mechanism has attracted significantly more interest than the FD-exploitation mechanism, but the eligible experimental papers represent only 1 and 2% of the relevant studies of these two mechanisms, respectively. This narrow range of studies, together with methodological variability (e.g., the variation in the percentage use of laboratory experiments) and the tendency of experimental studies to focus on a single mechanism, preclude definitive inferences about the relative importance of the various mechanisms in maintaining species coexistence in nature.
Reasons for the Theoretical-Experimental Gaps
Reviewing the above five topics allowed us to identify three main reasons for the current lack of coexistence experiments. Below, we discuss each of these reasons.
Interdisciplinary Gaps
The review of the five topics reflects a gap in knowledge transfer between disciplines. The gap is probably due to the independent development of the theories that included the use of discipline-specific terminology and mechanistic formulations in the models (compare Kneitel and Chase, 2004; Letten et al., 2017; Kotler and Brown, 2020). This gap may explain, at least partly, the different levels of research interest in particular mechanisms and the observed linkage between the target mechanism and the study system, which precludes a more comprehensive comparison of coexistence mechanisms among groups. It also limits the theories' accessibility to experimentalists, who are often aware only of the theories that fit their discipline-specific terminologies, scales, and schools of thought.
The Theory Is Not Accessible to Experimentalists
Converting theory to testable hypotheses is challenging due to the complex nature of models and their multifaceted nature (as illustrated in the section “search strategy and selection criteria for each review topic”). Thus, on some occasions, the experiment may not be suitably designed to address the target hypothesis (e.g., natural enemy partitioning and the storage effect), the study assumptions may not be justifiable (e.g., assuming coexistence without conducting invasibility or alternative tests), or parallel concepts may be explored independently (e.g., the analogy between natural enemy partitioning and the “killing the winner” hypotheses; see Glossary).
An Ongoing Tension Between the Theory's Strict Demands and Experimental Constraints
After “converting” a theory to testable hypotheses, it is often challenging for experimentalists to design an experiment that properly test them. This theoretical-experimental tension may partly explain the prevalence of experimental tests for the resource-ratio mechanism, which is relatively easy to test compared to the storage effect mechanism (Figure 2).
Future Directions to Bridge the Current Gaps
Below, we offer strategies to overcome the above-mentioned interdisciplinary, accessibility, and theoretical challenges in future work.
Formulating Testable Hypotheses for Each Mechanism, Using Common Terms
The formulation, using common terms, of testable hypotheses for each mechanism will bridge interdisciplinary gaps and make theories more accessible to experimentalists. For example, hypotheses can be descriptively formulated according to underlying trade-offs (e.g., resource-ratio mechanism) and the dependence of mechanisms on (i) species networks (e.g., intransitive interactions; Levine et al., 2017), (ii) additional biotic interactions (e.g., natural enemy partitioning and FD-exploitation), and (iii) environmental variability (e.g., storage effect) (Hawlena, 2022). We illustrated, with the four chosen mechanisms, how this can be done (see section “search strategy and selection criteria for each review topic”). The hypotheses should be formulated by considering specific theoretical assumptions—for example, the need to introduce the invader species at a low number of individuals per unit area while having many individuals at the regional level (assuming that the scale of competition is shorter than the dispersal scale), to prevent demographic stochasticity when testing the invasibility criterion. Moreover, the hypotheses should associate the mechanisms' features with demonstrated stabilization, such as mutual invasibility or the density and frequency dependence of long-term growth rates, and the theory can assist in formulating this association. For example, recent theoretical developments suggest that to demonstrate that the features of the storage effect mechanisms are stabilizing in any given system, one needs to demonstrate that the resident-state covariance between environment and competition is higher than the covariance of the invader-state (i.e., criterion 1 of the storage effect; Sears and Chesson, 2007; Chesson, 2008; Holt and Chesson, 2016) and that the buffered population growth criterion (criterion 2 of the storage effect), which is inherent in the spatial storage effect, is satisfied (Figure 3).
Determining the Required Experimental Design for Each Mechanism, Using Common Components
The determination, using common components, of the required experimental design for each mechanism will further bridge interdisciplinary gaps and make the theories more accessible to experimentalists. Most mechanisms rely on frequency-dependent and density-dependent species interactions (Adler et al., 2018). Accordingly, although the experimental conditions may differ, most experiments should assess the population response of the target species when each species is maintained alone and in a species mixture, at low and high densities (Figure 3A). We illustrated how such a basic design can be adjusted to different mechanisms by proposing a desirable experimental design for each of the four chosen mechanisms (Figure 3C). Obviously, experimental designs for other mechanisms would be different, involving alternative techniques and experimental conditions, but all should basically include the same density- and frequency-dependent components (Hawlena, 2022).
Broadening the Scope of Experimental Studies Through Interdisciplinary Communication
In considering the choice of the study system, we encourage researchers to extend the tests of coexistence mechanisms to under-represented model groups. Figure 5C, highlights the under-represented study systems that should be investigated in the context of the four chosen mechanisms, and more generally, this figure highlights the host-associated organisms and free-living terrestrial microbial, invertebrate, and vertebrate systems that are particularly neglected study systems.
Broadening the range of explored study systems will be made possible through interdisciplinary communication. For example, reciprocal transplant designs that are commonly used in plant ecology can be exploited for testing the invasibility criterion in other systems, such as host-associated microbes and vertebrates (e.g., using field enclosures). Broadening the system scope of the resource-ratio mechanism can be achieved by techniques, such as giving up densities (GUD, the remaining quantity of food when a consumer quits a patch; Bedoya-Perez et al., 2013) and Fretwell-based isoclines (FBI, a method for estimating species' zero isoclines from short-term behavioral experiments in natural communities; Abramsky et al., 1991), which can be exploited to quantify consumption vectors and ZNGIs in various organisms, including aquatic taxa placed in open systems and terrestrial animals fed by artificial apparatuses. The system scope of the natural enemy partitioning mechanism can be broadened by reconciling this mechanism with the “killing the winner” hypothesis, which focuses on microbes. The system scope of the FD-exploitation mechanism can be expanded by using GUDs to quantify consumption (Morgan and Brown, 1996) and extending the tests from predation (72% of the tested species combinations) to other exploitation interactions and to predatory microbes and longer-lived predators, with switching potential (e.g., enemies with functional response type 3, which have high potential to behave in an FD manner, because they often disproportionately exploit victims when they are highly available; Ellison and Gotelli, 2002). The system scope of the storage effect mechanism can be broadened by extending the tests to long-lived species. This can be done by removal manipulations or by quantifying the fitness-correlative traits (instead of determining the growth rate) of organisms living in distinct environments with fluctuating conditions, such as water pools and fragmented habitats (Sears and Chesson, 2007).
Considering the bias of the reviewed studies toward specific mechanisms, we urge experimentalists to design studies that will simultaneously test multiple mechanisms in a single model system. Such an integrative approach is indeed possible and highly productive, and it provides opportunities to compare the relative roles that the different mechanisms play in promoting species coexistence and to assess whether the mechanisms' effect on coexistence is additive or interactive (e.g., Kuang and Chesson, 2010; Zepeda and Martorell, 2019). In particular, considering the similarities in the experimental designs of resource-based and fluctuation-dependent mechanisms, these two mechanisms can be tested in the same study setup (Letten et al., 2018). Likewise, the FD-exploitation and the natural enemy partitioning mechanisms can be simultaneously tested through a full factorial design, including multiple victim densities and frequencies and a manipulation of both enemy types (Figure 3C; Hawlena, 2022).
Integration of Experimental Tests With Field Data and Population Dynamics Models
To address the practical challenges that the theoretical criteria entail while considering the relevant spatial and temporal scales, we encourage the integration of experimental tests with field data and population dynamics models. Such an integration between experimental tests and population dynamics models is already prevalent in invasibility tests (i.e., model parametrization studies; Figure 4A) and in tests of the storage effect (82% of the eligible studies), and would be particularly helpful in tests of the natural enemy partitioning and FD-exploitation mechanisms (column “model” in Supplementary Table S2). To adjust the theories into parametrized forms, theoreticians need to develop appropriate models that can fit organisms' natural histories, including those with complex and multi-stage life cycles. A better integration of experimental data into models will allow for experimental testing of invasibility and coexistence mechanisms in long-lived organisms (e.g., tropical trees, whose age- and size-specific mortality depends on the distance from conspecifics) and more complicated communities (e.g., organisms that reproduce through both sexual and asexual reproduction). It will also assist in associating specific mechanisms with demonstrated stabilization and in comparing the relative roles that various coexistence mechanisms play in a given community (Kuang and Chesson, 2010; Letten et al., 2018; Ellner et al., 2019).
Conclusions
Our synthesis highlights the need to restore the mechanistic approach to species coexistence in parallel with continuing to make progress in the phenomenological approach. Through a systematic review of five coexistence topics, we highlighted the overarching implications of mechanistic explorations and demonstrated that there is still a great deal of room for experimental research on the more classical mechanistic coexistence topics, including the basic distinction between species co-occurrence and coexistence. We also identified three main reasons for the current lack of coexistence experiments, namely, interdisciplinary gaps, lack of accessibility of the theory to experimentalists, and the tension between theory and experimental challenges. To overcome these obstacles, we offered directions for the future. Determining the required experimental design for each mechanism, using common components, and broadening the scope of experimental studies through interdisciplinary communication are expected to bridge interdisciplinary gaps and make the theories more accessible to experimentalists. The integration of experimental tests with field data and population dynamics models is proposed as a means to reduce the tension between the theory and experimental challenges. Using the five chosen topics, we illustrated the ways in which a mechanism's hypotheses can be formulated descriptively, theoretical criteria can be defined, and experiments can be uniformly designed, despite the multifaceted and complex theoretical models. We also illustrated how interdisciplinary communication can contribute to broadening the explored scope of each coexistence topic, opening opportunities to investigate unexplored study systems and conditions. We predict that this systematic review will serve as a proof of concept, emphasizing the usefulness and relevance to experimentalists of the coexistence theory. As such, it should convince leading experimentalists from a range of disciplines, who incorporate coexistence concepts in their studies, to adopt the mechanistic approach to coexistence. Together with continuous progress in the phenomenological approach, progress in the mechanistic approach will make our understanding of coexistence in nature more comprehensive.
Data Availability Statement
Publicly available articles were analyzed in this study. This data can be found here: https://figshare.com/s/06d9d65689794edaf3d7.
Author Contributions
HH contributed to the conception, design, interpretation of data, drafted the manuscript, and accepts responsibility for the integrity of the data analyzed. HH and MG contributed to the data synthesis. All authors were involved in data acquisition and revised its intellectual content and approved the final version of the submitted manuscript.
Funding
This study was supported by the Israel Science Foundation (ISF), Grant No. 1391/15 to HH.
Conflict of Interest
The authors declare that the research was conducted in the absence of any commercial or financial relationships that could be construed as a potential conflict of interest.
Publisher's Note
All claims expressed in this article are solely those of the authors and do not necessarily represent those of their affiliated organizations, or those of the publisher, the editors and the reviewers. Any product that may be evaluated in this article, or claim that may be made by its manufacturer, is not guaranteed or endorsed by the publisher.
Acknowledgments
We thank M. Seifan for her assistance in the early stages of the review, A. Siepielski for insightful discussions on trends in coexistence research, and A. Tsairi for his valuable help with the manuscript.
Supplementary Material
The Supplementary Material for this article can be found online at: https://www.frontiersin.org/articles/10.3389/fevo.2022.898074/full#supplementary-material
References
Abramsky, Z., Rosenzweig, M. L., and Pinshow, B. (1991). The shape of a gerbil isocline measured using principles of optimal habitat selection. Ecology 72, 329–340. doi: 10.2307/1938926
Adler, P. B., HilleRisLambers, J., and Levine, J. M. (2007). A niche for neutrality. Ecol. Lett. 10, 95–104. doi: 10.1111/j.1461-0248.2006.00996.x
Adler, P. B., Smull, D., Beard, K. H., Choi, R. T., Furniss, T., Kulmatiski, A., et al. (2018). Competition and coexistence in plant communities: intraspecific competition is stronger than interspecific competition. Ecol. Lett. 21, 1319–1329. doi: 10.1111/ele.13098
Agrawal, A. A., Ackerly, D. D., Adler, F., Arnold, A. E., Caceres, C., Doak, D. F., et al. (2007). Filling key gaps in population and community ecology. Front. Ecol. Environ. 5, 145–152. doi: 10.1890/1540-9295(2007)5[145:FKGIPA]2.0.CO;2
Allen, J. A (1988). Frequency-dependent selection by predators. Philos. Trans. R. Soc. Lond. B Biol. Sci. 319, 485–503. doi: 10.1098/rstb.1988.0061
Bartomeus, I., and Godoy, O. (2018). Biotic controls of plant coexistence. J. Ecol.106, 1767–1772. doi: 10.1111/1365-2745.13040
Bedoya-Perez, M. A., Carthey, A. J. R., Mella, V. S. A., McArthur, C., and Banks, P. B. (2013). A practical guide to avoid giving up on giving-up densities. Behav. Ecol. Sociobiol. 67, 1541–1553. doi: 10.1007/s00265-013-1609-3
Bell, C. R., Cummings, N. E., Canfield, M. L., and Moore, L. W. (1990). Competition of octopine-catabolizing Pseudomonas spp. and octopine-type Agrobacterium tumefaciens for octopine in chemostats. Appl. Environ. Microbiol. 56, 2840–2846. doi: 10.1128/aem.56.9.2840-2846.1990
Blackford, C., Germain, R. M., and Gilbert, B. (2020). Species differences in phenology shape coexistence. Am. Nat. 195, e168–e180. doi: 10.1086/708719
Broekman, M. J. E., Muller-Landau, H. C., Visser, M. D., Jongejans, E., Wright, S. J., and de Kroon, H. (2019). Signs of stabilisation and stable coexistence. Ecol. Lett. 22, 1957–1975. doi: 10.1111/ele.13349
Carson, W. P., Anderson, J. T., Leigh, E. G., and Schnitzer, S. A. (2008). “Challenges associated with testing and falsifying the Janzen-Connell hypothesis: a review and critique,” in Tropical Forest Community Ecology, eds W. P. Carson and S. A. Schnitzer (Chichester, UK: Wiley-Blackwell), 210–241.
Chesson, P (1990). Macarthur consumer-resource model. Theor. Popul. Biol. 37, 26–38. doi: 10.1016/0040-5809(90)90025-Q
Chesson, P (1994). Multispecies competition in variable environments. Theor. Popul. Biol. 45, 227–276. doi: 10.1006/tpbi.1994.1013
Chesson, P (2000a). General theory of competitive coexistence in spatially-varying environments. Theor. Popul. Biol. 58, 211–237. doi: 10.1006/tpbi.2000.1486
Chesson, P (2000b). Mechanisms of maintenance of species diversity. Annu. Rev. Ecol. Syst. 31, 343–366. doi: 10.1146/annurev.ecolsys.31.1.343
Chesson, P (2008). “Quantifying and testing species coexistence mechanisms,” in Unity in diversity: reflections on ecology after the legacy of ramon margalef, eds F. Valladares, A. Camacho, A. Elosegui, C. Gracia, M. Estrada, J. C. Senar and J. M. Gili (Vizcaya: Fundación Banco Bilbao), 119–164.
Chesson, P (2018). Updates on mechanisms of maintenance of species diversity. J. Ecol. 106, 1773–1794. doi: 10.1111/1365-2745.13035
Chesson, P (2020). Chesson's coexistence theory: comment. Ecology 101, e02851. doi: 10.1002/ecy.2851
Chesson, P., and Huntly, N. (1989). Short-term instabilities and long-term community dynamics. Trends Ecol. Evol. 4, 293–298. doi: 10.1016/0169-5347(89)90024-4
Chesson, P., and Huntly, N. (1997). The roles of harsh and fluctuating conditions in the dynamics of ecological communities. Am. Nat. 150, 519–553. doi: 10.1086/286080
Chesson, P., and Kuang, J. J. (2008). The interaction between predation and competition. Nature 456, 235–238. doi: 10.1038/nature07248
Chesson, P. L., and Warner, R. R. (1981). Environmental variability promotes coexistence in lottery competitive-systems. Am. Nat. 117, 923–943. doi: 10.1086/283778
Chung, Y. A., and Rudgers, J. A. (2016). Plant-soil feedbacks promote negative frequency dependence in the coexistence of two aridland grasses. Proc. R. Soc. B Biol. Sci. 283, ARTN 20160608. doi: 10.1098/rspb.2016.0608
Clay, K., Reinhart, K., Rudgers, J., Tintjer, T., Koslow, J., and Flory, L. S. (2007). “Red queen communities,” in Ecology of Infectious Diseases: Interactions Between Diseases and Ecosystems, eds V. Eviner, F. Keesing and R. Ostfeld (Princeton, NJ: Princeton University Press) 145–178.
Comita, L. S., Queenborough, S. A., Murphy, S. J., Eck, J. L., Xu, K. Y., Krishnadas, M., et al. (2014). Testing predictions of the Janzen-Connell hypothesis: a meta-analysis of experimental evidence for distance- and density-dependent seed and seedling survival. J. Ecol. 102, 845–856. doi: 10.1111/1365-2745.12232
Connell, J. H (1971). “On the role of natural enemies in preventing competitive exclusion in some marine animals and rain forest trees,” in Dynamics of Populations, eds P. J. D. Boer and G. R. Gradwell (Wageningen: Center for Agricultural Publication and Documentation), 298–312.
Descamps-Julien, B., and Gonzalez, A. (2005). Stable coexistence in a fluctuating environment: an experimental demonstration. Ecology 86, 2815–2824. doi: 10.1890/04-1700
Eidelman, A., Cohen, C., Navarro-Castilla, A., Filler, S., Gutierrez, R., Bar-Shira, E., et al. (2019). The dynamics between limited-term and lifelong coinfecting bacterial parasites in wild rodent hosts. J. Exp. Biol. 222, ARTN jeb203562. doi: 10.1242/jeb.203562
Ellison, A. M., and Gotelli, N. J. (2002). Nitrogen availability alters the expression of carnivory in the northern pitcher plant, Sarracenia purpurea. Proc. Natl. Acad. Sci. U. S.A. 99, 4409–4412. doi: 10.1073/pnas.022057199
Ellner, S. P., Snyder, R. E., Adler, P. B., and Hooker, G. (2019). An expanded modern coexistence theory for empirical applications. Ecol. Lett. 22, 3–18. doi: 10.1111/ele.13159
Epstein, G., Hawkins, S. J., and Smale, D. A. (2019). Identifying niche and fitness dissimilarities in invaded marine macroalgal canopies within the context of contemporary coexistence theory. Sci. Rep. 9, ARTN 8816. doi: 10.1038/s41598-019-45388-5
Gallego, I., Venail, P., and Ibelings, B. W. (2019). Size differences predict niche and relative fitness differences between phytoplankton species but not their coexistence. ISME J. 13, 1133–1143. doi: 10.1038/s41396-018-0330-7
Gause, G. F (1934). Experimental analysis of Vito Volterra's mathematical theory of the struggle for existence. Science 79, 16–17. doi: 10.1126/science.79.2036.16-a
Gaymer, C. F., Himmelman, J. H., and Johnson, L. E. (2001). Use of prey resources by the seastars Leptasterias polaris and Asterias vulgaris: a comparison between field observations and laboratory experiments. J. Exp. Mar. Biol. Ecol. 262, 13–30. doi: 10.1016/S0022-0981(01)00264-7
Germain, R. M., Weir, J. T., and Gilbert, B. (2016). Species coexistence: macroevolutionary relationships and the contingency of historical interactions. Proc. R. Soc. B 283, ARTN 20160047. doi: 10.1098/rspb.2016.0047
Godoy, O., Bartomeus, I., Rohr, R. P., and Saavedra, S. (2018). Towards the integration of niche and network theories. Trends Ecol. Evol. 33, 287–300. doi: 10.1016/j.tree.2018.01.007
Godoy, O., Kraft, N. J., and Levine, J. M. (2014). Phylogenetic relatedness and the determinants of competitive outcomes. Ecol. Lett. 17, 836–844. doi: 10.1111/ele.12289
Godwin, C. M., Chang, F. H., and Cardinale, B. J. (2020). An empiricist's guide to modern coexistence theory for competitive communities. Oikos 129, 1109–1127. doi: 10.1111/oik.06957
Goss-Custard, J. D (1977). Optimal foraging and size selection of worms by redshank, Tringa-Totanus, in field. Anim. Behav. 25, 10–29. doi: 10.1016/0003-3472(77)90063-X
Grainger, T. N., Letten, A. D., Gilbert, B., and Fukami, T. (2019a). Applying modern coexistence theory to priority effects. Proc. Natl. Acad. Sci. U.S.A. 116, 6205–6210. doi: 10.1073/pnas.1803122116
Grainger, T. N., Levine, J. M., and Gilbert, B. (2019b). The invasion criterion: a common currency for ecological research. Trends Ecol. Evol. 34, 925–935. doi: 10.1016/j.tree.2019.05.007
Greenwood, J. J. D (1984). The functional basis of frequency-dependent food selection. Biol. J. Linnean Soc. 23, 177–199. doi: 10.1111/j.1095-8312.1984.tb00137.x
Greenwood, J. J. D., and Elton, R. A. (1979). Analyzing experiments on frequency-dependent selection by predators. J. Anim. Ecol. 48, 721–737. doi: 10.2307/4192
Grover, J. P (1994). Assembly rules for communities of nutrient-limited plants and specialist herbivores. Am. Nat. 143, 258–282. doi: 10.1086/285603
Hallett, L. M., Shoemaker, L. G., White, C. T., and Suding, K. N. (2019). Rainfall variability maintains grass-forb species coexistence. Ecol. Lett. 22, 1658–1667. doi: 10.1111/ele.13341
Hanski, I., Hansson, L., and Henttonen, H. (1991). Specialist predators, generalist predators, and the microtine rodent cycle. J. Anim. Ecol. 60, 353–367. doi: 10.2307/5465
Harpole, W. S., and Suding, K. N. (2011). A test of the niche dimension hypothesis in an arid annual grassland. Oecologia 166, 197–205. doi: 10.1007/s00442-010-1808-9
Hart, S. P., Turcotte, M. M., and Levine, J. M. (2019). Effects of rapid evolution on species coexistence. Proc. Natl. Acad. Sci. U.S.A. 116, 2112–2117. doi: 10.1073/pnas.1816298116
Hart, S. P., Usinowicz, J., and Levine, J. M. (2017). The spatial scales of species coexistence. Nat. Ecol. Evolut. 1, 1066–1073. doi: 10.1038/s41559-017-0230-7
Hawlena, H (2022). Coexistence research requires more interdisciplinary communication. Ecol. Evol. 12, ARTN e8914. doi: 10.1002/ece3.8914
Hawlena, H., Garrido, M., Cohen, C., Halle, S., and Cohen, S. (2022). Bringing the Mechanistic Approach back to Life: A Systematic Review of the Experimental Evidence for Coexistence and Four of Its Classical Mechanisms [Online]. Figshare Repository. Available online at: https://figshare.com/s/06d9d65689794edaf3d7.
Holt, G., and Chesson, P. (2016). Scale-dependent community theory for streams and other linear habitats. Am. Nat. 188, E59–E73. doi: 10.1086/687525
Hutson, V (1984). Predator mediated coexistence with a switching Predator. Math. Biosci. 68, 233–246. doi: 10.1016/0025-5564(84)90033-6
Hyatt, L. A., Rosenberg, M. S., Howard, T. G., Bole, G., Fang, W., Anastasia, J., et al. (2003). The distance dependence prediction of the Janzen-Connell hypothesis: a meta-analysis. Oikos 103, 590–602. doi: 10.1034/j.1600-0706.2003.12235.x
Ishii, Y., and Shimada, M. (2012). Learning predator promotes coexistence of prey species in host-parasitoid systems. Proc. Natl. Acad. Sci. U.S.A. 109, 5116–5120. doi: 10.1073/pnas.1115133109
Janzen, D. H (1970). Herbivores and the number of tree species in tropical forests. Am. Nat. 104, 501–528. doi: 10.1086/282687
Ke, P. J., and Letten, A. D. (2018). Coexistence theory and the frequency-dependence of priority effects. Nat. Ecol. Evolut. 2, 1691–1695. doi: 10.1038/s41559-018-0679-z
Ke, P. J., and Wan, J. (2020). Effects of soil microbes on plant competition: a perspective from modern coexistence theory. Ecol. Monogr. 90, ARTN e01391. doi: 10.1002/ecm.1391
Kneitel, J. M., and Chase, J. M. (2004). Trade-offs in community ecology: linking spatial scales and species coexistence. Ecol. Lett. 7, 69–80. doi: 10.1046/j.1461-0248.2003.00551.x
Kotler, B. P., and Brown, J. S. (2020). Cancer community ecology. Cancer Control 27, ARTN 1073274820951776. doi: 10.1177/1073274820951776
Kraft, N. J., Godoy, O., and Levine, J. M. (2015). Plant functional traits and the multidimensional nature of species coexistence. Proc. Natl. Acad. Sci. U.S.A. 112, 797–802. doi: 10.1073/pnas.1413650112
Kuang, J. J., and Chesson, P. (2010). Interacting coexistence mechanisms in annual plant communities: frequency-dependent predation and the storage effect. Theor. Popul. Biol. 77, 56–70. doi: 10.1016/j.tpb.2009.11.002
Lawton, J. H., Beddington, J. R., and Bonser, R. (1974). “Switching in invertebrate predators,” in Ecological Stability, eds. M. B. Usher and M. H. Williamson (London: Chapman and Hall, London), 141–158.
Letten, A. D., Dhami, M. K., Ke, P. J., and Fukami, T. (2018). Species coexistence through simultaneous fluctuation-dependent mechanisms. Proc. Natl. Acad. Sci. U.S.A. 115, 6745–6750. doi: 10.1073/pnas.1801846115
Letten, A. D., Ke, P. J., and Fukami, T. (2017). Linking modern coexistence theory and contemporary niche theory. Ecol. Monogr. 87, 161–177. doi: 10.1002/ecm.1242
Levine, J. M., Bascompte, J., Adler, P. B., and Allesina, S. (2017). Beyond pairwise mechanisms of species coexistence in complex communities. Nature 546, 56–64. doi: 10.1038/nature22898
Liberati, A., Altman, D. G., Tetzlaff, J., Mulrow, C., Gotzsche, P. C., Ioannidis, J. P. A., et al. (2009). The PRISMA statement for reporting systematic reviews and meta-analyses of studies that evaluate health care interventions: explanation and elaboration. Ann. Intern. Med. 151, W65–W94. doi: 10.7326/0003-4819-151-4-200908180-00136
Liu, H., and Stiling, P. (2006). Testing the enemy release hypothesis: a review and meta-analysis. Biol. Invasions 8, 1535–1545. doi: 10.1007/s10530-005-5845-y
Long, W. C., Gamelin, E. F., Johnson, E. G., and Hines, A. H. (2012). Density-dependent indirect effects: apparent mutualism and apparent competition coexist in a two-prey system. Mar. Ecol. Prog. Ser. 456, 139–148. doi: 10.3354/meps09702
Luimstra, V. M., Verspagen, J. M. H., Xu, T. S., Schuurmans, J. M., and Huisman, J. (2020). Changes in water color shift competition between phytoplankton species with contrasting light-harvesting strategies. Ecology 101, ARTN e02951. doi: 10.1002/ecy.2951
MacArthur, R (1972). Geographical Ecology: Patterns in the Distribution of Species. New York, NY: Princeton University Press.
Mayfield, M. M., and Levine, J. M. (2010). Opposing effects of competitive exclusion on the phylogenetic structure of communities. Ecol. Lett. 13, 1085–1093. doi: 10.1111/j.1461-0248.2010.01509.x
McPeek, M. A (2012). Intraspecific density dependence and a guild of consumers coexisting on one resource. Ecology 93, 2728–2735. doi: 10.1890/12-0797.1
Miller, T. E., Burns, J. H., Munguia, P., Walters, E. L., Kneitel, J. M., Richards, P. M., et al. (2005). A critical review of twenty years' use of the resource-ratio theory. Am. Nat. 165, 439–448. doi: 10.1086/428681
Morgan, R. A., and Brown, J. S. (1996). Using giving-up densities to detect search images. Am. Nat. 148, 1059–1074. doi: 10.1086/285971
Murdoch, W. W (1969). Switching in general predators. Experiments on predator specificity and stability of prey populations. Ecol. Monogr. 39, 335-355. doi: 10.2307/1942352
Murdoch, W. W., Avery, S., and Smyth, M. E. B. (1975). Switching in predatory fish. Ecology 56, 1094–1105. doi: 10.2307/1936149
Murdoch, W. W., and Bence, J. (1987). “General predators and unstable prey populations,” in Predation: Direct and Indirect Impacts on Aquatic Communities, eds W. C. Kerfoot and A. Sih (London: University Press of New England), 17–30.
Murdoch, W. W., and Oaten, A. (1975). Predation and population stability. Adv. Ecol. Res. 9, 1–131. doi: 10.1016/S0065-2504(08)60288-3
Narwani, A., Alexandrou, M. A., Oakley, T. H., Carroll, I. T., and Cardinale, B. J. (2013). Experimental evidence that evolutionary relatedness does not affect the ecological mechanisms of coexistence in freshwater green algae. Ecol. Lett. 16, 1373–1381. doi: 10.1111/ele.12182
Oaten, A., and Murdoch, W. W. (1975). Switching, functional response, and stability in predator-prey systems. Am. Nat. 109, 299–318. doi: 10.1086/282999
Ocampo-Ariza, C., Bufford, J. L., Hulme, P. E., Champion, P. D., and Godsoe, W. (2018). Strong fitness differences impede coexistence between an alien water fern (Azolla pinnata R. Br.) and its native congener (Azolla rubra R. Br.) in New Zealand. Biol. Invasions 20, 2889–2897. doi: 10.1007/s10530-018-1740-1
Roughgarden, J., and Feldman, M. (1975). Species packing and predation pressure. Ecology 56, 489–492. doi: 10.2307/1934982
Saavedra, S., Rohr, R. P., Bascompte, J., Godoy, O., Kraft, N. J. B., and Levine, J. M. (2017). A structural approach for understanding multispecies coexistence. Ecol. Monogr. 87, 470–486. doi: 10.1002/ecm.1263
Sale, P. F (1977). Maintenance of high diversity in coral-reef fish communities. Am. Nat. 111, 337–359. doi: 10.1086/283164
Sears, A. L. W., and Chesson, P. (2007). New methods for quantifying the spatial storage effect: an illustration with desert annuals. Ecology 88, 2240–2247. doi: 10.1890/06-0645.1
Sherratt, T. N., and Harvey, I. F. (1993). Frequency-dependent food selection by arthropods - a review. Biol. J. Linnean Soc. 48, 167–186. doi: 10.1111/j.1095-8312.1993.tb00885.x
Shoemaker, L. G., Barner, A. K., Bittleston, L. S., and Teufel, A. I. (2020). Quantifying the relative importance of variation in predation and the environment for species coexistence. Ecol. Lett. 23, 939–950. doi: 10.1111/ele.13482
Siepielski, A. M., and McPeek, M. A. (2010). On the evidence for species coexistence: a critique of the coexistence program. Ecology 91, 3153–3164. doi: 10.1890/10-0154.1
Spaak, J. W., and De Laender, F. (2020). Intuitive and broadly applicable definitions of niche and fitness differences. Ecol. Lett. 23, 1117–1128. doi: 10.1111/ele.13511
Stein, C., Harpole, W. S., and Suding, K. N. (2016). Transitions and invasion along a grazing gradient in experimental California grasslands. Ecology 97, 2319–2330. doi: 10.1002/ecy.1478
Terborgh, J (2012). Enemies maintain hyperdiverse tropical forests. Am. Nat. 179, 303–314. doi: 10.1086/664183
Tilman, D (1980). Resources-a graphical mechanistic approach to competition and predation. Am. Nat. 116, 362–393. doi: 10.1086/283633
Tilman, D (1982). Resource Competition and Community Structure. Princeton, NJ: Princeton University Press.
Vage, S., Bratbak, G., Egge, J., Heldal, M., Larsen, A., Norland, S., et al. (2018). Simple models combining competition, defence and resource availability have broad implications in pelagic microbial food webs. Ecol. Lett. 21, 1440–1452. doi: 10.1111/ele.13122
Warner, R. R., and Chesson, P. L. (1985). Coexistence mediated by recruitment fluctuations-a field guide to the storage effect. Am. Nat. 125, 769–787. doi: 10.1086/284379
Wilson, J. B., Spijkerman, E., and Huisman, J. (2007). Is there really insufficient support for Tilman's R* concept? A comment on Miller et al. Am. Nat. 169, 700–706. doi: 10.1086/513113
Zepeda, V., and Martorell, C. (2019). Fluctuation-independent niche differentiation and relative non-linearity drive coexistence in a species-rich grassland. Ecology 100, ARTN e02726. doi: 10.1002/ecy.2726
Zhang, X. F., Mei, X. Y., Gulati, R. D., and Liu, Z. W. (2015). Effects of N and P enrichment on competition between phytoplankton and benthic algae in shallow lakes: a mesocosm study. Environ. Sci. Pollut. Res. 22, 4418–4424. doi: 10.1007/s11356-014-3680-3
Glossary
Co-occurring species: Two or more species that are observed together.
Coexisting species: See “Stable coexistence.”
Consumption vector: A vector that represents the rate of consumption of all resources by a species. It is often calculated using the resource quotients (the amount required to produce one individual, which is the inverse of the yield).
Fluctuation-independent coexistence mechanism: A mechanism that does not require environmental variability in time or space to promote coexistence.
Generalist enemies: Organisms, including predators, parasitoids, parasites, herbivores, and pathogens, that benefit from exploitation of their victims and are not specific to a victim species.
Invader species: Species that are introduced at low densities to a resident community.
Janzen–Connell hypothesis: Janzen (1970) and Connell (1971) suggested that the remarkable diversity in tropical forests and coral reefs derives from the density-dependent nature of specialist enemies of seeds and larvae, which reduces recruitment and survival near conspecific adults and at high offspring densities.
“Killing the winner” hypothesis: A model of population growth rates that was constructed to explain the observed coexistence between multiple species of prokaryotes (or protozoans) and their specialist viruses (Vage et al., 2018). It is based on the victim's trade-off between directing resources to growth (“winner”) or to defense against specialist enemies, and it assumes that enemies will mainly target the “winner.”
Model parameterization study: A study that includes a mathematical model, for which parameters are estimated from experimental data (reviewed in Godwin et al., 2020).
Modern coexistence theory (MCT): A general framework, formalized by the theoretical work of Chesson (e.g., Chesson, 1994, 2000b), for explaining species coexistence across multiple types of competition models. There are two forms of MCT in the literature; the first deals with the universal conditions for coexistence, i.e., those under which stabilizing niche differences will offset relative fitness differences, and it distinguishes between the stabilizing and equalizing mechanisms (i.e., those that increase negative intraspecific interactions relative to negative interspecific interactions and those that minimize fitness differences, respectively) for coexistence (e.g., Mayfield and Levine, 2010; Godoy et al., 2014; Saavedra et al., 2017; Spaak and De Laender, 2020). It mainly maps how species traits and environmental conditions relate to niche and fitness differences and, thus, remains phenomenological (i.e., it does not delve into details of mechanisms). The second provides analytical ways to compare the importance of niche- and fitness-differentiating processes for species coexistence through the various mechanisms, with special consideration of the role played by environmental fluctuations (Chesson, 2008, 2018; Kuang and Chesson, 2010; Ellner et al., 2019). As such, this form of MCT can be used to define a mechanism and to facilitate empirical studies of mechanisms.
R* rule prediction: R* is the level to which a limiting resource is reduced by a monoculture of a consumer species once it has attained its carrying capacity (reproductive rate is equal to mortality rate). Thus, R* is the minimum resource concentration that a species requires to maintain a stable population. The R* rule predicts that when multiple species are competing for a single limiting resource, the species who has the lowest R* will outcompete all others.
Resident community: Assembly of populations of two or more different species at their typical steady-state abundances.
Resource supply ratio: Concentration ratio of the limiting resources available in the environment before consumption by competing species.
Stable coexistence: The long-term co-persistence of species, without any species being competitively excluded by the others, and with each species able to recover from low density.
Victim: An organism that is exploited by enemies, including prey (for predation and herbivory) and hosts (for parasites, pathogens, and parasitoids).
Zero net growth isocline (ZNGI) of resources: Curves in state space that connect all concentration combinations of two resources in which the target consumers have a zero growth rate (birth rate is equal to mortality rate) (see Tilman, 1980).
Keywords: coexistence mechanisms, competition, density-dependence, experiment, frequency dependence, invisibility, modern coexistence theory
Citation: Hawlena H, Garrido M, Cohen C, Halle S and Cohen S (2022) Bringing the Mechanistic Approach Back to Life: A Systematic Review of the Experimental Evidence for Coexistence and Four of Its Classical Mechanisms. Front. Ecol. Evol. 10:898074. doi: 10.3389/fevo.2022.898074
Received: 16 March 2022; Accepted: 31 May 2022;
Published: 27 June 2022.
Edited by:
Lawrence Hurd, Washington and Lee University, United StatesReviewed by:
Rafael D'Andrea, Stony Brook University, United StatesPeter Chesson, University of Arizona, United States
Junpyo Park, Kyung Hee University, South Korea
Copyright © 2022 Hawlena, Garrido, Cohen, Halle and Cohen. This is an open-access article distributed under the terms of the Creative Commons Attribution License (CC BY). The use, distribution or reproduction in other forums is permitted, provided the original author(s) and the copyright owner(s) are credited and that the original publication in this journal is cited, in accordance with accepted academic practice. No use, distribution or reproduction is permitted which does not comply with these terms.
*Correspondence: Hadas Hawlena, aGFkYXNoYXdAYmd1LmFjLmls
†Present address: Mario Garrido, Departamento de Parasitología, Facultad de Farmacia, Universidad de Granada, Granada, Spain
Carmit Cohen, Anxiety and Stress Research Unit, Faculty of Health Sciences, Ben-Gurion University of the Negev, Beer Sheva, Israel
Snir Halle, George S. Wise Faculty of Life Sciences, School of Zoology, Tel-Aviv University, Tel-Aviv, Israel
Shahar Cohen, The Dead Sea and Arava Science Center, Mt. Masada, Tamar Regional Council, Masada, Israel