- Department of Biology, Lund University, Lund, Sweden
Billions of birds migrate every year. To conduct a successful migration, birds undergo a multitude of physiological adaptions. One such adaptation includes adjustments of immune function, however, little is known about intraspecies (between-individual) and interspecies (between-species) variation in immune modulations during migration. Here, we explore if early and late migrating individuals differ in their immune function, and if such patterns differ among species with short- vs. long-distance migration strategies. We quantified three parameters of baseline (constitutive) innate immune function and one parameter of baseline (constitutive) acquired immune function in 417 individuals of 10 species caught during autumn migration at Falsterbo (Sweden). Early and late migrating individuals differed in lysis and total immunoglobulins (IgY), but the patterns show different directions in long-distance migrants (LDMs) (wintering in Africa) as compared to short-distance migrants (SDMs) (wintering within Europe). Specifically, early migrating LDMs had lower lysis but higher immunoglobulin levels than late migrating individuals. In short distance migrants, there was no difference in lysis between early and late migrating individuals, but immunoglobulin levels were higher in late migrating individuals. We found no correlation between timing of migration and haptoglobin, but LDMs had lower levels of haptoglobin than SDMs. We also found that the prevalence of haemosporidian blood parasite infections decreased in LDMs, but increased in SDMs, as the autumn progressed. Taken together, our study suggests that the investment into immune function depends on the migratory strategy (short- vs. long-distance migrants), and that early and late migrating individuals of a migration strategy might invest differently in baseline immune function, potentially driven by differences in the trade-offs with timing and speed of migration. Our study highlights the potential adaptations of immune function that could help explain trade-offs with other physiological systems, and behavioural responses during migration.
Introduction
Each year billions of birds migrate from breeding to non-breeding grounds. The timing of migration, as well as the distance covered between the breeding and the wintering grounds, differs greatly both among and within species. Species migrating to winter in sub-Saharan Africa typically migrate in early autumn and are often referred to as long-distance migrants (LDMs) (Berthold, 1993; Newton, 2008). In contrast, species migrating from Northern Europe to winter in western or southern Europe are often referred to as short-distance (or medium-distance) migrants and typically migrate later during the autumn season (Berthold, 2001; Newton, 2008). LDMs are hypothesised to be time-minimisers, selected to arrive at the wintering grounds as early as possible (Alerstam and Lindström, 1990; Hedenström and Alerstam, 1997; Alerstam et al., 2003). On the other hand, short-distance migrants (SDMs) are more likely to be energy-minimisers (Alerstam and Lindström, 1990; Hedenström and Alerstam, 1997). During migration, birds need to adjust their physiology to a multitude of challenges, including but not limited to environmental changes and sustained periods of extreme physical exertion (Piersma and van Gils, 2011). During the last decades, studies on the physiological adjustments required during migration have focused on energetic aspects (and hormonal adjustments) (Blem, 1980; Alerstam and Lindström, 1990; Gwinner, 1990; Lindström and Alerstam, 1992; Lindström, 2003; Guglielmo, 2010; Piersma and van Gils, 2011). However, recent studies indicate that there are also a number of other physiological systems adjusted during migration (Piersma and van Gils, 2011; Cooper-Mullin and McWilliams, 2016; Hegemann et al., 2019; McWilliams et al., 2021). In particular, some studies have highlighted that migration is connected with changes in the immune system that can influence migratory behaviour and processes. For example, both constitutive immune function and responsiveness to immune challenges can be reduced during the migratory season (Owen and Moore, 2006, 2008a,2008b; Buehler et al., 2008b,2010b; Eikenaar and Hegemann, 2016). Also trade-offs between immune function and other physiological systems like oxidative balance may occur during migration (Eikenaar et al., 2018) and stopovers may not only be required for refuelling, but also for recovery of immune function (Owen and Moore, 2008b; Buehler et al., 2010b; Eikenaar et al., 2020a,b). Furthermore, levels of constitutive immune function relate to stopover duration (Hegemann et al., 2018b) and to the length of migration delays if individuals need to mount an immune response during the migratory season (Hegemann et al., 2018a). However, many questions regarding these physiological adaptations still remain open (Schmaljohann and Eikenaar, 2017; Hegemann et al., 2019).
The immune system protects the body from pathogens and other non-self particles and is therefore important for survival (Roitt et al., 1998), but at the same time, it incurs costs in terms of production, maintenance and activation (Klasing, 2004; Hasselquist and Nilsson, 2012). Several studies found evidence for downregulation of (parts of) constitutive immune function during migration (Owen and Moore, 2006; Buehler et al., 2010b; Eikenaar and Hegemann, 2016), presumably resulting from resource or risk trade-offs (Owen and Moore, 2008b; Buehler et al., 2010b; Eikenaar et al., 2020a). However, information whether there are general differences between species with different migratory strategies [e.g., long-distance vs. short-distance migrants (SDMs)] is currently lacking. Migratory strategies may also lead to different trade-offs with the demands for migration itself, resulting in differential investment in immune function (Hasselquist, 2007; Buehler and Piersma, 2008; Buehler et al., 2010a). In particular, LDMs have been hypothesised to have a higher susceptibility to infectious diseases, because the high physiological demands of long-distance migration may lead to reduced immune function (Klaassen et al., 2012). Furthermore, both the timing and the destination of migration may influence the risk of exposure to pathogens encountered by the migrants (Altizer et al., 2011; Hall et al., 2016). For example, many infectious diseases as well as vectors of pathogens show seasonal patterns and hence early migrating individuals and species may be exposed to other disease risks than late migrating species or individuals (Altizer et al., 2006; van Dijk et al., 2014), and tropical areas are supposed to harbour more pathogens than temperate regions (Altizer et al., 2011; Westerdahl et al., 2014). Finally, within species there is considerable variation in timing of migration, which may result in individual differences in trade-offs between immune function and migration. Yet, comprehensive data on seasonal patterns of immune function among long- and short-distance migrants, as well as early and late migrating individuals within a species, are lacking. Such knowledge would also help to understand disease transmission patterns and to identify the time windows with the highest risk of spreading of disease (Owen et al., 2021).
A recent meta-analysis of published papers on infection status and infection intensity in migratory hosts found that across animal taxa, parasites have a negative impact on migration performance and that migratory performance decreased with increasing infection intensity (Risely et al., 2018). Hence, infected birds migrate more slowly and may therefore lag behind conspecifics. Yet, data on temporal segregation of infected birds across a migratory season are still rare. An analysis of four passerine species on spring migration revealed that late migrating individuals had a much higher prevalence of haemosporidian blood parasites [one of the most common chronic infections in birds (Valkiūnas, 2004)] than early migrating individuals (Emmenegger et al., 2018). In Yellow-rumped Warblers Dendroica coronate during spring migration, both the probability and the intensity of blood parasite infections were higher for later migrating birds (DeGroote and Rodewald, 2010). Both studies suggest that blood parasite infections may hamper spring migration (see also Garvin et al., 2006) or that reduced feather growth during moult in infected individuals may delay the onset of spring migration (see Marzal et al., 2013). Indeed, during autumn migration, SDMs infected with avian haemosporidians had three times as long stopover periods as uninfected birds, though there was no difference in stopover duration between infected and uninfected LDMs (Hegemann et al., 2018b). However, that study did not investigate effects of seasonal timing. To our knowledge, the only study investigating seasonal patterns of blood parasite infections in autumn migrating birds found that late migrating European Robins Erithacus rubecula tended to have higher prevalence of avian malaria than early migrating individuals (Agh et al., 2019). Hence, comprehensive data on temporal differences between infected and non-infected individuals during autumn migration are still missing.
In this study, we investigated if LDMs and SDMs differ in baseline (constitutive) immune function and if, within either group, early-migrating individuals differ from late migrating individuals. We sampled 417 individual birds of 10 species during autumn migration stopover at Falsterbo (Sweden). Our aims were to quantify (1) if early migrating individuals (within a species) have different immune function or different haemosporidian prevalence compared to late migrating individuals, and (2) if these patterns differ between LDMs and SDMs. We hypothesised that due to stronger selection for time-minimisation, late LDMs would have lower baseline immune function compared to early migrating individuals as they need to invest more resources in fast migration. In contrast, we did not expect any differences in immune function between early and late migrants among SDMs as all of them were relatively close to their final destination and therefore not time-stressed. Regarding blood parasite infections, following earlier work during spring migration, we expected late individuals to have higher prevalence of blood parasites than early migrating individuals independent of the migration strategy.
Materials and Methods
We sampled passerine species during the 2014 autumn migration season at the Falsterbo Peninsula, a strategic stopover site in Southwest Sweden (55.383°N, 12.816°E). Birds were caught as part of a standardised ringing project in the lighthouse garden assumed to be migrants during stopover (Karlsson and Bentz, 2004; Hegemann et al., 2018a,b). We sampled birds between 26 August and 11 November. Birds were caught between sunrise and 12:45 h CET and mistnets (maximum of 21 nets of 9 m each; 16 mm mash size, see Karlsson and Bentz, 2004) were checked every half hour and birds were brought for further handling into the ringing hut. Birds were aged based on plumage characteristics (Svensson, 1992) and we included only hatch-year birds (which constitute about 90% of all ringed birds at Falsterbo; Falsterbo Bird Observatory, unpublished data) in our study. All species of which less than 10 individuals were sampled were excluded, resulting in samples from 417 individuals of 10 species. Six of those species (Blackbird Turdus merula, n = 15, Blue Tit Cyanistes caeruleus, n = 38, Chaffinch Fringilla coelebs, n = 24, Dunnock Prunella modularis, n = 63, Robin E. rubecula, n = 98, and Song Thrush Turdus philomelos, n = 62) are SDMs that winter in Western and Southern Europe, and four species (Common Redstart Phoenicurus phoenicurus, n = 33, Garden Warbler Sylvia borin, n = 20, Tree Pipit Anthus trivialis, n = 27, Willow Warbler Phylloscopus trochilus, n = 37) are LDMs that winter in sub-Saharan Africa.
We collected blood samples (∼100 μl; always less than 10% of an individual’s total blood volume) by puncturing the brachial vein with a sterile 27G needle and transferring blood via heparinised capillary tubes (Hirschmann Laborgeräte GmBH, Germany) into Eppendorf tubes. Blood samples were kept on ice in an Eppendorf tube until we returned to the lab the same day, and then centrifuged for 10 min at 7000 rpm to separate plasma from red blood cells. Samples were stored in Eppendorf tubes at −50°C until subsequent laboratory analysis.
Immune Assays
We used two assays that focus on the innate immune system and one assay that focus on the acquired immune system (Hegemann et al., 2018a,b). The innate immune system constitutes the important first line of defence (Janeway et al., 2005). Levels of constitutive innate immune function relate to pathogen pressure (Horrocks et al., 2012, 2015) and show consistencies over longer time scales (Hegemann et al., 2012), but also experience modulations during migration (Eikenaar et al., 2020a,b). Specifically, we used a haemolysis-haemagglutination assay to quantify titres of complement-like lytic enzymes (measured as lysis titres) and non-specific natural antibodies (measured as natural antibody titres) and followed the method described by Matson et al. (2005). Scanned images of individual samples serially diluted in Rabbit red blood cells (Alsevers, S.BC-0009, Envigo, United Kingdom) were randomised among all plates and scored blindly to sample ID (by AH). If scores differed by >1, then a third score was taken and the median was used as a final value. We used a commercially available colorimetric assay kit (Tp801; Tridelta Development Ltd.) to quantify haptoglobin concentrations in plasma samples (Matson et al., 2012). We followed the manual method as described in the manufacturer manual with a few minor modifications. We measured absorbance at three wavelengths (405, 450, and 630 nm) prior to the addition of the final reagent that initiated the colour change reaction. The 405 and 450 nm pre-scan enabled us to statistically analyse and correct for differences in plasma sample redness, an indication of haemolysis, which can affect the assay (Matson et al., 2012; Wemer et al., 2021). Haptoglobin is an acute phase protein that circulates in the blood and during a pathogenic challenge haptoglobin concentration typically increases following release from the liver. Acquired immune function reflects the investment into immune function over longer time scales and hence is likely related to phenotypic quality (Hasselquist et al., 2001). We used an enzyme-linked immunosorbent assay (ELISA) to quantify the total level of antibodies (IgY) following the methods exactly as outlined in Sköld-Chiriac et al. (2014). Samples were defrosted and refrozen between each assay, but these assays are robust to repeated freeze-thaw cycles (Hegemann et al., 2017).
Molecular Analyses of Blood Parasites
DNA from preserved blood was extracted using standard phenol/chloroform methods (Sambrook et al., 2002) and subsequently diluted to 25 ng μl–1 (concentration determined using a nanodrop). To determine infection status with haemosporidian species of the genera Haemoproteus, Plasmodium, and Leucocytozoon, we used a nested PCR amplifying a partial segment of the cytochrome b gene and using both Haem-F/Haem-R2 and Haem-FL/Haem-R2L primer pairs following Hellgren et al. (2004). We subsequently grouped the data by birds having no infection, birds having one infection (Haemoproteus/Plasmodium or Leucocytozoon), or birds having double infection (Haemoproteus/Plasmodium and Leucocytozoon). Since we only consider first year birds in our analyses, all infections must have occurred on the breeding grounds and birds cannot yet be infected with lineages that are transmitted on the wintering grounds (see Hellgren et al., 2007).
Statistics
We analysed our data using linear mixed models [function lmer, package lme4 (Bates et al., 2015)] and the program R version 3.6.3 (R Development Core Team, 2020). Even though measurements of baseline immune function are usually not affected by short-term handling stress (Buehler et al., 2008a; but see Zylberberg, 2015), we first checked if handling time and immune parameters were correlated in our dataset, but this was not the case for any parameter (always F < 2.31, p > 0.13).
To separate within-species effects from between-species effects, we applied the methods described by van de Pol and Wright (2009). We used the immune parameters and blood parasite infection as dependent variable and the two-way-interactions of within-species-date effect and strategy (long-distance vs. short distance migrant), and between-species-date effect and strategy as explanatory variables. Species was included as random intercept effect. The model with haptoglobin included a measurement at 405 nm as covariate to correct for plasma redness as this measurement explained more variation than then 450 nm measurement (Matson et al., 2012; Wemer et al., 2021). Blood parasite infections were grouped into three levels (no infection, single infection, double infection).
We always started with the full model and removed interactions if p > 0.1 based on backward elimination and the drop1 function in R. We kept all fixed effects independent of significance. Assumptions of all models were checked on the residuals of the final model. Residuals from the model having agglutination as dependent variable deviated from normality and transformations did not improve the fit. However, since general linear models are robust against deviation from normality (Zuur et al., 2010), we still consider the results to be valid. We extracted and plotted marginal means using the function ggpredict [package ggeffects (Lüdecke, 2018)].
Results
Overall, early migrating individuals of LDMs had lower lysis, higher immunoglobulin levels and a higher prevalence of blood parasite infections than late migrating individuals (Figures 1, 2 and Table 1). In contrast, among SDMs, early individuals did not differ from late individuals in lysis, but had lower immunoglobulin levels and lower prevalence of blood parasite infections than late migrating individuals (interaction within-species-date-effect × strategy: lysis: p = 0.062, immunoglobulins: p = 0.048, blood parasite infections: p = 0.005, Figures 1, 2, for full statistical results, see Table 1). Agglutination and haptoglobin concentrations did not differ between early and late migrating individuals, and there was no effect of migration strategy (interaction within-species-date-effect × strategy: agglutination: p = 0.283, haptoglobin: p = 0.713; within-species-date effect: agglutination: p = 0.404, haptoglobin: p = 0.984, Figures 1, 2 and Table 1). In general, LDMs had lower haptoglobin concentrations (p = 0.049) and a non-significant trend for lower agglutination (p = 0.087) than SDMs (Table 1). Species-specific figures showing all individual data points can be found in the Supplementary Figures 1–5.
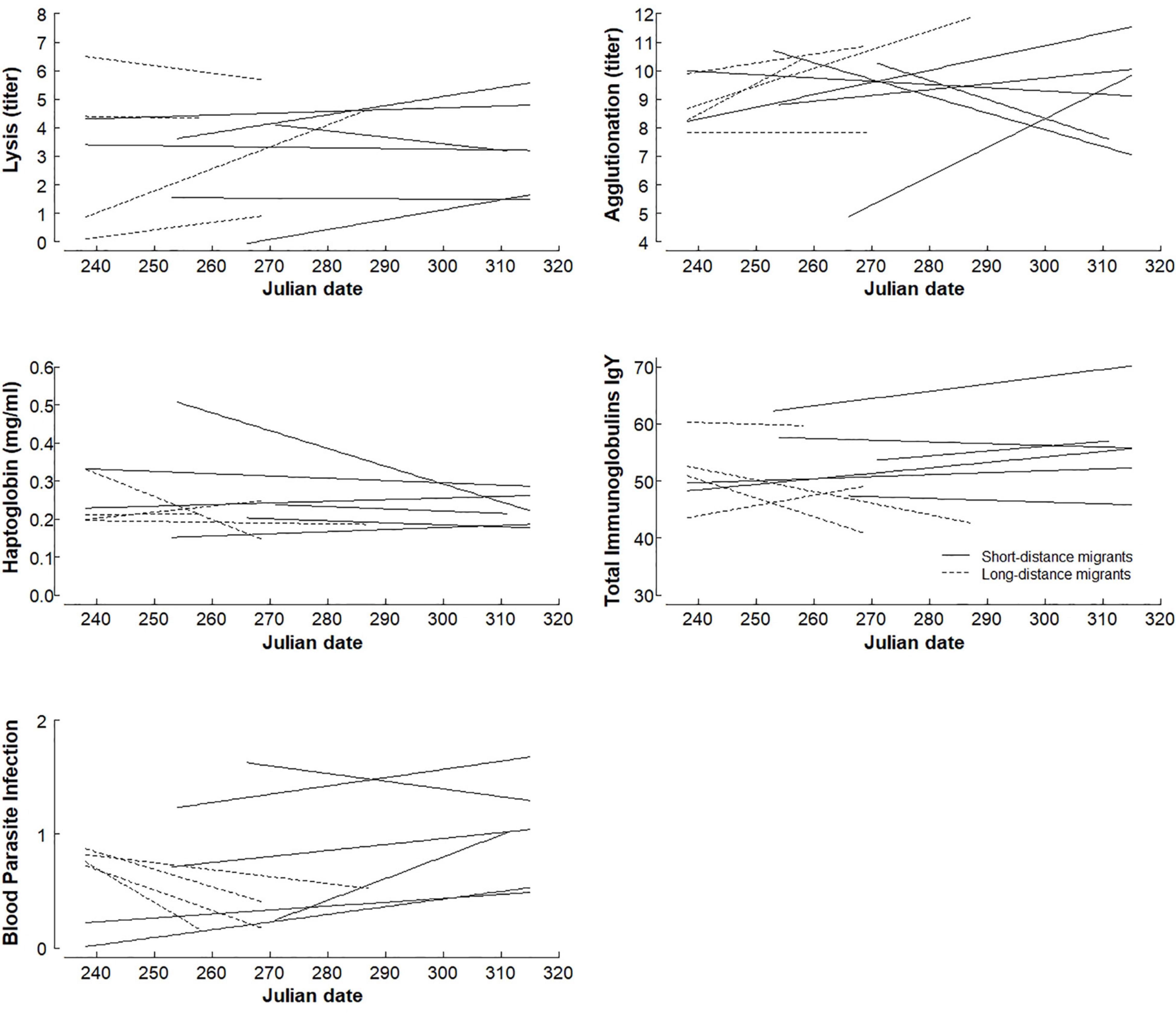
Figure 1. Within-seasonal patterns of constitutive immune function and blood parasite infections in 417 individual migrants from 10 species sampled during autumn migration in Falsterbo, Sweden. Each line represents the relationship between Julian date and immune function or blood parasite infection for a single species, with dashed lines representing long-distance migrants (wintering in Africa) and solid lines presentencing short-distance migrants (wintering in Southern and Western Europe). Note that y-axis for agglutination and total immunoglobulins do not start at zero. Figures showing individual data points for each species can be found in the Supplementary Material.
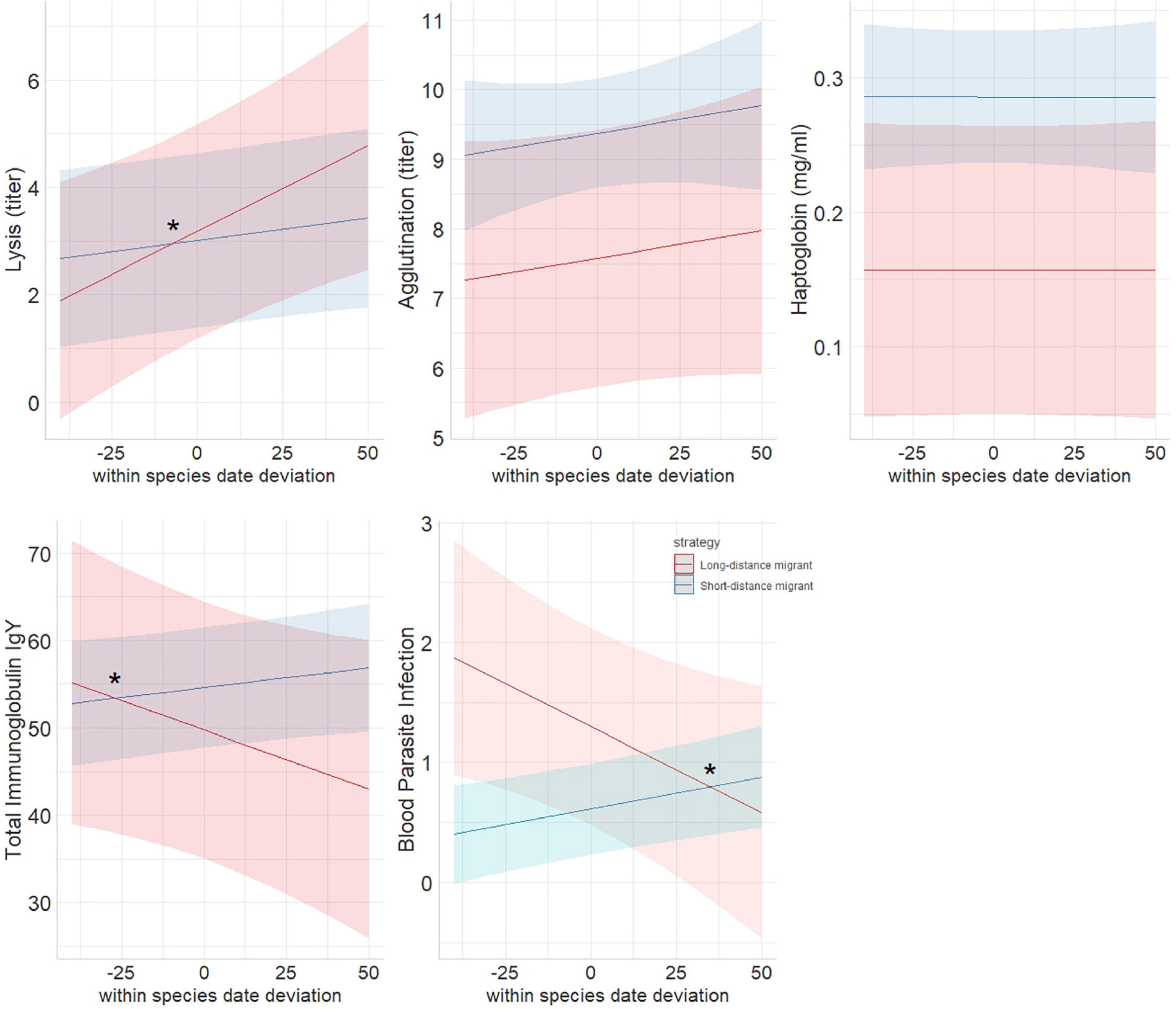
Figure 2. Marginal means from linear mixed models showing within-species-date effect on immune function in long-distance and short-distance migrants sampled during autumn migration at Falsterbo, Sweden. A negative slope means that early migrating individuals have a higher value than late migrating individuals, a positive slope that late migrating individuals have higher values than early migrating individuals. Stars denote significant interactions. Haptoglobin significantly differed between the strategies. For details of statistical results, see section “Results” and Table 1.
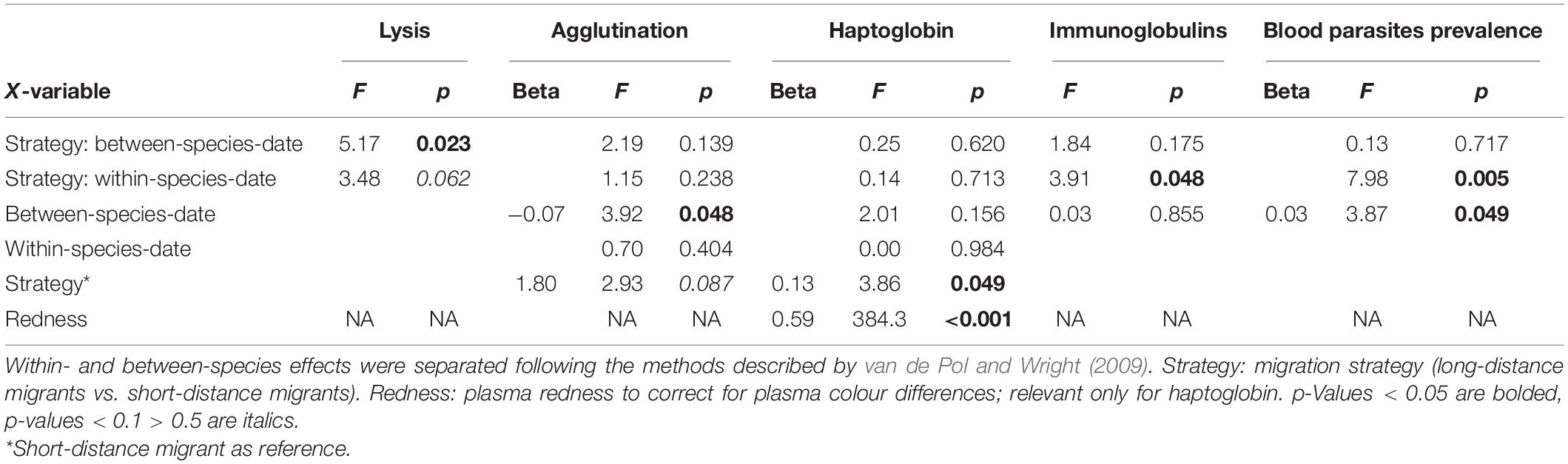
Table 1. Statistics and coefficients for linear mixed models analysing immune function and blood parasite infections in migrating birds during autumn migration at Falsterbo, Sweden.
Discussion
We show that several parameters of constitutive (baseline) immune function and blood parasite infections differ between early and late migrating individuals, and that these patterns differ between long- and short-distance migrants. Thus, our study suggests that over the autumn migration period immune function is modulated in different ways depending on migration strategy.
Specifically, early migrating LDMs had lower complement activity (lysis) than late migrating individuals, while there was no such difference in SDMs. That early and late individuals of LDMs differ indicates a trade-off with migration timing in these time-minimisers (Klaassen et al., 2012). Individuals migrating early may have to make a trade-off with investment in immune function, in this case complement activity, a parameter that has been linked to survival probabilities (Hegemann et al., 2015; Roast et al., 2020). While early departure from Scandinavia might be beneficial in terms of early crossing of the Sahara and hence early arrival at the wintering grounds (Alerstam and Hedenström, 1998) this may come at the cost of innate immune function which still develops in young birds after independence (Stambaugh et al., 2011; Vermeulen et al., 2017; van Dijk et al., 2020; Aastrup and Hegemann, 2021). The higher immunoglobulin levels in early migrating LDMs compared to late migrating individuals may seem to contradict this hypothesis. However, immunoglobulin levels are thought to reflect phenotypic quality/condition (Hasselquist et al., 2001) and particularly LDMs that will encounter more novel pathogens, may benefit from investing in this part of immune function to be prepared for entering (tropical) wintering quarters with a higher pathogen pressure (Westerdahl et al., 2014; O’Connor et al., 2020). Low immunoglobulin levels among late migrating LDMs may then suggest that these individuals are of lower quality/condition and that they can therefore not initiate migration as early as those with high immunoglobulin levels. For SDMs, immunoglobulin levels instead increased over the autumn migration period. This may reflect that among SDMs high quality/condition individuals are migrating later in the autumn. Alternatively, this might be an effect of that the levels of circulating immunoglobulins are increasing over the autumn in these young birds as they are exposed to more and more pathogens. Such an effect may be particularly visible in SDMs as those birds are on average 2 months older when they migrate as compared to LDMs, because SDMs hatch earlier and migrate later.
Natural antibodies (agglutination) did not differ between early and late migrating individuals, which may suggest that this part of immune function is not involved in trade-offs related to migration. In line with this, several previous studies failed to find any correlation between agglutination and migration related traits (Buehler et al., 2010b; Nebel et al., 2012, 2013; Hegemann et al., 2018a,b; Eikenaar et al., 2020b). These studies as well as our current study instead suggest that other immune parameters (e.g., complement activity, haptoglobin concentration, immunoglobulins, bacteria killing capacity) seem to be more important for trade-offs between immune function and physiological or behavioural adaptations related to migration.
Haptoglobin levels did not differ between early and late migrating individuals. Since haptoglobin is an acute phase protein that increases during infection (Quaye, 2008), similar levels between early and late individuals might not be surprising. Interestingly though, LDMs had on average lower haptoglobin concentrations compared to SDMs. Besides its role for immune function, haptoglobin has antioxidant properties as it can bind haemoglobin, a mechanism potentially important during long endurance flights as haptoglobin levels were negatively correlated with flight duration in European Starlings (Sturnus vulgaris) flying in a wind tunnel (Nebel et al., 2012). Since LDMs tend to fly longer distances during each migratory flight compared to SDMs (Schmaljohann, 2019; Packmor et al., 2020), this may explain the difference in haptoglobin between long- and short-distance migrants.
Prevalence of blood parasite infections increased in late migrating SDMs compared to early migrating birds and this is in line with findings in autumn migrating Robins in Hungary (Agh et al., 2019). An increase in blood parasite prevalence over the autumn in SDMs also supports the hypothesis that infected individuals migrate more slowly (Risely et al., 2018) and thus later, for example because of longer stopovers or shorter flight bouts (Hegemann et al., 2018b). Furthermore, infection with blood parasites can reduce feather growth (Marzal et al., 2013) and may delay the onset of migration in infected individuals. In contrast, migrating LDMs’ prevalence of blood parasites decreased over the autumn migration period, which is contrary to our prediction and to previous results for LDMs [though during spring migration (Emmenegger et al., 2018)]. This contradicts the hypothesis of infected migrants being slowed down. However, since LDMs have the majority of their migratory flight still ahead of them, we cannot rule out that infected individuals migrate more slowly and hence are lagging behind uninfected individuals and that this pattern may only be visible later in the migratory season. Alternatively, but not mutually exclusive, early departure from the breeding grounds of infected individuals may be an adaptive strategy if they can anticipate that blood parasite infection may slow them down along the route. Another explanation may include selective disappearance of late migrating low-quality individuals infected with blood parasites (Marzal et al., 2016). Specifically, if late migrating long-distance migratory individuals are of poorer quality, as indicated by their lower levels of immunoglobulins (see above), those low-quality individuals may have reduced capacities to fight a haemosporidian infection (Gonzalez et al., 1999) and hence experience higher mortality during infection than early migrating high-quality individuals. This explanation may also resolve the apparent contradiction that early migrating LDMs have both high immunoglobulin levels (indicating high quality) and high prevalence of blood parasites. It seems unlikely that infections had already retracted into organs and were undetectable in the blood (Cosgrove et al., 2008), because prevalence increased over time and was on average much higher in late migrating SDMs. Furthermore, in a local bird community in southern Sweden, several long-distant migratory species showed no trend in parasite prevalence in late summer and early autumn when considering only first-year birds (Huang et al., 2020), and in blue tits Plasmodium blood parasites become dormant only from the end of October onwards (Cosgrove et al., 2008). Even though the overall patterns between blood parasite infections and immunoglobulins seem to parallel each other, we found no correlation on the individual level (Hegemann et al., in preparation).
Conclusion and Future Directions
Our study adds to the growing literature that other physiological systems than those directly linked to energy turnover are modulated and thus variable during migration, and that this may be particularly true for the immune system (Buehler et al., 2010a; Hegemann et al., 2019) with potentially substantial impact on fitness-related factors (Roast et al., 2020; Dunn et al., 2021). That the different immune parameters did not show any common time patterns over the autumn migration period may highlight the different functions and costs of different immune parameters (Adamo, 2004; Matson et al., 2006; Demas et al., 2011) and that these trade-offs may differ between migration strategies. It also suggests that there is no general down-regulation or up-regulation of immune function, but that different parameters show different patterns within species, as well as between species using different migration strategies (i.e., short- and long-distance migrants). Hence, our results stress the importance of measuring multiple immune parameters in migration studies. The differences between early and late individuals also suggests that trade-offs between immune function and migration speed or other physiological systems might change over time as the migration season progresses. Future studies should aim at getting repeated samples from the same individuals both at the same stopover sites as well as during different stopovers along the migratory route to understand how flexible or fixed different immune strategies are. Satellite tracking of birds as well as radio-tracking in areas covered by a network of receiving stations like the Motus system (Taylor et al., 2017) may offer such possibilities. Understanding such variations in immune function will not only be vital for understanding the ecology and evolution of migration (Hegemann et al., 2019), but may also be important for predicting the interplay between birds and diseases under climate change (Hall, 2021).
Data Availability Statement
The raw data supporting the conclusions of this article will be made available by the authors on request.
Ethics Statement
The animal study was reviewed and approved by the Malmö/ Lund Ethical Committee.
Author Contributions
AH, J-ÅN, and DH: study design. AH: field work, statistical analyses, and first draft of the manuscript. AH and CB: labwork. AH, CB, DH, and J-ÅN: discussion and interpretation of data. All authors gave substantial input on the manuscript and approved the submitted version of the manuscript.
Funding
AH was supported by a Rubicon PostDoc-Fellowship (825.13.022) from the Netherlands Organization for Scientific Research. This study was also supported by grants from the Swedish Research Council (2018-04278 to AH; 2016-04391 and 2020-03976 to DH; and 621-2013-4386 and 2016-04240 to J-ÅN), and by grants from the Royal Physiographic Society of Lund (to AH). DH was also supported by the European Research Council (ERC) under the European Union’s Horizon 2020 Research and Innovation Programme (ERC Advanced grant no. 742646).
Conflict of Interest
The authors declare that the research was conducted in the absence of any commercial or financial relationships that could be construed as a potential conflict of interest.
Publisher’s Note
All claims expressed in this article are solely those of the authors and do not necessarily represent those of their affiliated organizations, or those of the publisher, the editors and the reviewers. Any product that may be evaluated in this article, or claim that may be made by its manufacturer, is not guaranteed or endorsed by the publisher.
Acknowledgments
We thank the staff and volunteers of the Falsterbo Bird Observatory, in particular Sophie Ehnbom, Caroline Sjöström, and Lennart Karlsson, for generous access to the birds, support, and assistance. We also thank Thomas Alerstam for valuable discussions and Pablo Alcalde Abril for much help during fieldwork. Martijn van de Pol and various colleagues at Lund University gave valuable advice and discussions on the statistical analyses. We also thank the two reviewers for their feedback. This is report no. 335 from Falsterbo Bird Observatory.
Supplementary Material
The Supplementary Material for this article can be found online at: https://www.frontiersin.org/articles/10.3389/fevo.2022.880426/full#supplementary-material
References
Aastrup, C., and Hegemann, A. (2021). Jackdaw nestlings rapidly increase innate immune function during the nestling phase but no evidence for a trade-off with growth. Dev. Comp. Immunol. 117:103967. S0145-305X(20)30521-8
Adamo, S. A. (2004). How should behavioural ecologists interpret measurements of immunity? Anim. Behav. 68, 1443–1449. doi: 10.1016/j.anbehav.2004.05.005
Agh, N., Piross, I. S., Majoros, G., Csorgo, T., and Szollosi, E. (2019). Malaria infection status of European Robins seems to associate with timing of autumn migration but not with actual condition. Parasitology 146, 814–820. doi: 10.1017/S0031182018002184
Alerstam, T., and Hedenström, A. (1998). The development of bird migration theory. J. Avian Biol. 29, 343–369. doi: 10.2307/3677155
Alerstam, T., Hedenstrom, A., and Akesson, S. (2003). Long-distance migration: evolution and determinants. Oikos 103, 247–260. doi: 10.1034/j.1600-0706.2003.12559.x
Alerstam, T., and Lindström, Å (1990). “Optimal bird migration: The relative importance of time, energy, and safety,” in Bird Migration: the Physiology and Ecophysiology, ed. E. Gwinner (Berlin: Springer), 331–351. doi: 10.1007/978-3-642-74542-3_22
Altizer, S., Bartel, R., and Han, B. A. (2011). Animal migration and infectious disease risk. Science 331, 296–302. doi: 10.1126/science.1194694
Altizer, S., Dobson, A., Hosseini, P., Hudson, P., Pascual, M., and Rohani, P. (2006). Seasonality and the dynamics of infectious diseases. Ecol. Lett. 9, 467–484. doi: 10.1111/j.1461-0248.2005.00879.x
Bates, D., Maechler, M., Bolker, B., and Walker, S. (2015). Fitting Linear Mixed-Effects Models Using lme4. J. Stat. Software 67, 1–148. doi: 10.18637/jss.v067.i01HH
Blem, C. R. (1980). “The energetics of migration,” in Animal Migration, Orientation, and Navigation, ed. S. A. J. Gauthreaux (New York: Academic Press), 175–224. doi: 10.1016/B978-0-08-091833-4.50008-0
Buehler, D. M., Bhola, N., Barjaktarov, D., Goymann, W., Schwabl, I., Tieleman, B. I., et al. (2008a). Constitutive immune function responds more slowly to handling stress than corticosterone in a shorebird. Physiol. Biochem. Zool. 81, 673–681. doi: 10.1086/588591
Buehler, D. M., Piersma, T., Matson, K., and Tieleman, B. I. (2008b). Seasonal redistribution of immune function in a migrant shorebird: annual-cycle effects override adjustments to thermal regime. Am. Nat. 172, 783–796. doi: 10.1086/592865
Buehler, D. M., and Piersma, T. (2008). Travelling on a budget: predictions and ecological evidence for bottlenecks in the annual cycle of long-distance migrants. Philos. Trans. R. Soc. Lond. B. 363, 247–266. doi: 10.1098/rstb.2007.2138
Buehler, D. M., Tieleman, B. I., and Piersma, T. (2010a). How Do Migratory Species Stay Healthy Over the Annual Cycle? A Conceptual Model for Immune Function and For Resistance to Disease. Integr. Comp. Biol. 50, 346–357. doi: 10.1093/icb/icq055
Buehler, D. M., Tieleman, B. I., and Piersma, T. (2010b). Indices of Immune Function are Lower in Red Knots (Calidris canutus) Recovering Protein than in those Storing Fat during Stopover in Delaware Bay. Auk 127, 394–401. doi: 10.1525/auk.2010.09017
Cooper-Mullin, C., and McWilliams, S. R. (2016). The role of the antioxidant system during intense endurance exercise: lessons from migrating birds. J. Exp. Biol. 219, 3684–3695. doi: 10.1242/jeb.123992
Cosgrove, C. L., Wood, M. J., Day, K. P., and Sheldon, B. C. (2008). Seasonal variation in Plasmodium prevalence in a population of blue tits Cyanistes caeruleus. J. Anim. Ecol. 77, 540–548. ER doi: 10.1111/j.1365-2656.2008.01370.x
DeGroote, L. W., and Rodewald, P. G. (2010). Blood parasites in migrating wood-warblers (Parulidae): effects on refueling, energetic condition, and migration timing. J. Avian Biol. 41, 147–153. doi: 10.1111/j.1600-048X.2009.04782.x
Demas, G. E., Zysling, D. A., Beechler, B. R., Muehlenbein, M. P., and French, S. S. (2011). Beyond phytohaemagglutinin: assessing vertebrate immune function across ecological contexts. J. Anim. Ecol. 80, 710–730. doi: 10.1111/j.1365-2656.2011.01813.x
Dunn, J., Hawley, D. M., Huyvaert, K. P., and Owen, J. C. (2021). “Fitness effects of parasite infection in birds,” in Infectious Disease Ecology of Wild Birds, eds J. Owen, D. M. Hawley, and K. P. Huyvaert (Oxford:Oxford University Press), 99–120. doi: 10.1093/oso/9780198746249.003.0006
Eikenaar, C., and Hegemann, A. (2016). Migratory common blackbirds have lower innate immune function during autumn migration than resident conspecifics. Biol. Lett. 12:20160078. doi: 10.1098/rsbl.2016.0078
Eikenaar, C., Hegemann, A., Packmor, F., Kleudgen, I., and Isaksson, C. (2020a). Not just fuel: energy stores are correlated with immune function and oxidative damage in a long-distance migrant. Curr. Zool. 66, 21–28. doi: 10.1093/cz/zoz009
Eikenaar, C., Hessler, S., and Hegemann, A. (2020b). Migrating birds rapidly increase constitutive immune function during stopover. R. Soc. Open Sci. 7:192031. doi: 10.1098/rsos.192031
Eikenaar, C., Isaksson, C., and Hegemann, A. (2018). A hidden cost of migration? Innate immune function versus antioxidant defense. Ecol. Evol. 8, 2721–2728. doi: 10.1002/ece3.3756
Emmenegger, T., Bauer, S., Hahn, S., Muller, S. B., Spina, F., and Jenni, L. (2018). Blood parasites prevalence of migrating passerines increases over the spring passage period. J. Zool. 306, 23–27. doi: 10.1111/jzo.12565
Garvin, M. C., Szell, C. C., and Moore, F. R. (2006). Blood parasites of Nearctic-Neotropical migrant passerine birds during spring trans-gulf migration: Impact on host body condition. J. Parasitol. 92, 990–996. doi: 10.1645/GE-758R.1
Gonzalez, G., Sorci, G., Moller, A. P., Ninni, P., Haussy, C., and De Lope, F. (1999). Immunocompetence and condition-dependent sexual advertisement in male house sparrows (Passer domesticus). J. Anim. Ecol. 68, 1225–1234. doi: 10.1046/j.1365-2656.1999.00364.x
Guglielmo, C. G. (2010). Move that fatty acid: fuel selection and transport in migratory birds and bats. Integr. Comp. Biol. 50, 336–345. doi: 10.1093/icb/icq097
Hall, R. (2021). “Climate change and avian disease,” in Infectious Disease Ecology of Wild Birds, eds J. Owen, D. M. Hawley, and K. P. Huyvaert (Oxford:Oxford University Press), 189–205.
Hall, R. J., Brown, L. M., and Altizer, S. (2016). Modeling Vector-Borne Disease Risk in Migratory Animals Under Climate Change. Integr. Comp. Biol. 56, 353–364. doi: 10.1093/icb/icw049
Hasselquist, D. (2007). Comparative immunoecology in birds: hypotheses and tests. J. Ornithol. 148, S571–S582. doi: 10.1007/s10336-007-0201-x
Hasselquist, D., and Nilsson, J. Å (2012). Physiological mechanisms mediating costs of immune responses: what can we learn from studies of birds? Anim. Behav. 83, 1303–1312. doi: 10.1016/j.anbehav.2012.03.025
Hasselquist, D., Wasson, M. F., and Winkler, D. W. (2001). Humoral immunocompetence correlates with date of egg-laying and reflects work load in female tree swallows. Behav. Ecol. 12, 93–97. doi: 10.1093/oxfordjournals.beheco.a000384
Hedenström, A., and Alerstam, T. (1997). Optimum fuel loads in migratory birds: Distinguishing between time and energy minimization. J. Theo. Biol. 189, 227–234. doi: 10.1006/jtbi.1997.0505
Hegemann, A., Alcalde Abril, P., Sjöberg, S., Muheim, R., Alerstam, T., Nilsson, J., et al. (2018a). A mimicked bacterial infection prolongs stopover duration in songbirds – but more pronounced in short- than long-distance migrants. J. Anim. Ecol. 87, 1698–1708. doi: 10.1111/1365-2656.12895
Hegemann, A., Alcalde Abril, P., Muheim, R., Sjöberg, S., Alerstam, T., Nilsson, J., et al. (2018b). Immune function and blood parasite infections impact stopover ecology in passerine birds. Oecologia 188, 1011–1024. doi: 10.1007/s00442-018-4291-3
Hegemann, A., Fudickar, A. M., and Nilsson, J. (2019). A physiological perspective on the ecology and evolution of partial migration. J. Ornithol. 160, 893–905. doi: 10.1007/s10336-019-01648-9
Hegemann, A., Marra, P. P., and Tieleman, B. I. (2015). Causes and consequences of partial migration in a passerine bird. Am. Nat. 186, 531–546. doi: 10.1086/682667
Hegemann, A., Matson, K. D., Both, C., and Tieleman, B. I. (2012). Immune function in a free-living bird varies over the annual cycle, but seasonal patterns differ between years. Oecologia 170, 605–618. doi: 10.1007/s00442-012-2339-3
Hegemann, A., Pardal, S., and Matson, K. D. (2017). Indices of immune function used by ecologists are mostly unaffected by repeated freeze-thaw cycles and methodological deviations. Front. Zool. 14:43. doi: 10.1186/s12983-017-0226-9
Hellgren, O., Waldenstrom, J., and Bensch, S. (2004). A new PCR assay for simultaneous studies of Leucocytozoon, Plasmodium, and Haemoproteus from avian blood. J. Parasitol. 90, 797–802. doi: 10.1645/GE-184R1
Hellgren, O., Waldenstrom, J., Perez-Tris, J., Szollosi, E., Hasselquist, D., Krizanauskiene, A., et al. (2007). Detecting shifts of transmission areas in avian blood parasites - a phylogenetic approach. Mol. Ecol. 16, 1281–1290. doi: 10.1111/j.1365-294X.2007.03277.x
Horrocks, N. P. C., Hegemann, A., Matson, K. D., Hine, K., Jaquier, S., Shobrak, M., et al. (2012). Immune Indexes of Larks from Desert and Temperate Regions Show Weak Associations with Life History but Stronger Links to Environmental Variation in Microbial Abundance. Physiol. Biochem. Zool. 85, 504–515. doi: 10.1086/666988
Horrocks, N. P. C., Hegemann, A., Ostrowski, S., Ndithia, H., Shobrak, M., Williams, J. B., et al. (2015). Environmental proxies of antigen exposure explain variation in immune investment better than indices of pace of life. Oecologia 177, 281–290. doi: 10.1007/s00442-014-3136-y
Huang, X., Jonsson, J., and Bensch, S. (2020). Persistence of avian haemosporidians in the wild: a case study to illustrate seasonal infection patterns in relation to host life stages. Int. J. Parasitol. 50, 611–619. doi: 10.1016/j.ijpara.2020.05.006
Janeway, C. A., Travers, P., Walport, M., and Shlomchik, M. J. (2005). Immunobiology: the Immune System In Health And Disease, 6th Edn. New York: Garland Science.
Klaassen, M., Hoye, B. J., Nolet, B. A., and Buttemer, W. A. (2012). Ecophysiology of avian migration in the face of current global hazards. Philos. Trans. R. Soc. B. 367, 1719–1732. doi: 10.1098/rstb.2012.0008
Lindström, Å (2003). “Fuel deposition rates in migrating birds: Causes, constraints and consequences,” in Avian Migration, eds P. Berthold, E. Gwinner, and E. Sonnenschein (Berlin: Springer), 307–320.
Lindström, Å, and Alerstam, T. (1992). Optimal Fat Loads in Migrating Birds - a Test of the Time-Minimization Hypothesis. Am. Nat. 140, 477–491. doi: 10.1086/285422
Lüdecke, D. (2018). ggeffects: Tidy Data Frames of Marginal Effects from Regression Models. J. Open Source Software 3:772.
Marzal, A., Balbontín, J., Reviriego, M., García-Longoria, L., Relinque, C., Hermosell, I. G., et al. (2016). A longitudinal study of age-related changes in Haemoproteus infection in a passerine bird. Oikos 125, 1092–1099.
Marzal, A., Reviriego, M., Hermosell, I. G., Balbontín, J., Bensch, S., Relinque, C., et al. (2013). Malaria infection and feather growth rate predict reproductive success in house martins. Oecologia 171, 853–861. doi: 10.1007/s00442-012-2444-3
Matson, K., Cohen, A., Klasing, K., Ricklefs, R., and Scheuerlein, A. (2006). No simple answers for ecological immunology: relationships among immune indices at the individual level break down at the species level in waterfowl. Proc. R. Soc. B. 273, 815–822. doi: 10.1098/rspb.2005.3376
Matson, K. D., Horrocks, N. P. C., Versteegh, M. A., and Tieleman, B. I. (2012). Baseline haptoglobin concentrations are repeatable and predictive of certain aspects of a subsequent experimentally-induced inflammatory response. Comp. Biochem. Phys. A. 162, 7–15. doi: 10.1016/j.cbpa.2012.01.010
Matson, K. D., Ricklefs, R. E., and Klasing, K. C. (2005). A hemolysis-hemagglutination assay for characterizing constitutive innate humoral immunity in wild and domestic birds. Dev. Comp. Immunol. 29, 275–286. doi: 10.1016/j.dci.2004.07.006
McWilliams, S., Carter, W., Cooper-Mullin, C., DeMoranville, K., Frawley, A., Pierce, B., et al. (2021). How Birds During Migration Maintain (Oxidative) Balance. Front. Ecol. Evol. 9:742642. doi: 10.3389/fevo.2021.742642
Nebel, S., Bauchinger, U., Buehler, D. M., Langlois, L. A., Boyles, M., Gerson, A. R., et al. (2012). Constitutive immune function in European starlings, Sturnus vulgaris, is decreased immediately after an endurance flight in a wind tunnel. J. Exp. Biol. 215, 272–278. doi: 10.1242/jeb.057885
Nebel, S., Buehler, D. M., MacMillan, A., and Guglielmo, C. G. (2013). Flight performance of western sandpipers, Calidris mauri, remains uncompromised when mounting an acute phase immune response. J. Exp. Biol. 216, 2752–2759. doi: 10.1242/jeb.083204
O’Connor, E. A., Hasselquist, D., Nilsson, J., Westerdahl, H., and Cornwallis, C. K. (2020). Wetter climates select for higher immune gene diversity in resident, but not migratory, songbirds. Proc. R. Soc. B. 287:20192675. doi: 10.1098/rspb.2019.2675
Owen, J. C., Hawley, D. M., and Huyvaert, K. P. (2021). Infectious Disease Ecology of Wild Birds. Oxford:Oxford University Press.
Owen, J. C., and Moore, F. R. (2006). Seasonal differences in immunological condition of three species of thrushes. Condor 108, 389–398.
Owen, J. C., and Moore, F. R. (2008a). Swainson’s thrushes in migratory disposition exhibit reduced immune function. J. Ethol. 26, 383–388. doi: 10.1007/s10164-008-0092-1
Owen, J. C., and Moore, F. R. (2008b). Relationship between energetic condition and indicators of immune function in thrushes during spring migration. Can. J. Zool. 86, 638–647. doi: 10.1139/7-08-038
Packmor, F., Klinner, T., Woodworth, B. K., Eikenaar, C., and Schmaljohann, H. (2020). Stopover departure decisions in songbirds: do long-distance migrants depart earlier and more independently of weather conditions than medium-distance migrants? Movement Ecol. 8:6. doi: 10.1186/s40462-020-0193-1
Piersma, T., and van Gils, J. A. (2011). The Flexible Phenotype: a Body-Centred Integration of Ecology, Physiology, and Behaviour. Oxford: Oxford University Press.
Quaye, I. K. (2008). Haptoglobin, inflammation and disease. Trans. R. Soc. Trop. Med. Hyg. 102, 735–742. doi: 10.1016/j.trstmh.2008.04.010
R Development Core Team (2020). A language and environment for statistical computing.Vienna, Aus: R Foundation for Statistical Computing.
Risely, A., Klaassen, M., and Hoye, B. J. (2018). Migratory animals feel the cost of getting sick: a meta-analysis across species. J. Anim. Ecol. 87, 301–314. doi: 10.1111/1365-2656.12766
Roast, M. J., Aranzamendi, N. H., Fan, M., Teunissen, N., Hall, M. D., and Peters, A. (2020). Fitness outcomes in relation to individual variation in constitutive innate immune function. Proc. R. Soc. B. 287:20201997. doi: 10.1098/rspb.2020.1997
Sambrook, J., Fritch, F. J., and Maniatis, T. (2002). Molecular Cloning, a Labaratory Manual. NewYork: Cold Spring Harbor Laboratory Press.
Schmaljohann, H. (2019). The start of migration correlates with arrival timing, and the total speed of migration increases with migration distance in migratory songbirds: a cross-continental analysis. Mov. Ecol. 7:25. doi: 10.1186/s40462-019-0169-1
Schmaljohann, H., and Eikenaar, C. (2017). How do energy stores and changes in these affect departure decisions by migratory birds? A critical view on stopover ecology studies and some future perspectives. J. Comp. Physiol. A 203, 411–429. doi: 10.1007/s00359-017-1166-8
Sköld-Chiriac, S., Nord, A., Nilsson, J., and Hasselquist, D. (2014). Physiological and Behavioral Responses to an Acute-Phase Response in Zebra Finches: Immediate and Short-Term Effects. Physiol. Biochem. Zool. 87, 288–298. doi: 10.1086/674789
Stambaugh, T., Houdek, B. J., Lombardo, M. P., Thorpe, P. A., and Caldwell Hahn, D. (2011). Innate immune response development in nestling tree swallows. Wilson J. Ornithol. 123, 779–787.
Svensson, L. (1992). Identification Guide To European Passerines, 4th Edn. Thetford:British Trust for Ornithology
Taylor, P., Crewe, T., Mackenzie, S., Lepage, D., Aubry, Y., Crysler, Z., et al. (2017). The Motus Wildlife Tracking System: a collaborative research network to enhance the understanding of wildlife movement. Avian Cons. Ecol. 18:8
van de Pol, M., and Wright, J. (2009). A simple method for distinguishing within- versus between-subject effects using mixed models. Anim. Behav. 77, 753–758. doi: 10.1016/j.anbehav.2008.11.006
van Dijk, J. G., Verhagen, J. H., Hegemann, A., Tolf, C., Olofsson, J., Järhult, J. D., et al. (2020). A Comparative Study of the Innate Humoral Immune Response to Avian Influenza Virus in Wild and Domestic Mallards. Front. Microbiol. 11:3042. doi: 10.3389/fmicb.2020.608274
van Dijk, Jacintha G. B, Hoye, B. J., Verhagen, J. H., Nolet, B. A., Fouchier, R. A. M., and Klaassen, M. (2014). Juveniles and migrants as drivers for seasonal epizootics of avian influenza virus. J. Anim. Ecol. 83, 266–275. doi: 10.1111/1365-2656.12131
Vermeulen, A., Eens, M., Van Dongen, S., and Muller, W. (2017). Does baseline innate immunity change with age? A multi-year study in great tits. Exp. Gerontol. 92, 67–73. doi: 10.1016/j.exger.2017.03.011
Wemer, L., Hegemann, A., Isaksson, C., Nebel, C., Kleindorfer, S., Gamauf, A., et al. (2021). Reduced ectoparasite load, body mass and blood haemolysis in Eurasian kestrels (Falco tinnunculus) along an urban-rural gradient. Naturwissenschaften 108:42. doi: 10.1007/s00114-021-01745-x
Westerdahl, H., Bensch, S., Nilsson, J., Sehgal, R., Tesson, S., and Hasselquist, D. (2014). “Pathogens and hosts on the move,” in Animal Movement Across Scales, eds L. Hansson and S. Åkesson (Oxford:Oxford University Press).
Zuur, A. F., Ieno, E. N., and Elphick, C. S. (2010). A protocol for data exploration to avoid common statistical problems. Methods Ecol. Evol. 1, 3–14.
Keywords: optimal migration theory, ecophysiology, avian migration, stopover ecology, eco-immunology, migratory strategy, avian malaria
Citation: Hegemann A, Birberg C, Hasselquist D and Nilsson J-Å (2022) Early and Late Migrating Avian Individuals Differ in Constitutive Immune Function and Blood Parasite Infections – But Patterns Depend on the Migratory Strategy. Front. Ecol. Evol. 10:880426. doi: 10.3389/fevo.2022.880426
Received: 21 February 2022; Accepted: 20 May 2022;
Published: 13 June 2022.
Edited by:
Jose A. Masero, University of Extremadura, SpainReviewed by:
Rebecca Cole, United States Geological Survey, United StatesAlfonso Marzal, University of Extremadura, Spain
Copyright © 2022 Hegemann, Birberg, Hasselquist and Nilsson. This is an open-access article distributed under the terms of the Creative Commons Attribution License (CC BY). The use, distribution or reproduction in other forums is permitted, provided the original author(s) and the copyright owner(s) are credited and that the original publication in this journal is cited, in accordance with accepted academic practice. No use, distribution or reproduction is permitted which does not comply with these terms.
*Correspondence: Arne Hegemann, YXJuZS5oZWdlbWFubkBiaW9sLmx1LnNl