- 1Department of Zoology and Entomology, University of Pretoria, Pretoria, South Africa
- 2Mammal Research Institute, University of Pretoria, Pretoria, South Africa
Biological rhythms are rhythmic fluctuations of biological functions that occur in almost all organisms and on several time scales. These rhythms are generated endogenously and entail the coordination of physiological and behavioural processes to predictable, external environmental rhythms. The light-dark cycle is usually the most prominent environmental cue to which animals synchronise their rhythms. Biological rhythms are believed to provide an adaptive advantage to organisms. In the present review, we will examine the occurrence of circadian and seasonal rhythms in African mole-rats (family Bathyergidae). African mole-rats are strictly subterranean, they very rarely emerge aboveground and therefore, do not have regular access to environmental light. A key adaptation to their specialised habitat is a reduction in the visual system. Mole-rats exhibit both daily and seasonal rhythmicity in a range of behaviours and physiological variables, albeit to different degrees and with large variability. We review previous research on the entire circadian system of African mole-rats and discuss output rhythms in detail. Laboratory experiments imply that light remains the strongest zeitgeber for entrainment but in the absence of light, animals can entrain to ambient temperature rhythms. Field studies report that rhythmic daily and seasonal behaviour is displayed in their natural habitat. We suggest that ambient temperature and rainfall play an important role in the timing of rhythmic behaviour in mole-rats, and that they likely respond directly to these zeitgebers in the field rather than exhibit robust endogenous rhythms. In the light of climate change, these subterranean animals are buffered from the direct and immediate effects of changes in temperature and rainfall, partly because they do not have robust circadian rhythms, however, on a longer term they are vulnerable to changes in their food sources and dispersal abilities.
Biological Rhythms
Biological timing is measured in the form of cyclical variations in physiological processes and behaviours of organisms. Biological rhythms are ubiquitous in nearly all organisms and range in frequency from milliseconds to many years and at all levels of organisation (Aschoff, 1981; Paranjpe and Sharma, 2005). Biological rhythms can be classified according to the lengths of their periods, ultradian rhythms are shorter than 24 h, circadian rhythms are about 24 h long and rhythms longer than 24 h are called infradian (Wollnik, 1989). These rhythms are frequently superimposed on one-another, and the integrated multi-frequency timekeeping enables organisms to keep track of their environment and promotes optimal performance and survival (Golombek and Rosenstein, 2010; Kuhlman et al., 2018).
Periodicity under natural conditions does not necessarily demonstrate the presence of a biological clock. Rhythms can be purely exogenous, in which case the rhythm is dependent on the external environment (Aschoff, 1960). However, by far the most common rhythms are the endogenous rhythms which are generated by biological clocks within an organism and continue or free run in the absence of external entraining factors, at least for some time. Endogenous biological rhythms frequently do not run precisely over daily or annual periods and a large number of internal and external factors can influence the rhythm lengths, causing them to deviate from that of external environmental rhythms. To be biologically relevant, endogenous rhythms are synchronised or entrained by periodic environmental rhythms to prevent them from drifting out of phase with the environment (Aschoff and Pohl, 1978). Circadian rhythms are the most widespread biological rhythms and have periods of about 24 h. The daily light-dark cycle is the most predictable cyclical environmental cue and is therefore the most prominent zeitgeber used by organisms for entrainment (Amir and Stewart, 1998). Non-photic cues such as temperature, exercise and social cues can also influence biological rhythms, however their effect is usually less potent than that of light (Golombek and Rosenstein, 2010; Refinetti, 2010). However, in nature zeitgebers do not act independently, they have compounding effects to generate a more robust entraining effect (Van Jaarsveld et al., 2019).
The Circadian System
The circadian system can be divided into three fundamental components, (a) the input pathway that collects external timing signals and relays it to the core clock, (b) the central clock that is responsible for the generation of rhythms, and (c) the output in the form or behavioural or physiological rhythms (Agostino et al., 2011). In mammals, photosensitive pigments are found only in the retina of the eye. The rods and cones in the photoreceptor layer of the retina are primarily used for vision, whereas the melanopsins that are present in intrinsically photosensitive retinal ganglion cells (ipRGC) are involved in the circadian system (Berson, 2003). The axons of the ipRGC’s form the retinohypothalamic tract (RHT) which projects to the suprachiasmatic nucleus (SCN) that is located in the basal hypothalamus (Moore, 2007). The SCN is the site of the central pacemaker in mammals (Moore, 1983). The neurons of the SCN respond to photic input in a gated fashion, their activation is blocked during the day but not the night. During the night, SCN neurons are activated to different degrees depending on the time at night when the light source is given, and this activation corresponds to periods when the presence of light can also cause behavioural phase shifts for entrainment (Kornhauser et al., 1992; Oosthuizen et al., 2005). Apart from the timing of light, the circadian system is also differentially affected by the quality of the light in terms of its intensity, duration, and spectrum (Gorman et al., 2003; Duffy and Wright, 2005; Aral et al., 2006; Zubidat et al., 2009, 2010).
General Mechanism of the Circadian Clock
All circadian clocks have a genetic basis and are driven by delayed transcription-translation feedback loops (TTFL) and post translational modifications (Sharma, 2003). The molecular genetics of circadian clocks have been described in several organisms ranging from prokaryotic organisms such as cyanobacteria (Kondo and Ishiura, 2000; Nakajima et al., 2005) to many kinds of eukaryotic organisms including Neurospora (Correa et al., 2003; Dunlap et al., 2007), plants (Gardner et al., 2006), insects (Bargiello and Young, 1984; Williams and Sehgal, 2001; Rosato et al., 2006), and mammals (King and Takahashi, 2000; Reppert and Weaver, 2001; Sato et al., 2004; Ko and Takahashi, 2006). Oscillations of roughly 24 h are generated by the expression, accumulation and degradation of positive and negative clock genes and their products to form loops (Patke et al., 2019). Although the genes involved in the clock mechanisms of different organisms differ, they have a similar functionality in terms of the feedback loops under which they operate. In mammals, two interlocked feedback loops drive the circadian clock at the cellular level (Brown and Doyle, 2020). The core feedback loop, positive element proteins CLOCK and BMAL1 form a dimer and binds to an Ebox region to initiate the transcription of negative element genes Period (Per) and Cryptochrome (Cry). After translation, PERIOD and CRYPTOCHROME form heterodimers and translocate back to the nucleus to act on CLOCK:BMAL1 and suppress their own transcription (Ko and Takahashi, 2006). The second regulatory loop is also activated by CLOCK:BMAL1 acting on a promotor region to activate transcription of the retinoic acid-related orphan nuclear receptors, Rev-erbα and Rorα, which are subsequently translated to their respective proteins. These proteins aid in the regulation of Bmal1 by controlling the rate of its transcription (Ko and Takahashi, 2006). This secondary, or auxiliary loop is thought to stabilise oscillations (Brown and Doyle, 2020). The stability and period of the circadian oscillations are also affected by post translational modifications such as phosphorylation and ubiquitination, which contribute to the stability of the proteins and is involved in nuclear translocation (Ko and Takahashi, 2006).
Nearly all mammalian cells and tissues contain circadian clocks that can generate autonomous circadian rhythms that persist in isolation and free run at their own innate period (Yoo et al., 2004; Husse et al., 2015). Since the central pacemaker in the SCN receives external photic input, it can synchronise to the external environment, and in turn it maintains temporal synchrony of the downstream peripheral clocks with the environment. Circadian clocks are also involved in the regulation of seasonal rhythms via the hormone melatonin. In mammals, pineal melatonin synthesis is under the control of the SCN (Maronde et al., 1999). Melatonin expression peaks at night and is suppressed during the day, and can thus provide photoperiodic information as the daylength varies across seasons (Hardeland et al., 2006). Seasonality is frequently effected by seasonal changes in hormones from the anterior pituitary. The photoperiodic effects of melatonin on endocrine function is mediated by the pars tuberalis of the pituitary gland, a region rich in melatonin receptors (Morgan and Williams, 1996).
Evolution of Rhythmicity and Its Functional Importance
Due to the ubiquitous nature of rhythmicity in prokaryotic and eukaryotic organisms, the evolution of a temporal order is thought to have originated with early life forms (Hastings et al., 1991; Paranjpe and Sharma, 2005). In primitive organisms, rhythmicity serves to segregate photophilic and photophobic processes essential for survival (Stal and Krumbein, 1985; Nikaido and Johnson, 2000). The evolution of homeothermy in early eutherian mammals enabled them to exploit the nocturnal niche (Crompton et al., 1978) and thereby avoid predation and interspecific competition with dinosaurs (Walls, 1942; Gerkema et al., 2013). Other physiological and biochemical rhythms such as body temperature and metabolism usually show corresponding peaks compared to locomotor activity, indicating that internal rhythms are synchronised (Refinetti, 1999; Riccio and Goldman, 2000a). Similar to daily rhythmicity, animals may also show seasonal rhythmicity. Many animals are exposed to annual fluctuations in their environment, and organisms tend to restrict their energetically expensive processes, such as reproduction, to times of the year when food is abundant and other environmental factors are most favourable. Other seasonally timed behaviours include migration, hibernation and colour changes of pelages to blend in with seasonal environmental colouring.
Rhythmicity provides organisms with both intrinsic and extrinsic fitness benefits. The intrinsic adaptive value of circadian rhythms refers to the temporal coordination of internal processes, for example to segregate incompatible processes or synchronise others. Rhythms also allows organisms to keep track of external time, providing an extrinsic adaptive value (Sharma, 2003). Both of these processes are crucial for survival in natural environments (Paranjpe and Sharma, 2005). Rhythmicity enables animals to keep track of proximate factors such as light and temperature in order to predict and prepare for ultimate factors such as predation risks, food availability and mating opportunities, thereby providing an adaptive advantage (Helm et al., 2013).
The importance of the circadian timing system and its entrainment by light-dark cycles is best demonstrated by the prevalence of increased health risks and in some cases disturbances within ecological systems, which emerge from disruptions of the circadian clock network and desynchronization in timing of the different biological rhythms (Bird et al., 2004; Navara and Nelson, 2007; Rotics et al., 2011; Haim and Portnov, 2013). In modern society there are many artificially induced disruptions of the circadian system that have implications for both humans and animals that have received much attention both in the laboratory and more recently also in field studies. Animals are most severely affected by light pollution and human interference with ecosystems (Longcore and Rich, 2004).
When the internal clock mechanisms of organisms are not appropriately aligned with the external environment, many physiological processes are compromised. Effects of this misalignment include reduced longevity and accelerated aging, increased risk of cancer, metabolic, cardiovascular as well as reproductive disorders and immune dysfunction (Evans and Davidson, 2013). Laboratory studies indicate that alterations to the LD cycle increase mortality of animals (Halberg and Cadotte, 1975; Penev et al., 1998; Davidson et al., 2006; Vinogradova et al., 2009). In a natural habitat, animals with ablated SCNs have a higher mortality as a result of increased predation (DeCoursey et al., 1997; DeCoursey and Krulas, 1998). Artificial light at night (ALAN) contributes to a higher prevalence of several forms of cancer, accelerates tumour growth and increases oxidative stress (Dauchy et al., 1999; Vinogradova et al., 2009), with the disruption of the melatonin rhythm thought to play a crucial role (Shah et al., 1984; Blask and Hill, 1986; Reiter et al., 2000; Baydas et al., 2001; Schernhammer and Schulmeister, 2004). Circadian disruption can lead to a host of adverse metabolic effects such as increased weight gain, obesity and glucose intolerance which may result from altered feeding behaviours (Oishi, 2009; Karatsoreos et al., 2011; Varcoe et al., 2011), and an increased risk of cardiovascular disorders (Knutsson and Boggild, 2000; Ruger and Scheer, 2009). The immune system may also be adversely affected by circadian disruptions, with infections and inflammations arising that can act synergistically with other health consequences that are associated with disturbances in rhythmicity (Scheiermann et al., 2013; Philips et al., 2015; Comas et al., 2017). External disturbances of the circadian system appear to have overall negative effects on organisms.
The changing climates also cause shifts in seasons, which can have serious implications for organisms as their phenological environments are altered (Visser et al., 2010). Animals are adapted to certain environmental factors in their habitats, and they time processes such as reproduction, hibernation and migration accordingly. When seasonal changes occur at times that are different from those animals anticipate and prepare for, mismatches and mistiming occur between the animal and its environment. This effect often spans several trophic levels, for example when shifts in food sources occur there is a disruption in the food chain (Visser et al., 2006). This in turn can have direct implications on the reproduction of animals (Visser et al., 2009). Phenological mismatches in migrating animals can also have severe fitness consequences (Saino et al., 2010), and similarly a recent publication described a phenological mismatch between sexes in a hibernating rodent following a heatwave (Kucheravy et al., 2021). In species that undergo seasonal pelage colour changes, temperature shifts can cause snow to arrive earlier or melt sooner, causing a mismatch between the animal and its environment (Mills et al., 2013). Proper timing of biological events is crucial for the continued survival and fitness of species.
Rhythmicity in Constant Habitats
Most organisms inhabit highly rhythmic environments where daylight and temperature fluctuate daily or seasonally. There are, however, certain habitats that experience minimal daily and seasonal fluctuations in ambient conditions. While the significance of rhythmicity may be clear-cut for animals that are frequently exposed to environmental fluctuations, it is less obvious for animals that inhabit relatively constant environments. In the absence of rhythmic external cues some organisms may not benefit from entraining their internal clocks to regulate rhythmic behaviour that might be deemed to be less advantageous. In such conditions, rhythmicity may be actively selected against, in particular to preclude the maintenance of energetically expensive input pathways (eyes) and clock mechanisms (Niven and Laughlin, 2008; Porter and Sumner-Rooney, 2018). There is indeed evidence of regression in species that utilise constant habitats, with a gradient of morphological adaptations dependent on the extent of habitat utilisation. Some organisms return to a rhythmic environment periodically and could therefore show lower degrees of adaptation to constant environments. Specifically with regards to vision, adaptations range from animals with slightly reduced eyes to others that are completely eyeless, with all degrees of variation in between (Menna-Barreto and Trajano, 2015). Since photic information reaches the SCN via the eyes in mammals, a regressed visual system has implications for the circadian system. Species that utilise constant habitats but are morphologically more similar to species that do not, i.e., possessing acute vision and functional eyes, should display distinct rhythms, whereas eyeless species with absent visual structures should not express circadian rhythmicity, and intermediate species probably exhibit a mosaic of characteristics.
Polar regions are by no means subject to stable environmental conditions since they are subject to large seasonal fluctuations in both temperature and photoperiod. However, changes in photoperiod are so extreme that during the middle of the summer the sun does not set and in winter it does not rise (Bloch et al., 2013). Animals are thus exposed to periods of constant light or dark during mid-summer and winter, during which mammals inhabiting these regions cannot use light to entrain their biological rhythms, resulting in significant variation in rhythmicity amongst animals. Some animals lose rhythmicity completely during this time and may become almost constantly active (Swade and Pittendrigh, 1967; Reierth et al., 1999; Van Oort et al., 2005; Lu et al., 2010), some animals lose rhythmicity intermittently (Arnold et al., 2018), while others retain rhythmicity, but at a lower amplitude (Swade and Pittendrigh, 1967; Hau et al., 2002; Ashley et al., 2014; Ware et al., 2020). Polar animals are still exposed to rhythmic environmental conditions for the remainder of the year, during which they exhibit rhythmicity in behaviour and physiological functions.
More than 60% of the Earth’s surface is covered by deep oceans, and deep seas of more than 200 m have long been considered as arrhythmic environments (Mat et al., 2020). Although water temperatures do not show daily variations within a few meters from the surface (Kawai and Wada, 2007), seasonal differences are still noticeable for depths of up to 1 km (Talley, 2011). Light can also penetrate water for up to 1 km (Warrant and Locket, 2004). Internal tides as a result of hydrothermal vents occur at all depths (Mat et al., 2020). Although this biome is poorly studied due to its inaccessibility and technical constraints, rhythmic behaviour has been observed in deep sea organisms. In deep sea organisms that have been brought to the surface, circadian feeding rhythms (Maynou and Cartes, 1988; Modica et al., 2014) and lunar rhythms in melatonin secretion (Wagner et al., 2007) have been detected. Video recordings of deep-sea invertebrates and mussels in their natural environment also reveal circadian rhythmicity in their activity and behaviour (Cuvelier et al., 2014; Mat et al., 2020). Functional melanopsin receptors have been identified in some deep-sea fish, suggesting the potential for photic stimulation of the circadian clock (Davies et al., 2012). Candidate clock genes have also been discovered (Mat et al., 2020), indicating that the circadian clocks of these animals are still functional.
Caves probably have the most stable microclimates of all arrhythmic habitats, as they are completely isolated from light and temperature fluctuations (Beale et al., 2016). Cave dwelling organisms show large variation in their use of the caves as well as in the expression of their biological rhythms. Many species, such as bats, use caves to sleep in, but forage outside, and have robust circadian rhythms (Marimuthu and Chandrashekaran, 1985). Some other organisms are facultative cave dwellers and are strongly specialised to their habitat, with some species even regressing their eyes completely. Despite this, many of these organisms have retained the ability to display circadian rhythms, albeit dampened and with evidence for clock gene mutations, and they do not persist long in constant conditions (Cavallari et al., 2011; Beale et al., 2013, 2016).
The subterranean niche is characterised by constant darkness and otherwise dampened short term climatic fluctuations (Bennett and Faulkes, 2000). A large number of vertebrates, including some 300 mammalian species from different taxa, have evolved fossorial or subterranean lifestyles (Nevo, 1979; Bennett and Faulkes, 2000; Lacey et al., 2001; Peichl et al., 2004). As with cave dwelling species, fossorial species range from species using burrows purely for shelter to others that permanently live underground and surface very infrequently, if at all (Nevo, 1979; Mason and Narins, 2001). Technically, animals that use underground tunnels to sleep in, but forage aboveground are classified as fossorial (Camin and Madoery, 1994; Tomotani et al., 2012). However, since they are frequently exposed to daily environmental light and temperature fluctuations, they do not permanently live in a constant environment and usually display robust rhythmicity.
Many species have evolved to show specific adaptations to a life underground. These adaptations include both morphological and physiological features, including adaptations to the visual system. Eye sizes vary considerably in subterranean animals, with this variation reflecting differences in selection pressures of the various lifestyles (Borghi et al., 2002). Strictly subterranean mammals that very rarely emerge aboveground frequently have reduced or no visual capabilities. These animals can have microphthalmic external eyes, subcutaneous eyes or a complete loss of eyes (Nevo, 1979; Burda et al., 1990; Cooper et al., 1993a). Animals with small external or subcutaneous eyes usually have regressed or malformed retinal structures, and brain areas that are associated with vision are reduced or absent, however, their circadian systems appear to be intact (Cooper et al., 1993a; Peichl, 2005; Němec et al., 2008a,b; Vega-Zuniga et al., 2017).
Mole-Rats
African mole-rats from the family Bathyergidae are endemic to sub-Saharan Africa, and all species are subterranean. There are six genera within this family, and a total of around 30 described species, three of the genera contain solitary species and three social species (Figure 1; Van Daele et al., 2007; Faulkes and Bennett, 2013). Solitary species are confined to mesic habitats, whereas social species occur throughout much of the continent south of the Sahel.
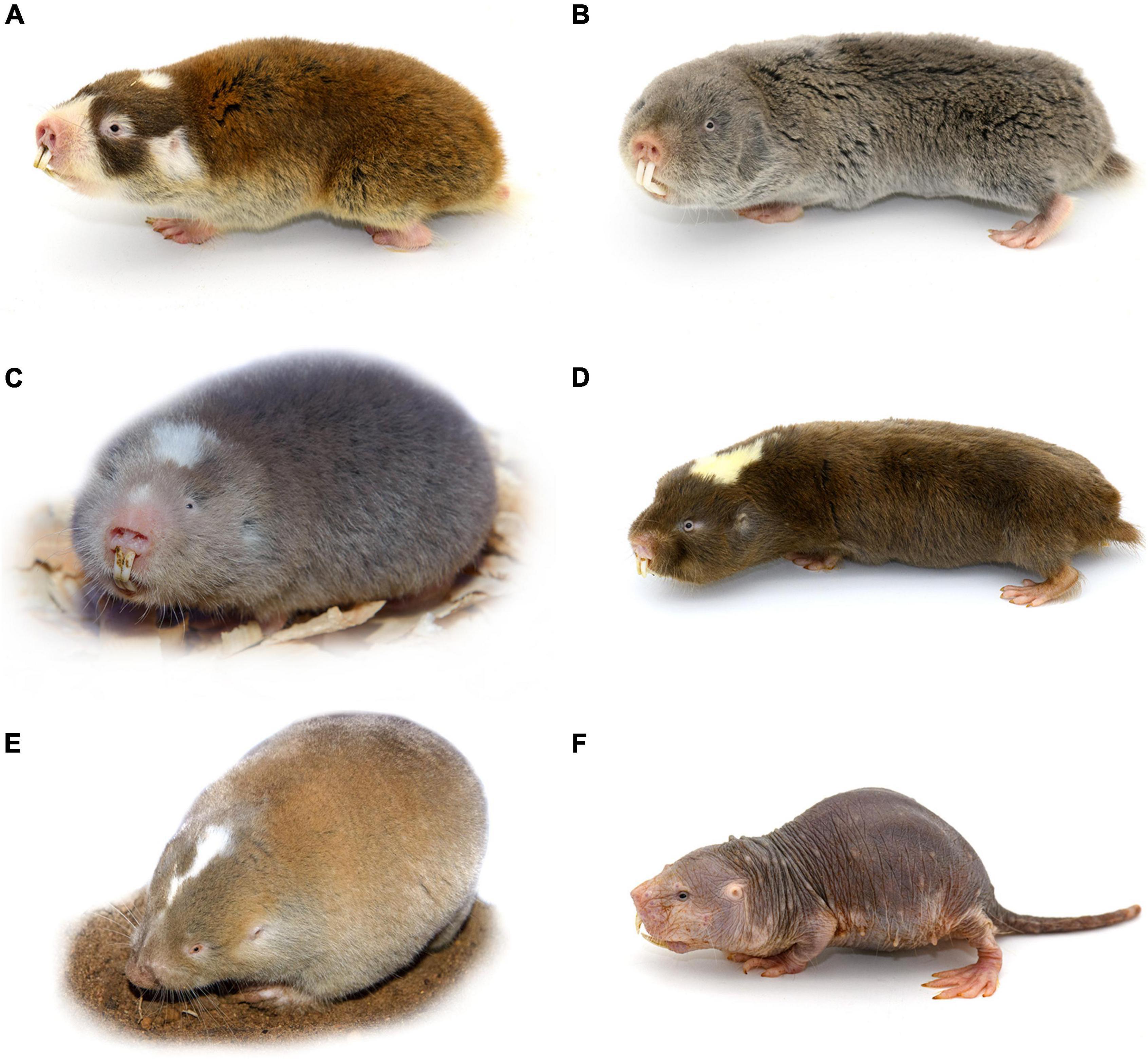
Figure 1. Images of a representative animal of each of the six genera of the family Bathyergidae. (A) Georychus capensis; (B) Cryptomys hottentotus pretoriae; (C) Heliophobius emeni; (D) Fukomys damarensis; (E) Bathyergus suillus; (F) Heterocephalus glaber. Panels (A,C,E) are solitary species, while panels (B,D,F) represent social species.
Mole-rats are believed to have evolved from surface-dwelling to a subterranean existence about 20 million years ago, in the early Miocene (Nevo, 1995; Lavocat, 2013). They have since adapted very successfully to their habitat and show several morphological and physiological adaptations to their environment. These include a regressed visual system which can pose obvious challenges for the circadian system and rhythmicity. Despite their reduced visual system, all mole-rat species investigated to date show some form of rhythmicity, albeit to varying degrees. This suggests that their circadian systems are intact and functional.
Naked mole-rats (Heterocephalus glaber) are of particular interest for a variety of medical applications including aging, cancer and pain research and have therefore been the focus of many diverse studies over the past decades (Buffenstein, 2005; Park et al., 2008; Shepard and Kilssil, 2020). Hence, much of the research on mole-rats, specifically the molecular aspects of the circadian clock and its output, were conducted on this species. More recently, there was a spike in interest also in the other mole-rat species in the family Bathyergidae for comparative research in an evolutionary and ecological sense. This family occurs in a wide range of habitats, and exhibits a continuum of sociality, ranging from strictly solitary (Bathyergus, Georychus, and Heliophobius) to social (Cryptomys) and highly social (Fukomys and Heterocephalus). In addition, the species in this family show interesting thermoregulatory properties, some species are homeothermic, some more heterothermic and the naked mole-rat has been described as poikilothermic (Buffenstein and Yahav, 1991; Bennett et al., 1993, 1994b; Boyles et al., 2012; Oosthuizen et al., 2021). Finally, the circadian biology of African mole-rats has received much attention over the past two decades.
Mole-Rat Circadian System
Input Pathways
As with other mammals, photic information can only enter the circadian system the via eyes in mole-rats (Němec et al., 2004; Crish et al., 2006). African mole-rats have small superficial eyes, and initially it was suggested that mole-rats were not completely blind (Sclater, 1900). Most researchers, however, were in agreement that mole-rats cannot see Eloff (1958); Jarvis (1973). Eloff (1958) reported that the eyes of mole-rats (Cryptomys and Bathyergus species) are insensitive to light and supported this by the apparent lack of response to bright light being applied to the eye, the lack of pupillary reflex and certain anatomical deviations of the visual system, such as a thin optic tract and the apparent absence of circular muscle fibres around the lens. A thickened cornea was also noted by Eloff (1958). The general consensus amongst researchers studying bathyergids was that they are blind (Peichl et al., 2004), and several, more recent publications referred to ‘blind’ African mole-rats (Lovegrove et al., 1993; Kössl et al., 1996; Brückmann and Burda, 1997; Oelschlager et al., 2000; Heth et al., 2002). The eyes and visual system of mole-rats have subsequently been investigated in more detail. Although microphthalmic, the architecture of mole-rat eyes is similar to that of surface-dwelling rodents (Peichl et al., 2004; Němec et al., 2008a). Although considerable species variation is obvious, the structural organization seems to be less regular (Nikitina et al., 2004; Peichl et al., 2004). The eye lens is small in most species, except the naked mole-rat, where it is very large, uneven in shape and free floating (Nikitina et al., 2004). The retina is well developed, although in the naked mole-rat, it appears to be folded (Nikitina et al., 2004; Němec et al., 2008a). Structurally, it has all the layers expected in a sighted animal, with rod and cone photoreceptor cells, and multiple types of horizontal, bipolar, amacrine and ganglion cells (Mills and Catania, 2004; Peichl et al., 2004). As a result of the small eye size, mole-rats have low overall numbers of photoreceptors, but like most other mammals, the retina is rod dominated. Nevertheless, a surprisingly high proportion of cones are present, most of which are S-opsin immunoreactive (Peichl et al., 2004). The structural organisation of the eye negates the ability for image formation, but that of the retina suggests that these animals retained the ability to discriminate between light and dark (Nikitina et al., 2004). Indeed, behavioural studies in several mole-rat species revealed severe visual deficits in terms of image formation, visuomotor integration and depth perception (Kott et al., 2016).
In addition to structural constraints, certain genes in the naked mole-rat eye display substitutions that constitute retinal degeneration (Zhou et al., 2020). These genes include CRX, a photoreceptor specific transcription factor, and mutations that are associated with dominant rod-cone dystrophy and Leber’s congenital amaurosis (Sohocki et al., 1998), and RPE65 whose encoded protein is part of the vitamin A cycle of the retina, mutations cause retinal degeneration and is also associated with Leber’s congenital amaurosis (Wang et al., 2020). Both of these genes have been implicated with the maintenance of circadian function (Gamse et al., 2001; Doyle et al., 2008). This is consistent with the poor vision displayed by the naked mole-rat, and likely other mole-rats as well.
Retinal ganglion cells are fewer in number, the optic tract is thin and contains more unmyelinated fibres compared to surface dwelling rodents such as mice and rats (Supplementary Figure 1; Omlin, 1997; Negroni et al., 2003; Němec et al., 2004, 2008a). The optic tract projects to all the usual visual structures in the brain, but compared to surface dwelling rodents, both the brain structure sizes, and proportions of the innervations differ. The SCN is well developed and receives dense bilateral projections, proportionally larger compared to surface dwelling rodents, whereas all other visual structures are reduced and receives proportionally smaller projections (Negroni et al., 2003). Light reaches retinorecipient regions of the brain, as indicated by immunohistochemical labelling (Oelschlager et al., 2000). Structures involved in brightness discrimination are better developed than those that regulate coordination of visuomotor reflexes, as is also indicated by the structure of the eye (Peichl et al., 2004; Němec et al., 2008a). Correspondingly mole-rats have low visual acuity, reflecting their reliance on other sensory senses in their habitat.
The circadian system of the blind mole-rat, Spalax ehrenbergi, a subterranean rodent from another family, has been much more extensively studied. It shows a more severe regression of the visual system, with subcutaneous eyes, a complete loss of vision and corresponding small or absent retinal projections to visual structures, as in African mole-rats (Bronchti et al., 1991; Cooper et al., 1993b). The blind mole-rat have less than a thousand retinal ganglion cells, almost all of which contain melanopsin, suggesting a functional circadian system (Hannibal et al., 2002; Esquiva et al., 2016). The mole-rat visual system suggests selective progressive and regressive evolution of the structural attributes, driven by selection forces of their subterranean habitat.
The Clock and Its Mechanism
In mole-rats, as with other mammals, the central pacemaker is located in the SCN and receives bilateral innervation from a subset of retinal ganglion cells (Němec et al., 2008a). Light sensitivity of the SCN has been tested in several mole-rat species by measuring the expression of the immediate early gene c-fos, which is a marker of light activated neuronal activity in the SCN (Oosthuizen et al., 2005). The SCN neurons appear to react to light in most of the species tested, however, the response is variable (Oosthuizen et al., 2005, 2010a). In rodents, Fos expression is dependent on the phase of the circadian clock, with low Fos induction during the day and higher during the night (Rose et al., 1999). This is also the case in the Cape mole-rat (Georychus capensis), a solitary species, whereas gating according to the phase of the day is not apparent in the highveld mole-rat (Cryptomys hottentotus pretoriae), a social species (Oosthuizen et al., 2005). Fos expression in the SCN of rodents is directly proportional to the number of photons in the light stimulus. Increasing light intensities or longer light pulse durations evokes higher Fos expression in the SCN (Nelson and Takahashi, 1991; Dkhissi-Benyahya et al., 2000). The solitary Cape mole-rat exhibits responses similar to other aboveground rodents, light induces a significant Fos response in the SCN that increases with increasing light intensities (Oosthuizen et al., 2010a). In social species, this response is rather different, large variability is obvious in the common mole-rat (Cryptomys hottentotus hottentotus) and the Damaraland mole-rat (Fukomys damarensis), both within and between the groups exposed to specific light intensities. The naked mole-rat does not appear to display any Fos expression in response to light illumination (Oosthuizen et al., 2010a). The lack of responsiveness of the naked mole-rat may be in part as a consequence of the size of their eyes. Naked mole-rats are one of the smallest mole-rats, as are their eye sizes. The small size of the eyes limits the number of photoreceptors and the amount of light that can enter. There appears to be a gradient of light sensitivity in the African mole-rats in parallel with their social structures, with solitary species more sensitive to light compared to the more social species (Figure 2).
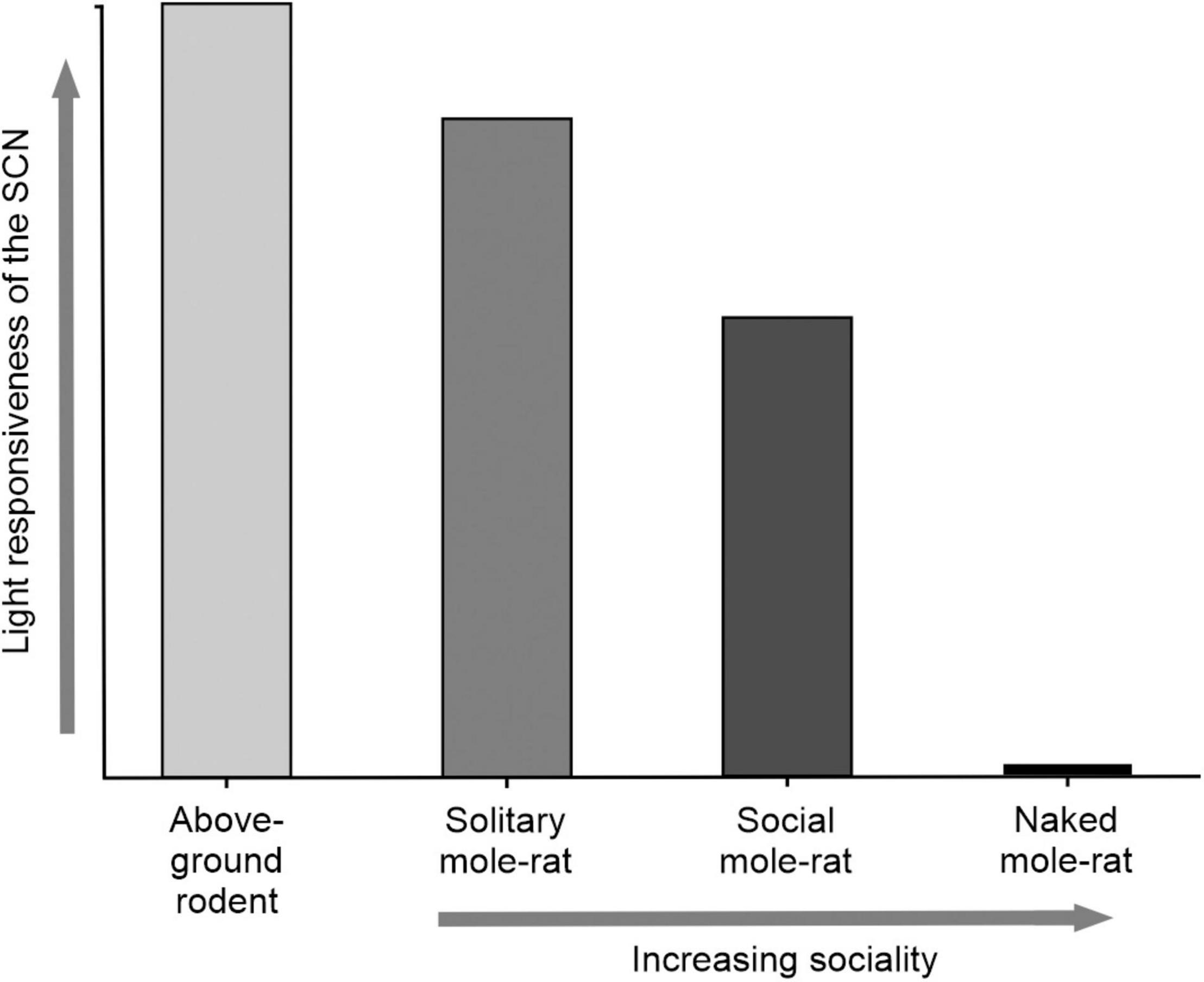
Figure 2. An illustration of the light responsiveness of the SCN comparing aboveground rodents to mole-rats with different degrees of sociality. Aboveground dwelling rodents have a short integration time of light to the SCN and show gating of the circadian clock. Solitary mole-rats show similar gating of light compared to aboveground dwelling rodents but have a longer integration time/higher threshold to light. Social mole-rats do not show gating of the circadian clock and have higher and more variable thresholds to light. Naked mole-rats do not exhibit any Fos expression in the SCN in response to light.
Of the African mole-rats, only the naked mole-rat circadian clock mechanism has been investigated. In addition, it was studied in liver tissue, not the SCN, and patterns observed in the liver could therefore be different from that in the SCN. Ghosh et al. (2021) compared several aspects of the circadian clock between naked mole-rats and mice. All the core circadian clock genes (Bmal1, Clock, Per1/2, Cry1/2) as well as the nuclear receptor genes, Rev-Erbα and β, and three Ror-s, were identified in the naked mole-rat. In addition, some cytoplasmic Casein kinases were also detected in the naked mole-rat genome (Ghosh et al., 2021). The circadian clock proteins appear to be highly conserved among mammalian species. The presence of the appropriate clock genes does not necessarily guarantee robust rhythmicity. To produce and coordinate overt rhythms, different clock gene expression peak at different times of the day. Positive clock genes (Bmal1) normally peak in the morning whereas the negative clock genes (Per and Cry) peak in the evening according to when certain genes are suppressed or not. In the naked mole-rat, the positive and negative clock genes all peak at the same time in the early morning. These differences may potentially stem from variations in the promotors of clock genes. While the regulatory elements of the promotor regions are highly conserved between species, the number of promotors for specific genes differ between mice and mole-rats and could affect the secondary feedback loops (Ghosh et al., 2021). These results could suggest that the naked mole-rat circadian oscillator is organised differently to that of surface-dwelling rodents, or that evolutionary pressures are driving a loss of circadian function in this species.
The clock genes of the blind mole-rat are much better characterised than those of the bathyergid mole-rats. The two positive element genes Clock and Mop3 (Bmal1) have been cloned and sequenced, MOP3 expression shows circadian variation but not Clock. The CLOCK/MOP3 dimer appears relatively conserved but less effective at driving transcription than other mammalian species (Avivi et al., 2001). Three period genes have also been cloned and sequenced, and oscillate with 24 h periodicity in the SCN, retina and peripheral clocks (Avivi et al., 2002). Given the similarity in habitat and selection pressures on blind mole-rats and African mole-rats, it is likely that the other bathyergid mole-rats would also show conserved, functional clock components, although this remains to be discovered.
Output
The output of the circadian clock is manifested in the countless biochemical, physiological and behavioural rhythms displayed by animals. Output rhythms are frequently the only way to determine the phase of the circadian clock. In African mole-rats, several circadian rhythms, including locomotor activity, body temperature, melatonin and metabolism, have been investigated. Locomotor activity received the most attention while other rhythms are rather poorly studied in only a few species.
Daily Rhythmicity in Mole-Rats
Melatonin
In mammals, melatonin is primarily produced in the pineal gland and is involved in the regulation of the sleep-wake rhythm and body temperature (Cagnacci et al., 1992). The naked mole-rat melatonin signal appears to be disrupted at several steps along its pathway. The pineal gland is atrophied (Quay, 1981) and although the genes involved in the synthesis of melatonin are intact, the expression of some of the genes are very low or undetectable (Kim et al., 2011). In addition, the melatonin receptors have mutations that introduce premature stop signals (Fang et al., 2014). In rodents, melatonin has two high-affinity receptors, MTNR1a and MTNR1b. In naked mole-rats, both of these receptors are non-functional, whereas the Damaraland mole-rat has an intact MTNR1a receptor while the MTNR1b is inactive (Fang et al., 2014). The MTNR1a receptor alone has been shown to be sufficient to maintain photoperiodic responses in the hamster (Prendergast, 2010), suggesting a functional melatonin pathway for at least some of the mole-rat species. Indeed, several species from the genus Fukomys, the Damaraland (F. damarensis) and Mashona mole-rats (Fukomys darlingi), as well as the genus Cryptomys, the highveld (C.h. pretoriae) and Natal mole-rats (C.h. natalensis), display daily rhythms of plasma melatonin concentrations (Richter et al., 2003; Gutjahr et al., 2004; Hart et al., 2004; Vasicek et al., 2005a). Plasma melatonin rhythms have apparently not been investigated in the naked mole-rat, probably because of the evidence of interruption in the pathway.
Metabolism
It appears that rhythms of metabolism have only been examined in the naked mole-rat. Genes involved in the metabolism of glucose and fructose were investigated in mice and naked mole-rats. For both pathways, the mole-rat genes show circadian rhythmicity and appear to be more synchronised than mouse genes (Ghosh et al., 2021). Similarly, the mTOR signalling pathway, that senses the cellular environments and a major regulator of mammalian metabolism and physiology, displays highly synchronised expression in the naked mole-rat, but not in mice (Ghosh et al., 2021). Consistent with this, circadian rhythms of metabolic rate were measured in the naked mole-rat, which increases corresponding to increased body temperature and wheel running activity (Riccio and Goldman, 2000a). Stringent control over metabolic pathways may be essential for the survival in challenging subterranean environments where food may be limited and/or energetically expensive to retrieve. Since the naked mole-rats and other African mole-rats share similar habitat constraints, this is likely also the case in the other genera.
Body Temperature
Most mammals show daily variations in body temperature (Tb), which is usually closely related to locomotor activity, Tb is higher when animals are active (Refinetti, 1999). This is also the case in mole-rats (Figure 3). The Tb rhythms of several species have been investigated in the laboratory and in the field, and all of those species show rhythmic Tb fluctuations (Table 1; Lovegrove and Muir, 1996; Riccio and Goldman, 2000a; Streicher et al., 2011; Haupt et al., 2017; Van Jaarsveld et al., 2019; Okrouhlík et al., 2021; Oosthuizen et al., 2021). Mole-rats, like many other fossorial rodents, overall have lower Tb compared to aboveground dwelling rodents (Fioretti et al., 1974; Wollnik and Schmidt, 1995; Castillo et al., 2005; Gordon, 2017). Solitary species have mean Tb of around 35.5–36°C (Lovegrove and Muir, 1996; Okrouhlík et al., 2021) whereas the social species have even lower Tb’s of between 34°C and 35°C (Riccio and Goldman, 2000a; Streicher et al., 2011; Haupt et al., 2017; Oosthuizen et al., 2021). The amplitude of Tb rhythms in mole-rats is also smaller compared to other rodents (Castillo et al., 2005; Haupt et al., 2017; Okrouhlík et al., 2021; Oosthuizen et al., 2021). Both the lower body temperatures and rhythm amplitudes are likely thermoregulatory adaptations to the subterranean ecotope (Lovegrove, 1986). Nevertheless, Tb patterns conformed to that of the activity rhythms in mole-rats that have been investigated in the laboratory (Lovegrove and Muir, 1996; Riccio and Goldman, 2000a; Haupt et al., 2017; Van Jaarsveld et al., 2019). Hence, Tb can be used as a proxy for activity when the measurement thereof is challenging, for example in underground dwelling animals.
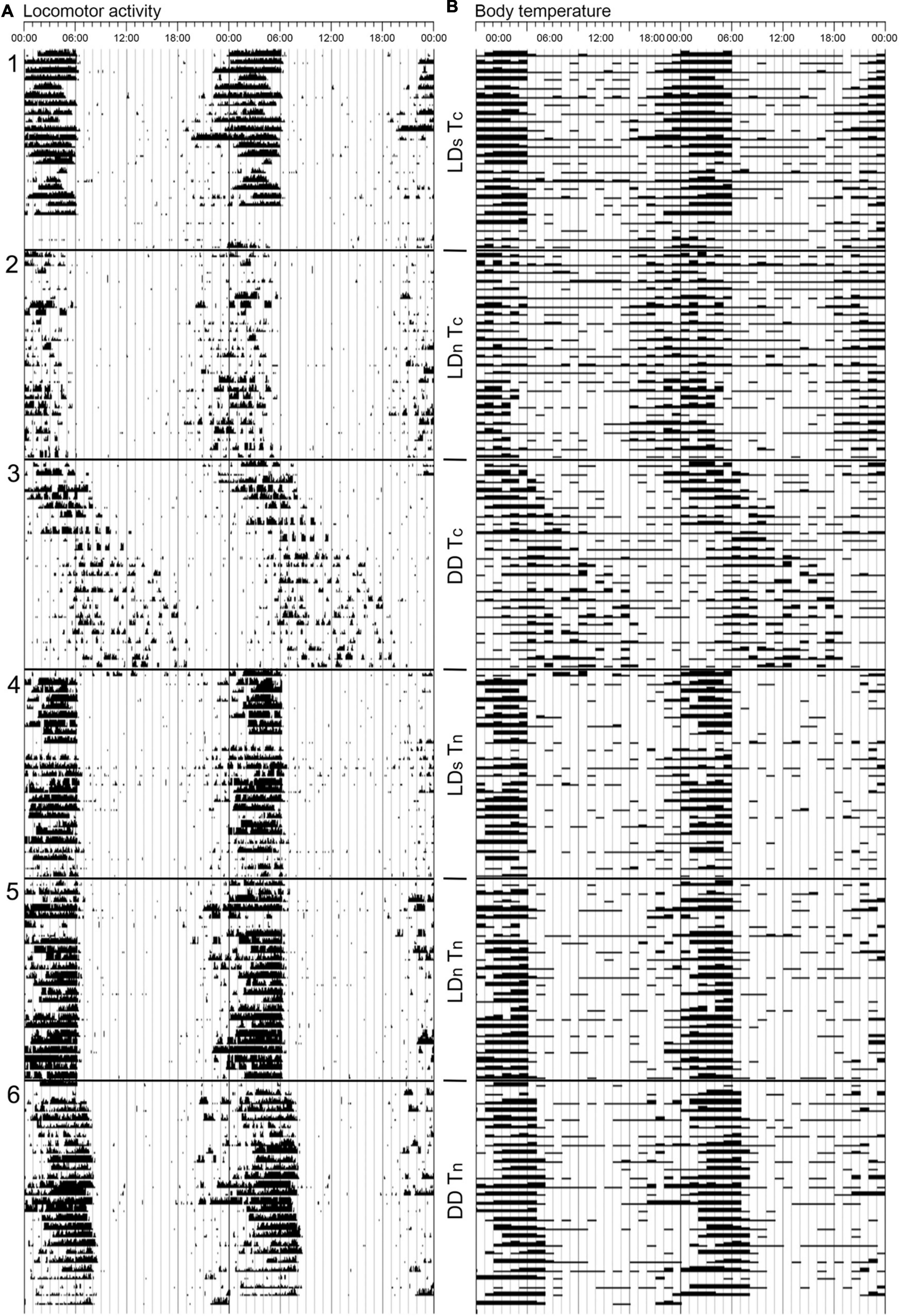
Figure 3. An example of actograms for (A) locomotor activity and (B) body temperature of a Mahali mole-rat (Cryptomys hottentotus mahali) that was subjected to six consecutive light and temperature regimes. The light cycle order was a square wave cycle (12L:12D), simulated dawn and dusk natural cycle (1 h each, 12 h complete darkness) and DD, repeated twice (cycles 1–3, and 4–6). The first three cycles (1–3) had a constant ambient temperature of 25°C, and the last three (4–6) were subjected to a temperature cycle varying between 18 and 28°C over 24 h. The actograms show the close relationship between locomotor activity and body temperature. Cycles 4–6 show more robust rhythmicity, implying the supportive role of ambient temperature to entrainment. A comparison between the DD cycles shows free-running under constant ambient temperature (cycle 3) while activity remains entrained but slightly shifted with a temperature cycle (cycle 6). Actograms are double plotted with the time on the X-axis. The number of days is on the Y-axis and the experimental conditions are illustrated between the two actograms. LDs, square wave; LDn, natural wave; Tc, constant ambient temperature; Tn, natural ambient temperature cycle. (Modified from Van Jaarsveld et al., 2019, with permission from Elsevier).
Locomotor Activity
Much of the early literature claims that there is no locomotor rhythmicity in mole-rats, with initial behavioural studies reporting dispersed activity patterns for both solitary and social species (Table 1; Genelly, 1965; Jarvis, 1973; Hickman, 1980; Lovegrove, 1988; Bennett, 1992). Interest in the circadian biology of African mole-rats started to gain traction in the 1990s and Lovegrove was the first to show evidence of locomotor activity rhythms in both a solitary (Lovegrove and Papenfus, 1995) and a social mole-rat species (Lovegrove et al., 1993). Subsequently, locomotor activity rhythms have been investigated in several species in the laboratory, and all species exhibited rhythmicity, albeit to different degrees and with a great deal of variability both within and between species (Figure 3 and Table 1; Riccio and Goldman, 2000b; Oosthuizen et al., 2003; Hart et al., 2004; Vasicek et al., 2005b; Schöttner et al., 2006; de Vries et al., 2008). Intraspecific variability is evident from the proportion of rhythmic and arrhythmic chronotypes in mole-rat species. Overall, there are many animals that are arrhythmic. Differences in sleep has been identified between rhythmic and arrhythmic animals, arrhythmic animals spend a larger proportion of their time awake compared to rhythmic individuals (Bhagwandin et al., 2011b). These differences are also reflected in the numbers of orexinergic cells present in the animals. Orexin promotes wakefulness, and indeed, the arrhythmic animals of several mole-rat species display more orexinergic cell bodies compared to rhythmic chronotypes (Bhagwandin et al., 2011a).
Potential sources of variation in locomotor activity studies in the laboratory include the method of recording, the housing conditions of the animals and the ambient conditions. Locomotor activity can be measured in several different ways, for single animals, passive infrared captors are quite popular, they work reasonably well, but inter- and intra-experimental variation can occur if the sensitivity of the sensors is different. Running wheels can also be used for singly housed animals, and usually render the ‘cleanest’ results, but have the disadvantage that general activities when the animal is not on the wheel, are not recorded. In addition, not all animals run on wheels. Another option is the use of implanted e-mitters, although these devices are quite expensive and record every movement of the animal, it can render rather noisy results. The recording activity of multiple animals while maintained in a colony is tricky, video recordings can be used if the animals are individually identifiable from above, but it takes very long to analyse subsequently. Alternatively, RFID tags can be implanted in the animals and tag readers can be placed strategically over a laboratory tunnel system. These tags and readers are also costly and do not provide a continuous activity feed.
Housing conditions can also potentially affect activity of animals, firstly the actual housing containers mole-rats are frequently maintained in are square box containers while they live in tunnels in their natural habitat. In addition, social animals are frequently housed in isolation to record locomotor activity of a single animal as a result of recording constraints mentioned above. Social entrainment of rhythms has been implicated for social mole-rat species (Šklíba et al., 2014), therefore rhythmicity of animals may differ according to whether they are isolated or in their natal colonies.
The ambient conditions in the laboratories can also influence activity rhythms of animals. Light is the primary cue for circadian rhythmicity in most animals, and mole-rats are fully capable of perceiving it. In the Highveld mole-rat, C.h. pretoriae, it has been shown that these animals are sensitive to the intensity of light, activity is masked by light at higher intensities (above 10 lux), and animals show higher levels of activity at lower light intensities (below 10 lux), although the temporal profile remains relatively similar. Ambient temperature also affects the level of activity, with mole-rats being less active at higher temperatures that approaches the thermoneutral zone (around 30°C), and more active at cooler temperatures (Oosthuizen and Bennett, 2015; Haupt et al., 2017).
Laboratory vs. Field
The results of several field studies indicate that many mole-rat species have distinct peaks in their daily activity (Šklíba et al., 2007; Streicher et al., 2011; Okrouhlík et al., 2021; Oosthuizen et al., 2021; Finn et al., 2022). These peaks appear to be related to the burrow temperatures, which is not surprising as temperatures in the burrows fluctuate daily and seasonally (Bennett et al., 1988; Šklíba et al., 2007; Lövy et al., 2013). Whether these rhythms are in fact circadian or merely in response to thermoregulatory challenges would require further investigation. Most laboratory studies include light in the testing regimes, and although mole-rats can perceive light this is not a natural situation for them. In their natural habitat, mole-rats are not exposed to light on a regular basis, but they are subjected to slight temperature fluctuations in their burrows. It is therefore to be expected that mole-rats would be more sensitive to temperature changes and also be able to entrain to rhythmic temperature cycles. Entrainment to temperature cycles has in fact been tested in the laboratory, it seems that light still overrides temperature as a zeitgeber. When both light and temperature cycles are presented, temperature cues play a supportive role but if light is not present, animals entrain their activity to temperature cues (Figure 3; Van Jaarsveld et al., 2019; Hart et al., 2021b).
Interestingly, early studies found no difference between laboratory and field activity in mole-rats (Jarvis, 1973; Hickman, 1980) but noted that the type of activity in the two environments may differ. More recent studies under more controlled and stringent laboratory conditions, and thus more unnatural set-ups, may result in the larger differences observed between laboratory and field rhythmicity.
Diurnality vs. Nocturnality
Most animals are adapted to a specific temporal niche and arrange their physiology and behaviour accordingly to display diurnal, nocturnal or crepuscular rhythms of activity. From an evolutionary perspective, rodents are thought to share a nocturnal ancestor, and indeed the majority of modern rodents are nocturnal, while diurnality has evolved secondarily in the order Rodentia (Roll et al., 2006). Mole-rats are no exception and many display distinct activity rhythms that correspond with day or night. However, the activity patterns displayed by mole-rats show large inter and intraspecies variation, and a significant proportion of animals show arrhythmic activity (Oosthuizen et al., 2003; Hart et al., 2004; de Vries et al., 2008; Oosthuizen and Bennett, 2015; Ackermann et al., 2017; Haupt et al., 2017).
In aboveground dwelling animals, the visual environments and light levels of nocturnal and diurnal animals differ markedly, and over time, the eyes of animals have adapted to the amount and quality of light they are exposed to in their particular environments (Hall and Ross, 2006). The anatomy of the eye is therefore usually a good indicator of the temporal niche that animals occupy (Gerkema et al., 2013). The general morphology of the eye (size and shape) and types of photoreceptors present differ between nocturnal and diurnal animals (Schmitz and Motani, 2010). Nocturnal animals frequently have large eyes, large pupils to allow more light to enter the eye, and large corneas and lenses relative to eye size as an adaptation for increased visual sensitivity, whereas the opposite is true for diurnal animals (Hall et al., 2012). The retinas of nocturnal animals typically contain greater numbers of rod photoreceptors for higher light sensitivity, while diurnal animals have more cone photoreceptors for colour vision and have a higher threshold for bright light (Kelber, 2018). Commonly, nocturnal rodents have cone populations in the order of 0.5–3% of the total photoreceptor complement whereas diurnal species have much higher proportions of cones in their retina (Feldman and Phillips, 1984; Szél and Röhlich, 1992; Calderone and Jacobs, 1995; Peichl and Moutairou, 1998; Bobu et al., 2008; Gaillard et al., 2008; Kryger et al., 2018; Van der Merwe et al., 2018).
Surprisingly, the eye morphology of mole-rats resembles that of diurnal rather than nocturnal animals in several aspects. While the eye size of all mole-rats is microphthalmic (1.3–3.5 mm range), most species (except the naked mole-rat) have small lenses compared to their eye sizes (Nikitina et al., 2004; Němec et al., 2008a). In addition, the photoreceptor proportions are more similar to diurnal rodents than nocturnal rodents. Although the retina is rod dominated, mole-rats possess in the vicinity of 10% cones, which is very high in comparison to nocturnal rodents [mouse: 3% (Jeon et al., 1998); rat: <1% (Szél and Röhlich, 1992; Peichl et al., 2004; Němec et al., 2008a)]. In reality, some mole-rat species show diurnal activity [Damaraland mole-rat (Lovegrove et al., 1993; Oosthuizen et al., 2003), Silvery mole-rat (Ackermann et al., 2017)], and some others display nocturnal activity (Cape mole-rat (Lovegrove and Papenfus, 1995; Oosthuizen et al., 2003), all Cryptomys species investigated (Hart et al., 2004; Schöttner et al., 2006; Haupt et al., 2017), Ansell’s mole-rat (de Vries et al., 2008) and the naked mole-rat (Riccio and Goldman, 2000b). In some cases, animals from a species display different temporal activity in different experiments, for example Damaraland mole-rats displayed diurnal activity in the study by Lovegrove and Papenfus (1995), whereas Oosthuizen and colleagues found most animals to be diurnal, but also some nocturnal animals in 2003, and in 2015, all 16 animals displayed nocturnal activity (Oosthuizen et al., 2003; Oosthuizen and Bennett, 2015).
Laboratory conditions, however, are very unnatural for mole-rats. In their natural habitat, their burrow systems are sealed and devoid of light, therefore the animals are not routinely exposed to light for entrainment (Bennett and Faulkes, 2000). Even if a burrow is opened, very little light penetrates to the burrow, and it dissipates very rapidly (Kott et al., 2014). Hence, temporal activity patterns of mole-rats are likely more strongly influenced by their social environment and conditions in their microenvironment. Social entrainment is generally not regarded as a strong zeitgeber, but in the absence of light, it may play a larger role in synchronising activity of animals (Mistlberger and Skene, 2004). Some studies indicate that free-living mole-rats in colonies are active at the same time (Šklíba et al., 2014; Oosthuizen et al., 2021), whereas another suggests that individual activity patterns are not synchronised (Lövy et al., 2013). Social synchronisation of activity is probably also to some degree dependent on the body size of the animals and ambient temperature in the burrow systems. Species with smaller body sizes may be more synchronised than larger-bodied species for thermoregulatory purposes, as animals would huddle together the nest when it is colder (Šumbera, 2019). There are daily and seasonal temperature fluctuations in the burrows, albeit dampened compared to aboveground (Bennett et al., 1988; Lovegrove and Knight-Eloff, 1988; Lövy, 2011; Šklíba et al., 2014). Mole-rats seem to be very sensitive to these changes and have been shown to entrain their locomotor activity to temperature rhythms in the laboratory (Van Jaarsveld et al., 2019; Hart et al., 2021b). Under field conditions, in the absence of light, ambient temperature could substitute as the primary cue for daily entrainment (Oosthuizen et al., 2021).
Seasonal Rhythmicity in Mole-Rats
Many animals exhibit seasonal changes in their behaviour and physiology, especially ones that inhabit temperate regions with large annual environmental changes (Prendergast et al., 2002). The pineal hormone, melatonin, plays a crucial role the mediation of seasonality. The pineal gland activity is under circadian control, such that melatonin is secreted exclusively during the night and is suppressed by light (Wehr, 1997; Richter et al., 2003). The duration of the nocturnal melatonin peak is used for photoperiodic information. The melatonin rhythm is closely associated with the Tb rhythm and can also be modulated by non-photic cues such as exercise (Wyatt et al., 1999; Yamanaka et al., 2014). Animals use proximate factors such as day length and temperature to time ultimate processes such as reproduction, hibernation and migration, all of which require prior anticipation and preparation.
Locomotor Activity and Body Temperature
Mole-rats do not hibernate, although some species are more heterothermic (Lovegrove, 1986; Bennett et al., 1993; Marhold and Nagel, 1995; Boyles et al., 2012; Oosthuizen et al., 2021), and a recent study shows evidence of occasional torpor events in one of the social Cryptomys species. These torpor bouts were not frequent, did not show any rhythmic pattern and were not related to Ta (Oosthuizen et al., 2021).
Several laboratory studies have investigated photoperiodic changes in locomotor activity, however these studies invariably subjected animals to long and short-day light conditions without taking ambient temperature into account. All laboratory studies indicate that both solitary and social mole-rats can adjust their activity periods according to different day lengths, thus effectively synchronising to long and short-day light cycles (Lovegrove et al., 1993; Oosthuizen et al., 2003; Ackermann et al., 2017). Since it is difficult to recreate authentic seasonal changes in the laboratory, seasonal variations in locomotor activity and Tb can best be observed in the field. The measurement of locomotor activity of free-living mole-rats can be challenging and labour intensive, but radiotelemetry and implanted data loggers have been used successfully in the field (Šklíba et al., 2007; Streicher et al., 2011; Lövy et al., 2013; Okrouhlík et al., 2021; Oosthuizen et al., 2021). Data loggers measure body temperature variations, and locomotor activity can be inferred from increases and decreases in body temperature (Refinetti, 1999; Oosthuizen et al., 2021). Mound production has also been used to assess activity of animals (Genelly, 1965; Herbst and Bennett, 2006), however, this is not a very accurate measure of circadian or seasonal activity. Although mole-rats may create more mounds during certain times of the day, they may be active during other times as well (Hickman, 1980). Seasonally, mound production is highly dependent on rainfall, several species have been reported to be more active after rainfalls (Genelly, 1965; De Graaf, 1972; Lovegrove, 1988; Buffenstein et al., 2012). Mole-rats can still extend burrows during the dry season, but instead of digging through the hard top layers of soil, old tunnels are filled up while no aboveground activity is visible (Jarvis et al., 1998; Šumbera et al., 2003b). Some of the solitary species change their burrow architecture seasonally by changing burrowing strategy, but in most species, burrow systems are not significantly modified according to season (Šumbera et al., 2003b; Thomas et al., 2012a,b, 2013, 2016).
Field data shows that there are seasonal shifts in locomotor activity and Tb in several species of mole-rats (Table 2; Šklíba et al., 2007; Streicher et al., 2011; Okrouhlík et al., 2021; Oosthuizen et al., 2021; Finn et al., 2022). Locomotor activity and Tb of the smaller bodied social species, and solitary animals seem to be correlated with burrow temperature, whereas the large bodied social species appear to be arrhythmic in the field and activity has no relation to burrow temperatures (Lövy et al., 2013). These shifts in activity could again be related to thermoregulatory constraints of smaller animals, and it is not known whether the rhythms exhibited are endogenous or in response to environmental conditions.
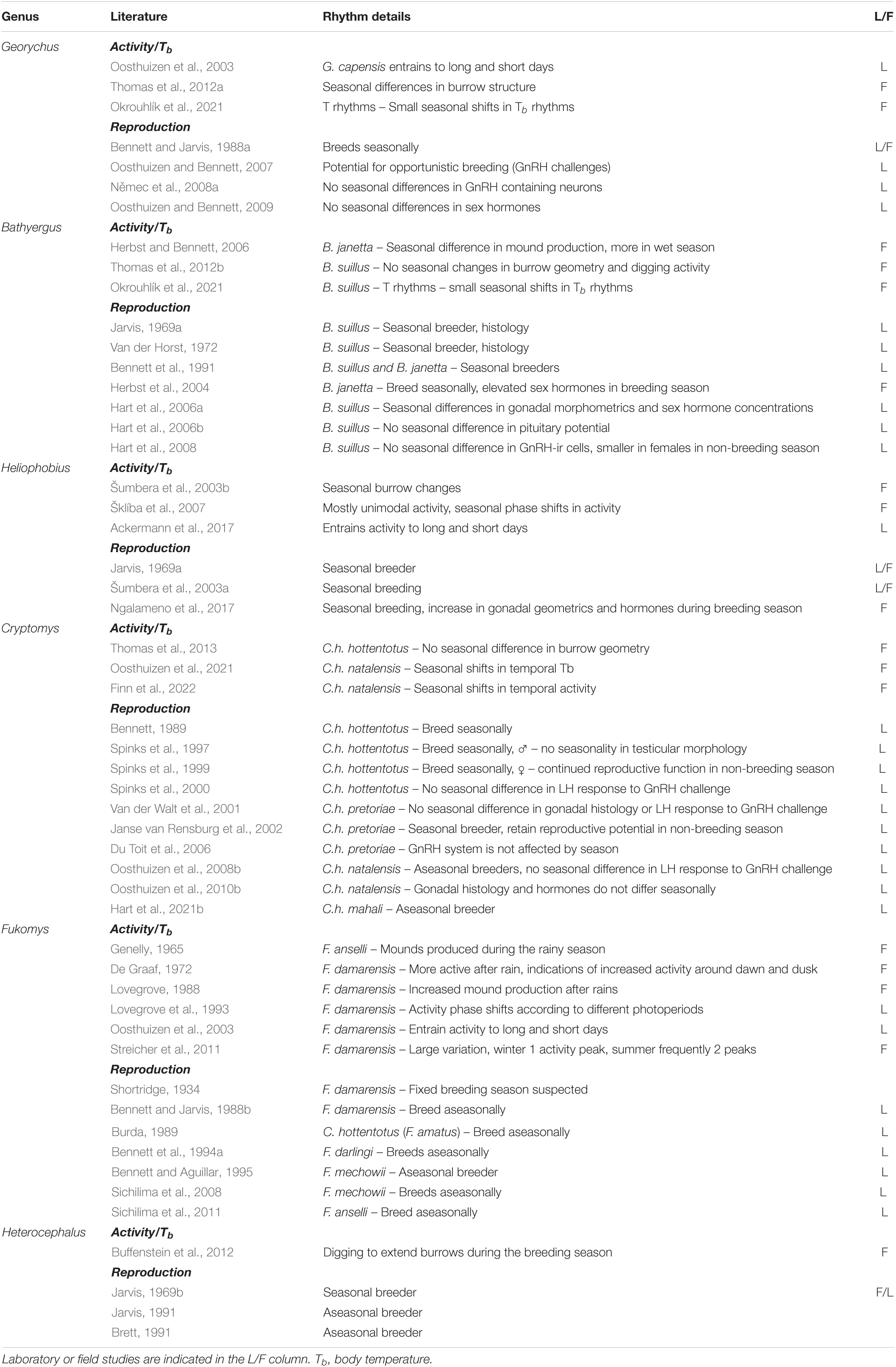
Table 2. A summary of the literature on the presence or absence of seasonal rhythms in African mole-rats.
Seasonal Breeding
Solitary subterranean mammals frequently breed seasonally (Nevo, 1961; Bennett and Faulkes, 2000; Sol Fanjul et al., 2006; Katandukila et al., 2013), with the breeding season typically associated with rainfall and food availability to ensure that young are born at the optimal time of the year (Katandukila et al., 2013). This is also true for African mole-rats, all solitary mole-rat species are seasonal breeders (Jarvis, 1969b; Van der Horst, 1972; Bennett and Jarvis, 1988a; Bennett et al., 1991; Šumbera et al., 2003a; Herbst et al., 2004; Hart et al., 2006a,b). Solitary mole-rats are notoriously difficult to breed in the laboratory, thus all information on the seasonality of breeding originates from field studies. However, laboratory studies have confirmed that all of the solitary species do have the potential for opportunistic breeding should environmental conditions allow it (Herbst et al., 2004; Hart et al., 2006a,b, 2008; Oosthuizen and Bennett, 2007, 2009; Oosthuizen et al., 2008a; Ngalameno et al., 2017).
Solitary mole-rats are usually aggressive and territorial, in particular outside the breeding season (Nevo, 1979; Šumbera, 2001; Bennett et al., 2006). They also typically have long gestation times (Bathyergus ∼50 days, Georychus ∼46 days and Heliophobius ∼90 days) (Bennett and Faulkes, 2000), which suggests that they would have to anticipate the breeding season well in advance and require a proximal environmental cue other than light. Most of the solitary bathyergids inhabit mesic areas with relatively predictable rainfall seasons (Bennett and Faulkes, 2000), and indeed, the breeding of solitary mole-rats appear to be strongly linked with the rainy seasons (Šumbera et al., 2003a; Herbst et al., 2004; Hart et al., 2006a). Mole-rats have also been shown to be very sensitive to small fluctuations in burrow temperatures (Bennett et al., 1988; Šklíba et al., 2007; Lövy et al., 2013). It is likely that solitary mole-rats use burrow temperature as a proximate factor to anticipate the rainy season with its more abundant food and dispersal opportunities.
Some social mole-rat species have also been described as seasonal breeders (Shortridge, 1934; Jarvis, 1969a; Bennett, 1989; Janse van Rensburg et al., 2002), with more recent studies contradicting some of the older ones (Bennett and Jarvis, 1988b; Brett, 1991). Nevertheless, the majority of the social species breed aseasonally, with pregnant females captured at all times of the year (Bennett and Jarvis, 1988b; Burda, 1989; Brett, 1991; Bennett et al., 1994a; Bennett and Aguillar, 1995; Oosthuizen et al., 2008b; Sichilima et al., 2008, 2011; Hart et al., 2021a). In both seasonally and aseasonally breeding species, there are no seasonal differences in gonadal histology, gonadal hormones, LH responses to GnRH challenges, or the GnRH system in the brain (Spinks et al., 1997, 1999, 2000; Van der Walt et al., 2001; Du Toit et al., 2006; Oosthuizen et al., 2008b, 2010b).
Once in an established colony, social mole-rat species have the advantage that they do not have to search for breeding partners. The seasonally breeding C.h. hottentotus lives in a mesic habitat with a predictable rainfall pattern, occurring sympatrically with two of the solitary species. This species has a long gestation period of about 2 months (Bennett, 1989), suggesting the need for an external cue for the onset of the breeding season. This species probably also responds to burrow temperature changes to trigger breeding activity. Although aseasonally breeding social species also have long gestation periods, ranging from around 60–110 days (Bennett and Jarvis, 1988b; Burda, 1989; Jarvis, 1991; Bennett et al., 1994a; Bennett and Aguillar, 1995; Bennett and Faulkes, 2000; Hart et al., 2021a), they breed throughout the year, independent of external factors. For most of the social mole-rat species, the only seasonal consideration would be dispersal. Depending on their habitat, many of the species are confined to their natal colonies during drier periods and can only disperse after rainfall when the soil is soft enough to dig. Timing of dispersal does not require an endogenous rhythm; it is most likely a direct response to rainfall and triggered by softer soils.
Do Mole-Rats Really Need Rhythms?
The ubiquitous nature of the circadian clock suggests an adaptive significance, and whilst this may be true for many species, it may be less obvious for animals that inhabit stable habitats. All mole-rats show a regression of the visual system with small eyes and reduced structures that are related to vision. Their circadian systems are functional, but also appear to show some degree of regression, although not as severe as for the visual system. Mole-rat circadian systems are less sensitive to light compared to aboveground dwelling rodents, nevertheless light still seems to override other environmental zeitgebers when it is present. In the absence of light, mole-rats are responsive to non-photic cues such as ambient temperature and to some extent, also social entrainment. The extent of regression of the visual system and circadian system in the various mole-rat appears to reflect the need or dependence on daily and seasonal rhythms. Daily variations in the activity and physiology of mole-rats do not require an endogenous rhythm per se, it can purely be a response to environmental conditions that can vary from day to day. In terms of seasonalbreeding, mole-rats have long gestation times and hence would require advance warning for the onset of the breeding season. The breeding season of all the solitary mole-rat species occurs at the end of the rainy seasons, both in South Africa and in East Africa (Bennett and Faulkes, 2000; Ngalameno et al., 2017; Okrouhlík et al., 2021), they can therefore respond to rainfall and moister soil to initiate reproductive behaviour. Anticipation of the breeding season may be most pertinent for the Namaqua dune mole-rat (B. janetta), which occurs in more arid habitats where rainfall may be less regular, unless they simply refrain from breeding when it is too dry. Overall, it appears that the survival and fitness of African mole-rats do not depend heavily on endogenous circadian or seasonal rhythmicity. It is likely that their circadian systems are in the process of regression and disappearance, although at a different speed compared to the rest of the visual system.
Ecological and Evolutionary Implications
Climate change is one of the biggest threats to biodiversity currently (Williams et al., 2008). It brings about unpredictable and extreme changes in environmental conditions such as temperature and rainfall (Huber and Gulledge, 2011). Aboveground dwelling species are perhaps more directly and immediately affected by these threats, while the subterranean environment largely buffers its inhabitants against environmental extremes. Nevertheless, both temperature and rainfall are important zeitgebers for mole-rats to time their daily and seasonal activities. Responding directly to environmental conditions instead of having very robust endogenous rhythms may be viewed as an advantage in these conditions since rapid shifts in daily activity would not affect the animals adversely. Nevertheless, changes in temperatures and rainfall can influence the food sources and the availability thereof, as well as the hardness of soil for extending burrows and dispersal. A considerable disadvantage of being strictly subterranean is that animals cannot escape their habitat easily should it become uninhabitable. Climate change is therefore a very real threat for African mole-rats, although their particular vulnerabilities are associated with their habitat and differ from those of aboveground dwelling rodents.
Author Contributions
MO wrote the manuscript. NB reviewed the manuscript. Both authors contributed to the article and approved the submitted version.
Conflict of Interest
The authors declare that the research was conducted in the absence of any commercial or financial relationships that could be construed as a potential conflict of interest.
Publisher’s Note
All claims expressed in this article are solely those of the authors and do not necessarily represent those of their affiliated organizations, or those of the publisher, the editors and the reviewers. Any product that may be evaluated in this article, or claim that may be made by its manufacturer, is not guaranteed or endorsed by the publisher.
Supplementary Material
The Supplementary Material for this article can be found online at: https://www.frontiersin.org/articles/10.3389/fevo.2022.878533/full#supplementary-material
References
Ackermann, S., Bennett, N. C., Katandukila, J. V., and Oosthuizen, M. K. (2017). Circadian rhythms of locomotor activity in captive Emin’s mole-rats, Heliophobius emini (Rodentia: Bathyergidae). J. Mammal. 98, 194–203. doi: 10.1093/jmammal/gyw166
Agostino, P. V., Golombek, D. A., and Meck, W. H. (2011). Unwinding the molecular basis of interval and circadian timing. Front. Integr. Neurosci. 5:64. doi: 10.3389/fnint.2011.00064
Amir, S., and Stewart, J. (1998). Conditioning in the circadian system. Chronobiol. Int0 15, 447–456. doi: 10.3109/07420529808998701
Aral, E., Uslu, S., Sunal, E., Sariboyaci, A., Okar, I., and Aral, E. (2006). Response of the pineal gland in the rats exposed to three different light spectra of short periods. Turk. J. Vet. Anim. Sci. 30, 29–34.
Arnold, W., Ruf, T., Loe, L. E., Irvine, R. J., Ropstad, E., Veiberg, V., et al. (2018). Circadian rhythmicity persists through the polar night and midnight sun in Svalbard reindeer. Sci. Rep. 8:14466. doi: 10.1038/s41598-018-32778-4
Aschoff, J. (1960). Exogenous and endogenous components in circadian rhythms. Cold Spring Harb. Symp. Quant. Biol. 25, 11–28. doi: 10.1101/sqb.1960.025.01.004
Aschoff, J., and Pohl, H. (1978). Phase relations between a circadian rhythm and its zeitgeber within the range of entrainment. Naturwissenschaften 65, 80–84. doi: 10.1007/BF00440545
Ashley, N. T., Ubuka, T., and Schwabl, I. (2014). Revealing a circadian clock in captive arctic-breeding songbirds, lapland longspurs (Calcarius lapponicus), under constant illumination. J. Biol. Rhythms 29, 456–469. doi: 10.1177/0748730414552323
Avivi, A., Albrecht, U., Oster, H., Joel, A., Beiles, A., and Nevo, E. (2001). Biological clock in total darkness: the Clock/MOP3 circadian system of the blind subterranean mole rat. Proc. Natl. Acad. Sci. U.S.A. 98, 13751–13756. doi: 10.1073/pnas.181484498
Avivi, A., Oster, H., Joel, A., Beiles, A., Albrecht, U., and Nevo, E. (2002). Circadian genes in a blind subterranean mammal II: conservation and uniqueness of the three Period homologs in the blind subterranean mole rat, Spalax ehrenbergi superspecies. Proc. Natl. Acad. Sci. U.S.A. 99, 11718–11723. doi: 10.1073/pnas.182423299
Bargiello, T. A., and Young, M. W. (1984). Molecular genetics of a biological clock in Drosophila. Proc. Natl. Acad. Sci. U.S.A. 81, 2142–2146. doi: 10.1073/pnas.81.7.2142
Baydas, G., Ercel, E., Canatan, H., Donder, E., and Akyol, A. (2001). Effect of melatonin on oxidative status of rat brain, liver and kidney tissues under constant light exposure. Cell Biochem. Funct. 19, 37–41. doi: 10.1002/cbf.897
Beale, A. D., Whitmore, D., and Moran, D. (2016). Life in a dark biosphere: a review of circadian physiology in “arrhythmic” environments. J. Comparat. Physiol. B 186, 947–968. doi: 10.1007/s00360-016-1000-6
Beale, A., Guibal, C., Tamai, T., Klotz, L., Cowen, S., Peyric, E., et al. (2013). Circadian rhythms in mexican blind cavefish Astyanax mexicanus in the lab and in the field. Nat. Commun. 4:2769. doi: 10.1038/ncomms3769
Bennett, N. (1989). The social structure and reproductive biology of the common mole-rat, Cryptomys h. hottentotus and remarks on the trends in reproduction and sociality in the family Bathyergidae. J. Zool. Lond. 219, 45–59. doi: 10.1111/J.1469-7998.1989.TB02564.X
Bennett, N. C. (1992). The locomotory activity patterns of a functionally complete colony of Cryptomys hottentotus hottentotus (Rodentia: Bathyergidae). J. Zool. Lond. 228, 425–443.
Bennett, N. C., Jarvis, J., and Cotterill, F. (1994a). The colony structure and reproductive biology of the afrotropical Mashona mole-rat, Cryptomys darlingi. J. Zool. Lond. 234, 477–487. doi: 10.1111/j.1469-7998.1994.tb04861.x
Bennett, N. C., Aguilar, G. H., Jarvis, J. U., and Faulkes, C. G. (1994b). Thermoregulation in three species of Afrotropical subterranean mole-rats (Rodentia: Bathyergidae) from Zambia and Angola and scaling within the genus Cryptomys. Oecologia 97, 222–227. doi: 10.1007/BF00323153
Bennett, N. C., and Aguillar, G. H. (1995). The reproductive biology of the giant Zambian mole-rat, Cryptomys mechowi (Rodentia: Bathyergidae). S. Afr. J. Zool. 30, 1–4. doi: 10.1080/02541858.1995.11448364
Bennett, N. C., and Faulkes, C. G. (2000). African Mole-Rats: Ecology and Eusociality. Cambridge: Cambridge University Press.
Bennett, N. C., and Jarvis, J. U. (1988a). The reproductive biology of the Cape mole-rat, Georychus capensis (Rodentia, Bathyergidae). J. Zool. Lond. 214, 95–106. doi: 10.1111/j.1469-7998.1988.tb04989.x
Bennett, N. C., and Jarvis, J. U. (1988b). The social structure and reproductive biology of colonies of the mole-rat, Cryptomys damarensis (Rodentia, Bathyergidae). J. Mammal. 69, 293–302. doi: 10.2307/1381379
Bennett, N. C., Jarvis, J. U. M., and Cotterill, F. P. D. (1993). Poikilothermic traits and thermoregulation in the Afrotropical social subterranean Mashona mole-rat (Cryptomys hottentotus darlingi) (Rodentia: Bathyergidae). J. Zool. Lond. 231, 179–186. doi: 10.1111/j.1469-7998.1993.tb01910.x
Bennett, N. C., Jarvis, J. U., Aguilar, G. H., and McDaid, E. J. (1991). Growth and development in six species of African mole-rats (Rodentia: Bathyergidae). J. Zool. Lond. 225, 13–26. doi: 10.1016/j.jtherbio.2015.08.003
Bennett, N. C., Jarvis, J. U., and Davies, K. C. (1988). Daily and seasonal temperatures in the burrows of African rodent moles. S. Afr. J. Zool. 23, 189–195. doi: 10.1080/02541858.1988.11448101
Bennett, N. C., Maree, S., and Faulkes, C. G. (2006). Georychus capensis. Mammal. Spec. 799, 1–4. doi: 10.1644/799.1
Berson, D. M. (2003). Strange vision: ganglion cells as circadian photoreceptors. Trends Neurosci. 26, 314–320. doi: 10.1016/s0166-2236(03)00130-9
Bhagwandin, A., Gravett, N., Hemmingway, J., Oosthuizen, M. K., Bennett, N. C., Siegel, J. M., et al. (2011a). Orexinergic neuron numbers in three species of african mole-rats with rhythmic and arrhythmic chronotypes. Neuroscience 199, 153–165. doi: 10.1016/j.neuroscience.2011.10.023
Bhagwandin, A., Gravett, N., Lyamin, O. I., Oosthuizen, M. K., Bennett, N. C., Siegel, J. M., et al. (2011b). Sleep and wake in rhythmic versus arrhythmic chronotypes of a microphthalmic species of African mole rat (Fukomys mechowii). Brain Behav. Evol. 78, 162–183. doi: 10.1159/000330360
Bird, B. L., Branch, L. C., and Miller, D. L. (2004). Effects of coastal lighting on foraging behavior of beach mice. Conserv. Biol. 18, 1435–1439.
Blask, D. E., and Hill, S. M. (1986). Effects of melatonin on cancer: studies on MCF-7 human breast cancer cells in culture. J. Neural Transmiss. 21, 433–449.
Bloch, G., Barnes, B. M., Gerkema, M. P., and Helm, B. (2013). Animal activity around the clock with no overt circadian rhythms: patterns, mechanisms and adaptive value. Proc. Biol. Sci. 280:20130019. doi: 10.1098/rspb.2013.0019
Bobu, C., Lahmam, M., Vuillez, P., Ouarour, A., and Hicks, D. (2008). Photoreceptor organisation and phenotypic characterization in retinas of two diurnal rodent species: potential use as experimental animal models for human vision research. Vis. Res. 48, 424–432. doi: 10.1016/j.visres.2007.08.011
Borghi, C. E., Giannoni, S. M., and Roig, V. G. (2002). Eye reduction in subterranean mammals and eye protective behavior in Ctenomys. J. Neotrop. Mammal. 9, 123–134.
Boyles, J. G., Verburgt, L., McKechnie, A. E., and Bennett, N. C. (2012). Heterothermy in two mole-rat species subjected to interacting thermoregulatory challenges. J. Exp. Zool. A Ecol. Genet. Physiol. 317, 73–82.
Brett, R. A. (1991). “The population structure of naked mole-rat colonies,” in The Biology of the Naked Mole-Rat, eds P. W. Sherman, J. U. M. Jarvis, and R. D. Alexande (Princeton, NJ: Princeton University Press), 97–136.
Bronchti, G., Rado, R., Terkel, J., and Wollberg, Z. (1991). Retinal projections in the blind mole rat: a WGA-HRP tracing study of a natural degeneration. Dev. Brain Res. 58, 159–170. doi: 10.1016/0165-3806(91)90002-Z
Brown, L. S., and Doyle, F. J. I. (2020). A dual feedback loop model of the mammalian circadian clock for multi-input control of circadian phase. PLoS Comput. Biol. 16:e1008459. doi: 10.1371/journal.pcbi.1008459
Brückmann, G., and Burda, H. (1997). Hearing in blind subterranean Zambian mole-rats (Cryptomys sp.): collective behavioural audiogram in a highly social rodent. J. Comp. Physiol. A 181, 83–88. doi: 10.1007/s003590050095
Buffenstein, R. (2005). The naked mole-rat: a new long-living model for human aging research. J. Gerontol. Ser. A Biol. Sci. Med. Sci. 60, 1369–1377.
Buffenstein, R., and Yahav, S. (1991). Is the naked mole-rat Heterocephalus glaber an endothermic yet poikilothermic mammal? J. Therm. Biol. 16, 227–242. doi: 10.1016/0306-4565(91)90030-6
Buffenstein, R., Park, T., Hanes, H., and Artwohl, J. E. (2012). “Naked mole rat,” in The Laboratory Rabbit, Guinea Pig, Hamster, and Other Rodents, eds M. A. Suckow, K. A. Stevens, and R. P. Wilson (Cambridge, MA: Academic Press).
Burda, H. (1989). Reproductive biology (behaviour, breeding, and postnatal development) in subterranean mole-rats, Cryptomys hottentotus (Bathyergus). Z. Säugetierkd. 54, 360–376.
Burda, H., Bruns, V., and Muller, J. (1990). Evolution of Subterranean Mammals a the Organismal and Molecular Levels. New York, NY: Wiley.
Cagnacci, A., Elliott, J. A., and Yen, S. S. (1992). Melatonin: a major regulator of the circadian rhythm of core temperature in humans. J. Clin. Endocrinol. Metab. 75, 447–452. doi: 10.1210/jcem.75.2.1639946
Calderone, J. B., and Jacobs, G. H. (1995). Regional variations in the relative sensitivity to UV light in the mosue retina. Vis. Neurosci. 12, 463–468. doi: 10.1017/s0952523800008361
Camin, S. R., and Madoery, L. A. (1994). Feeding behavior of the tuco tuco (Ctenomys mendocinus): its modifications according to food availability and the changes in the harvest pattern and consumption. Rev. Chil. Hist. Nat. 67, 257–263.
Castillo, M. R., Hochstetler, K. J., Greene, D. M., Firmin, S. I., Tavernier, R. J., Raap, D. K., et al. (2005). Circadian rhythm of core body temperature in two laboratory mouse lines. Physiol. Behav. 86, 538–545. doi: 10.1016/j.physbeh.2005.08.018
Cavallari, N., Frigato, E., Vallone, D., Fröhlich, F., Lopez-Olmeda, J. F., Foà, A., et al. (2011). A blind circadian clock in cavefish reveals that opsins mediate peripheral clock photoreception. PLoS Biol. 9:e1001142. doi: 10.1371/journal.pbio.1001142
Comas, M., Gordon, C. J., Oliver, B. G., Stow, N. W., King, G., Sharma, P., et al. (2017). A circadian based inflammatory response – implications for respiratory disease and treatment. BMC Sleep Sci. Pract. 1:18. doi: 10.1186/s41606-017-0019-2
Cooper, H. M., Herbin, M., and Nevo, E. (1993a). Ocular recression conceals adaptive progression of the visual system in a blind subterranean mammal. Nature 361, 156–159. doi: 10.1038/361156a0
Cooper, H. M., Herbin, M., and Nevo, E. (1993b). Visual system of the naturally microphthalmic mammal: the blind mole rat, Spalax ehrenbergi. J. Comp. Neurol. 328, 313–350. doi: 10.1002/cne.903280302
Correa, A., Greene, A. V., Lewis, Z. A., and Bell-Pedersen, D. (2003). Molecular genetics of circadian rhythms in Neurospora crassa. Appl. Mycol. Biotechnol. 3, 43–63. doi: 10.1016/S1874-5334(03)80006-0
Crish, S. D., Dengler-Crish, C. M., and Catania, K. C. (2006). Central visual system of the naked mole-rat (Heterocephalus glaber). Anatom. Rec. A 288, 205–212. doi: 10.1002/ar.a.20288
Crompton, A. W., Taylor, C. R., and Jagger, J. A. (1978). Evolution of homeothermy in mammals. Nature 272, 333–336. doi: 10.1038/272333a0
Cuvelier, D., Legendre, P., Laes, A., Sarradin, P.-M., and Sarrazin, J. (2014). Rhythms and community dynamics of a hydrothermal tubeworm assemblage at main endeavour field—a multidisciplinary deep-sea observatory approach. PLoS One 9:e96924. doi: 10.1371/journal.pone.0096924
Dauchy, R. T., Blask, D. E., Sauer, L. A., Brainard, G. C., and Krause, J. A. (1999). Dim light during darkness stimulates tumor progression by enhancing tumor fatty acid uptake and metabolism. Cancer Lett. 144, 131–136. doi: 10.1016/s0304-3835(99)00207-4
Davidson, A. J., Sellix, M. T., Daniel, J., Yamazaki, S., Menaker, M., and Block, G. D. (2006). Chronic jet-lag increases mortality in aged mice. Curr. Biol. 16, R914–R916. doi: 10.1016/j.cub.2006.09.058
Davies, W. I. L., Tay, B.-H., Zheng, L., Danks, J. A., Brenner, S., Foster, R. G., et al. (2012). Evolution and functional characterisation of melanopsins in a deep-sea chimaera (elephant shark, Callorhinchus milii). PLoS One 7:e51276. doi: 10.1371/journal.pone.0051276
Davis-Walton, J., and Sherman, P. W. (1994). Sleep arrhythmia in the Eusocial naked mole-rat. Naturwissenschaften 81, 272–275. doi: 10.1007/BF01131581
De Graaf, G. (1972). On the mole-rat (Cryptomys hottentotus damarensis) (Rodentia) in the Kalahari Gemsbok National Park. Koedoe 15, 25–35.
de Vries, J. L., Oosthuizen, M. K., Sichilima, A. M., and Bennett, N. C. (2008). Circadian rhythms of locomotor activity in Ansell’s mole-rat: are mole-rat’s clocks ticking? J. Zool. 276, 343–349. doi: 10.1111/j.1469-7998.2008.00496.x
DeCoursey, P. J., and Krulas, J. R. (1998). Behavior of SCN-lesioned chipmunks in natural habitat: a pilot study. J. Biol. Rhythms 13, 229–244. doi: 10.1177/074873098129000075
DeCoursey, P. J., Krulas, J. R., Mele, G., and Holley, D. C. (1997). Circadian performance of suprachiasmatic nuclei (SCN)-lesioned antelope ground squirrels in a desert enclosure. Physiol. Behav. 62, 1099–1108. doi: 10.1016/s0031-9384(97)00263-1
Dkhissi-Benyahya, O., Sicard, B., and Cooper, H. M. (2000). Effects of irradiance and stimulus duration on early gene expression (Fos) in the suprachiasmatic nucleus: temporal summation and reciprocity. J. Neurosci. 20, 7790–7797. doi: 10.1523/JNEUROSCI.20-20-07790.2000
Doyle, S. E., Yoshikawa, T., Hillson, H., and Menaker, M. (2008). Retinal pathways influence temporal niche. Proc. Natl. Acad. Sci. U.S.A. 105, 13133–13138. doi: 10.1073/pnas.0801728105
Du Toit, L., Bennett, N. C., Katz, A. A., Kallo, I., and Coen, C. W. (2006). Relations between social status and the gonadotrophin-releasing hormone system in females of two cooperatively breeding species of African mole-rats, Cryptomys hottentotus hottentotus and Cryptomys hottentotus pretoriae: neuroanatomical and neuroendocrinological studies. J. Comparat. Neurol. 494, 303–313. doi: 10.1002/cne.20792
Duffy, J. F., and Wright, K. P. (2005). Entrainment of the human circadian system by light. J. Biol. Rhythms 20, 326–338. doi: 10.1177/0748730405277983
Dunlap, J. C., Loros, J. J., Colot, H. V., Mehra, A., Belden, W. J., Shi, M., et al. (2007). A circadian clock in neurospora: how genes and proteins cooperate to produce a sustained, entrainable, and compensated biological oscillator with a period of about a day. Cold Spring Harb. Symp. Quant. Biol. 72, 57–68. doi: 10.1101/sqb.2007.72.072
Eloff, G. (1958). The functional and structural degeneration of the eye of the South African rodent moles, Cryptomys bigalkei and Bathyergus maritimus. S. Afr. J. Sci. 54, 293–302.
Esquiva, G., Avivi, A., and Hannibal, J. (2016). Non-image forming light detection by melanopsin, rhodopsin, and lon-middlewave (L/M) cone opsin in the subterranean blond mole rat, Spalax ehrenbergi: immunohistochemical characterization, distribution, and connectivity. Front. Neuroanat. 10:61. doi: 10.3389/fnana.2016.00061
Evans, J. A., and Davidson, A. J. (2013). “Health consequences of circadian disruption in humans and animal models,” in Chronobiology: Biological Timing in Health and Disease, ed. M. Gillette (Amsterdarm: Elsevier), 283–323. doi: 10.1016/B978-0-12-396971-2.00010-5
Fang, X., Sim, I., Huang, Z., Gerashchenko, M. V., Xiong, Z., Turanov, A. A., et al. (2014). Adaptations to a subterranean environment and longevity revealed by the analysis of mole rat genomes. Cell Rep. 8, 1354–1364. doi: 10.1016/j.celrep.2014.07.030
Faulkes, C. G., and Bennett, N. C. (2013). Plasticity and constraints on social evolution in African mole-rats: ultimate and proximate factors. Philos. Trans. R. Soc. Lond. B Biol. Sci. 368, 20120347. doi: 10.1098/rstb.2012.0347
Feldman, J. L., and Phillips, C. J. (1984). Comparative retinal pigment epithelium and photoreceptor ultrastructure in nocturnal and fossorial rodents: the eastern woodrat, Neotoma floridana, and the plains pocket gopher, Geomys bursarius. J. Mammal. 65, 231–245. doi: 10.2307/1381162
Finn, K., Janse Van Vuuren, A., Hart, D. W., Süess, T., Zöttl, M., and Bennett, N. C. (2022). Seasonal changes in locomotor activity patterns of wild social Natal mole-rats (Cryptomys hottentotus natalensis). Front. Ecol. Evol. 10:819393. doi: 10.3389/fevo.2022.819393
Fioretti, M. C., Riccardi, C., Menconi, E., and Martini, L. (1974). Control of the circadian rhythm of the body temperature in the rat. Life Sci. 14, 2111–2119. doi: 10.1016/0024-3205(74)90093-9
Gaillard, F., Bonfield, S., Gilmour, G. S., Kuny, S., Mema, S. C., Martin, B. T., et al. (2008). Retinal anatomy and visual performance in a diurnal cone-rich laboratory rodent, the Nile grass rat (Arvicanthis niloticus). J. Comparat. Neurol. 510, 525–538. doi: 10.1002/cne.21798
Gamse, J. T., Shen, Y. C., Thisse, C., Thisse, B., Raymond, P. A., Halpern, M. E., et al. (2001). Otx5 regulates genes that show circadian expression in the zebrafish pineal complex. Nat. Genet. 30, 117–121. doi: 10.1038/ng793
Gardner, M. J., Hubbard, K. E., Hotta, C. T., Dodd, A. N., and Webb, A. A. R. (2006). How plants tell the time. Biochemical Journal 397, 15–24. doi: 10.1042/BJ20060484
Genelly, R. E. (1965). Ecology of the common mole-rat (Cryptomys hottentotus) in Rhodesia. J. Mammal. 46, 647–665. doi: 10.2307/1377935
Gerkema, M. P., Davies, W. L., Foster, R. G., Menaker, M., and Hut, R. A. (2013). The nocturnal bottleneck and the evolution of activity patterns in mammals. Proc. R. Soc. Lond. B Biol. Sci. 280:20120508. doi: 10.1098/rspb.2013.0508
Ghosh, S., Lewis, K. N., Tulsian, R., Astafev, A. A., Buffenstein, R., and Kondratoc, R. V. (2021). It’s about time; divergent circadian clocks in livers of mice and naked mole-rats. FASEB J. 35:321590. doi: 10.1096/fj.202100116R
Golombek, D. A., and Rosenstein, R. E. (2010). Physiology of circadian entrainment. Physiol. Rev. 90, 1063–1102. doi: 10.1152/physrev.00009.2009
Gordon, C. J. (2017). The mouse thermoregulatory system: its impact on translating biomedical data to humans. Physiol. Behav. 179, 55–66. doi: 10.1016/j.physbeh.2017.05.026
Gorman, M. R., Elliott, J. A., and Evans, J. A. (2003). Plasticity of hamster circadian entrainment patterns depends on light intensity. Chronobiol. Int. 20, 233–248. doi: 10.1081/cbi-120018576
Gutjahr, G. H., Janse van Rensburg, L., Malpaux, B., Richter, T. A., and Bennett, N. C. (2004). The endogenous rhythm of plasma melatonin and its regulation by light in the highveld mole-rat (Cryptomys hottentotus pretoriae): a mircothalmic, seasonally breedin rodent. J. Pineal Res. 37, 185–192. doi: 10.1111/j.1600-079X.2004.00151.x
Haim, A., and Portnov, B. A. (2013). Light Pollution as a New Risk Factor for Human Breast and Prostate Cancers. Dordrecht: Springer.
Halberg, F., and Cadotte, I. (1975). Increased mortality in mice exposed to weekly 180 shifts of lighting regime LD 12:12 beginning at 1 year of age. Chronobiologia 2:26.
Hall, M. I., and Ross, C. F. (2006). Eye shape and activity pattern in birds. J. Zool. 271, 437–444. doi: 10.1111/j.1469-7998.2006.00227.x
Hall, M. I., Kamilar, J. M., and Kirk, E. C. (2012). Eye shape and the nocturnal bottleneck of mammals. Proc. R. Soc. Lond. B Biol. Sci. 279, 4962–4968. doi: 10.1098/rspb.2012.2258
Hannibal, J., Hindersson, P., Nevo, E., and Fahrenkrug, J. (2002). The circadian photopigment melanopsin is expressed in the blind subterranean mole rat, Spalax. Neuroreport 13, 1411–1414. doi: 10.1097/00001756-200208070-00013
Hardeland, R., Pandi-Perumal, S. R., and Cardinali, D. P. (2006). Melatonin. Int. J. Biochem. Cell Biol. 38, 313–316. doi: 10.1016/j.biocel.2005.08.020
Hart, D. W., Medger, K., Van Jaarsveld, B., and Bennett, N. C. (2021a). Filling in the holes: the reproductive biology fo hte understudied Mahali mole-rat (Cryptomys hottentotus mahali). Canad. J. Zool 99, 801–811. doi: 10.1139/cjz-2020-0158
Hart, D. W., van Jaarsveld, B., Lasch, K. G., Grenfell, K. L., Oosthuizen, M. K., and Bennett, N. C. (2021b). Ambient temperature as a strong zeitgeber of circadian rhythms in response to temperature sensitivity and poor heat dissipation abilities in subterranean African mole-rats. J. Biol. Rhythms 36, 461–469. doi: 10.1177/07487304211034287
Hart, L., Bennett, N. C., Kalamatianos, T., Oosthuizen, M. K., Jarvis, J. U., O’Riain, M. J., et al. (2008). Neuroanatomical investigation of the gonadotrophin-releasing hormone 1 system in the seasonally breeding Cape dune mole-rat, Bathyergus suillus. Brain Res Bull. 77, 185–188. doi: 10.1016/j.brainresbull.2008.07.004
Hart, L., Bennett, N. C., Malpaux, B., Chimimba, C. T., and Oosthuizen, M. K. (2004). The chronobiology of the Natal mole-rat, Cryptomys hottentotus natalensis. Physiol. Behav. 82, 563–569. doi: 10.1016/j.physbeh.2004.05.008
Hart, L., O’Riain, M. J., Jarvis, J. U., and Bennett, N. C. (2006a). The pituitary potential for opportunistic breeding in the Cape dune mole-rat, Bathyergus suillus. Physiol. Behav. 88, 615–619. doi: 10.1016/j.physbeh.2006.05.020
Hart, L., O’Riain, M. J., Jarvis, J. U. M., and Bennett, N. C. (2006b). Is the Cape dune mole-rat, Bathyergus suillus (Rodentia: Bathyergidae), a seasonal or aseasonal breeder? J. Mammal. 87, 1078–1085. doi: 10.1644/05-MAMM-A-411R2.1
Hastings, J. W., Rusak, B., and Boulos, Z. (1991). “Circadian rhythms: the physiology of biological timing,” in Neural and Integrative Animal Physiology, ed. C. L. Prosser (New York, NY: Wiley-Liss), 435–544.
Hau, M., Romero, L. M., Brawn, J. D., and Van’t Hof, T. J. (2002). Effect of polar day on plasma profiles of melatonin, testosterone, and estradiol in high-Arctic Lapland Longspurs. Gen. Comparat. Endocrinol. 126, 101–112. doi: 10.1006/gcen.2002.7776
Haupt, M., Bennett, N. C., and Oosthuizen, M. K. (2017). Locomotor activity and body temperature patterns over a temperature gradient in the highveld mole-rat (Cryptomys hottentotus pretoriae). PLos One 12:e169644. doi: 10.1371/journal.pone.0169644
Helm, B., Ben-Shlomo, R., Sheriff, M. J., Hut, R. A., Foster, R., Barnes, B. M., et al. (2013). Annual rhythms that underlie phenology: biological time-keeping meets environmental change. Proc. R. Soc. Lond. B Biol. Sci. 280:20130016. doi: 10.1098/rspb.2013.0016
Herbst, M., and Bennett, N. C. (2006). Burrow architecture and burrowing dynamics of the endangered Namaqua dune mole rat (Bathyergus janetta) (Rodentia: Bathyergidae). J. Zool. 270, 420–428. doi: 10.1111/j.1469-7998.2006.00151.x
Herbst, M., Jarvis, J. U. M., and Bennett, N. C. (2004). A field assessment of reproductive seasonality in the threatened wild Namaqua dune mole-rat (Bathyergus janetta). J. Zool. 263, 259–268. doi: 10.1017/s0952836904005114
Heth, G., Todrank, J., Begall, S., Koch, R., Zilbiger, Y., Nevo, E., et al. (2002). Odours underground: subterranean rodents may not forage “blindly”. Behav. Ecol. Sociobiol. 52, 53–58. doi: 10.1007/s00265-002-0476-0
Hickman, G. C. (1980). Locomotory activity of captive Cryptomys hottentotus, (Mammalia: Bathyergidae), a fossorial rodent. J. Zool. Lond. 192, 225–235. doi: 10.1111/j.1469-7998.1980.tb04231.x
Huber, D. G., and Gulledge, J. (2011). Extreme Weather and Climate Change: Understanding the Link, Managing the Risk. Arlington, VA: Pew Center on Global Climate Change.
Husse, J., Eichele, G., and Oster, H. (2015). Synchronization of the mammalian circadian timing system: light can control peripheral clocks independently of the SCN clock. Bioessays 37, 1119–1128. doi: 10.1002/bies.201500026
Janse van Rensburg, L., Bennett, N. C., Van der Merwe, M., and Schoeman, A. S. (2002). Seasonal reproduction in the highveld mole-rat, Cryptomys hottentotus pretoriae (Rodentia: Bathyergidae). Canad. J. Zool. 80, 810–820. doi: 10.1139/z02-051
Jarvis, J. U. M. (1969a). The breeding season and litter size of African mole-rats. J. Reprod. Fert. 6, 237–248.
Jarvis, J. U. M. (1969b). Some Aspects of the Biology of East African Mole-Rats. Ph.D. thesis. Kampala: University of East Africa.
Jarvis, J. U. M. (1973). Activity patterns in the mole-rats Tachyoryctes splendens and Heliophobius argenteocinerius. Zool. Afr. 8, 101–119. doi: 10.1080/00445096.1973.11447470
Jarvis, J. U. M. (1991). Reproduction of Naked Mole-Rats. Princeton, NJ: Princeton University Press.
Jarvis, J. U., Bennett, N. C., and Spinks, A. C. (1998). Food availability and foraging by wild colonies of Damaraland mole-rats (Cryptomys damarensis): implications for sociality. Oecologia 113, 290–298. doi: 10.1007/s004420050380
Jeon, C. J., EStrettoi, E., and Maslinad, R. H. (1998). The major cell populations in the mouse retina. J. Neurosci. 19, 8936–8946. doi: 10.1523/JNEUROSCI.18-21-08936.1998
Karatsoreos, I. N., Bhagat, S., Bloss, E. B., Morisson, J. H., and McEwen, B. S. (2011). Disruption of circadian clocs has ramifications for metabolisms, brain, and behavior. Proc. Natl. Acad. Sci. U.S.A. 108, 1657–1662. doi: 10.1073/pnas.1018375108
Katandukila, J. V., Faulkes, C. G., Chimimba, C. T., and Bennett, N. C. (2013). Reproduction in the East African root rat (Tachyoryctes splendens; Rodentia: Spalacidae) from Tanzania: the importance of rainfall. J. Zool. 291, 258–268.
Kawai, H., and Wada, A. (2007). Diurnal sea surface temperature variation and its impact on the atmosphere and ocean: a review. J. Oceanogr. 63, 721–744. doi: 10.1007/s10872-007-0063-0
Kelber, A. (2018). Vision: rods see in bright light. Curr. Biol. 28, R364–R366. doi: 10.1016/j.cub.2018.02.062
Kim, E., Fang, X., Fushan, A., Huang, Z., Lobanov, A. V., Han, L., et al. (2011). Genome sequencing reveals insights into physiology and longevity of the naked mole rat. Nature 479, 223–227. doi: 10.1038/nature10533
King, D. P., and Takahashi, J. S. (2000). Molecular genetics of circadian rhythms in mammals. Annu. Rev. Neurosci. 23, 713–742. doi: 10.1146/annurev.neuro.23.1.713
Knutsson, A., and Boggild, H. R. E. H. (2000). Shiftwork and cardiovascular disease: review of disease mechanisms. Rev. Environ. Health 15, 359–372. doi: 10.1515/reveh.2000.15.4.359
Ko, C. H., and Takahashi, J. S. (2006). Molecular components of the mammalian circadian clock. Hum. Mol. Genet. 15, 271–277. doi: 10.1093/hmg/ddl207
Kondo, T., and Ishiura, M. (2000). The circadian clock of cyanobacteria. Bioessays 22, 10–15. doi: 10.1002/(SICI)1521-1878(200001)22:1<10::AID-BIES4<3.0.CO;2-A
Kornhauser, J. M., Nelson, D. E., Mayo, K. E., and Takahashi, J. S. (1992). Regulation of junB messenger RNA and AP-1 activity by light and a circadian clock. Science 225, 1581–1584. doi: 10.1126/science.1549784
Kössl, M., Frank, G., Burda, H., and Muller, M. (1996). Acoustic distortion products from the cochlea of the blind African mole rat, Cryptomys spec. J. Comparat. Physiol. A 178, 427–434. doi: 10.1007/BF00193979
Kott, O., Mortitz, R., Šumbera, R., Burda, H., and Němec, P. (2014). Light propagation in burrows of subterranean rodents: tunnel system architecture but not photoreceptor sensitivity limits light sensation range. J. Zool. Lond. 294, 68–76. doi: 10.1111/jzo.12152
Kott, O., Němec, P., Fremlová, A., Mazoch, V., and Šumbera, R. (2016). Behavioural tests reveal severe visual deficits in the strictly subterranean African mole-rats (Bathyergidae) but efficient vision in the fossorial rodent coruro (Spalacopus cyanus,Octodontidae). Ethology 122, 682–694. doi: 10.1111/eth.12515
Kryger, Z., Galli-Resta, L., Jacobs, G. H., and Reese, B. E. (2018). The topography of rod and cone photoreceptors in the retina of the ground squirrel. Vis. Neurosci. 15, 685–691. doi: 10.1017/s0952523898154081
Kucheravy, C. E., Waterman, J. M., dos Anjos, E. A. C., Hare, J. F., Enright, C., and Berkvens, C. N. (2021). Extreme climate event promotes phenological mismatch between sexes in hibernating ground squirrels. Sci. Rep. 11:21684. doi: 10.1038/s41598-021-01214-5
Kuhlman, S. J., Craig, L. M., and Duffy, J. F. (2018). Introduction to Chronobiology. Cold Spring Harb. Perspect. Biol. 10:a033613. doi: 10.1101/cshperspect.a033613
Lacey, E. A., Patton, J. L., and Cameron, G. N. (2001). Life underground: the biology of subterranean rodents. Austr. Mammal. 23, 75–76. doi: 10.1071/AM01075_BR
Lavocat, V. (2013). “Rodentia and lagomorpha,” in Evolution of African Mammals, eds V. J. Maglio and H. B. S. Cooke (Cambridge, MA: Harvard University Press).
Longcore, T., and Rich, C. (2004). Ecological light pollution. Front. Ecol. Environ. 2:191–198. doi: 10.1890/1540-92952004002[0191:ELP]2.0.CO;2
Lovegrove, B. G. (1986). Thermoregulation of the subterranean rodent genus Bathyergus (Bathyergidae). S. Afr. J. Zool. 21, 283–288. doi: 10.1080/02541858.1986.11447999
Lovegrove, B. G. (1988). Colony size and structure, activity patterns and foraging behaviour of a colony of the social mole-rat Cryptomys damarensis (Bathyergidae). J. Zool. 216, 391–402. doi: 10.1111/j.1469-7998.1988.tb02437.x
Lovegrove, B. G., and Knight-Eloff, A. (1988). Soil and burrow temperatures, and the resource characteristics of the social mole-rat Cryptomys damarensis (Bathyergidae) in the Kalahari desert. J. Zool. 216, 403–416. doi: 10.1111/j.1469-7998.1988.tb02438.x
Lovegrove, B. G., and Muir, A. (1996). Circadian body temperature rhythms of the solitary cape mole rat Georychus capensis (Bathyergidae). Physiol. Behav. 60, 991–998. doi: 10.1016/0031-9384(96)00076-5
Lovegrove, B. G., and Papenfus, M. E. (1995). Circadian activity rhythms in the solitary Cape mole rat (Georychus capensis: Bathyergidae) wit hsome evidence of splitting. Physiol. Behav. 58, 679–685. doi: 10.1016/0031-9384(95)00106-s
Lovegrove, B. G., Heldmaier, G., and Ruf, T. (1993). Circadian activity rhythms in colonies of ‘blind’ molerats, Cryptomys damarensis (Bathyergidae). S. Afr. J. Zool. 28, 46–55. doi: 10.1080/02541858.1993.11448287
Lövy, M. (2011). Ecology and Activity of Mesic Afrotropic Mole-Rats. Ph.D. thesis. Ceske Budejovice: University of South Bohemia.
Lövy, M., Šklíba, J., and Šumbera, R. (2013). Spatial and temporal activity patterns of the free-living giant mole-rat (Fukomys mechowii), the largest social bathyergid. PLoS One 8:e55357. doi: 10.1371/journal.pone.0055357
Lu, W., Meng, Q. J., Tyler, N. J., Stokkan, K. A., and Loudon, A. S. (2010). A circadian clock is not required in an arctic mammal. Curr. Biol. 20, 533–537. doi: 10.1016/j.cub.2010.01.042
Marhold, S., and Nagel, A. (1995). The energetics of the common molerat Cryptomys, a subterranean eusocial rodent from Zambia. J. Comparat. Physiol. B 164, 636–645. doi: 10.1007/BF00389805
Marimuthu, G., and Chandrashekaran, M. K. (1985). Communication and synchronization of circadian rhythms in insectivorous bats. Proc. Anim. Sci. 94, 655–665. doi: 10.1007/bf03191866
Maronde, E., Pfeffer, M., Olcese, J., Molina, C. A., Schlotter, F., Dehghani, F., et al. (1999). Transcription factors in neuroendocrine regulation: rhythmic changes in pCREB and ICER levels frame melatonin synthesis. J. Neurosci. 19, 3326–3336. doi: 10.1523/JNEUROSCI.19-09-03326.1999
Mason, M. J., and Narins, P. M. (2001). Seismic signal use by fossorial mammals. Am. Zool. 41, 1171–1184. doi: 10.1093/icb/41.5.1171
Mat, A. M., Sarrazin, J., Markov, G. V., Apremont, V., Dubeuil, C., Eché, C., et al. (2020). Biological rhythms in the deep-sea hydrothermal mussel Bathymodiolus azoricus. Nat. Commun. 11:3454. doi: 10.1038/s41467-020-17284-4
Maynou, F., and Cartes, J. E. (1988). Daily ration estimates and comparative study of food consumption in nine species of deep-water decapod crustaceans of the NW Mediterranean. Mar. Ecol. Progr. Ser. 171, 221–231. doi: 10.3354/meps171221
Menna-Barreto, L., and Trajano, E. (2015). Biological Rhythmicity in Subterranean Animals: A Function Risking Extinction?. London: Springer.
Mills, L. S., Zimova, M., Oyler, J., Steven Running, S., Abatzoglouc, J. T., and Lukacs, P. M. (2013). Camouflage mismatch in seasonal coat color due to decreased snow duration. Proc. Natl. Acad. Sci. U.S.A. 110, 7360–7365. doi: 10.1073/pnas.1222724110
Mills, S. L., and Catania, K. C. (2004). Identification of retinal neurons in a regressive rodent eye (the naked mole-rat). Vis. Neurosci. 21, 107–117. doi: 10.1017/S0952523887043025
Mistlberger, R. E., and Skene, D. J. (2004). Social influences on mammalian circadian rhythms: animal and human studies. Biol. Rev. 79, 533–556. doi: 10.1017/s1464793103006353
Modica, L., Cartes, J. E., and Carrassón, M. (2014). Food consumption of five deep-sea fishes in the Balearic Basin (western Mediterranean Sea): are there daily feeding rhythms in fishes living below 1000 m? J. Fish Biol. 85, 800–820. doi: 10.1111/jfb.12459
Moore, R. (1983). Organization and function of a central nervous system circadian oscillator: the suprachiasmatic nucleus. Fed. Proc. 42, 2783–2789.
Moore, R. (2007). “Organization of the mammalian circadian system,” in Proceedings of the Ciba Foundation Symposium 183 – Circadian Clocks and their Adjustment, eds K. A. Derek and J. Chadwick (Indianapolis, IN: Ciba Foundation), 88–116. doi: 10.1002/9780470514597.ch6
Morgan, P., and Williams, L. M. (1996). The pars tuberalis of the pituitary: a gateway for neuroendocrine output. Rev. Reprod. 1, 153–161. doi: 10.1530/ror.0.0010153
Nakajima, M., Imai, K., Ito, H., Nishiwaki, T., Murayama, Y., Iwasaki, H., et al. (2005). Reconstitution of circadian oscillation of cyanobacterial KaiC phosphorylation in vitro. Science 308, 414–415. doi: 10.1126/science.1108451
Navara, K. J., and Nelson, R. (2007). The dark side of light at night: physiological, epidemiological and ecological consequences. J. Pineal Res. 43, 215–224. doi: 10.1111/j.1600-079X.2007.00473.x
Negroni, J., Bennett, N. C., and Cooper, H. M. (2003). Organization of the circadian system in the subterranean mole rat, Cryptomys hottentotus (Bathyergidae). Brain Res. 967, 48–62. doi: 10.1016/s0006-8993(02)04208-7
Nelson, D. E., and Takahashi, J. S. (1991). Sensitivity and integration in a visual pathway for circadian entrainment in the hamster (Mesocricetus auratus). J. Physiol. 439, 115–145. doi: 10.1113/jphysiol.1991.sp018660
Němec, P., Burda, H., and Peichl, L. (2004). Subcortical visual system of the African mole-rat Cryptomys anselli: to see or not to see? Eur. J. Neurosci. 20, 757–768. doi: 10.1111/j.1460-9568.2004.03510.x
Němec, P., Cvekova, P., Benada, O., Wielkopolska, E., Olkowicz, S., Turlejski, K., et al. (2008a). The visual system in subterranean African mole-rats (Rodentia, Bathyergidae): retina, subcortical visual nuclei and primary visual cortex. Brain Res. Bull. 75, 356–364. doi: 10.1016/j.brainresbull.2007.10.055
Němec, P., Cvekova, P., Burda, H., Benada, O., and Peichl, L. (2008b). “Visual systems and the role of vision in subterranean rodents: diversity of retinal properties and visual system designs,” in Subterranean rodents – News from the underground, eds S. Begall, H. Burda, and C. Schleich (Heidelberg: Springer).
Nevo, E. (1961). Observation on Israeli population of the mole rat Spalax ehrenbergi Nehring 1898. Mammalia 25, 127–144.
Nevo, E. (1979). Adaptive convergence and divergence of subterranean mammals. Ann. Rev. Ecol. Syst. 10, 269–308. doi: 10.1146/annurev.es.10.110179.001413
Nevo, E. (1995). Mammalian evolution underground. The ecological-genetic-phenetic interfaces. Acta Theriol. Suppl. 3, 9–31. doi: 10.4098/at.arch.95-43
Ngalameno, M. K., Bastos, A. D. S., Mgode, G., and Bennett, N. C. (2017). The pattern of reproduction in the mole-rat Heliophobius from Tanzania: do not refrain during the long rains! Can. J. Zool. 95, 107–114. doi: 10.1139/cjz-2016-0153
Nikaido, S. S., and Johnson, C. H. (2000). Daily and circadian variation in survival from ultraviolet radiation in Chlamydomonas reinhardtii. Photochem. Photobiol. 71, 758–765. doi: 10.1562/0031-86552000071<0758:dacvis<2.0.co;2
Nikitina, N. V., Maughan-Brown, B., O’Riain, M. J., and Kidson, S. H. (2004). Postnatal development of the eye in the naked mole rat (Heterocephalus glaber). Anatom. Rec. 277, 317–337. doi: 10.1002/ar.a.20025
Niven, J. E., and Laughlin, S. B. (2008). Energy limitation as a selective pressure on the evolution of sensory systems. J. Exp. Biol. 211, 1792–1804. doi: 10.1242/jeb.017574
Oelschlager, H. A., Namkamura, M., Herzog, M., and Burda, H. (2000). Visual system labeled by c-Fos immunohistochemistry after light exposure in the ‘blind’ subterranean Zambian mole-rat (Cryptomys anselli). Brain Behav. Evol. 55, 209–220. doi: 10.1159/000006653
Oishi, K. (2009). Disrupted light-dark cycle induces obesity with hyperglycemia in genetically intact animals. Neuro Endocrinol. Lett. 30, 458–461.
Okrouhlík, J., Šumbera, R., Gardner, B., Schoemann, K., Lövy, M., and Bennett, N. C. (2021). Are southern African solitary mole-rats homeothermic or heterothermic under natural field conditions? J. Therm. Biol. 95:102810. doi: 10.1016/j.jtherbio.2020.102810
Omlin, F. X. (1997). Optic disc and optic nerve of the blind cape mole-rat (Georychus capensis): a proposed model for naturally occuring reactive gliosis. Brain Res. Bull. 44, 627–632. doi: 10.1016/s0361-9230(97)00283-9
Oosthuizen, M. K., and Bennett, N. C. (2007). LH responses to single doses of exogenous GnRH in the Cape mole rat (Georychus capensis): the pituitary potential for opportunistic breeding. J. Zool. 271, 198–202. doi: 10.1111/j.1469-7998.2006.00201.x
Oosthuizen, M. K., and Bennett, N. C. (2009). Seasonal variation in gonadal steroids of males and females in the Cape mole-rat (Georychus capensis): the potential for opportunistic breeding. Afr. Zool. 44, 117–122. doi: 10.3377/004.044.0111
Oosthuizen, M. K., and Bennett, N. C. (2015). The effect of ambient temperature on locomotor activity patterns in reproductive and non-reproductive female Damaraland mole-rats. J. Zool. 297, 1–8. doi: 10.1111/jzo.12254
Oosthuizen, M. K., Bennett, N. C., and Coen, C. W. (2008a). An immunohistochemical study of the gonadotrophin-releasing hormone 1 system in solitary Cape mole-rats, Georychus capensis, and social Natal mole-rats, Cryptomys hottentotus natalensis. Neuroscience 157, 164–173. doi: 10.1016/j.neuroscience.2008.08.054
Oosthuizen, M. K., Bennett, N. C., Lutermann, H., and Coen, C. W. (2008b). Reproductive suppression and the seasonality of reproduction in the social Natal mole-rat (Cryptomys hottentotus natalensis). Gen. Comparat. Endocrinol. 159, 236–240. doi: 10.1016/j.ygcen.2008.09.004
Oosthuizen, M. K., Bennett, N. C., and Cooper, H. M. (2005). Fos expression in the suptrachiasmatic nucleus in response to light stimulation in a solitary and social species of African mole-rat (family Bathyergidae). Neuroscience 133, 555–560. doi: 10.1016/j.neuroscience.2005.01.017
Oosthuizen, M. K., Bennett, N. C., and Cooper, H. M. (2010a). Photic induction of Fos in the suprachiasmatic nucleus of African mole-rats: responses to increasing irradiance. Chronobiol. Int. 27, 1532–1545. doi: 10.3109/07420528.2010.510227
Oosthuizen, M. K., Viljoen, H., and Bennett, N. C. (2010b). Effects of season and social status on gonadal function in social Natal mole-rats. J. Mammal. 91, 429–436. doi: 10.1644/08-mamm-a-368.1
Oosthuizen, M. K., Cooper, H. M., and Bennett, N. C. (2003). Circadian rhythms of locomotor activity in solitary and social species of African mole-rats (family: Bathyergidae). J. Biol. Rhythms 18, 481–490. doi: 10.1177/0748730403259109
Oosthuizen, M. K., Robb, G., Harrison, A., Froneman, A., Joubert, K., and Bennett, N. C. (2021). Flexibility in body temperature rhythms of free-living Natal mole-rats (Cryptomys hottentotus natalensis). J. Anim. Ecol. 99:102952. doi: 10.1016/j.jtherbio.2021.102973
Paranjpe, D. A., and Sharma, V. K. (2005). Evolution of temporal order in living organisms. J. Circ. Rhythms 3:7. doi: 10.1186/1740-3391-3-7
Park, T. J., Lu, Y., Juttner, R., Smith, E. S. J., Hu, J., Brand, A., et al. (2008). Selective inflammatory pain insensitivity in the African naked mole-rat (Heterocephalus glaber). PLoS Biol. 6:e13. doi: 10.1371/journal.pbio.0060013
Patke, A., Young, M. W., and Axelrod, S. (2019). Molecular mechanisms and physiological importance of circadian rhythms. Nat. Rev. Mol. Cell Biol. 21, 67–84. doi: 10.1038/s41580-019-0179-2
Peichl, L. (2005). Diversity of mammalian photoreceptor properties: adaptations to habitat and lifestyle? Anatom. Rec. A 287, 1001–1012. doi: 10.1002/ar.a.20262
Peichl, L., and Moutairou, K. (1998). Absence of short-wavelength sensitive cones in the retinae of seals (Carnivora) and African giant rats (Rodentia). Eur. J. Neurosci. 10, 2586–2594. doi: 10.1046/j.1460-9568.1998.00265.x
Peichl, L., Němec, P., and Burda, H. (2004). Unusual cone and rod properties in subterranean African mole-rats (Rodentia, Bathyergidae). Eur. J. Neurosci. 19, 1545–1558. doi: 10.1111/j.1460-9568.2004.03263.x
Penev, P. D., Kolker, D. E., Zee, P. C., and Turek, F. W. (1998). Chronic circadian desynchronization decreases the survival of animals with cardiomyopathic heart disease. Am. J. Physiol. 275, H2334–H2337. doi: 10.1152/ajpheart.1998.275.6.H2334
Philips, D. J., Savenkova, M. I., and Karatsoreos, I. N. (2015). Environmental disruption of the circadian clock leads to altered sleep and immune responses in mouse. Brain Behav. Immun. 47, 14–23. doi: 10.1016/j.bbi.2014.12.008
Porter, M. L., and Sumner-Rooney, L. (2018). Evolution in the dark: unifying our understanding of eye loss. Integr. Comparat. Biol. 58, 367–371. doi: 10.1093/icb/icy082
Prendergast, B. J. (2010). MR1 Melatonin receptors mediate somatic, behavioral, and reproductive neuroendocrine responses to photoperiod and melatonin in Siberian hamsters (Phodopus sungorus). Endocrinology 151, 714–721. doi: 10.1210/en.2009-0710
Prendergast, B. J., Nelson, R. J., and Zucker, I. (2002). “Mammalian seasonal rhythms,” in Hormones, Brain and Behavior, eds D. W. Pfaff, S. E. Fahrbach, R. T. Rubin, A. P. Arnold, and A. M. Etgen (Amsterdam: Elsevier), 93–156. doi: 10.1016/b978-012532104-4/50021-4
Quay, W. B. (1981). Pineal atrophy and other neuroendocrine and circumventricular features of the naked mole-rat, Heterocephalus glaber (Rüppell), a fossorial, equatorial rodent. J. Neural Transm. 52, 107–115. doi: 10.1007/BF01253102
Refinetti, R. (1999). Relationship between the daily rhythms of locomotor activity and body temperature in eight mammalian species. Am. J. Physiol. 227, 1493–1500. doi: 10.1152/ajpregu.1999.277.5.R1493
Refinetti, R. (2010). Entrainment of circadian rhythm by ambient tempeature cycles in mice. Chronobiol. Int. 25, 247–256. doi: 10.1177/0748730410372074
Reierth, E., Van’t Hof, T. J., and Stokkan, K. A. (1999). Seasonal and daily variations in plasma melatonin in the high-arctic Svalbard ptarmigan (Lagopus mutus hyperboreus). J. Biol. Rhythms 14, 314–319. doi: 10.1177/074873099129000731
Reiter, R. J., Tan, D. X., Osuna, C., and Gitto, E. (2000). Actions of melatonin in the reduction of oxidative stress. A review. J. Biomed. Sci. 7, 444–458. doi: 10.1007/BF02253360
Reppert, S. M., and Weaver, D. R. (2001). Molecular analysis of mammalian circadian rhythms. Annu. Rev. Physiol. 63, 647–676. doi: 10.1146/annurev.physiol.63.1.647
Riccio, A. P., and Goldman, B. D. (2000a). Circadian rhythms of body temperature and metabolic rate in naked mole-rats. Physiol. Behav. 71, 15–22. doi: 10.1016/s0031-9384(00)00280-8
Riccio, A. P., and Goldman, B. D. (2000b). Circadian rhythms of locomotor activity in naked mole-rats (Heterocephalus glaber). Physiol. Behav. 71, 1–13. doi: 10.1016/s0031-9384(00)00281-x
Richter, T. A., Malpaux, B., Flemming, P. A., Molteno, A. J., and Bennett, N. C. (2003). Melatonin secretion in a strictly subterranean mammal, the Damaraland mole-rat (Cryptomys damarensis). J. Zool. Lond. 261, 313–319. doi: 10.1017/S0952836903004254
Roll, U., Dayan, T., and Kronfeld-Schor, N. (2006). On the role of phylogeny in determining activity patterns of rodents. Evol. Ecol. 20, 479–490. doi: 10.1007/s10682-006-0015-y
Rosato, E., Tauber, E., and Kyriacou, C. P. (2006). Molecular genetics of the fruit-fly circadian clock. Eur. J. Hum. Genet. 14, 729–738. doi: 10.1038/sj.ejhg.5201547
Rose, S., Novak, C. M., Mahoney, M. M., Nunez, A. A., and Smale, L. (1999). Fos expression within vasopressin-containing neurons in the suprachiasmatic nucleus of diurnal rodents compared to nocturnal rodents. J. Biol. Rhythms 14, 37–46. doi: 10.1177/074873099129000425
Rotics, S., Dayan, T., and Kronfeld-Schor, N. (2011). Effect of artificial night lighting on temporally partitioned spiny mice. J. Mammal. 92, 159–168. doi: 10.1644/10-mamm-a-112.1
Ruger, M., and Scheer, F. A. (2009). Effects of circadian disruption on the cardiometabolic system. Rev. Endocr. Metab. Disord. 10, 245–260. doi: 10.1007/s11154-009-9122-8
Saino, N., Ambrosini, R., Rubolini, D., von Hardenberg, J., Provenzale, A., Hüppop, K., et al. (2010). Climate warming, ecological mismatch at arrival and population decline in migratory birds. Proc. R. Soc. B Biol. Sci. 278, 835–842. doi: 10.1098/rspb.2010.1778
Sato, T. K., Panda, S., Miraglia, L. J., Reyes, T. M., Rudic, R. D., McNamara, P., et al. (2004). A functional genomics strategy reveals Rora as a component of the mammalian circadian clock. Neuron 43, 527–537. doi: 10.1016/j.neuron.2004.07.018
Scheiermann, C., Kunisaki, Y., and Frenette, P. S. (2013). Circadian control of the immune system. Nat. Rev. Immunol. 13, 190–198. doi: 10.1038/nri3386
Schielke, C. K. M., Burda, H., and Begall, S. (2012). Reproductive status does not influence activity budgets of eusocial Ansell’s mole-rats, Fukomys anselli (Rodentia, Bathyergidae): a study of locomotor activity by means of RFID. Mamm. Biol. 77, 1–5. doi: 10.1016/j.mambio.2011.09.004
Schernhammer, E. S., and Schulmeister, K. (2004). Melatonin and cancer risk: does light at night compromise physiologic cancer protection by lowering serum melatonin levels? Br. J. Cancer 90, 941–943. doi: 10.1038/sj.bjc.6601626
Schmitz, L., and Motani, R. (2010). Morphological differences between the eyeballs of nocturnal and diurnal amniotes revisited from optical perspectives of visual environments. Vis. Res. 50, 936–946. doi: 10.1016/j.visres.2010.03.009
Schöttner, K., Oosthuizen, M. K., Broekman, M., and Bennett, N. C. (2006). Circadian rhythms of locomotor activity in the Lesotho mole-rat, Cryptomys hottentotus subspecies from Sani Pass, South Africa. Physiol. Behav. 89, 205–212. doi: 10.1016/j.physbeh.2006.06.014
Shah, P. N., Mhatre, M. C., and Kothari, L. S. (1984). Effect of melatonin on mammary carcinogenesis in intact and pinealectomized rats in varying photoperiods. Cancer Res. 44, 3403–3407.
Sharma, V. K. (2003). Adaptive significance of circadian clocks. Chronobiol. Int. 20, 901–919. doi: 10.1081/CBI-120026099
Shepard, A., and Kilssil, J. L. (2020). The use of non-traditional models in the study of cancer resistance—the case of the naked mole rat. Oncogene 39, 5083–5097. doi: 10.1038/s41388-020-1355-8
Shortridge, G. C. (1934). The Mammals of South West Africa: A Biological Account of the Forms Occuring in that Region. London: Carnegie Corporation of New York.
Sichilima, A. M., Bennett, N. C., and Faulkes, C. G. (2011). Field evidence for colony size and aseasonality of breeding and in Ansell’s mole-rat, Fukomys anselli (Rodentia: Bathyergidae). Afr. Zool. 46, 334–339. doi: 10.3377/004.046.0212
Sichilima, A. M., Faulkes, C. G., and Bennett, N. C. (2008). Field evidence for seasonality of reproduction and colony size in the Afrotropical giant mole-rat Fukomys mechowii (Rodentia: Bathyergidae). Afr. Zool. 43, 144–149. doi: 10.1080/15627020.2008.11657231
Šklíba, J., Lövy, M., Hrouzková, E., Kott, O., Okrouhlík, J., and Šumbera, R. (2014). Social and environmental influences on daily activity pattern in free-living subterranean rodents: the case of a eusocial Bathyergid. J. Biol. Rhythms 29, 203–214. doi: 10.1177/0748730414526358
Šklíba, J., Šumbera, R., Chitaukali, W. N., and Burda, H. (2007). Determinants of daily activity patterns in a free-living afrotropical solitary subterranean rodent. J. Mammal. 88, 1009–1016. doi: 10.1644/06-MAMM-A-235R1.1
Sohocki, M. M., Sullivan, L. S., Mintz-Hittner, H. A., Birch, D., Heckenlively, J. R., Freund, C. L., et al. (1998). A range of clinical phenotypes associated with mutations in CRX, a photoreceptor transcription-factor gene. Am. J. Hum. Genet. 63, 1307–1315. doi: 10.1086/302101
Sol Fanjul, M., Zenuto, R., and Busch, C. (2006). Seasonality of breeding in wild tuco-tucos Ctenomys talarum in relation to climate and food availability. Acta Theriol. 51, 283–293. doi: 10.1007/BF03192680
Spinks, A. C., Bennett, N. C., and Jarvis, J. U. M. (1999). Regulation of reproductive female common mole-rats (Cryptomys hottentotus hottentotus): the effects of breeding season and reproductive status. J. Zool. Lond. 248, 161–168. doi: 10.1111/j.1469-7998.1999.tb01192.x
Spinks, A. C., Bennett, N. C., Faulkes, C. G., and Jarvis, J. U. M. (2000). Circulating LH levels and the response to exogenous GnRH in the common mole-rat: implications for reproductive regulation in this social, seasonal breeding species. Horm. Behav. 37, 221–228. doi: 10.1006/hbeh.2000.1576
Spinks, A. C., Van der Horst, G., and Bennett, N. C. (1997). Influence of breeding season and reproductive status on male reproductive characteristics in the common mole-rat, Cryptomys hottentotus hottentotus. J. Reprod. Fertil. 109, 79–86. doi: 10.1530/jrf.0.1090079
Stal, L. J., and Krumbein, W. E. (1985). Nitrogenase activity in the non-heterocystous cyanobacterium Oscillatoria sp. Grown under alternating light-dark cycles. Arch. Microbiol. 143, 67–71. doi: 10.1007/bf00414770
Streicher, S., Boyles, J. G., Oosthuizen, M. K., and Bennett, N. C. (2011). Body temperature patterns and rhythmicity in free-ranging subterranean Damaraland mole-rats, Fukomys damarensis. PLoS One 6, e26346. doi: 10.1371/journal.pone.0026346
Šumbera, R. (2001). “The mating behaviour of the Silvery mole-rat (Heliophobius argenteocinereus),” in African Small Mammals / Petits Mammifères Africains, eds C. Denys, G. Laurent, and P. Alain (Paris: IRD Editions).
Šumbera, R. (2019). Thermal biology of a strictly subterranean mammalian family, the african mole-rats (Bathyergidae, Rodentia). J. Therm. Biol. 79, 166–189. doi: 10.1016/j.jtherbio.2018.11.003
Šumbera, R., Burda, H., and Chitaukali, W. N. (2003a). Reproductive biology of a solitary subterranean bathyergid rodent, the silvery mole-rat (Heliophobius argenteocinereus). J. Mammal. 84, 278–287. doi: 10.1644/1545-15422003084<0278:RBOASS<2.0.CO;2
Šumbera, R., Burda, H., Chitaukali, W. N., and Kubova, J. (2003b). Silvery mole-rats (Heliophobius argenteocinereus, Bathyergidae) change their burrow architecture seasonally. Naturwissenschaften 90, 370–373. doi: 10.1007/s00114-003-0439-y
Swade, R. H., and Pittendrigh, C. S. (1967). Circadian locomotor rhythms of rodents in the arctic. Am. Nat. 101, 431–466. doi: 10.1086/282510
Szél, Á, and Röhlich, P. (1992). Two cone types of rat retina detected by anti-visual pigment antibodies. Exp. Eye Res. 55, 47–55. doi: 10.1016/0014-4835(92)90090-f
Thomas, H. G., Bateman, P. W., Scantlebury, D. M., and Bennett, N. C. (2012a). Seasonal effects on digging activity and burrow architecture in the Cape dune mole-rat, Bathyergus suillus (Rodentia: Batherygidae). Afr. Zool. 47, 332–340. doi: 10.3377/004.047.0222
Thomas, H. G., Bateman, P. W., Scantlebury, M., Bennett, N. C., and Hayssen, V. (2012b). Season but not sex influences burrow length and complexity in the non-sexually dimorphic solitary Cape mole-rat (Rodentia: Bathyergidae). J. Zool. 288, 214–221. doi: 10.1111/j.1469-7998.2012.00944.x
Thomas, H. G., Scantlebury, D. M., Swanepoel, D., Bateman, P. W., and Bennett, N. C. (2013). Seasonal changes in burrow geometry of the common mole-rat (Rodentia: Bathyergidae). Sci. Nat. 100, 1023–1030. doi: 10.1007/s00114-013-1105-7
Thomas, H. G., Swanepoel, D., and Bennett, N. C. (2016). Burrow architecture of the Damaraland mole-rat (Fukomys damarensis) from South Africa. Afr. Zool. 51, 29–36. doi: 10.1080/15627020.2015.1128355
Tomotani, B. M., Flores, D. E., Tachinardi, P., Paliza, J. D., Oda, G. A., and Valentinuzzi, V. S. (2012). Field and laboratory studies provide insights into the meaning of day-time activity in a subterranean rodent (Ctenomys aff. knighti), the tuco-tuco. PLoS One 7:e37918. doi: 10.1371/journal.pone.0037918
Van Daele, P. A., Verheyen, E., Brunain, M., and Adriaens, D. (2007). Cytochrome b sequence analysis reveals differential molecular evolution in African mole-rats of the chromosomally hyperdiverse genus Fukomys (Bathyergidae, Rodentia) from the Zambezian region. Mol. Phylogenet. Evol. 45, 142–157. doi: 10.1016/j.ympev.2007.04.008
Van der Horst, G. (1972). Seasonal effects of anatomy and histology on the reproductive tract of the male rodent mole. Zool. Afr. 7, 491–520. doi: 10.1080/00445096.1972.11447458
Van der Merwe, I., Lukats, A., Blahova, V., Oosthuizen, M. K., Bennett, N. C., and Nemec, P. (2018). The topography of rods, cones and intrinsically photosensitive retinal ganglion cells in the retinas of a nocturnal (Micaelamys namaquensis) and a diurnal (Rhabdomys pumilio) rodent. PLoS One 13:e0202106. doi: 10.1371/journal.pone.0202106
Van der Walt, L., Bennett, N. C., and Schoeman, A. S. (2001). Reproductive suppressive and pituiary sensitivity to exogenous GnRH in the highveld mole-rat (Cryptomys hottentotus pretoriae). J. Zool. Lond. 254, 177–184. doi: 10.1017/S0952836901000681
Van Jaarsveld, B., Bennett, N. C., Hart, D. W., and Oosthuizen, M. K. (2019). Locomotor activity and body temperature rhythms in the Mahali mole-rat (C. h. mahali): the effect of ight and ambient temperature variations. J. Therm. Biol. 79, 24–32. doi: 10.1016/j.jtherbio.2018.11.013
Van Oort, B. E. H., Tyler, N. J. C., Gerkema, M. P., Folkow, L., Blix, A. S., and Stokkan, K.-A. (2005). Circadian organization in reindeer. Nature 438, 1095–1096. doi: 10.1038/4381095a
Varcoe, T. J., Wight, N., Voultsios, A., Salkeld, M. D., and Kennaway, D. J. (2011). Chronic phase shifts of the photoperiod throughout pregnancy programmes glucose intolerance and insulin resistance in the rat. PLoS One 6:e18504. doi: 10.1371/journal.pone.0018504
Vasicek, C. A., Malpaux, B., Fleming, P. A., and Bennett, N. C. (2005a). Melatonin secretion in the Mashona mole-rat, Cryptomys darlingi - influence of light on rhythmicity. Physiol. Behav. 83, 689–697. doi: 10.1016/j.physbeh.2004.09.011
Vasicek, C. A., Oosthuizen, M. K., Cooper, H. M., and Bennett, N. C. (2005b). Circadian rhythms of locomotor activity in the subterranean Mashona mole rat, Cryptomys darlingi. Physiol. Behav. 84, 181–191.
Vega-Zuniga, T., Medina, S., Marín, G., Letelier, J. C. A., Palacios, G., Němec, P., et al. (2017). Selective binocular vision loss in two subterranean caviomorph rodents: Spalacopus cyanus and Ctenomys talarum. Sci. Rep. 7:41704.
Vinogradova, I. A., Anisimov, V. N., Bukalev, A. V., Semenchenko, A. V., and Zabezhinski, M. A. (2009). Circadian disruption induced by light-at-night accelerates aging and promotes tumorigenesis in rats. Aging 1, 855–865.
Visser, M. E., Caro, S. P., van Oers, K., Schaper, S. V., and Helm, B. (2010). Phenology, seasonal timing and circannual rhythms: towards a unified framework. Proc. R. Soc. Lond. B Biol. Sci. 365, 3113–3127. doi: 10.1098/rstb.2010.0111
Visser, M. E., Holleman, L. J. M., and Caro, S. P. (2009). Temperature has a causal effect on avian timing of reproduction. Proc. R. Soc. Lond. B Biol. Sci. 276, 2323–2331. doi: 10.1098/rspb.2009.0213
Visser, M. E., Holleman, L. J. M., and Gienapp, P. (2006). Shifts in caterpillar biomass phenology due to climate change and its impact on the breeding biology of an insectivorous bird. Oecologia 147, 164–172. doi: 10.1007/s00442-005-0299-6
Wagner, H. J., Kemp, K., Mattheus, U., and Priede, I. G. (2007). Rhythms at the bottom of the deep sea: cyclic current flow changes and melatonin patterns in two species of demersal fish. Deep Sea Res. Part I 54, 1944–1956. doi: 10.1016/j.dsr.2007.08.005
Walls, G. L. (1942). The Vertebrate Eye and its Adaptive Radiation. Bloomfield Hills, MI: Cranbrook Institute of Science.
Wang, X., Yu, C., Tzekov, R. T., Zhu, Y., and Li, W. (2020). The effect of human gene therapy for RPE65-associated Leber’s congenital amaurosis on visual function: a systematic review and meta-analysis. Orph. J. Rare Dis. 15:49. doi: 10.1186/s13023-020-1304-1
Ware, J. V., Rode, K. D., Robbins, C. T., Leise, T., Weil, C. R., and Jansen, H. T. (2020). The clock keeps ticking: circadian rhythms of free-ranging polar bears. J. Biol. Rhythms 35, 180–194. doi: 10.1177/0748730419900877
Warrant, E., and Locket, N. A. (2004). Vision in the deep sea. Biol. Rev. 79, 671–712. doi: 10.1017/S1464793103006420
Wehr, T. A. (1997). Melatonin and seasonal rhythms. J. Biol. Rhythms 12, 518–527. doi: 10.1177/074873049701200605
Williams, J. A., and Sehgal, A. (2001). Molecular components of the circadian system in Drosophila. Annu. Rev. Physiol. 63, 729–755. doi: 10.1146/annurev.physiol.63.1.729
Williams, S. E., Shoo, L. P., Isaac, J. L., Hoffmann, A. A., and Langham, G. (2008). Towards an integrated framework for assessing the vulnerability of species to climate change. PLoS Biol. 6:e325. doi: 10.1371/journal.pbio.0060325
Wollnik, F. (1989). Physiology and regulation of biological rhythms in laboratory animals: an overview. Laboratory animals 23, 107–125. doi: 10.1258/002367789780863538
Wollnik, F., and Schmidt, B. (1995). Seasonal and daily rhythms of body temperature in the European hamster (Cricetus cricetus) under semi-natural conditions. J. Comp. Physiol. B 165, 171–182. doi: 10.1007/BF00260808
Wyatt, J. K., Ritz-De Cecco, A., Czeisler, C. A., and Derk-Jan Dijk, D.-J. (1999). Circadian temperature and melatonin rhythms, sleep, and neurobehavioral function in humans living on a 20-h day. Am. J. Physiol. 46, R1152–R1163. doi: 10.1152/ajpregu.1999.277.4.R1152
Yamanaka, Y., Hashimoto, S., Masubuchi, S., Natsubori, I., Nishide, S.-Y., Honma, S., et al. (2014). Differential regulation of circadian melatonin rhythm and sleep-wake cycle bybright lights and nonphotic time cues in humans. Am. J. Physiol. 307, R546–R557. doi: 10.1152/ajpregu.00087.2014
Yoo, S.-H., Yamazaki, S., Lowrey, P. L., Shimomura, K., Ho, C. H., Buhr, E. D., et al. (2004). PERIOD2::LUCIFERASE real-time reporting of circadian dynamics reveals persistent circadian oscillations in mouse peripheral tissues. Proc. Natl. Acad. Sci. U.S.A. 101, 5339–5346. doi: 10.1073/pnas.0308709101
Zhou, X., Dou, Q., Fan, G., Zhang, Q., Sanderford, M., Kaya, A., et al. (2020). Beaver and naked mole rat genomes reveal common paths to longevity. Cell Rep. 32:107949. doi: 10.1016/j.celrep.2020.107949
Zubidat, A. E., Nelson, R. J., and Haim, A. (2009). Photosensitivity to different light intensities in blind and sighted rodents. J. Exp. Biol. 212(Pt 23), 3857–3864. doi: 10.1242/jeb.033969
Keywords: Bathyergidae, circadian rhythm, seasonal rhythm, rhythmicity, light, temperature, social, solitary
Citation: Oosthuizen MK and Bennett NC (2022) Clocks Ticking in the Dark: A Review of Biological Rhythms in Subterranean African Mole-Rats. Front. Ecol. Evol. 10:878533. doi: 10.3389/fevo.2022.878533
Received: 18 February 2022; Accepted: 30 March 2022;
Published: 22 April 2022.
Edited by:
Wayne Iwan Lee Davies, Umeå University, SwedenReviewed by:
Konrad Schoettner, Concordia University, CanadaPascal Malkemper, Max Planck Institute for Neurobiology of Behavior – Caesar, Germany
Copyright © 2022 Oosthuizen and Bennett. This is an open-access article distributed under the terms of the Creative Commons Attribution License (CC BY). The use, distribution or reproduction in other forums is permitted, provided the original author(s) and the copyright owner(s) are credited and that the original publication in this journal is cited, in accordance with accepted academic practice. No use, distribution or reproduction is permitted which does not comply with these terms.
*Correspondence: Maria K. Oosthuizen, bW9vc3RodWl6ZW5Aem9vbG9neS51cC5hYy56YQ==