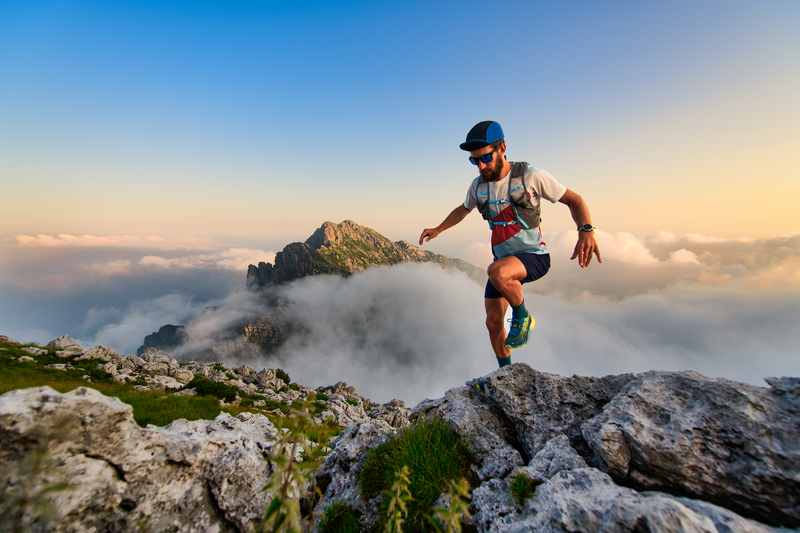
95% of researchers rate our articles as excellent or good
Learn more about the work of our research integrity team to safeguard the quality of each article we publish.
Find out more
ORIGINAL RESEARCH article
Front. Ecol. Evol. , 11 August 2022
Sec. Paleoecology
Volume 10 - 2022 | https://doi.org/10.3389/fevo.2022.884850
This article is part of the Research Topic Integrating Conservation Biology and Paleobiology to Manage Biodiversity and Ecosystems in a Changing World View all 22 articles
High-latitude habitats have become increasingly recognized as a potential climate refuge for coral communities, supporting both tropical and sub-tropical corals. Despite the increasing interest in the ecology of high-latitude corals, our current knowledge of their temporal dynamics is limited, especially within urbanized settings. Here, we examined the entire history of a high-latitude coral reef ecosystem in an urbanized setting. We surveyed Holocene fossil and modern coral communities along a water quality gradient in Moreton Bay, southeast Queensland, Australia, representing near-river (Wellington Point), intermediate (Peel Island) and near-oceanic (Myora Reef) environmental conditions. Reef accretion occurred during three discrete episodes from 7,400 to 5,800, 4,900 to 3,000, and 2,100 to 300 years BP, each separated by roughly 1,000-year hiatuses, where conditions were probably not favorable enough for reef accretion to occur. Episodic reef initiation and termination suggests strong environmental controls over reef development. Eastern Australian Holocene reef growth and cessation has been linked previously to sea level fluctuations and climatic regimes (e.g., ENSO). Within each reef building episode, there were few changes in coral assemblages over time. The fast growing and branching Acropora had a relative abundance greater than 90% in ten of the 13 sediment cores and all the submerged terrace excavations. However, substantial modification of adjacent coastal catchments from European colonization in the mid 1800’s resulted in increased sediment and nutrient discharge into the bay. This perturbation coincided with a greater abundance of stress-tolerant coral species (e.g., Dipsastraea, Goniastrea, and Goniopora) and the near extirpation of Acropora in the modern coral communities of near-river and intermediate sites due to poor water quality. In contrast, the modern coral assemblage at the near-oceanic site (Myora Reef) continues to be dominated by Acropora, likely due to the consistent oceanic input, resulting in lower sediment loading and higher water quality. In order for conditions for reef growth to improve, especially within the near-river portions of the bay, further sediment and nutrient runoff from anthropogenic land-use changes need to be mitigated. Given the historical abundance of Acropora, we recommend this genus be used as an indicator of natural resource management success in the bay.
Globally, coral reefs are experiencing a dramatic loss of hard coral abundance and habitat structure from a myriad of local and global anthropogenic and climatic factors (Bellwood et al., 2004; Bruno and Selig, 2007; Heron et al., 2016). Of increasing concern are the direct anthropogenic effects of urbanization (reviewed in Heery et al., 2018). Increases in population and coastal infrastructure are major threats to coral reef ecosystems, causing increased sediment delivery as well as the proliferation of nutrients and pollutants (Fabricius, 2005; McDonnell, 2011). Studies of urban coral reefs in Hong Kong (Zhao et al., 2012), Singapore (Guest et al., 2016), Brazil (Barros et al., 2021), and Vietnam (Nguyen and Phan, 2008) note key characteristics of urban coral reefs, such as: increased turbidity, dominance of domed coral growth forms (causing subsequent low reef complexity and the potential to negatively affect reef accretion rates), declines in branching corals and an inshore to offshore gradient in coral cover and condition (Heery et al., 2018). However, most studies of urbanized coral reefs have occurred within the tropics.
Historical records reveal corals expanded their geographic ranges toward the poles during previous periods of climate warming (Greenstein and Pandolfi, 2008; Kiessling et al., 2012). Contemporary records echo this pattern of poleward range expansions of Scleractinian corals (Woodroffe et al., 2010), tropical reef fish (Denis et al., 2013), and many other marine taxa (Poloczanska et al., 2013). Consequently, high-latitude settings have been increasingly recognized for their potential to act as a climate refugia for coral communities, potentially supporting both tropical and sub-tropical corals as climate continues to warm (Kleypas et al., 1999; Wallace et al., 2009; Lybolt et al., 2011; Denis et al., 2013; Beger et al., 2014). However, high-latitude coral communities occur in an environment with lower seawater temperature as compared to their more tropical counterparts. As such, these communities typically have a lower diversity of corals with lower growth rates (Denis et al., 2013), and also contain a reduced diversity of coral associated invertebrates (De Palmas et al., 2015; Chan et al., 2018). High-latitude reefs also contend with similar localized threats as compared to their tropical counterparts, such as boring sponge and crown of thorn starfish outbreaks (Yomogida et al., 2017; Adjeroud et al., 2018; Hirose et al., 2021). Despite the geographic distribution of coral reefs, and the increasing interest in high-latitude coral ecology (Bridge et al., 2014; Sommer et al., 2014, 2021; Kim et al., 2019), our current knowledge of fundamental ecological dynamics in high-latitude coral reef settings is limited. Thus, questions still persist as to whether high-latitude marine ecosystems are more or less susceptible to the effects of coastal modification/urbanization than their tropical counterparts, and whether they can serve as a climate refugia for corals under future warming scenarios.
Historical ecology provides critical context for predicting how coral communities might respond to current and future environmental disturbances (e.g., ocean acidification, thermal stress, sea level rise, urbanization), and for prioritizing protected areas for management, restoration, and conservation. The study of paleoecology enables long-term understanding of ecological changes on ecosystems and places the magnitude and effects of current disturbance regimes in an historical context (Pandolfi et al., 2011; Roff et al., 2013; O’Dea et al., 2020). Such interpretations aid the reconstruction of past environments and produce a baseline against which modern environmental conditions and biotic composition can be compared. By using historical data, paleoecology provides the framework necessary to anticipate and plan for future changes and discern a reef’s potential to act as a coral refugia.
The Quandamooka sea-country of Moreton Bay (MB), southeast Queensland (QLD), Australia (Figure 1), is a region that contains coral communities disproportionately affected by anthropogenic disturbances (Fellegara and Harrison, 2008; Lybolt et al., 2011). The reefs developed during the late Holocene (∼7,400 years), leaving behind a multi-millennial fossil record of reef growth and coral composition (Lybolt and Pandolfi, 2019; Pandolfi et al., 2019). A previous historical study involving U-Th dating of surficial death assemblages revealed four discrete episodes when conditions in the bay were conducive for coral growth (Lybolt et al., 2011). Owing to the Bay’s high latitudinal location (∼27 S), these reefs experience cool water temperatures during the winter (e.g., mean range of winter monthly temperatures between 18 and 20°C), and are highly turbid, with reduced solar irradiance, making them light-limited (i.e., low photosynthetically active radiation (PAR) and high light attenuation with depth; Fellegara and Harrison, 2008). Due to this environmental setting, MB is considered a marginal environment for coral reef growth (Perry and Larcombe, 2003; Fellegara et al., 2013; Sommer et al., 2014). In addition, the semi-enclosed geomorphology of MB with its reduced tidal flow in parts of the bay, acts to amplify the effects of natural and anthropogenic stressors, potentially making ecological responses readily detectable (Neil, 1998; Fellegara and Harrison, 2008; Lybolt et al., 2011). Climatic changes during the past several thousand years within the bay have principally manifested as sea level fluctuations and variations between drier and wetter conditions due to El Nino Southern Oscillation events (ENSO; Donders et al., 2007; Sloss et al., 2007; Lewis et al., 2008; Lybolt et al., 2011; Lybolt and Pandolfi, 2019). Anthropogenic disturbance was likely minimal during the late Holocene, due to a small and gradually increasing aboriginal population, until the mid–1800’s (Walters, 1989; Hiscock, 1994; Neil, 1998; Lybolt et al., 2011) when European colonization along the MB catchment resulted in substantial modification of adjacent coastal wetlands, increasing sediment and nutrient discharge (principally from the outflow of the Brisbane River). This perturbation created a strong water quality gradient within the bay and has been previously implicated as the primary driver for suppressing modern reef growth and altering coral species composition (Neil, 1998; Fellegara and Harrison, 2008; Lybolt et al., 2011; Lybolt and Pandolfi, 2019). Following European colonization, increased urbanization and population growth along the Brisbane catchment has increasingly threatened coral communities. Despite recent degradation, there are a few locations where high coral cover still persists (Wallace et al., 2009; Lybolt et al., 2011; Sommer et al., 2014). Of these, Myora Reef is unique due to its Acropora-dominated living reef flat, which overlies a submerged fossil reef terrace that preserves a history of the reef over hundreds of years. While MB is known to contain degraded modern coral communities (e.g., Fellegara and Harrison, 2008; Lybolt et al., 2011; Lybolt and Pandolfi, 2019), the magnitude of that change has not been quantified using historical data, limiting our ability to predict how coral communities may function into the future and what management actions can be taken to aid recovery.
Figure 1. Map of Moreton Bay, southeast Australia with location of sampling sites in the Bay (Satellite images courtesy of Google Maps). Representative photographs of coral assemblages from: (A) Myora Reef, (B) Peel Island, and (C) Wellington Point (Photo credit: Nicholas M. Hammerman).
While the modern and geological record hints at the potential for high-latitude reefs to act as a lifeboat under future climate change (Beger et al., 2014; Lybolt and Pandolfi, 2019), the management of coral reefs at regional and global scales is hindered by a lack of understanding of the distribution and environmental constraints of high-latitude reef growth. As such, this study represents rare insight into the history of an entire high-latitude coral reef ecosystem in an urbanized setting. To test the susceptibility of high-latitude coral communities to coastal modification/urbanization, we combined coral composition data from the submerged fossil reef terrace of Myora Reef with those from previously collected reef sediment cores along Moreton Bay’s current water quality gradient. We used this historical repository to discern how coral communities have both spatially and temporally varied in relation to past climatic and anthropogenic disturbance. In this paper we: (1) reconstruct the millennial-scale history of coral assemblages in a high-latitude and marginal environment, (2) correlate climactic and environmental drivers with fossil and modern coral reef growth, and (3) predict how MB coral communities may respond to future warming and urbanization.
We selected sites within MB where modern reef communities occur along the dominant water quality gradient, from terrigenous-dominated near-river (Wellington Point) to intermediate (southwest corner of Peel Island) to near-oceanic (Myora Reef) reefs (Figure 1). We avoided sites historically and currently disturbed by dredging. Historical and modern data on coral community composition exists from previous investigations at all three of our sites (e.g., Fellegara and Harrison, 2008; Lybolt et al., 2011; Figure 1 and Supplementary Tables 1, 2).
Separate sampling strategies were used for analyzing the fossil vs. modern reefs at each site. To assess the fossil assemblages from the three sites from MB, we analyzed 13 previously extracted reef sediment cores from the reef slope and reef flat of Wellington Point (3 cores), Peel Island (5 cores) and Myora Reef (5 cores; Supplementary Table 1). Reef sediment cores were sampled from both the reef flat (0 m water depth) and reef slope (2 and 4 m water depth) at each of the three sites. Core names are a 3-character code indicating the site name (W = Wellington, P = Peel, and M = Myora), depth (0, 2, 4 m) and replicate (a, b, c). After cores were sectioned longitudinally, one half of each core was archived at 4°C, and the other half was logged and used for compositional and geochemical analyses. These core halves were logged at 5 cm intervals using four sieve fractions (4 mm, 1 mm, 500 μm, and 63 μm) and dried overnight at 60°C. To determine the contribution of corals to each interval within each core, all coral material from the 4 mm sieve fractions was grouped according to morphological characteristics (e.g., overall morphology, corallite preservation) with reference to taxonomic features described in Veron and Pichon (1980) and Veron (2000), and when possible, identified to genus level, using the current understanding of coral systematics (WoRMS Editorial Board, 2021). To characterize the coral composition of each core, the contribution of each genus to the total weight of the identified coral constituents within each interval (refer to Supplementary Table 4) was plotted using the R statistical environment (R Core Team, 2020). Core compaction was calculated using core and penetration measurements, assuming compaction was linear and consistent along the core (Supplementary Table 1).
At Myora Reef, a channel exists parallel to the reef flat and contains a submerged fossil reef terrace, which is roughly 80 m long and occurs from 4 to 12 m depth. We sampled three locations spaced at least ten meters apart from one another and chosen randomly along the terrace. Within each location, 20 m transects were run horizontally along the submerged terrace at 4, 5, 6, 8, and 10 m depth intervals, resulting in 15 transects. At 5 m intervals along each horizontal transect, corals were excavated from the fossil outcrop using a chisel and on average from 30 to 50 cm depth into the terrace, resulting in roughly 50 cm (length) by 50 cm (height) excavations. The collected corals were placed into calico bags and equated to roughly 1 L of material per collection. Generic coral relative abundance data were calculated by weight per excavation. To characterize the coral composition of each terrace excavation, the contribution of each genus to the total weight of the identified coral constituents within each excavation was plotted using the R statistical environment (R Core Team, 2020).
At Myora Reef, we surveyed the living reef flat in August of 2019 using four 20-m belt transects (1 m wide × 20 m long) at ∼2 m water depth using SCUBA. The transects were photographed at one-meter intervals (n = 20 x 1 m2 image field per transect), and the benthic percent cover was determined by point annotations using Coral Point Count with Excel (CPCe) extensions by overlaying 50 random points on top of each 1 m2 photo (Kohler and Gill, 2006). Benthic composition was categorized by quantifying total cover of live hard coral, dead hard coral, soft coral, other substrate (e.g., sand, pavement, rubble) and algae (e.g., macroalgae, coralline algae, turf). For Wellington Point and Peel Island, modern coral community compositional data was taken from previous investigations conducted in 2002 (Fellegara and Harrison, 2008; Lybolt, 2012; Supplementary Table 2). Relative abundance data for the modern assemblages were calculated at the genus level using the same taxonomic resources as described above.
To assess temporal trends in coral cover, data were supplemented with surveys conducted in 1975 (Lovell, 1975), 1991, 1994 (Maguire, 1994), 2002 (Fellegara and Harrison, 2008), and 2004 (Environmental Protection Agency, EPA). Additionally, we incorporated permanent transect data on broad benthic substrate categories (i.e., hard coral, soft coral, sand, rubble, algae) from citizen science surveys conducted at Myora Reef and Peel Island from 2009 until present by Reef Check Australia (RCA, 2020) as scatterplots of overall coral cover through time. However, RCA does not quantify coral community data to any taxonomic level, solely grouping it as hard or soft coral. Thus, there is a paucity of data in the characterization of modern reef communities post-2002 and there are limitations for relying on RCA data for an understanding of MB coral communities.
We analyzed modern and fossil coral assemblage composition through time and between sites (Wellington, Peel and Myora) using relative abundance data. Relative abundance data were square root transformed prior to multivariate analyses to reduce the influence of dominant genera and increase the influence of rare genera in subsequent analyses (as in Clark et al., 2017). Multivariate analysis of coral community structure was used to visualize variability in coral modern and fossil assemblage composition, using non-metric multidimensional scaling (nMDS). The nMDS used the weighted Jaccard index (aka Ružička index) and was computed using the vegan R package (Oksanen et al., 2017). The weighted Jaccard index was chosen because it preserves 2D shape from multi-dimensional data better than other indices (Oksanen et al., 2017). We overlaid ordination vectors as the weighted centroids of the relative abundance of each genus. We used a two-way permutational analysis of variance (PERMANOVA) to examine differences in taxonomic composition among Myora, Peel and Wellington and between life and fossil assemblages. These models stratified modern transects and fossil assemblages within each site constraining permutations to account for nested data structure (i.e., sites in time). Values of the pseudo-F statistic were computed using 1,000 permutations. Post hoc pairwise PERMANOVAs were conducted separately between each pair of sites to identify whether there was a compositional difference between modern and fossil coral assemblages. P-values from these pairwise models were adjusted for multiple comparisons using a Bonferroni correction factor equivalent to the number of pairwise PERMANOVAs performed. Dispersion was evaluated using the “betadisper” function in the vegan R package (Oksanen et al., 2017). All analyses were conducted in R (R Core Team, 2020).
High-precision (U-Th) radiometric age dating of fossil assemblages were used to estimate rates of reef accretion and bracket periods of reef growth and hiatuses. A total of 65 corals were U-Th radiometrically age-dated from the 13 reef sediment cores across all three sites, with an additional 39 corals from the fossil reef terrace (n = 28) and modern reef flat (n = 11) of Myora Reef (Supplementary Table 3). Aragonite for U-Th dating was taken from well-preserved areas near the coral growth margin, thereby representing growth just prior to coral mortality (Clark et al., 2017). Each sample was first sectioned laterally using a lapidary saw (2–3 cm from apical tip) and a sub-sample (2–3 g) of skeleton was taken using a Dremel drill. Coral sub-samples were crushed in an agate mortar and pestle to ∼ sand size. Crushed samples were subsequently soaked in 15% H2O2 overnight, followed by 3–5 rounds of rinsing and ultrasonication in Milli-Q-water (18.2 Ω). Samples were then dried and hand-picked under a stereoscopic microscope to select for the purest aragonite (i.e., no detritus, not altered). Details for sample preparation and column chemistry can be found in the appendices of Zhao et al. (2009) and Clark et al. (2012, 2014). Samples were analyzed on a Nu I multi-collector inductively coupled plasma mass spectrometer (MC-ICP-MS) optimized for U-Th dating (Zhao et al., 2009; Clark et al., 2012) at the Radiogenic Isotope Facility at The University of Queensland. To correct for non-radiogenic 230Th contributions the two-component equation described by Clark et al. (2014) was used. This correction was applied to all dates using Isoplot 3.75 (Ludwig, 2012). Mean 230Th ages were reported with two sigma error bars (Tukey’s bi-weighted means for repeated analysis). Peak distributions of age-dates from the reef sediment cores and the Myora submerged fossil reef terrace were fitted to the raw age data using Kernel Density Estimates (KDEs). The Kernel Density Estimation works by creating a curve of the distribution. The curve is calculated by weighting the distance of all the points in each specific region along the distribution. The bandwidth for the KDEs was set to default settings using the density function in R, thus the bandwidth of the kernel changes its shape in response to the density distribution, and the shaded densities sum to 1.
Uncompacted down-core distances were used for the rate calculations. Within the fossil composition and age-depth plots, all core and terrace depths were corrected to lowest astronomical tide (LAT). Rates of accretion from the sediment cores and from the Myora fossil reef terrace were calculated by depth as the vertical distance between two adjacent dated core/terrace sections divided by the age difference between the two core/terrace sections. Classifications for vertical accretion within each time-series were derived from the data by excluding negative rates (i.e., age reversals, < 0 m ka–1) and defining all positive rates within ± 1 standard error of the mean as “medium.” “Fast” and “slow” were all rates greater and smaller than “medium,” respectively. When core/terrace sections had paired dates, the average of those two values was used in the interval rate calculation.
U-Th dating of reef flat and reef slope sediment cores from Wellington Point, Peel Island and Myora Reef revealed reefs vertically accreted during three discrete episodes from roughly 7,400 to 5,800 (Episode 1), 4,900 to 3,000 (Episode 2), and 2,100 to 300 (Episode 3) years BP (Figure 2). Each of these episodes of reef accretion were separated by roughly 1,000-year hiatuses where no reef accretion was detected (Figure 2). During periods of growth, all three reefs displayed variable rates of accretion but when averaged together equated to approximately 5.2 mm yr–1 (Figure 3 and Supplementary Table 4). The wave exposed side of the submerged fossil reef terrace of Myora Reef developed during the latter episode of MB reef development, roughly 1,000 years ago (Supplementary Figure 1). This submerged fossil reef terrace revealed several meters of linear reef growth from 4 m depth to the edge of the reef flat at ∼ 2 m depth, averaging between 5.1 and 8.6 mm yr–1 (Figure 3D). Between 4 and 8 m depth, however, pronounced age reversals indicates progradation of the reef slope from detrital accumulation of reef material (Supplementary Figure 1). U-Th dating of dead corals on the Myora modern reef flat showed a bi-modal age distribution, indicative of pronounced time averaging. The earliest dates occurred roughly 1,600 years ago, whereas the most recent dates occurred roughly 21 years ago (Supplementary Figure 1 – top row).
Figure 2. Relative probability plots of radiometric (U-Th) age-dates for Moreton Bay, derived from reef sediment cores showing episodes of reef growth: Episode 1 (7,400 to 5,800 years BP), Episode 2 (4,900 to 3,000 years BP), and Episode 3 (2,100 to 300 years BP). Error bars are ± 2SD per date. Gray shaded regions represent Kernel Density Estimates (KDEs).
Figure 3. Age-depth plots showing reef growth and accretion rates from individual reef sediment cores and the submerged fossil reef terrace of: (A) Wellington Point, (B) Peel Island, (C) Myora Reef cores, and (D) Myora fossil reef terrace. Core depths were uncompacted and corrected to lowest astronomical tide (LAT). Note differences in x-axis scale between cores and the Myora fossil reef terrace. Different colors and font styles within site are used to visually differentiate cores and terrace sections. All accretion rates are presented as mm yr– 1.
Within each reef the taxonomic composition of coral assemblages was similar over Holocene time. Ten of the 13 reef sediment cores in this study contained greater than 90% Acropora by relative abundance. The coral composition of the three reef slope cores and submerged fossil reef terrace at Myora Reef was 100% arborescent Acropora (Figure 4 and Supplementary Tables 5, 6). Two of the three cores from Wellington Point contained a mixed coral assemblage, one each from the reef flat (W0a) and reef slope (W2b), with Acropora, Dipsastraea (formerly Favia) and Goniopora in similar proportions (Figure 4 and Supplementary Tables 5, 6). During periods of reef growth, which equated to roughly 50% of the time during the past 7,400 years, conditions in MB were conducive for Acropora corals.
Figure 4. Percent contribution of coral genera from the fossil assemblages per reef sediment core and the submerged fossil reef terrace of Myora Reef. U-series radiometric ages are reported per core and terrace as Calendar year (AD) ± 2SD error. Note differences in y-axis scales among cores; these were adjusted to accommodate the longest core from each site. Fossil coral assemblages are plotted per interval accretion rate as per Supplementary Table 4.
Wellington Point had a modern community composition comprised of corals mostly from the Merulinidae family and a lack of Acropora (Figure 5A). Peel Island had a similar modern community composition, but with scattered Acropora corals persisting (Figure 5B), particularly on the northeastern edge of the island. Myora Reef differs in that the modern assemblage is dominated by Acropora corals (Figure 5C). The relative percentage of live coral in the overall benthic composition followed an inshore to offshore gradient, with 1.8 ± 0.8% (mean ± SD) at Wellington Point, 13.5 ± 8.3% at Peel Island and 34.9 ± 11.7% at Myora Reef (Supplementary Figure 2).
Figure 5. Relative abundance (percent ± SE) for most common coral genera from: (A) Wellington Point, (B) Peel Island, and (C) Myora Reef in modern versus fossil assemblages from Moreton Bay. Each symbol represents pooled transects or cores from each site. Diagonal line represents equal contribution for modern versus fossil assemblages.
To assess the temporal and spatial extent of coral community variability, we compared the fossil coral assemblages from the three sites with their respective contemporary counterparts. Wellington Point, the site closest to shore, had a modern community composition that was significantly different from its fossil assemblage, which was characterized by mixed Acroporidae and Merulinidae corals (Figure 6; PERMANOVA, F = 3.75, P = 0.036; Table 1). The modern and fossil coral assemblages were significantly different at Peel Island (Figure 6; PERMANOVA, F = 8.68, P = 0.01; Table 1). Myora Reef differs in that there is no significant difference between the fossil and modern assemblages (Figure 6; PERMANOVA, F = 2.66, P = 0.1; Table 1), with both assemblages dominated by Acropora corals. Tests for dispersion revealed heterogeneity of variance across sites (P < 0.05); thus, significant differences among groups from the PERMANOVA results should be interpreted with caution. However, clear compositional differences between fossil and modern assemblages within and between sites is supported by relative abundance scatterplots of coral composition (Figure 5) and the clustering of modern and fossil coral assemblages within and among sites in the nMDS ordination (Figure 6). Within the nMDS the fossil assemblages are strikingly consistent in space (i.e., they are constrained), whereas the modern assemblages are dispersed. This most likely does not represent an increase in diversity through time, but more likely an artifact of different sampling methods between the fossil and modern assemblages. Dispersion in fossil and modern assemblages through time is similar at Wellington Point but varies substantially within Peel Island and Myora Reef. The fossil assemblages are remarkably consistent through time (i.e., Acropora dominated). The modern assemblages show a clear water quality gradient, where Wellington Point and Peel Island modern assemblages are dominated by more stress tolerant genera (i.e., Dipsastraea, Acanthastrea, Goniastrea, and Cyphastrea), whereas Myora Reef modern assemblages are dominated by Acropora. Lastly, the modern assemblages are very different than the fossil assemblages at all sites apart from Myora Reef, where there is overlap between both assemblages (Figure 6).
Figure 6. Non-metric multi-dimensional scaling (nMDS) ordinations of community composition among modern and fossil coral assemblages from all three sites using relative abundance data, to infer historical variation for reefs in Moreton Bay. Each symbol represents either the modern or fossil assemblage per site. Overlaid vectors are the weighted contribution of each genera’s relative abundance and are only shown for the genera with a significant contribution (p < 0.05) to the dissimilarities between fossil and modern assemblages among sites. Black outline represents total hull space. Gray shading represents total hull volume for both the fossil and modern coral assemblages per site.
Table 1. Permutational analysis of variance (PERMANOVA) for the coral composition among sites, between modern and fossil assemblages, and between assemblages among sites from Moreton Bay, including post hoc pairwise comparisons between coral modern and fossil assemblages within sites.
Historical studies are vital to conceptualize rates and magnitude of ecosystem change. Resource management is often predicated on decision making derived from already highly altered ecosystems over short time periods, while natural systems change and evolve across multiple timescales. Long-term studies reconstruct a baseline of past environments that can be compared with modern environmental conditions and biotic composition (Knowlton and Jackson, 2008). By doing so, the relative abundance of key species can be identified as indicators of impending change and/or help understand resource management success. Historical datasets have shown remarkable fidelity among coral life, death, and fossil assemblages from both the Caribbean (Greenstein et al., 1996; Greenstein and Pandolfi, 1997; Pandolfi and Greenstein, 1997) and Indo-Pacific (Pandolfi and Minchin, 1995), this is especially true for dominant taxa such as, Acropora. Thus, conservation-minded palaeoecological studies are especially vital for coral reefs, as these systems are experiencing a dramatic loss of hard coral abundance and habitat structure from a myriad of local and global anthropogenic and climatic factors (Bellwood et al., 2004; Bruno and Selig, 2007; Heron et al., 2016), and of increasing concern is the urbanization of coastal wetlands fringing coral reefs. Yet, most studies of coral-related ecosystem change occur within the tropics, and generally lack assessments of long term temporal dynamics. This study revealed the magnitude of coral community change within an urbanized high-latitude setting, allowing for predictions of how MB coral communities may respond to future warming and urbanization.
The coral reefs of MB grew episodically during the late Holocene in three discrete periods separated by ∼1,000-year intervals, during which conditions in the bay were not conducive for reef accretion (Leonard et al., 2013; Lybolt and Pandolfi, 2019; Pandolfi et al., 2019). Patterns of reef growth derived from the fossil assemblages largely corroborate a previous study using surficial death assemblages (Lybolt et al., 2011). However, Lybolt et al. (2011) found a fourth episode of coral and reef growth occurring from 400 years BP to present. This contrasting pattern is likely the result of focused age-dating of surficial death assemblages that represented the youngest available coral communities inhabiting the Bay. Regardless, Lybolt et al.’s (2011) first three episodes of coral growth largely overlap with our three episodes of reef growth. Similar patterns of episodic high-latitude Holocene reef growth has occurred within the northern Caribbean (26oN; Toth et al., 2021), northern South China Sea (22oN; Yan et al., 2019) and southern Great Barrier Reef (GBR; 26–27oS; Smithers et al., 2006; Perry and Smithers, 2011; Leonard et al., 2013, 2016). Episodic Holocene reef initiation and termination (i.e., hiatuses) suggests strong environmental controls over reef development (reviewed in Donders et al., 2007; Sloss et al., 2007; Lewis et al., 2008, 2012; Perry and Smithers, 2011; Leonard et al., 2013, 2016).
The earliest episode of MB reef growth occurred during the mid-Holocene from ∼8–6 ka. During this time period, Sr/Ca analysis of Holocene corals from the western Pacific revealed mean annual high temperature anomalies upwards of ∼0.5–3°C (Sadler et al., 2016). Global trends in sea surface temperature anomalies echo this pattern (Figure 7; Rosenthal et al., 2022). Sedimentation to nearshore reefs, as evidenced from a study on the Great Barrier Reef lagoon, was an estimated 40% less than today because of enhanced vegetation, which reduced erosion (Neil, 1998, Neil, 2002). Specific to MB, this period of reef growth occurred when sea level was an estimated 1.1 m above modern lowest astronomical tide (LAT; Lewis et al., 2008; Leonard et al., 2013). Paleo-ENSO records of the central and western Pacific also revealed strong reductions in ENSO variance (Cobb et al., 2013; Grothe et al., 2019), with some datasets suggesting this period of reduced ENSO instability lasted from ∼5–7 ka (White et al., 2018). This period of climatic stability, termed the Holocene climatic optimum (HCO), is a time when prolific reef growth occurred within MB and other high-latitude locations (Lybolt et al., 2011; Yan et al., 2019).
Figure 7. Composite representation of selected environmental variables from eastern Australia and the greater Pacific Ocean during the past ∼ 7,000 years. Sea level curves of Sloss et al. (2007) and Lewis et al. (2008) were re-scaled to the same elevation datum. Holocene reconstruction of surface water temperature anomalies from Rosenthal et al. (2022) are a global average. ENSO frequency record of Moy et al. (2002) was recorded from sediments from southern Ecuador.
Abruptly at 5 ka conditions changed, and the western Pacific became cooler, with cycles of extreme flood and drought (Neil, 1998; Donders et al., 2007; Rosenthal et al., 2022), which may have played a role in reduced accretion. This climatic change caused erosion and sedimentation rates to nearly double that of the HCO (Neil et al., 2002; Perry and Smithers, 2011). Oscillations in relative sea level (RSL) during this time period may have also led to reduced reef accretion (turn-off), followed by significant hiatuses in reef growth that lasted for nearly two millennia (Sloss et al., 2007; Leonard et al., 2013, 2016). Then a resurgence of high-latitude reefs occurred ∼2 ka, despite a significant increase in El Niño events and ENSO instability (Figure 7; Moy et al., 2002; Donders et al., 2007; Grothe et al., 2019). Our U-series dating from MB and other studies from parts of the southern GBR (Leonard et al., 2013, 2020), suggest reef growth halted roughly 300–400 years ago. This modern hiatus is in contrast to the Lybolt et al. (2011) study, which identified an additional, more recent episode of MB reef growth from surficial death assemblages from 400 years BP to present. Their four episodes of coral growth occurred in progressively deeper water over time, with the upper depth range of earlier episodes in the intertidal, while the last two episodes did not leave deposits in the upper subtidal zone (Figure 4 in Lybolt et al., 2011). Overall, a key consideration from their surficial death assemblages and our sediment cores is that while there is a history of intertidal accretion in the bay, this zone is no longer suitable for coral substrate. Thus, Holocene reef development is linked to natural geomorphological responses to vertical accommodation space (i.e., reefs growing to sea-level, as in Leonard et al., 2013, 2020) and climatic regimes (i.e., ENSO, as in Perry and Smithers, 2011).
During episodes of reef growth, rates of MB reef accretion were similar to those that characterize nearshore fringing reefs on the northern and southern GBR (Perry and Smithers, 2011; Roff et al., 2015; Leonard et al., 2018, 2020). During the mid-Holocene climatic and environmental optimum along eastern Australia, minimal latitudinal disparity in net accretion rates occurred between tropical and high-latitude reef habitats (Perry and Smithers, 2011; Leonard et al., 2013). Each episode of reef growth occurred during different climatic regimes and sea levels (Leonard et al., 2013; reviewed in Lybolt and Pandolfi, 2019), so differences in coral composition might be expected among Holocene episodes of reef growth. Instead, within each site there were few changes in coral assemblages over Holocene time. During periods of reef growth, which equated to roughly 50% of the time during the past 7,400 years (Lybolt et al., 2011), conditions in MB were conducive for Acropora corals.
U-Th dating of modern coral death assemblages revealed a transition in community composition of MB reefs (Lybolt et al., 2011), and temporally bracketed this change between 165 and 52 years ago (1852 to 1956 AD), coincident with European settlement that increased land-use changes and amplified sediment and nutrient runoff along the entire Queensland coastline (reviewed in Lybolt et al., 2011; Lybolt and Pandolfi, 2019; Lewis et al., 2021). Modification and urbanization of the local bay catchments potentially enhanced erosion by 2–10 times as compared to pre-colonization levels (Neil et al., 2002). This erosion was further amplified due to direct harvest of MB corals for use as building materials, a practice continued into the late 1990’s (Neil, 1998). Such coastal modification inhibited reef building Acropora corals from persisting on many reefs in MB and facilitated a shift to more stress- tolerant corals, such as Dipsastraea, Goniastrea and, Goniopora (Johnson and Neil, 1998; Fellegara and Harrison, 2008; Pandolfi et al., 2019).
While Holocene to modern shifts in coral community composition characterized reef communities on the near-river western edge (Wellington Point) and central portion (Peel Island) of MB, the fossil assemblage at Wellington Point had a high abundance of non-acroporids, so the modern shift away from Acropora here is not entirely unprecedented. In contrast, we found that over thousands of years, the Myora coral assemblage of the near-oceanic eastern edge of the bay was dominated by Acropora corals, and this trend continues for modern coral assemblages. Myora has been previously singled out as the bay’s last vestige of an Acropora dominated modern community (Johnson and Neil, 1998), containing several unique Acropora species not found elsewhere in the bay (A. hyacinthus, A. latistella, A. loripes, A. valida, and A. verweyi; Fellegara and Harrison, 2008; Wallace et al., 2009). A similar gradient of coral community composition in relation to distance from shore was revealed for the turbid high-latitude reefs of Hervey Bay, roughly 200 km north of MB (Sommer et al., 2021), where water quality varies spatially with distance from shore and river mouths, and urban development (Butler et al., 2015). Coastal development is a critical issue for coral reefs; for example, coastal development has led to an 80% decline in coastal fringing reefs in the South China Sea over the past 30 years (Zhao et al., 2012). However, high turbidity can confer resilience to thermal stress, even in urbanized environments (Guest et al., 2016). Thus, high turbidity and urbanization may not always be detrimental for reef growth. Although poor water quality due to urbanization has been shown to generally degrade inshore high-latitude reefs similarly to the tropics, resulting in a dominance of domed coral growth forms, declines in branching corals, decline in bathymetric range of corals, and an inshore to offshore gradient in coral cover and condition (as reviewed in Fabricius, 2005; Heery et al., 2018; Zweifler et al., 2021).
Of the three sites investigated, Myora Reef is furthest from the Brisbane River and least impacted by the urbanization of coastal catchments, presumably benefiting from far less freshwater discharge and resultant turbidity. These abiotic differences could explain why Myora still maintains high Acropora cover leading to the marked difference in biotic composition (i.e., coral cover and community composition). In addition, Myora lies directly within the path of an oceanic inlet (i.e., the Rainbow Channel). This channel enters the bay through the south passage (Figure 1; an entrance between Moreton Island and North Stradbroke Island to the Pacific Ocean) and brings with it a continual influx of oceanic water, and thus flushes Myora on a semi-diurnal tidal pattern. This creates both clearer water and perhaps produces a greater tidal flow, which potentially reduces the accumulation of harmful reactive oxygen species and thermal stress (Page et al., 2019). Notably, Myora Reef is not the only location in the bay that contains relatively high abundance of Acropora (e.g., Goat Island, and the northeastern edge of Peel Island). However, the latter two reefs do not have the same diversity of Acroporids as Myora Reef. Regardless, these other two reefs are within 1–2 kms of Myora and also within the outflow of the Rainbow channel. Their geographical position on the eastern edge of the bay further reinforces the notion of a water quality gradient driving modern coral distributions.
Approaches that quantify historical variability are particularly valuable for coral reef ecosystems, as reefs change slowly on human timescales, and because their complexity often requires decades or centuries of observation to elucidate the relevant driver–response relationships (Hughes and Connell, 1999; Pandolfi et al., 2003). For the near river portion of the Bay, Wellington Point, the implications of mixed coral assemblages during the Holocene suggests that turbidity may have begun influencing this inshore site long before urbanization, although, urbanization may have made it worse and led to the extirpation of Acropora in the modern era. In the context of coral-reef management, it seems likely Acropora dominance might not be a realistic expectation of successful management at this site. However, for MB as a whole, most Holocene coral communities were dominated by Acropora during periods of episodic reef growth over the past 7,400 years. As with other high-latitude and urban regions (e.g., Heery et al., 2018), increased sediment and nutrient runoff from anthropogenic land-use changes drove the modern decline of Acropora- dominated reefs of MB (Lybolt et al., 2011; Butler et al., 2015; Sommer et al., 2021). The scale of Acropora-dominated benthic community structure so typical of the history of MB benthic habitats is now greatly reduced, giving resource managers essential context from which to measure success in their efforts to improve water quality. As water quality improves, benthic community structure should accommodate a greater component of branching Acropora corals over an increasing number of sites. That is, unless there are other problems that also suppress Acropora recovery such as thermal stress events and/or recruitment failure.
Under future projections for increasing atmospheric CO2, increases in SST will result in most low latitude tropical coral reefs (15°N – 15°S) exceeding their thermal tolerance limits by the mid-late 21st century (Heron et al., 2016; Camp et al., 2018). Thus, cooler high-latitude reefs may represent a critical refuge for coral reefs under future climate change (Greenstein and Pandolfi, 2008; Beger et al., 2014; Bridge et al., 2014; Kim et al., 2019). Although high latitude localities will likely vary geographically in their response to future climate change (e.g., Florida Toth et al., 2021), there is evidence that high-latitude reefs may still function into the future, given that MB and other high-latitude regions experienced reef growth during the mid-Holocene (∼6–7 ka; Smithers et al., 2006; Perry and Smithers, 2011; Lybolt et al., 2011) when sea surface temperatures were as high as 0.5–1°C warmer than today (Sadler et al., 2016; Rosenthal et al., 2022), and during periods of fluctuating sea level (Sloss et al., 2007; Lewis et al., 2008). Such fluctuations in sea level warrant consideration from a management perspective, since both our study and that of Lybolt et al. (2011) found the subtidal zone not suitable for coral growth. This limitation may change in the future with rising sea levels enabling expansion of the area in which corals in the bay can grow. On a site specific level, the millennial stability in coral community composition (Figure 4), amid European colonization and recent recurrent thermal stress, points to the resilience of Myora Reef and its potential for continued Acropora growth, which might be important for maintaining local sources of larvae and providing habitat complexity for use by other ecologically important reef species in MB. However, we have shown that, as a whole, Moreton Bay’s potential to act as a coral refuge for neighboring coral populations, such as the GBR, may be limited. The Bay was only conducive for Acropora growth roughly 50% of the time in the last 7,400 years and roughly 150 years ago it only took ∼20k European settlers to affect water quality to a point where coral communities were negatively affected (Neil, 1998; Lybolt et al., 2011), albeit this impact was unprecedented in scale due to unregulated land clearing for grazing. Thus, on a larger than MB scale, management approaches must deal with uncertainty in regard to the degree to which this subtropical setting might harbor suitable habitat for coral species migrating from more northern regions as climate change continues to unfold.
Global stressors such as climate change are difficult to regulate or control without global effort but mitigating local-scale stressors of water quality can have substantial effects upon recovery of coral reefs. The Brisbane catchment now hosts more than 2 million people; however, recent research shows that water quality in the western MB has improved significantly since the 1970s, due to increased coastal catchment management (Narayan, 2011). If conditions for reef growth are to continue to improve, especially within the western and central portions of the bay, further sediment and nutrient runoff from anthropogenic land-use changes need to be mitigated. Natural resource management plans can use increases in the abundance of Acropora as a historically relevant indicator species of reduced anthropogenic impact. Measuring the recovery success of Acropora is easy within MB since the genus is so uncommon and simple presence-absence surveys are likely to indicate meaningful changes.
The original contributions presented in this study are included in the article/Supplementary material, further inquiries can be directed to the corresponding author.
NH, TL, GE, and JP designed the study. NH led the study, data analysis, interpretation, and manuscript writing with input from all authors. NH, GR, TL, and GE conducted the fieldwork. NH and TL performed laboratory analyses. TL, GR, and GE helped with data manipulation, visualization, and analyses. JP and NH provided the funding. All authors contributed to the article and approved the submitted version.
This research was supported by funding from two grants awarded to NH, The Paleoecology Society G. Arthur Cooper Student Research Award, and the Moreton Bay Research Station (MBRS) Sibelco Scholarship and the ARC Centre of Excellence for Coral Reef Studies grant (CE140100020) awarded to JP and others. This manuscript was also partially funded by a University of Queensland Research Support Package, Allocation 3 Strategic Research Investment grant for Sustainable Urban Seascapes.
We thank the crew and technicians at MBRS for our field excursion in Moreton Bay; K. Townsend, C. Cotterell, L. Trippett, and L. Borey. We thank N. Gutierrez for help in the field. We thank A. Nguyen, N. Leonard, Y. Feng, J. Zhao, and F. Liu from the Radiogenic Isotope Facility (UQ); G. Xia from the Earth Science Sample Preparation Laboratory (UQ); and K. Zwiep, K. Gomez-Cabrera, C. Chong-Montenegro, H. Markham, and C. Sims from the Marine Palaeoecology Lab (UQ). We thank B. Sommer and S. Kim for their helpful discussions and revisions of this work. We also thank the Quandamooka Yoolooburrabee Aboriginal Corporation (QYAC) for allowing us to work within their sea-country. NH was also supported with a University of Queensland Research Training Scholarship.
TL was employed by Tetra Tech.
The remaining authors declare that the research was conducted in the absence of any commercial or financial relationships that could be construed as a potential conflict of interest.
All claims expressed in this article are solely those of the authors and do not necessarily represent those of their affiliated organizations, or those of the publisher, the editors and the reviewers. Any product that may be evaluated in this article, or claim that may be made by its manufacturer, is not guaranteed or endorsed by the publisher.
The Supplementary Material for this article can be found online at: https://www.frontiersin.org/articles/10.3389/fevo.2022.884850/full#supplementary-material
Adjeroud, M., Kayal, M., Peignon, C., Juncker, M., Mills, S. C., Beldade, R., et al. (2018). Ephemeral and localized outbreaks of the coral predator Acanthaster cf. solaris in the southwestern lagoon of New Caledonia. Zool. Stud. 57:e4. doi: 10.6620/ZS.2018.57-04
Barros, Y., Lucas, C. C., and Soares, M. O. (2021). An urban intertidal reef in dominated by fleshy macroalgae, sediment, and bleaching of a resilient coral (Siderastrea stellata). Mar. Pollut. Bull. 173:112967. doi: 10.1016/j.marpolbul.2021.112967
Beger, M., Sommer, B., Harrison, P. L., Smith, D. A., and Pandolfi, J. M. (2014). Conserving potential coral reef refuges at high latitudes. Divers. Distrib. 20, 245–257. doi: 10.1007/s11356-022-21881-9
Bellwood, D. R., Hughes, T. P., Folke, C., and Nyström, M. (2004). Confronting the coral reef crisis. Nature 429, 827–833. doi: 10.1038/nature02691
Bridge, T. C. L., Ferrari, R., Bryson, M., Hovey, R., Figueira, W. F., Williams, S. B., et al. (2014). Variable responses of benthic communities to anomalously warm sea temperatures on a high-latitude coral reef. PLoS One 9:e113079. doi: 10.1371/journal.pone.0113079
Bruno, J. F., and Selig, E. R. (2007). Regional decline of coral cover in the Indo-Pacific: Timing, extent, and subregional comparisons. PLoS One 2:e711. doi: 10.1371/journal.pone.0000711
Butler, I. R., Sommer, B., Zann, M., Zhao, J. X., and Pandolfi, J. M. (2015). The cumulative impacts of repeated heavy rainfall, flooding and altered water quality on the high-latitude coral reefs of Hervey Bay, Queensland, Australia. Mar. Pollut. Bull. 96, 356–367. doi: 10.1016/j.marpolbul.2015.04.047
Camp, E. F., Schoepf, V., Mumby, P. J., Hardtke, L. A., Rodolfo-Metalpa, R., Smith, D. J., et al. (2018). The future of coral reefs subject to rapid climate change: Lessons from natural extreme environments. Front. Mar. Sci. 5:4. doi: 10.3389/fmars.2018.00004
Chan, B. K. K., Xu, G., Kim, H. K., Park, J.-H., and Kim, W. (2018). Living with marginal coral communities: Diversity and host-specificity in coral-associated barnacles in the northern coral distribution limit of the East China Sea. PLoS One 13:e0196309. doi: 10.1371/journal.pone.0196309
Clark, T. R., Roff, G., Zhao, J., Feng, Y., Done, T. J., and Pandolfi, J. M. (2014). Testing the precision andaccuracy of the U-Th chronometer for dating coral mortality events in the last 100 years. Q. Geochronol. 23, 35–45. doi: 10.1016/j.quageo.2014.05.002
Clark, T. R., Roff, G., Zhao, J., Feng, Y., Done, T. J., McCook, L. J., et al. (2017). U-Th dating revealsregional-scale decline of branching Acropora corals on the Great Barrier Reef over the past century. Proc. Natl. Acad. Sci. U.S.A. 114, 10350–10355. doi: 10.1073/pnas.1705351114
Clark, T. R., Zhao, J., Feng, Y., Done, T. J., Jupiter, S., Lough, J., et al. (2012). Spatial variability of initial 230Th/232Th in modern Porites from the inshore region of the Great Barrier Reef. Geochim. Cosmochimica Acta 78, 99–118. doi: 10.1016/j.gca.2011.11.032
Cobb, K. M., Westphal, N., Sayani, H. R., Watson, J. T., Lorenzo, E., Cheng, H., et al. (2013). Highly variable El Niño-Southern Oscillation throughout the Holocene. Science 339, 67–70. doi: 10.1093/gbe/evw292
De Palmas, S., Denis, V., Ribas-Deulofeu, L., Loubeyres, M., Woo, S., Hwang, S. J., et al. (2015). Sympobiodinium spp. associated with high latitude Scleractinian corals from Jeju Island, South Korea. Coral Reefs 34, 919–925. doi: 10.1007/s00338-015-1286-y
Denis, V., Mezaki, T., Tanaka, K., Kuo, C. Y., De Palmas, S., Keshavmurthy, S., et al. (2013). Coverage, Diversity, and Functionality of a high-latitude coral community (Tatsukushi, Shikoku Island, Japan). PLoS One 8:e54330. doi: 10.1371/journal.pone.0054330
Donders, T. H., Haberle, S. G., Hope, G., Wagner, F., and Visscher, H. (2007). Pollen evidence for the transition of the eastern Australia climate system from the post-glacial to the present-day ENSO mode. Q. Sci. Rev. 26, 1621–1637. doi: 10.1016/j.quascirev.2006.11.018
Fabricius, K. E. (2005). Effects of terrestrial runoff on the ecology of corals and coral reefs: Review and synthesis. Mar. Pollut. Bull. 50, 125–146. doi: 10.1016/j.marpolbul.2004.11.028
Fellegara, I., and Harrison, P. L. (2008). “Status of the subtropical Scleractinian coral communities in the turbid environment of Moreton Bay, southeast Queensland,” in Proceedings of the 30th international marine biological workshop, the marine fauna and flora of moreton bay, Vol. 54, eds P. J. F. Davie and J. A. Phillips (Brisbane, QLD: Memoirs of the Queensland Museum – Nature), 277–291.
Fellegara, I., Baird, A. H., and Ward, S. (2013). Coral reproduction in a high-latitude, marginal reef environment (Moreton Bay, southeast Queensland, Australia). Invert. Reprod. Deve. 57, 219–223. doi: 10.1080/07924259.2012.752766
Greenstein, B. J., and Pandolfi, J. M. (1997). Preservation of community structure in modern reef coral life and death assemblages of the Florida Keys: Implications for the Quaternary fossil record of coral reefs. Bull. Mar. Sci. 61, 431–452.
Greenstein, B. J., and Pandolfi, J. M. (2008). Escaping the heat: Range shifts of reef coral taxa in coastal Western Australia. Glob. Change Biol. 14, 513–528. doi: 10.1111/j.1365-2486.2007.01506.x
Greenstein, B. J., Harris, L. A., and Curran, H. A. (1996). Comparison of recent coral life and death assemblages to Pleistocene Reef communities: Implications for rapid faunal replacement on recent reefs. Carbonates Evaporates 13, 23–31. doi: 10.1007/BF03175431
Grothe, P. R., Cobb, K. M., Liguori, G., Lorenzo, Capotondi, A., Lu, Y., et al. (2019). Enhanced El-Niño- Southern Oscillation variability in recent decades. Geophys. Res. Lett. 46:e2019GL083906. doi: 10.13287/j.1001-9332.202103.035
Guest, J. R., Tun, K., Low, J., Verges, A., Marzinelli, E. M., Campbell, A. H., et al. (2016). 27 years of benthic and coral community dynamics on turbid, highly urbanized reefs off Singapore. Sci. Rep. 6:36260. doi: 10.1038/srep36260
Heery, E. C., Hoeksema, B. W., Browne, N. K., Reimer, J. D., Ang, P. O., et al. (2018). Urban coral reefs: Degradation and resilience of hard coral assemblages in coastal cities of East and Southeast Asia. Mar. Pollut. Bull. 135, 654–681. doi: 10.1016/j.marpolbul.2018.07.041
Heron, S. F., Maynard, J. A., Hooidonk, R. V., and Eakin, C. M. (2016). Warming trends and bleaching stress of the world’s coral reefs 1985-2012. Sci. Rep. 6:38402. doi: 10.1038/srep38402
Hirose, Y., Aini, S. N., and Yamashiro, H. (2021). Contact reactions between individuals of the coral-killing sponge, Terpios hoshinota. Zool. Stud. 60:e41. doi: 10.6620/ZS.2021.60-41
Hiscock, P. (1994). Technological responses to risk in Holocene Australia. J. World Prehistory 8, 267–292. doi: 10.1016/j.jhevol.2017.09.006
Hughes, T. P., and Connell, J. H. (1999). Multiple stressors on coral reefs: A long-term perspective. Limnol. Oceanogr. 44, 932–940. doi: 10.1038/s41467-019-12431-y
Johnson, P. R., and Neil, D. T. (1998). “The corals of Moreton Bay: Living with extremes,” in Moreton Bay and catchment, eds I. R. Tibbetts, N. J. Hall, and W. C. Dennison (Brisbane, QLD).
Kiessling, W., Simpson, C., Beck, B., Mewis, H., and Pandolfi, J. M. (2012). Equatorial decline of reef corals during the last Pleistocene interglacial. Proc. Natl. Acad. Sci. U.S.A. 109, 21378–21383. doi: 10.1073/pnas.1214037110
Kim, S. W., Sampayo, E. M., Sommer, B., Sims, C. A., Gomez-Cabrera, M. D. C., Dalton, S. J., et al. (2019). Refugia under threat: Mass bleaching of coral assemblages in high-latitude eastern Australia. Glob. Change Biol. 25, 3918–3931. doi: 10.1111/gcb.14772
Kleypas, J. A., McManus, J. W., and Menez, L. A. B. (1999). Environmental limits to coral reef development: Where do we draw the line? Am. Zool. 39, 146–159. doi: 10.1093/icb/39.1.146
Knowlton, N., and Jackson, J. B. C. (2008). Shifting baselines, local impacts, and global change on coral reefs. PLoS Biol. 6, e54. doi: 10.1371/journal.pbio.0060054
Kohler, K. E., and Gill, S. M. (2006). Coral point count with excel extensions (CPCe): A visual basic program for the determination of coral and substrate coverage using random point count methodology. Comput. Geosci. 32, 1259–1269. doi: 10.1016/j.cageo.2005.11.009
Leonard, N. D., Lepore, M. L., Zhao, J. X., Rodriguez-Ramirez, A., Butler, I., Clark, T. R., et al. (2020). A U-Th dating approach to understanding past coral reef dynamics and geomorphological constraints on future reef growth potential; Mazie Bay, Southern Great Barrier Reef. Paleoceanogr. Paleoclimatol. 35:e2019A003768.
Leonard, N. D., Welsh, K. J., Clarke, T. R., Feng, Y. X., Pandolfi, J. M., and Zhao, J-x (2018). New evidence for “far-field” Holocene Sea level oscillations and links to global climate records. Earth Planet. Sci. Lett. 487, 67–73. doi: 10.1016/j.epsl.2018.02.008
Leonard, N. D., Welsh, K. J., Zhao, Jx, Nothdurft, L. D., Webb, G. E., et al. (2013). Mid-Holocene sea-level and coral reef demise: U-Th dating of subfossil corals in Moreton Bay, Australia. Holocene 12, 1841–1852. doi: 10.1177/0959683613508156
Leonard, N. D., Zhao, J-x, Welsh, K. J., Feng, Y. X., Smithers, S. G., Pandolfi, J. M., et al. (2016). Holocene Sea level instability in the southern Great Barrier Reef, Australia: High-precision U-Th dating of fossil microatolls. Coral Reefs 35, 625–639. doi: 10.1007/s00338-015-1384-x
Lewis, S. E., Bartley, R., Wilkinson, S., Bainbridge, Z., Henderson, A. E., Irvine, S. A., et al. (2021). Land use change in the river basins of the Great Barrier Reef, 1860 to 2018: Constructing foundational records to interpret environmental history across the catchment to reef continuum. Mar. Pollut. Bull. 166:112193. doi: 10.1016/j.marpolbul.2021.112193
Lewis, S. E., Wu, R. A. J., Webster, J. M., and Shields, G. A. (2008). Mid-late Holocene sea-level variability eastern Australia. Terra Nova 20, 74–81. doi: 10.1111/j.1365-3121.2007.00789.x
Lewis, S. E., Wüst, R. A. J., Webster, J. M., Shields, G. A., Renema, W., Lough, J. M., et al. (2012). Development of an inshore fringing coral reef using textual, compositional, and stratigraphic data from Magnetic Island, Great Barrier Reef, Australia. Mar. Geol. 29, 18–32. doi: 10.1016/j.margeo.2012.01.003
Lovell, E. R. (1975). Evidence for a higher sea level in Moreton Bay, Queensland. Mar. Geol. 18, 87–94. doi: 10.1016/0025-3227(75)90044-4
Lybolt, M. (2012). Dynamics of marginal coral reef ecosystems: Historical responses to climatic and anthropogenic change. Ph.D. Thesis, Centre for Marine Studies. Brisbane, QLD: The University of Queensland.
Lybolt, M., and Pandolfi, J. M. (2019). “Holocene history of Moreton Bay reef habitats,” in Moreton Bay Quandamooka & Catchment: Past, present, and future, eds I. R. Tibbetts, P. C. Rothlisberg, D. T. Neil, T. A. Homburg, D. T. Brewer, and A. H. Arthington (Brisbane, QLD: The Moreton Bay Foundation).
Lybolt, M., Neil, D., Zhao, J-x, Feng, Y., Yu, K. F., and Pandolfi, J. M. (2011). Instability in a marginal coral reef: The shift from natural variability to a human-dominated seascape. Front. Ecol. Environ. 3, 154–161. doi: 10.1890/090176
Maguire, D. (1994). A survey of the reef flat coral communities of Peel Island and Goat Island, Moreton Bay, Queensland. Integrated project. Lismore, NSW: Southern Cross University.
McDonnell, M. J. (2011). “The history of urban ecology: An ecologist’s perspective,” in Urban ecology: Patterns, processes and applications, ed. J. Niemela (Oxford: Oxford University Press), 5–13. doi: 10.1111/cobi.13244
Moy, C. M., Seltzer, G. O., Rodbell, D. T., and Anderson, D. M. (2002). Variability of El Niño/Southern Oscillation activity at millennial timescales during the Holocene epoch. Nature 420, 162–165. doi: 10.1038/nature01194
Narayan, Y. R. (2011). Benthic foraminifera as Holocene to recent indicators in marginal marine environments: Modern distribution and the palaeoecological response to environmental changes in Moreton Bay, southeast Queensland, Australia. Dissertation. Brisbane, QLD: The University of Queensland.
Neil, D. T. (1998). “Moreton Bay and its catchment: Seascape and landscape, development, and degradation,” in Moreton Bay and Catchment. School of Marine Science, eds I. R. Tibbetts, N. J. Hall, and W. C. Dennison (Brisbane, QLD: The University of Queensland), 3–54.
Neil, D. T., Orpin, A. R., Ridd, P. V., and Yu, B. (2002). Sediment yield and impacts from river catchments to the Great Barrier Reef lagoon. Mar. Freshw. Res. 53, 733–752. doi: 10.1071/MF00151
Nguyen, L. V., and Phan, H. K. (2008). Distribution and factors influencing on structure of reef fish communities in Nha Trang Bay marine protected area, South-Central Vietnam. Environ. Biol. Fish 82, 309–324. doi: 10.1007/s10641-007-9293-7
O’Dea, A., Lepore, M., Altieri, A. H., Chan, M., Morales-Saldaña, J. M., Muñoz, N. H., et al. (2020). Defining variation in pre-human ecosystems can guide conservation: An example from a Caribbean coral reef. Sci. Rep. 10:2922. doi: 10.1038/s41598-020-59436-y
Oksanen, J., Blanchet, F. G., Friendly, M., Kindt, R., Legendre, P., McGlinn, D., et al. (2017). Community ecology package v.2.4-2.
Page, C. E., Leggat, W., Heron, S. F., Choukroun, S. M., Lloyd, J., and Ainsworth, T. D. (2019). Seeking resistance in coral reef ecosystems: The interplay of biophysical factors and bleaching resistance under a changing climate. Bioessays 41:1800226. doi: 10.1002/bies.201800226
Pandolfi, J. M., and Greenstein, B. J. (1997). Preservation of community structure in death assemblages of deep-water Caribbean reef corals. Limnol. Oceanogr. 42, 1505–1516. doi: 10.4319/lo.1997.42.7.1505
Pandolfi, J. M., and Minchin, P. R. (1995). A comparison of taxonomic composition and diversity between reef coral life and death assemblages in Madang Lagoon, Papua New Guinea. Paleogeogr. Paleoclimatol. Palaeoecol. 119, 321–341. doi: 10.1016/0031-0182(95)00016-X
Pandolfi, J. M., Bradbury, R. H., Sala, E., Hughes, T. P., Bjorndal, K. A., Cooke, R. G., et al. (2003). Global trajectories of the long-term decline of coral reef ecosystems. Science 301, 955–958. doi: 10.1126/science.1085706
Pandolfi, J. M., Connolly, S. R., Marshall, D. J., and Cohen, A. L. (2011). Projecting coral reef futures under global warming and ocean acidification. Science 333, 418–422. doi: 10.1126/science.1204794
Pandolfi, J. M., Lybolt, M., Sommer, B., Narayan, R., and Rachello-Dolmen, P. (2019). “Coral and micro-benthic assemblages from reef habitats in Moreton Bay,” in Moreton Bay Quandamooka & Catchment: Past, present, and future, eds I. R. Tibbetts, P. C. Rothlisberg, D. T. Neil, T. A. Homburg, D. T. Brewer, and A. H. Arthington (Brisbane, QLD: The Moreton Bay Foundation).
Perry, C. T., and Larcombe, L. (2003). Marginal and non-reef-building coral environments. Coral Reefs 22, 427–432. doi: 10.1007/s00338-003-0330-5
Perry, C. T., and Smithers, S. G. (2011). Cycles of coral reef ‘turn-on’, rapid growth, and ‘turn-off’ over the past 8500 years: A context for understanding modern ecological states and trajectories. Glob. Change Biol. 17, 76–86. doi: 10.1111/j.1365-2486.2010.02181.x
Poloczanska, E. S., Brown, C. J., Sydeman, W. J., Kiessling, W., Schoeman, D. S., Moore, P. J., et al. (2013). Global imprint of climate change on marine life. Nat. Clim. Change 3, 919–925. doi: 10.1038/nclimate1958
RCA (2020). Reef Check Australia: Using citizen science to inspire positive action for our reefs and oceans. Available online at: https://www.reefcheckaustralia.org/
R Core Team (2020). R: A language and environment for statistical computing. Vienna: R Foundation for Statistical Computing.
Roff, G., Clark, T. R., Reymond, C. E., Zhao, J., Feng, Y., McCook, L. J., et al. (2013). Palaeoecological evidence of a historical collapse of corals at Pelorus Island, inshore Great Barrier Reef, following European settlement. Proc. R. B Soc. 280:20122100. doi: 10.1098/rspb.2012.2100
Roff, G., Zhao, J. X., and Pandolfi, J. M. (2015). Rapid accretion of inshore reef slopes from the central Great Barrier Reef during the late Holocene. Geology 43, 343–346. doi: 10.1130/G36478.1
Rosenthal, Y., Linsley, B. K., and Oppo, D. W. (2022). Pacific ocean heat content during the past 10,000 years. Science 342, 617–621. doi: 10.1126/science.1240837
Sadler, J., Webb, G. E., Leonard, N. D., Nothdurft, L. D., and Clark, T. R. (2016). Reef core insights into mid-Holocene water temperatures of the southern Great Barrier Reef. Paleoceanogr. Paleoclimatol. 31, 1395–1408. doi: 10.1002/2016PA002943
Sloss, C. R., Murray-Wallace, C. V., and Jones, B. G. (2007). Holocene sea-level change on the southeast coast of Australia: A review. Holocene 17, 999–1014. doi: 10.1177/0959683607082415
Smithers, S. G., Hopley, D., and Parnell, K. E. (2006). Fringing and nearshore coral reefs of the Great Barrier Reef: Episodic Holocene development and future prospects. J. Coast. Res. 2006, 175–187. doi: 10.2112/05A-0013.1
Sommer, B., Butler, I. R., and Pandolfi, J. M. (2021). Trait-based approach reveals how marginal reefs respond to acute and chronic disturbance. Coral Reefs 40, 735–749. doi: 10.1007/s00338-021-02077-y
Sommer, B., Harrison, P. L., Beger, M., and Pandolfi, J. M. (2014). Trait-mediated environmental filtering drives assembly at biogeographic transition zones. Ecology 4, 1000–1009. doi: 10.1890/13-1445.1
Toth, L. T., Precht, W. F., Modys, A. B., Stathakopoulous, A., Robbart, M. L., Hudson, J. H., et al. (2021). Climate and the latitudinal limits of subtropical reef development. Sci. Rep. 11:13044. doi: 10.1038/s41598-021-87883-8
Veron, J. E. N. (2000). Corals of the World. Vol. 1–3. Brisbane, QLD: Australian Institute of Marine Science and CRR.
Veron, J. E. N., and Pichon, M. (1980). Scleractinia of Eastern Australia – Part I-III. Brisbane, QLD: Australian Institute of Marine Science Monograph Series.
Wallace, C. C., Fellegara, I., Muir, P. R., and Harrison, P. L. (2009). The Scleractinian corals of Moreton Bay, S.E. Eastern Australia: High latitude, marginal assemblages with increasing species richness. Mem. QLd. Mus. 54, 1–118.
Walters, I. (1989). Intensified fishery production at Moreton Bay, southeast Queensland, in the late Holocene. Antiquity 63, 215–224. doi: 10.1017/S0003598X00075943
White, S. M., Ravelo, A. C., and Polissar, P. J. (2018). Dampened El Ninþo in the Early and Mid-Holocene due to insolation-forced warming/deepening of the thermocline. Geophys. Res. Lett. 45, 316–326. doi: 10.1002/2017GL075433
Woodroffe, C. D., Brooke, B. P., Linklater, M., Kennedy, D. M., Jones, B. G., Buchanan, C., et al. (2010). Response of coral reefs to climate change: Expansion and demise of the southernmost Pacific coral reef. Geophys. Res. Lett. 37:L15602. doi: 10.1029/2010GL044067
WoRMS Editorial Board (2021). World register of Marine species. Available online at: https://www.marinespecies.org at VLIZ (accessed May 17, 2021).
Yan, S., Zhao, J. X., Lau, A. Y. A., Roff, G., Leonard, N. D., Clark, T. R., et al. (2019). Episodic reef growth in the northern South China Sea linked to warm climate during the past 7,000 years: Potential for future coral refugia. Biogeosciences 124, 1032–1043. doi: 10.1029/2018JG004939
Yomogida, M., Mizuyama, M., Kubomura, T., and Reimer, J. D. (2017). Disappearance and return of an outbreak of the coral-killing cyanobacteriosponge Terpios hoshinota in southern Japan. Zool. Stud. 56:e7. doi: 10.6620/ZS.2017.56-07
Zhao, J., Yu, K., and Feng, Y. (2009). High-precision 238U- 234U – 230Th disequilibrium dating of the recent past: A review. Q. Geochronol. 4, 423–433. doi: 10.1016/j.quageo.2009.01.012
Zhao, M., Yu, K., Zhang, Q., Shi, Q., and Price, G. J. (2012). Long-term decline of a fringing coral reef in the Northern South China sea. J. Coast. Restoration 28, 1088–1099. doi: 10.2112/JCOASTRES-D-10-00172.1
Keywords: historical ecology, high-latitude reefs, urban reefs, water quality, coral reef management, marine paleoecology, climate refuge
Citation: Hammerman NM, Roff G, Lybolt T, Eyal G and Pandolfi JM (2022) Unraveling Moreton Bay reef history: An urban high-latitude setting for coral development. Front. Ecol. Evol. 10:884850. doi: 10.3389/fevo.2022.884850
Received: 27 February 2022; Accepted: 26 July 2022;
Published: 11 August 2022.
Edited by:
Chris Schneider, University of Alberta, CanadaReviewed by:
Benny K. K. Chan, Academia Sinica, TaiwanCopyright © 2022 Hammerman, Roff, Lybolt, Eyal and Pandolfi. This is an open-access article distributed under the terms of the Creative Commons Attribution License (CC BY). The use, distribution or reproduction in other forums is permitted, provided the original author(s) and the copyright owner(s) are credited and that the original publication in this journal is cited, in accordance with accepted academic practice. No use, distribution or reproduction is permitted which does not comply with these terms.
*Correspondence: Nicholas M. Hammerman, bi5oYW1tZXJtYW5AdXEubmV0LmF1
Disclaimer: All claims expressed in this article are solely those of the authors and do not necessarily represent those of their affiliated organizations, or those of the publisher, the editors and the reviewers. Any product that may be evaluated in this article or claim that may be made by its manufacturer is not guaranteed or endorsed by the publisher.
Research integrity at Frontiers
Learn more about the work of our research integrity team to safeguard the quality of each article we publish.