- Animal Flight Laboratory, Department of Evolutionary and Environmental Biology and Institute of Evolution, University of Haifa, Haifa, Israel
Fuel deposition rate is predicted to determine departure fuel load during stopover in two models of optimal behavior of migrating birds. Yet, near ecological barriers, such as wide deserts, birds may switch to a different strategy of departing with just enough fuel to enable the long cross-barrier flight, thus reaching a threshold of fuel load regardless of the rate of fuel deposition. To test these predictions we studied autumn migrating Red-backed Shrikes (Lanius collurio) before they departed for a ∼2,000 km journey across the Sahara Desert. The body mass of fourteen individuals was measured on a daily basis throughout their stopover using field-deployed scales while being tracked by the ATLAS biotelemetry system in the Hula Valley, Israel. Statistical analysis found that the natural log of departure fuel load was positively related to both the capture fuel load and the fuel deposition rate. Hence, the results of this analysis suggest that bird condition at departure depended on the rate of fuel deposition, as predicted by models of time-minimization migration and the minimization of the total energy cost of migration. Departure fuel load and stopover duration were negatively related to each other as birds that remained for a long time in stopover departed with relatively low fuel loads. These findings suggest that even near a wide ecological barrier, departure fuel load is sensitive to the rate of fuel deposition, especially at lower values of fuel deposition rate. Birds that were able to accumulate fuel at higher rates showed a nearly constant departure fuel load and as such we could not exclude the possibility that the birds were trying to reach a certain threshold of fuel stores. Randomized 1,000 repeats of the aforementioned correlation suggest that the correlation between fuel deposition rate and the log of departure fuel load is valid and does not represent a spurious result. Following bird migration simulation using the program Flight, we conclude that fuel loads allowed most individual to accomplish the journey across the desert. Our findings suggest high between-individual variation in stopover parameters with likely consequences for bird migration performance and survival.
Introduction
The migration journey of most migratory birds is made from alternating phases of cross-country flights and stopovers. The cross-country flight of passerines usually takes place during nighttime, and the stopover may last several days and even several weeks (Carpenter et al., 1993; Schaub and Jenni, 2001). During stopover, migratory birds accumulate fuel (mostly lipids) to enable the following flight and to safeguard against starvation (Alerstam and Lindström, 1990; McWilliams et al., 2004; Kobylkov et al., 2014; Guglielmo, 2018). Yet, the stopover period may have several additional functions that may affect bird fitness, including physiological recovery from enduring flight and avoidance of inclement meteorological conditions during flight (Schmaljohann et al., 2022). How long to stay in a stopover site and how much fuel to deposit before departing from the site are two related questions with fitness consequences. This is due to the effects of migratory time schedules and fuel storage on bird survival during the journey and their carry over effects on reproduction (Both and Visser, 2001; Studds and Marra, 2005; Drent et al., 2006; Jonzén et al., 2007; Bauer et al., 2008; Møller et al., 2008; Gill et al., 2009). Consequently, it has been proposed that migrating birds behave in an optimal way such that their stopover duration is adjusted to maximize their fitness (Alerstam and Lindström, 1990).
Several different models have been developed to predict bird behavior during stopover. Specifically, Hedenström and Alerstam (1997) suggested that two models, namely time-minimization migration and minimization of the total energy cost of migration, predict a positive, non-linear, diminishing effect of fuel deposition rate on departure fuel load. These relationships were empirically supported by many field studies (e.g., Lindström and Alerstam, 1992; Bayly, 2006; Schmaljohann and Eikenaar, 2017) in different parts of the world (primarily in Western Europe), as reviewed by Alerstam (2011). These two models suggest that the bird’s overall migration speed relates to its fuel deposition rate and as such fuel deposition rate determines the optimum departure fuel load. Time-minimization migration theory suggests that birds are sensitive to the overall duration of the migration. Consequently, having the highest overall speed of migration is beneficial. Since the speed of migration is determined by both flight times and stopover times, the latter being the larger component (about 85% or higher) of the total migration duration (Hedenström and Alerstam, 1997), quick departure from stopover sites is expected to have the largest effect on the overall speed of migration. Minimizing the total energy cost of migration is expected to depend on the fueling conditions along the migration journey. Migrating birds adopting one of these behavioral strategies are expected to exhibit a positive relationship between fuel deposition rate and departure fuel load, but this relationship is expected to be steeper according to the former theory.
A different model is the minimization of the energy cost of transport during migration (Alerstam and Lindström, 1990; Hedenström and Alerstam, 1997). This model predicts constant departure fuel load, regardless of fuel deposition rate. Thus, birds adopting this strategy are expected to fuel to a certain fuel load that would allow them to reach the next stopover site with minimal energetic costs, and avoid the high metabolic cost of carrying a heavy load, which is predicted according to the time-minimization strategy (Alerstam and Lindström, 1990). Notably, Erni et al. (2002) simulated a simple behavioral rule with similar outcomes. In this simulation, birds departed from the stopover site when their fuel load was equal or exceeded a certain threshold. Thus, these two models predict no positive relationship between departure fuel load and fuel deposition rate as birds are predicted to depart regardless of fuel deposition rate. When the next stopover site is located far away from the site of departure, birds departing for migration may exhibit a threshold departure fuel load allowing them to reach the next site. Reaching a threshold of fuel load is essential under some circumstances in which the availability of suitable stopover habitats is limited. For example, migrating land-birds cannot usually alight in large water bodies (oceans and seas) and thus they require a certain amount of fuel to be able to cross them. Also, for some birds, suitable food could only be found in isolated stopover sites where birds may concentrate, as in the case of waders (e.g., Clark and Butler, 1999). Due to their extreme aridity, food availability in deserts is usually insufficient for fueling and consequently, when encountering a wide ecological barrier such as a wide desert, migrating birds must store sufficiently high amount of lipids to facilitate the crossing of the barrier (Fransson et al., 2001). Consequently, birds facing the 1,800 wide Sahara Desert (Moreau, 1972) are expected to store high fuel loads before departing for a cross-desert journey (Rubolini et al., 2002). Following the predictions of the energy minimization model (Alerstam and Lindström, 1990; Hedenström and Alerstam, 1997) and the threshold fuel load model (Erni et al., 2002; see also Schaub et al., 2008), we predict an alternative fuel loading behavior of threshold fuel loading, in which birds accumulate fuel to a threshold level that will enable the crossing of the barrier. This hypothesis proposes a rather constant departure fuel load among different individuals that need to fly across the same wide barrier and no positive relationship between fuel deposition rate and departure fuel load during stopover at the desert’s edge.
Calculating the threshold fuel load required for a certain migration could be done using range equations (e.g., Alerstam and Lindström, 1990; Gudmundsson et al., 1991), while a more comprehensive approach was proposed by Pennycuick (1998). This latter method, which was also distributed in a computer simulation program named Flight (Pennycuick, 2008), allows taking into account many different factors related to bird morphology, body mass, physiology, including lipid stores, and environmental conditions, which are known to affect flight performance. This method has been applied for studying bird migration, including over wide barriers (e.g., Pennycuick and Battley, 2003). Thus, using this program to simulated bird flight across the Sahara Desert and comparing model predictions with empirical data collected in the field can enhance our understanding of bird migration.
Another important component of stopover ecology is the duration of stopover. While no formal theoretical predictions have been outlined in studies that developed optimal time- and energy-minimization models, with regard to how stopover duration is expected to be optimized in relation fuel deposition rate, a graphical model presented as part of the time-minimization model (Figure 2 in Alerstam and Lindström, 1990; Figure 1 in Hedenström and Alerstam, 1997) provides predictions that can be deduced from the graph. Accordingly, when the overall migration time is minimized (speed of migration is maximized), stopover duration is expected to decrease with increasing fuel deposition rate as birds are expected to leave a stopover site sooner upon depositing a higher fuel load. Alternatively, Erni et al. (2002) proposed a stopover strategy of constant stopover duration. According to this latter model, the birds are not expected to vary their stopover duration in relation to habitat quality, as manifested in their fuel deposition rate. Hence, no relationship (either negative or positive) is expected between fuel deposition rate and stopover duration.
We studied migrating Red-backed Shrikes (Lanius collurio) while stopping-over during autumn in the Hula Valley, Israel. This stopover area is located about 150 km north of the northern fringes of the 1,800 km wide Saharo-Arabian desert belt (Moreau, 1972). By assuming a nocturnal flight range of at least 200 km, it is likely that birds will enter the desert during their first migration night after departing from the area. We measured daily body mass using field-deployed scales and tracked bird movement by a recently developed automatic telemetry system (Advanced Tracking and Localization of Animals in real-life Systems, ATLAS; Toledo et al., 2020) to determine their departure timing (see example in Supplementary Figure 1). The moment of departure was determined as the time the bird had initiated a flight due south that continued in this general direction until the signal was lost, 10–25 km from the point of departure (due to limited coverage of the telemetry system). Moreover, the ATLAS allowed following the birds throughout the stopover duration in detail, and, importantly, enabled identification of departure flights as opposed to local flights and movement to other feeding territories in the area. We used body mass and departure timing data to determine if bird stopover behavior, and specifically the relationship between bird fuel deposition rate and departure fuel load, as well as the relationship between fuel deposition rate and stopover duration, followed the predictions of the afore-mentioned models. Due to the scarcity of field studies that are conducted before the crossing of wide ecological barriers and specifically before the crossing of the Sahara Desert, our work is important for exploring how birds manage their fuel deposition and stopover duration in these ecological circumstances that are relevant to many long-distance migrants that move between the Western Palearctic and the Afro-tropical regions.
Materials and methods
Field measurements
The Red-backed Shrike (L. collurio) is a long-distance, nocturnal migrant that breeds in Europe and Western and Central Asia and over-winters in Eastern and Southern Africa. This predatory (mostly of insects and small vertebrate) bird species uses perches when foraging, and it is known as territorial during different times of the year, including while stopping-over (Lindström, 1995; Shirihai, 1996). The characteristic foraging of this species that includes repeated feeding bouts from perches allows direct observations of bird behavior and measuring bird body mass (Lindström, 1995). During autumn, many Red-backed Shrikes stop-over in the Hula Valley (33°06′N/35°36′E), a major stopover site for birds that travel between Africa, Europe, and Asia (Shirihai, 1996; Newton, 2007). Consequently, our field work took place in the Hula Valley during September and October of 2017 and 2018. Since the Red-backed Shrike is a fairly rare migrant in the country during spring, we could not study its stopover behavior during this period.
Many Red-backed Shrike individuals arriving at the Hula Valley use fences surrounding agricultural fields as vantage points and establish territories during their stopover. We undertook surveys in two predesignated areas just north and south of the Agamon Hula wetland within the valley where we inspected fences in an agricultural area twice a day, in the morning and evening (see additional details below). The northern area had a total length of 4.3 km of its perimeter and the southern transect had a total length of 3.6 km (Supplementary Figure 2). During the surveys we recorded the localities of Red-backed Shrike territories. Individuals that occupied specific sites that were devoid of shrikes in the previous day were considered as newly arriving birds and were the target of our field measurements. While we could not study bird movement before the birds were actually present at their stopover sites, showing faithfulness to it over time, we noted that arriving birds were usually anxious and behaved in an erratic way. After a while, most birds became much less erratic and occupied a territory which they usually held during their entire stopover period. After locating candidate birds for the study, we initiated a habituation procedure that lasted 2–4 days depending on the behavior of each individual and then started the routine measurement of the body mass of each individual until it departed from the area due south, as confirmed by the ATLAS (see example in Supplementary Figure 1). Birds that moved from their territories during their stopover within the valley as determined by data from the ATLAS, as well as those flying from the valley in a reverse direction (e.g., due north) were excluded from the analysis. Thus, the use of ATLAS facilitated a highly detailed tracking of each individual, which is rarely the case in studies of bird stopover, allowing us to include in the data only birds that were tracked on a daily basis throughout the period between their capture and departure.
For each bird we explored its departure track that was 10–25 km long, to ascertain that the bird indeed departed from the area toward the seasonally appropriate migration direction. No bird that has departed southward returned later to the area, according to data from the ATLAS. A description of all the birds’ departure flights is currently in preparation (Zinßmeister et al., in preparation). We note that unlike many other field studies of passerine stopover fuel deposition in which bird movement was not monitored at all (Lindström and Alerstam, 1992; Dierschke et al., 2005; Schmaljohann and Dierschke, 2005; Bayly, 2006; Schmaljohann et al., 2013; and many more – reviewed in Schmaljohann and Eikenaar, 2017) or was monitored over a coarse spatial resolution or at low frequency (Goymann et al., 2010; Schmaljohann et al., 2011; Taylor et al., 2011; Crysler et al., 2016—see the review of Schmaljohann and Eikenaar, 2017), our work is likely the most detailed of its kind, as we closely followed the movement of each bird from capture until the transmitter’s signals were lost following its departure, at least 10 km after the start of its migratory flight.
Following a preliminary fieldwork in autumn 2016, we did not trap a bird before it was habituated to the feeding station as doing so may cause avoidance behavior toward the feeding station, even though traps and feeding stations were never presented simultaneously to the birds. In each territory we placed a feeding station that was positioned near the main perch of the bird. The feeding station consisted of a glass bowl placed on a 20 cm × 20 cm plate of measuring scales (FMW-AFW, Larit Measurements Ltd., Ra’anana, Israel) with a 0.1 g resolution (Figure 1). To attract the birds to the station we used two superworms (Zoophobas morio; average 0.61 g/individual, consisting 57.9% water, 19.7% protein, and 17.7% lipid; Finke, 2002) as bait within the bowl each morning and afternoon to facilitate body mass measurement remotely by the scales. We note that based on our observations, this supplementary food was not a major food source of the birds, as birds continued to forage for extended periods after consuming the worms, and their daily body mass increase could not be attributed to this rather minor food source. As soon as birds showed high tendency to use the feeding station, the habituation period ended and the birds were trapped using spring traps (trap model TSB30N, Moudry-traps, Czechia) baited with Superworms. The traps were never deployed when the shrikes were near the bowl and were not positioned near the locality of the bowl to disassociate the trapping from the food source. If a trapping attempt did not succeeded immediately, usually that particular bird could not be trapped at all. Upon trapping, all individuals were ringed with a metal ring and a unique combination of color rings for individual identification in the field (Figure 1). Body mass, wing chord length, visual subcutaneous fat index (Kaiser, 1993), and age and the sex of adult birds (Svensson, 1992) were determined for each bird. In addition, several undertail coverts were plucked and used to determine the sex of first calendar year birds using molecular DNA testing (Karnieli Vet Ltd., Tivon, Israel).
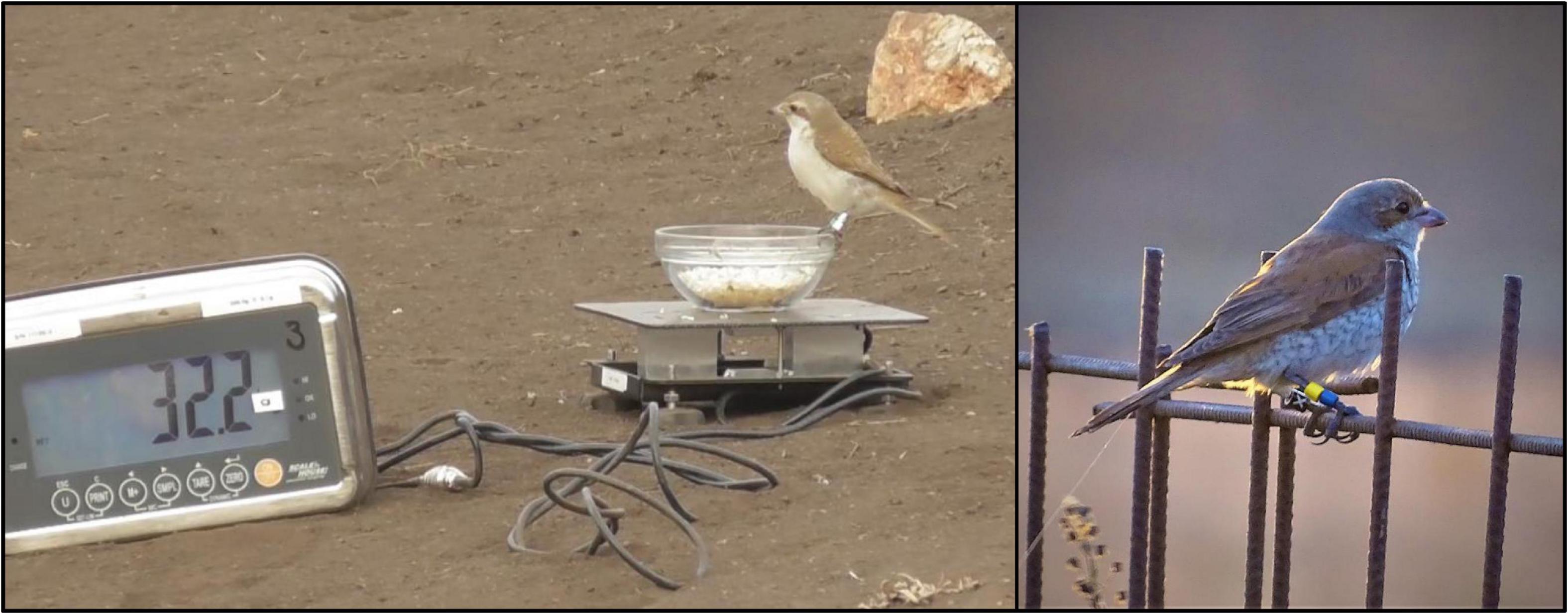
Figure 1. Field measurement and observation of Red-backed Shrikes during stopover at the study area in the Hula Valley, Israel. (Left) A shrike perching on the feeding station, allowing the observer to record its body mass throughout its stopover period. (Right) Adult female shrike (ring number AB47045) with color rings allowing individual identification, as well as ATLAS transmitter (see the antenna of the transmitter near the tail of the bird) to record its movement during the stopover period and at departure from the area.
After completing bird sampling and measurements, we fitted the bird with an ATLAS tag (Toledo et al., 2020). The tags weighed on average 4.7% of bird body mass at capture with tag mass range of 1.35–1.85 g which was 3.8–5.9% of bird body mass. The tags weighed on average 4.0% of bird body mass at departure from the stopover site (range 3.4–4.9% of bird body mass (Macdonald and Amlaner, 1980; Kenward, 1987; Naef-Daenzer, 1993). The tags were attached to the birds using a harness that was individually fitted to each bird (modified from the harness described by Naef-Daenzer, 2007). The harness consisted of a rubber band that expanded with body motion and fell off within 1 month from the time of deployment due to temperature changes that degrade the rubber with time. We thoroughly tested the harness design using field observations and concluded that bird movement was not jeopardized or limited due to the harness. Birds were released back to their territories after a handling time of up to 20 min. Birds were examined thoroughly before released with a tag to make sure full motility is normal in the hand. After release birds were observed using binoculars from a nearby car. Usually the birds went to hide inside a bush or tree close by and after a certain time (usually within half an hour) returned to their main perch on the fence and resumed foraging activity. Some individuals went directly back to the fence after tagging, without any hiding, and behaved as if they were not affected at all by handling.
From the total number of birds that were trapped and tagged during 2017 (19 individuals) and 2018 (62 individuals), we were able to habituate a total of 36 individuals. Notably, of the 36 habituated birds, we were able to follow a total of 15 birds while the other 21 birds were not followed due to several reasons. One bird was predated by a Common Kestrel (Falco tinnunculus) while perching on the feeding station (D. Zinßmeister personal observation), three birds moved to areas where their body mass could not be measured until their departure and two birds had a decreasing body mass during their stopover to the extent that we removed their tags. In addition, transmission was lost entirely or during some part of the stopover period for a total of 15 birds due to self-removal of tags and harnesses by the birds, telemetry system failure or tag failure. We followed 15 individuals (3 in 2017 and 12 in 2008) throughout their entire stopover period until their departure. Since 14 of these birds were 1-year individuals and preliminary analysis suggested that the adult bird differed from the rest of the birds in several parameters of its stopover fuel deposition, we did not include it in this work.
Bird body mass was determined by directly observing the scales’ digital display by an observer throughout the migrants’ stopover period (Figure 1). The observer used binoculars while sitting in a car 20–30 m away from the scales. Only data recorded in the first moment the bird perched motionless on the bowl were used in the analysis. Body mass measurements took place twice a day between 6:00 and 10:00 a.m. and between 4:00 and 8:00 p.m. local time. During these times of the day the birds showed high foraging activity and were inclined to feed on the scale. Recorded data per session were bird ID, time, bird body mass, and number of superworms eaten during the feeding session and number of superworms eaten before the weight was determined. We used these data to determine bird body mass at the day of trapping and later throughout the course of the bird’s stopover (Lindström and Alerstam, 1992; Fransson, 1998; Schmaljohann and Dierschke, 2005; Bayly, 2006). We noted that many of the birds exhibited a bi-phasic fuel loading pattern (e.g., Carpenter et al., 1993), with stable or decreasing body mass in the beginning of their staging, followed by an increase of their body mass until departure (see examples in Supplementary Figure 3). We converted bird body mass measurements to fuel loads (see details below) in order to express variation in body mass in relation to lean, fuel-free body mass in percentages, following a large number of studies that used this methodology (e.g., Lindström and Alerstam, 1992; Dänhardt and Lindström, 2001; Schmaljohann and Dierschke, 2005; Bayly, 2006; Delingat et al., 2008). Fuel deposition rate was calculated as the total change in fuel load (derived from body mass measurements) between the date of bird trapping and the date of bird departure for migration, divided by the number of days the bird was stopping-over in the area. Obviously, this estimate does not include data from the period prior to capture. The calculation of departure fuel load was based on evening body mass measurements in 10 individuals. We could not obtain evening body mass measurements from the remaining four birds and consequently used their morning body mass. We did not interpolate the evening body mass based on morning body mass as changes in body mass between morning and evening at the day of departure were highly variable and inconsistent between individuals [range: (−0.7)–1.4 g, mean ± S.D. difference: 0.36 ± 0.72, paired t-test, t6 = 1.31, p = 0.239].
The ATLAS was used to track the birds’ movement. ATLAS is a highly accurate, automated radio-telemetry system that is based on time-difference-of-arrival and records animal position once in 1–8 s with a spatial accuracy of about 10 m (Toledo et al., 2020). A network of ATLAS stations (10 stations in 2017 and 11 in 2018) were deployed in the surroundings of the Hula Valley, recording multiple tagged individuals over an area of about 15 km × 30 km, covering the whole stopover area. Departure tracks of migrating birds were recorded beyond the regular reception coverage area of the system in the Hula Valley due to improved reception of tags carried by high flying birds during departure (Supplementary Figure 1). The transmitters used in the present study transmitted once every 8 s.
Following bird tagging and tracking by the ATLAS, we calculated the minimum stopover duration of each bird, which was defined as the number of days a bird spent in the Hula Valley from the day of trapping until it departed for migratory flight. Since all except two individuals underwent habituation prior to trapping, the actual stopover duration is about two to four days longer than the minimum stopover duration. The habituation days were not included in this calculation to avoid errors due to bird misidentification of specific individuals before the individuals were marked and tagged. The date and time of departure of each individual was determined by the ATLAS (Supplementary Figure 1).
Data analysis
To calculate fuel load of autumn migrating Red-backed Shrike individuals at the study area, we used body mass and wing chord data from individuals trapped at the Hula Valley bird ringing station during autumn in the years 2005–2018. Of these, 187 birds had no signs of visible fat and can thus be regarded as lean birds. To test if bird size affected its body mass in this group of lean birds, we applied linear regression and found that wing chord length did not significantly affect bird body mass (R2 = 0.007, p = 0.261). We consequently considered the average body mass (25.55 g) of this group of birds as the lean body mass of the Red-backed Shrike individuals. Using this value, we calculated fuel loads, which are expressed in percentages relative to this reference value (for example, a shrike with a body mass of 30.00 g is considered to have a fuel load of 17.4%).
To calculate the fuel load required for a successful crossing of the Sahara, we estimated that a bird departing from the Hula Valley will usually not be able to feed in the coming 2,000 km of its journey. Using the program Flight (version 1.25 by Colin Pennycuick) we simulated the migration of a Red-backed Shrike that was trapped in autumn 2018 in the Carmel Coast, about 90 km southwest of the Hula Valley (see Supplementary material). This individual, which was measured during a field course, was a 1-year bird. Using Flight, we estimated that the bird will need 6.9 g of lipids to cover a distance of 2,005 km. This added lipid mass corresponds to a departure fuel load of 27%. We explored the birds’ fuel deposition by comparing capture fuel loads (i.e., fuel load when captured) and departure fuel loads using a paired t-test. Then, we tested if bird stopover parameters (capture fuel load, fuel deposition rate, departure fuel load, and stopover duration) differed between male and female birds using independent samples t-tests. For exploring the effects of fuel deposition rate on departure fuel load and stopover duration, we tested the normality of the latter two variables and found that they indeed were normally distributed (Shapiro–Wilk tests; p = 0.850, 0.266, respectively). We used generalized linear modeling (GLM, Gaussian family linear link function) for testing, separately, two dependent factors: the natural log of departure fuel load (according to the expected relationship in Figure 3 in Hedenström and Alerstam, 1997) and stopover duration using the R package “lme4” (Bates et al., 2015). For each of the two dependent variables we tested the AICc (the Akaike information criterion, modified for small sample sizes; Akaike, 1987) of five different models (no explanatory factor, one of each explanatory factor, two explanatory factors and two explanatory factors and their interaction). We selected a specific model only if its ΔAICc was > 2.00 compared to other models. Further, we report significant terms in the models and their R2. We applied Pearson correlation to test if the two explanatory factors correlate with each other and also to examine if departure fuel load and stopover duration were correlated. All tests were two-tailed tested with α of 0.05. The analyses were done using SPSS (version 25, IBM, Armonk, NY, United States) and R (version 3.6.1, R Development Core Team).
Yet, one has to bear in mind that the results of these analyses could produce spurious results (Williams et al., 2022). This is because fuel deposition rate, an independent factor in the analyses, is calculated using both departure fuel load and stopover duration, which are dependent factors in the same statistical models. Thus, these calculations could result in spurious relationships and thus a statistical approach that will take these inherent relationships into account is required. In order to determine if the observed correlations between fuel deposition rate and the response variables (departure fuel load and stopover duration) is different from those expected by chance, we applied a randomization test (Jackson et al., 1990). We simulated 20 values of each of the following variables: departure fuel load and capture fuel load. These values were generated using their mean and standard deviation based on the normal distribution. We additionally simulated 20 values of the variable stopover duration, based on uniform distribution of integer numbers truncated between the minimum (5 days) and maximum (31 days) values recorded in the field. Then, we calculated the correlation between fuel deposition rate and the natural log of departure fuel load and the correlation between fuel deposition rate and stopover duration. This procedure was repeated 1,000 times, yielding two distributions of 1,000 correlation coefficient values, each for the two explored relationships. We present the two distributions of the correlations in the Supplementary material, as well as the R code used to produce the distribution of 1,000 correlations between the natural log of departure fuel load and fuel deposition rate. Based on the formula used for calculating fuel deposition rate, the relationship between log departure fuel load and fuel deposition rate is expected to be positive, and we consequently considered the top 5% of the distribution as the threshold of the rejection area at 95% confidence. Since the relationship between stopover duration and fuel deposition rate is expected to be negative, we considered the lowest 5% of the distribution as the rejection area threshold at 95% confidence.
Results
We studied a total of 14 first-year birds (bird details are in Supplementary Table 1) that were followed in the field throughout their stopover period in 2017 (3 birds) and 2018 (11 birds). Mean ± SD and the range of bird capture fuel load, departure fuel load, fuel deposition rate and stopover duration are provided in Table 1. We compared same-bird capture and departure fuel loads and found that all the birds departed with higher fuel load than their capture fuel load, and this difference was statistically significant [paired t-test, mean ± SD difference: 16.02 ± 10.31%, t13 = (5.814), p < 0.0001]. No differences in stopover parameters (capture fuel load, departure fuel load, fuel deposition rate, and stopover duration) were found between male (N = 8) and female (N = 6) birds (independent samples t-tests; t12 = 0.83, p = 0.42; t12 = 0.40, p = 0.97; t12 = 0.74, p = 0.48; t12 = 0.90, p = 0.39, respectively). We found that both capture fuel load and fuel deposition rate [which were not correlated to each other: Pearson correlation; r = (−0.33), p = 0.250], affected positively on departure fuel load (Table 2 and Figure 2), and that these two factors affected negatively on minimal stopover duration (Table 3 and Figure 2). Departure fuel load and minimal stopover duration were negatively correlated [Pearson correlation; r = (−0.61), p = 0.021; Figure 2].
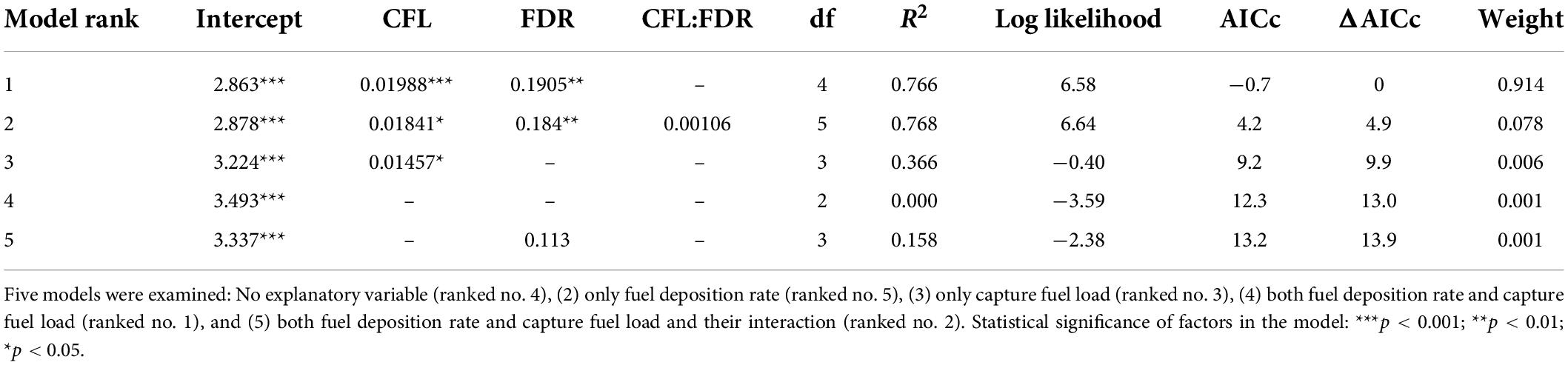
Table 2. Model selection table that outlines the effects of capture fuel load (CFL) and fuel deposition rate (FDR) on the natural log of departure fuel load.
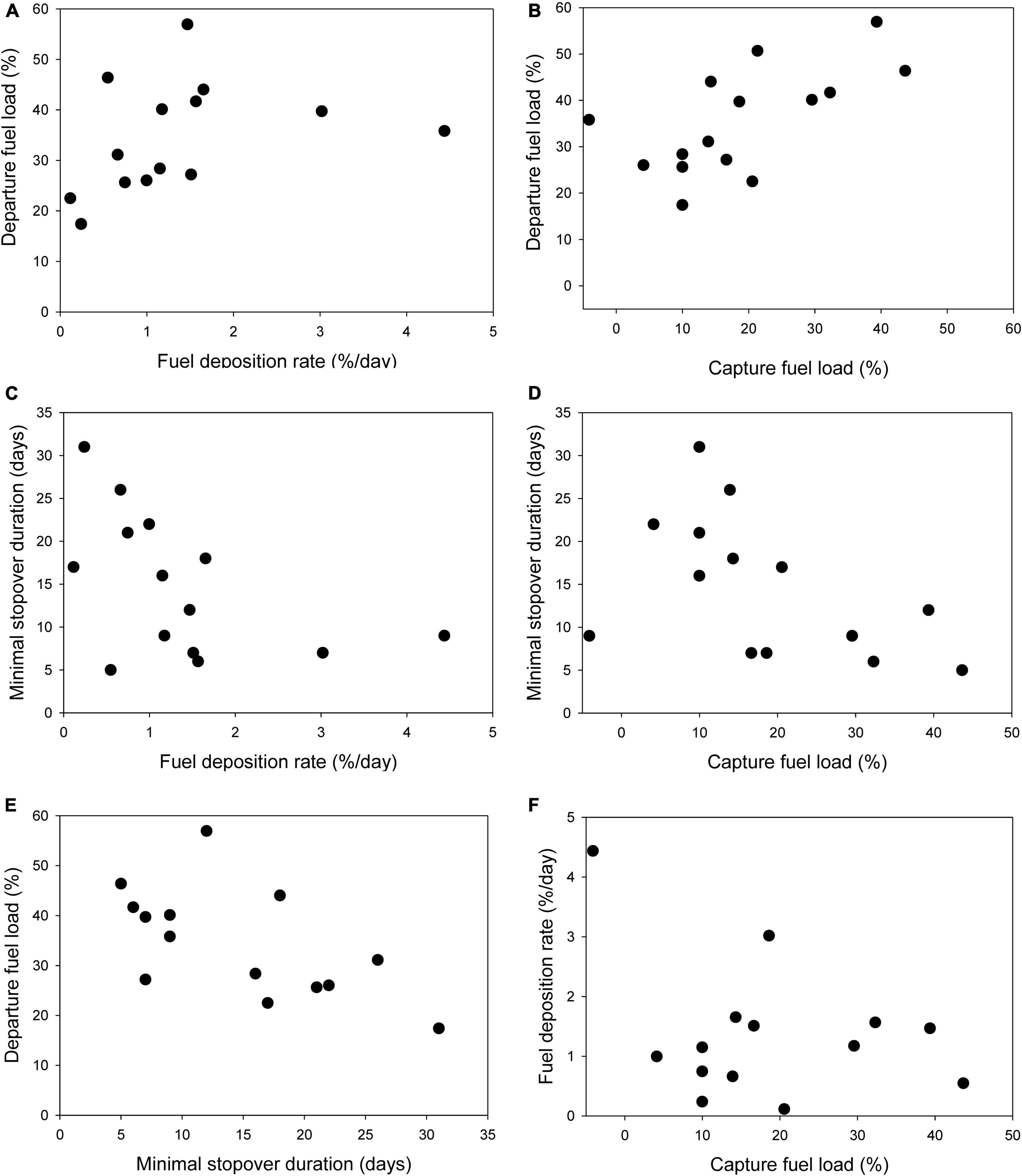
Figure 2. The relationships between different stopover parameters of autumn migrating Red-backed Shrikes at the Hula Valley, Israel. (A) Fuel deposition rate and departure fuel load. (B) Capture fuel load and departure fuel load. (C) Fuel deposition rate minimal and stopover duration. (D) Capture fuel load and minimal stopover duration. (E) Minimal stopover duration and departure fuel load. (F) Capture fuel load and fuel deposition rate.
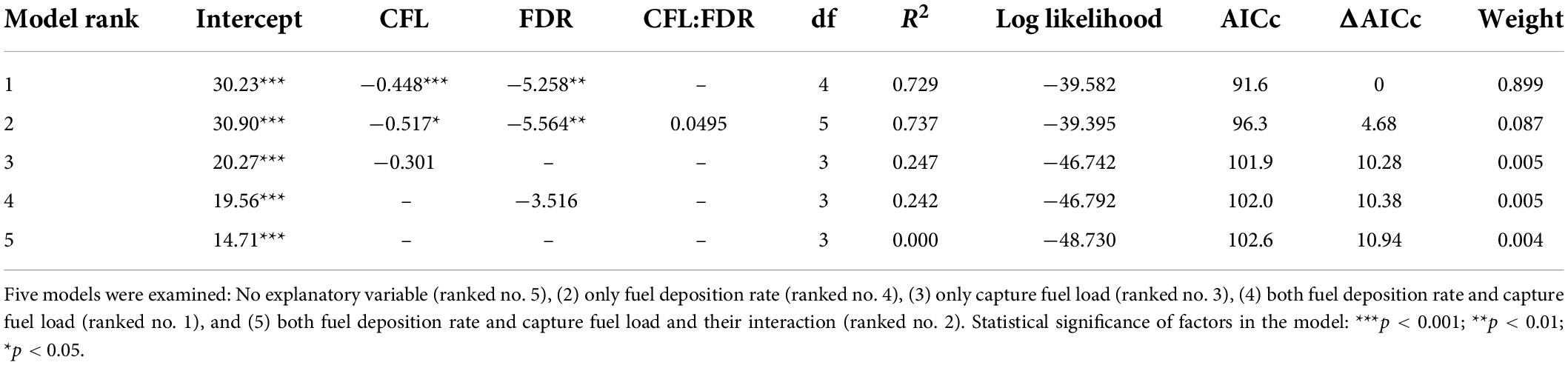
Table 3. Model selection table that outlines the effects of capture fuel load (CFL) and fuel deposition rate (FDR) on minimal stopover duration.
Histograms of the distributions of the simulated correlations between log departure fuel load and fuel deposition rate and between minimal stopover duration and fuel deposition rate are presented in the Supplementary material (Supplementary Figures 4,5, respectively). Since the observed correlation between fuel deposition rate and the natural log of departure fuel load is r = 0.398, and the upper 5% rejection area value of the distribution of 1,000 randomized correlation is r = (−0.401), we conclude that the observed correlation is within the 5% rejection region and thus this correlation is meaningful and allows inferences regarding the relationship between the variables. Regarding the relationship between fuel deposition rate and stopover duration, the observed correlation is r = (−0.492) and the lower 5% rejection is r = (−0.584). Therefore, the observed correlation is not within the rejection area and the correlation is thus spurious and should be ignored. Thus, we conclude that stopover duration is not related to fuel deposition rate despite the significant observed correlation.
Discussion
Our study combined repeated weighing of bird body mass using field-deployed scales with advanced biotelemetry to examine predictions of optimal migration theories. We investigated the relationships between departure fuel load, stopover duration and fuel deposition rate in a passerine species that used the Hula Valley near the northern fringes of the Sahara Desert for stopover during autumn migration. We found a positive relationship between fuel deposition rate and departure fuel load. These findings suggest that the birds behaved in ways predicted by the time-minimization migration theory or the minimization of the total energy cost of migration (Hedenström and Alerstam, 1997). Several empirical studies that were carried out mostly in temperate latitudes over the past three decades have generally found similar relationships (Lindström and Alerstam, 1992; Schmaljohann and Dierschke, 2005; Bayly, 2006; Delingat et al., 2008; but see Fransson, 1998; Dänhardt and Lindström, 2001). Notably, Schaub et al. (2008) demonstrated conformity to this prediction in two sites in Europe. Yet, in North Africa, where autumn migrants are about to cross the Sahara Desert, birds accumulate fuel to a threshold level. With regard to our findings, one must bear in mind that while the aforementioned relationship between fuel deposition rate and departure fuel load was significant, it was not steep. As clearly seen in Figure 2A, the relationship between fuel deposition rate and departure fuel load is rather constant at the middle and higher part of the range of fuel deposition rate values, albeit with some variation, which could be explained by between-individual differences in physiology or other migration-related properties (Piersma and Van Gils, 2011). At low values of fuel deposition rate, there is a strong positive relationship between fuel deposition rate and departure fuel load (Figure 2A). Therefore, it cannot be excluded that some of the birds try to reach a certain fuel load threshold, but fail to do it due to their lower rate of fuel deposition (see also Bulyuk and Tsvey, 2013). Following the randomization test we applied, our data cannot rule out the constant stopover duration model, proposed by Erni et al. (2002). Although stopover duration was highly variable between different individuals, the apparent correlation between it and fuel deposition rate might be the outcome of a spurious relationship, based on the randomization procedure.
Notably, the birds’ capture fuel load had a strong impact on both the departure fuel load and stopover duration. Birds that arrived with high fuel loads tended to depart with high fuel loads, suggesting that the process of accumulating fuel toward the enduring journey across the Sahara is starting well before the birds depart for the cross-desert flight (see also Rubolini et al., 2002). Unfortunately, no data exists for other localities north and south of the Hula Valley, which could reveal the spatial patterns of fuel deposition. Importantly, successful foraging that translated to high fuel deposition rates allowed the birds to depart for migration with high fuel reserves for the journey. Since capture fuel load and fuel deposition rate did not correlate, it seems that they had separate contribution to the observed variation in departure fuel load. Furthermore, it also suggests that good body condition at the start of the stopover does not predict bird performance during this period. Remarkably, even after long stopover periods and despite the increase in their fuel loads, some of the birds were still departing for migration with rather low departure fuel loads. Altogether, these findings suggest that both capture fuel load (Goymann et al., 2010; Schmaljohann and Eikenaar, 2017; Schmaljohann and Klinner, 2020) and fuel deposition rate (Lindström and Alerstam, 1992; Bayly, 2006; Delingat et al., 2008) have critical consequences for migration timing. These might translate to bird fitness by possibly affecting the chances to accomplish the cross-desert journey.
To the best of our knowledge, fuel deposition of migrating Red-backed Shrikes has not been studied yet elsewhere, precluding comparisons of our findings with those of others from the same species. In general though, passerines accumulate fuel at daily rates of 1–3% (Lindström, 2003), as found in our study. Interestingly, the shrikes’ average fuel deposition rate (1.4%) is identical to that reported for autumn migrating Robins (Erithacus rubecula) in the eastern Baltic Sea (Bulyuk and Tsvey, 2013). Comparison of our data with those reported from other passerine species about to cross the Sahara Desert, suggests that the Red-backed Shrike’s fuel deposition rate is similar to that reported from autumn migrating Reed Warbler (Acrocephalus scirpaceus) and Garden Warbler (Sylvia borin) in Southern Spain and lower that that reported from the northern part of North Africa (Schaub and Jenni, 2000). As can be expected, fuel deposition rates of Red-backed Shrikes found in our study are substantially lower than those reported for birds that were measured in the field and supplied with ad libitum food (Schmaljohann and Eikenaar, 2017). In addition, when comparing our findings to the expected maximal fuel deposition rates reported in Lindström (1991), we find that the bird with the highest average fuel deposition rate in our study accumulated fuel at about half the expected maximal rate. Since fuel deposition rates were averaged over the stopover period, data from specific days might be closer to theoretical expectations of maximum fuel deposition rates. Indeed, a bird with ring number AB47066 increased its body mass from 28.4 to 31.2 g (10.96% increase in fuel loading) in a single day, between 12 and 13 of October 2018. This value is expected for birds in the size of Red-backed Shrike Lindström (1991, 2003).
The departure fuel loads of the Red-backed Shrikes that were followed during their stopover were highly variable, ranging between 17.4 and 57% (Supplementary Table 1). The simulation results using the program Flight suggested that a fuel load of at least 27% is required for accomplishing the migration over the Sahara. Out of the 14 birds, eight had departure fuel loads well above this minimal value (31.1–57.0%), four had departure fuel load values slightly above or somewhat below this expected threshold (25.6–28.4%) and two birds had substantially lower fuel load at departure (17.4 and 22.5%). We do not have any information about the fate of these latter two birds, which preliminary analysis suggested that their departure properties (timing, direction, and speed) was similar to those of the birds with higher fuel load at departure. While birds that depart from the Hula Valley may stop-over in the following 150 km after their departure before entering the desert, some birds may even be able to stop-over within the desert in oases and other localities where food is available, allowing them to accomplish the cross-desert journey even if their fuel loads are low when departing for the desert crossing (Biebach, 1985).
Performance differences between individuals, which could affect their survival and reproduction, may be caused by various factors. While no differences were found between female and male birds, we did not have sufficient data to test possible age-related differences. Including additional adult birds in future studies exploring Red-backed Shrike stopover behavior may help resolve if adults perform better than first calendar year birds, as found in other migratory species (e.g., Woodrey and Moore, 1997; Jones, 2002; Rotics et al., 2016). We could not find any noticeable morphological differences between individuals that had variable stopover behavior parameters. Yet, such variation could result from factors that were not examined in this work, including deterioration of bird immune function and allocation of energy and resources for compensatory measures (Buehler and Piersma, 2008; Eikenaar and Hegemann, 2016), oxidative stress and investment in anti-oxidation mechanisms (Costantini et al., 2007; Isaksson et al., 2011; Jenni-Eiermann et al., 2014; Eikenaar et al., 2017), digestion physiology constraints (Karasov and Pinshow, 2000; Kvist and Lindström, 2000; McWilliams et al., 2004), competitive abilities and territoriality (Rappole and Warner, 1976; Bibby and Green, 1980; Carpenter et al., 1983; Stillman et al., 2000), as well as recovery from a previous enduring flight or fasting periods before arriving at the study area (Eikenaar et al., 2014). Furthermore, variation in stopover parameters could result from the response of the birds to environmental factors (Schmaljohann et al., 2013). We hope that future studies will allow testing these factors to uncover their influence on the shrikes and other birds during stopover and at their departure.
Our focal species, the Red-backed Shrike, is highly suitable for studying stopover behavior due its characteristic territorial behavior that facilitated repeated measurements in the same spot and its use of high perches that allowed observing it in the field (Lindström, 1995). Nevertheless, we were not able to follow many of the individuals that were attached with a transmitter in the field due to various technical issues (i.e., transmitter and system failure), bird behavior and movement (translocating to territories that were inaccessible to us and movement outside the area covered by the system, for example, due north across the border to Lebanon). This resulted in a rather modest sample size and an overall low rate of success in collecting data from the tagged individuals (see full information above).
Another potential issue could be the limited range of tracking of the birds after their departure. Although the shrikes departed at seasonally appropriate direction of migration (southward), our work cannot rule out that some of the birds terminated their nocturnal flight after a short while and stopped-over again before entering the desert. The recent deployment of another ATLAS system in the Harod Valley, 65 km south of the Hula Valley, might facilitate the detection of individuals departing from the Hula Valley during their nocturnal flight, partly alleviating this concern. The development of global tracking systems, such as the ICARUS (Jetz et al., 2022), which, in the future, might facilitate the tracking of small passerines, could revolutionize our understanding of bird migration biology by studying both stopover and migratory flight of the same individuals.
Data availability statement
The original contributions presented in this study are included in the article/Supplementary material, further inquiries can be directed to the corresponding author.
Ethics statement
Ethical review and approval was not required for the animal study because ethical approval is not mandatory for this kind of study that is not an invasive study. The study was approved by permits from the Nature and Parks Authority of Israel.
Author contributions
NS conceived the study. DZ collected data in the field and processed data. DZ, DT, and NS analyzed the data and run the simulations. DZ and NS wrote the manuscript. All authors contributed to the article and approved the submitted version.
Funding
This study was supported by the Israel Science Foundation (Grant 702/17).
Acknowledgments
We would like to thank Noa Valtzer, Emmanuel Lourie, Noa Fischer, and Yuval Cohen for field assistance, Yoni Vortman, Efi Naim, and Inbar Rubin for facilitating our work in the Agamon Hula agricultural areas, Sivan Toledo, Ran Nathan, Yotam Orchan, Sivan Margalit, Ingo Schiffner, and Yoav Bartan for facilitating the use of the ATLAS, Francis Argyle and Shai Agmon for assistance with bird ringing and field work, Sondra Turjeman for help with bird sex determination using molecular techniques and Motti Charter, Idan Barnea, Oren Reichmann, Dana Klein, and Anat Levy for technical and logistical assistance.
Conflict of interest
The authors declare that the research was conducted in the absence of any commercial or financial relationships that could be construed as a potential conflict of interest.
Publisher’s note
All claims expressed in this article are solely those of the authors and do not necessarily represent those of their affiliated organizations, or those of the publisher, the editors and the reviewers. Any product that may be evaluated in this article, or claim that may be made by its manufacturer, is not guaranteed or endorsed by the publisher.
Supplementary material
The Supplementary Material for this article can be found online at: https://www.frontiersin.org/articles/10.3389/fevo.2022.874923/full#supplementary-material
References
Alerstam, T. (2011). Optimal bird migration revisited. J. Ornithol. 152, 5–23. doi: 10.1007/s10336-011-0694-1
Alerstam, T., and Lindström, Å (1990). “Optimal bird migration: The relative importance of time, energy and safety,” in Bird Migration, ed. E. Gwinner (Berlin: Springer), 331–351. doi: 10.1007/978-3-642-74542-3
Bates, D., Mächler, M., Bolker, B., and Walker, S. (2015). Fitting linear mixed-effects models using lme4. J. Stat. Softw. 67, 1–48. doi: 10.18637/jss.v067.i01
Bauer, S., Van Dinther, M., Høgda, K. A., Klaassen, M., and Madsen, J. (2008). The consequences of climate-driven stop-over sites changes on migration schedules and fitness of Arctic geese. J. Anim. Ecol. 77, 654–660. doi: 10.HH/j.1365-2656.2008.01381.x
Bayly, N. J. (2006). Optimality in avian migratory fuelling behaviour: A study of a trans-Saharan migrant. Anim. Behav. 71, 173–182. doi: 10.1016/j.anbehav.2005.04.008
Bibby, C. J., and Green, R. E. (1980). Foraging behaviour of migrant Pied Flycatchers, Ficedula hypoleuca, on temporary territories. J. Anim. Ecol. 49, 507–521. doi: 10.2307/4260
Biebach, H. (1985). Sahara stopover in migratory flycatchers: Fat and food affect the time program. Experientia 41, 695–697. doi: 10.1007/BF02007727
Both, C., and Visser, M. E. (2001). Adjustment to climate change is constrained by arrival date in a long-distance migrant bird. Nature 411, 296–298. doi: 10.1038/35077063
Buehler, D. M., and Piersma, T. (2008). Travelling on a budget: Predictions and ecological evidence for bottlenecks in the annual cycle of long-distance migrants. Philos. Trans. R. Soc. B 363, 247–266. doi: 10.1098/rstb.2007.2138
Bulyuk, V. N., and Tsvey, A. (2013). Regulation of stopover duration in the European Robin Erithacus rubecula. J. Ornithol. 154, 1115–1126. doi: 10.1007/s10336-013-0981-0
Carpenter, F. L., Hixon, M. A., Beuchat, C. A., Russell, R. W., and Paton, D. C. (1993). Biphasic mass gain in migrant hummingbirds: Body composition changes, torpor, and ecological significance. Ecology 74, 1173–1182. doi: 10.2307/1940487
Carpenter, F. L., Paton, D. C., and Hixon, M. A. (1983). Weight gain and adjustment of feeding territory size in migrant hummingbirds. Proc. Natl. Acad. Sci. U.S.A. 80, 7259–7263. doi: 10.1073/pnas.80.23.7259
Clark, C. W., and Butler, R. W. (1999). Fitness components of avian migration: A dynamic model of Western Sandpiper migration. Evol. Ecol. Res. 1, 443–457.
Costantini, D., Cardinale, M., and Carere, C. (2007). Oxidative damage and anti-oxidant capacity in two migratory bird species at a stop-over site. Comp. Biochem. Physiol. C 144, 363–371. doi: 10.1016/j.cbpc.2006.11.005
Crysler, Z. J., Ronconi, R. A., and Taylor, P. D. (2016). Differential fall migratory routes of adult and juvenile Ipswich Sparrows (Passerculus sandwichensis princeps). Mov. Ecol. 4:3. doi: 10.1186/s40462-016-0067-8
Dänhardt, J., and Lindström, Å (2001). Optimal departure decisions of songbirds from an experimental stopover site and the significance of weather. Anim. Behav. 62, 235–243. doi: 10.1006/anbe.2001.1749
Delingat, J., Bairlein, F., and Hedenström, A. (2008). Obligatory barrier crossing and adaptive fuel management in migratory birds: The case of the Atlantic crossing in Northern Wheatears (Oenanthe oenanthe). Behav. Ecol. Sociobiol. 62, 1069–1078. doi: 10.1007/s00265-007-0534-8
Dierschke, V., Mendel, B., and Schmaljohann, H. (2005). Differential timing of spring migration in Northern Wheatears Oenanthe oenanthe: Hurried males or weak females? Behav. Ecol. Sociobiol. 57, 470–480. doi: 10.1007/S00265-004-0872-8
Drent, R. H., Fox, A. D., and Stahl, J. (2006). Travelling to breed. J. Ornithol. 147, 122–134. doi: 10.1007/s10336-006-0066-4
Eikenaar, C., and Hegemann, A. (2016). Migratory Common Blackbirds have lower innate immune function during autumn migration than resident conspecifics. Biol. Lett. 12:20160078. doi: 10.1098/rsbl.2016.0078
Eikenaar, C., Källstig, E., Andersson, M. N., Herrera-Dueñas, A., and Isaksson, C. (2017). Oxidative challenges of avian migration: A comparative field study on a partial migrant. Physiol. Biochem. Zool. 90, 223–229. doi: 10.1086/689191
Eikenaar, C., Klinner, T., de Lille, T., Bairlein, F., and Schmaljohann, H. (2014). Fuel loss and flexible fuel deposition rates in a long-distance migrant. Behav. Ecol. Sociobiol. 68, 1465–1471. doi: 10.1007/s00265-014-1753-4
Erni, B., Liechti, F., and Bruderer, B. (2002). Stopover strategies in passerine bird migration: A simulation study. J. Theor. Biol. 219, 479–493. doi: 10.1006/jtbi.2002.3138
Finke, M. D. (2002). Complete nutrient composition of commercially raised invertebrates used as food for insectivores. Zoo Biol. 21, 269–285. doi: 10.1002/zoo.10031
Fransson, T. (1998). A feeding experiment on migratory fuelling in Whitethroats. Sylvia Communis. Anim. Behav. 55, 153–162. doi: 10.1006/anbe.1997.0573
Fransson, T., Jakobsson, S., Johansson, P., Kullberg, C., Lind, J., and Vallin, A. (2001). Magnetic cues trigger extensive refuelling. Nature 414, 35–36. doi: 10.1038/35102115
Gill, R. E. Jr., Tibbitts, T. L., Douglas, D. C., Handel, C. M., Mulcahy, D. M., and Gottschalck, J. C. (2009). Extreme endurance flights by landbirds crossing the Pacific Ocean: Ecological corridor rather than barrier? Proc. R. Soc. B 276, 447–457. doi: 10.1098/rspb.2008.1142
Goymann, W., Spina, F., Ferri, A., and Fusani, L. (2010). Body fat influences departure from stopover sites in migratory birds: Evidence from whole-island telemetry. Biol. Lett. 6, 478–481. doi: 10.1098/rsbl.2009.1028
Gudmundsson, G. A., Lindström, Å, and Alerstam, T. (1991). Optimal fat loads and long distance flights by migrating Knots Calidris canutus, Sanderlings C. alba and Turnstones Arenaria interpres. Ibis 133, 140–152. doi: 10.1111/j.1474-919X.1991.tb04825.x
Guglielmo, C. G. (2018). Obese super athletes: Fat-fueled migration in birds and bats. J. Exp. Biol. 221:jeb165753. doi: 10.1242/jeb.165753
Hedenström, A., and Alerstam, T. (1997). Optimum fuel loads in migratory birds: Distinguishing between time and energy minimization. J. Theor. Biol. 189, 227–234. doi: 10.1006/jtbi.1997.0505
Isaksson, C., Sheldon, B. C., and Uller, T. (2011). The challenges of integrating oxidative stress into life-history biology. Bioscience 61, 194–202. doi: 10.1111/1365-2435.12861
Jackson, D. A., Harvey, H. H., and Somers, K. M. (1990). Ratios in aquatic sciences: Statistical shortcomings with mean depth and the morphoedaphic index. Canad. J. Fish. Aqua. Sci. 47, 1788–1795. doi: 10.1139/f90-203
Jenni-Eiermann, S., Jenni, L., Smith, S., and Costantini, D. (2014). Oxidative stress in endurance flight: An unconsidered factor in bird migration. PLoS One 9:e97650. doi: 10.1371/journal.pone.0097650
Jetz, W., Tertitski, G., Kays, R., Mueller, U., and Wikelski, M. (2022). Biological Earth observation with animal sensors. Trends Ecol. Evol. 37, 293–298. doi: 10.1016/j.tree.2021.11.011
Jones, J. (2002). Age-related differences in body mass and rates of mass gain of passerines during autumn migratory stopover. Condor 104, 49–58. doi: 10.1650/0010-54222002104
Jonzén, N., Hedenström, A., and Lundberg, P. (2007). Climate change and the optimal arrival of migratory birds. Proc. R. Soc. B 274, 269–274. doi: 10.1098/rspb.2006.3719
Kaiser, A. (1993). A new multi-category classification of subcutaneous fat deposits of songbirds. J. Field Ornithol. 64, 246–255.
Karasov, W. H., and Pinshow, B. (2000). Test for physiological limitation to nutrient assimilation in a long-distance passerine migrant at a springtime stopover site. Physiol. Biochem. Zool. 73, 335–343. doi: 10.1086/316746
Kobylkov, D., Kosarev, V., and Mukhin, A. (2014). Fuel for the road: The pre-migratory fuel loading of adult Eurasian Reed Warblers (Acrocephalus scirpaceus). J. Ornithol. 155, 979–986. doi: 10.1007/s10336-014-1084-2
Kvist, A., and Lindström, Å (2000). Maximum daily energy intake: It takes time to lift the metabolic ceiling. Physiol. Biochem. Zool. 73, 30–36. doi: 10.1086/316719
Lindström, Å (1991). Maximum fat deposition rates in migrating birds. Ornis. Scand. 22, 12–19. doi: 10.2307/3676616
Lindström, Å (1995). Stopover ecology of migrating birds: Some unsolved questions. Israel J. Zool. 41, 407–416. doi: 10.1080/00212210.1995.10688810
Lindström, Å (2003). “Fuel deposition rates in migrating birds: Causes, constraints and consequences,” in Avian Migration, eds P. Berthold, E. Gwinner, and E. Sonnenschein (Berlin: Springer).
Lindström, Å, and Alerstam, T. (1992). Optimal fat loads in migrating birds: A test of the time-minimization hypothesis. Amer. Natur. 140, 477–491. doi: 10.1086/285422
Macdonald, D. W., and Amlaner, C. J. (1980). “A practical guide to radio tracking,” in A Handbook on Biotelemetry and Radio Tracking, eds D. W. Macdonald and C. Amlaner (Oxford: Pergamon Press).
McWilliams, S. R., Guglielmo, C., Pierce, B., and Klaassen, M. (2004). Flying, fasting, and feeding in birds during migration: A nutritional and physiological ecology perspective. J. Avian Biol. 35, 377–393. doi: 10.1111/j.0908-8857.2004.03378.x
Møller, A. P., Rubolini, D., and Lehikoinen, E. (2008). Populations of migratory bird species that did not show a phenological response to climate change are declining. Proc. Natl. Acad. Sci. U.S.A. 105, 16195–16200. doi: 10.1073/pnas.0803825105
Naef-Daenzer, B. (1993). A new transmitter for small animals and enhanced methods of home-range analysis. J. Wildl. Manage 57, 68–689.
Naef-Daenzer, B. (2007). An allometric function to fit leg-loop harnesses to terrestrial birds. J. Avian Biol. 38, 404–407. doi: 10.1111/j.2007.0908-8857.03863.x
Pennycuick, C. J. (1998). Computer simulation of fat and muscle burn in long-distance bird migration. J. Theor. Biol. 191, 47–61. doi: 10.1006/jtbi.1997.0572
Pennycuick, C. J., and Battley, P. F. (2003). Burning the engine: A time-marching computation of fat and protein consumption in a 5420-km non-stop flight by Great Knots. Calidris tenuirostris Oikos 103, 323–332. doi: 10.1034/j.1600-0706.2003.12124.x
Piersma, T., and Van Gils, J. A. (2011). The Flexible Phenotype: A Body-Centred Integration of Ecology, Physiology, and Behaviour. Oxford: Oxford University Press.
Rappole, J. H., and Warner, D. W. (1976). Relationships between behavior, physiology and weather in avian transients at a migration stopover site. Oecologia 26, 193–212. doi: 10.1007/BF00345289
Rotics, S., Kaatz, M., Resheff, Y. S., Turjeman, S. F., Zurell, D., Sapir, N., et al. (2016). The challenges of the first migration: Movement and behaviour of juvenile vs. adult White Storks with insights regarding juvenile mortality. J. Anim. Ecol. 85, 938–947. doi: 10.1111/1365-2656.12525
Rubolini, D., Pastor, A. G., Pilastro, A., and Spina, F. (2002). Ecological barriers shaping fuel stores in barn swallows Hirundo rustica following the central and western Mediterranean flyways. J. Avian Biol. 33, 15–22. doi: 10.1034/j.1600-048X.2002.330104.x
Schaub, M., and Jenni, L. (2000). Fuel deposition of three passerine bird species along the migration route. Oecologia 122, 306–317. doi: 10.1007/s004420050036
Schaub, M., and Jenni, L. (2001). Stopover durations of three warbler species along their autumn migration route. Oecologia 128, 217–227. doi: 10.1007/s004420100654
Schaub, M., Jenni, L., and Bairlein, F. (2008). Fuel stores, fuel accumulation, and the decision to depart from a migration stopover site. Behav. Ecol. 19, 657–666. doi: 10.1093/beheco/arn023
Schmaljohann, H., Becker, P. J. J., Karaardic, H., Liechti, F., Naef-Daenzer, B., and Grande, C. (2011). Nocturnal exploratory flights, departure time, and direction in a migratory songbird. J. Ornithol. 152, 439–452. doi: 10.1007/s10336-010-0604-y
Schmaljohann, H., and Dierschke, V. (2005). Optimal bird migration and predation risk: A field experiment with Northern Wheatears Oenanthe oenanthe. J. Anim. Ecol. 74, 131–138. doi: 10.1111/j.1365-2656.2004.00905.x
Schmaljohann, H., and Eikenaar, C. (2017). How do energy stores and changes in these affect departure decisions by migratory birds? A critical view on stopover ecology studies and some future perspectives. J. Comp. Physiol. A 203, 411–429. doi: 10.1007/s00359-017-1166-8
Schmaljohann, H., Eikenaar, C., and Sapir, N. (2022). Understanding the ecological and evolutionary function of stopover in migrating birds. Biol. Rev. 97, 1231–1252. doi: 10.1111/brv.12839
Schmaljohann, H., and Klinner, T. (2020). A quasi-experimental approach using telemetry to assess migration-strategy-specific differences in the decision-making processes at stopover. BMC Ecol. 20:36. doi: 10.1186/s12898-020-00307-5
Schmaljohann, H., Korner-Nievergelt, F., Naef-Daenzer, B., Nagel, R., Maggini, I., Bulte, M., et al. (2013). Stopover optimization in a long-distance migrant: The role of fuel load and nocturnal take-off time in Alaskan Northern Wheatears (Oenanthe oenanthe). Front. Zool. 10:26. doi: 10.1186/1742-9994-10-26
Stillman, R. A., Caldow, R. W. G., Goss-Custard, J. D., and Alexander, M. J. (2000). Individual variation in intake rate: The relative importance of foraging efficiency and dominance. J. Anim. Ecol. 69, 484–493. doi: 10.1046/j.1365-2656.2000.00410.x
Studds, C. E., and Marra, P. P. (2005). Nonbreeding habitat occupancy and population processes: An upgrade experiment with a migratory bird. Ecology 86, 2380–2385. doi: 10.1890/04-1145
Svensson, L. (1992). Identification Guide to European Passerines, 4th Edn. Norfolk: British Trust for Ornithology.
Taylor, P. D., Mackenzie, S. A., Thurber, B. G., Calvert, A. M., Mills, A. M., McGuire, L. P., et al. (2011). Landscape movements of migratory birds and bats reveal an expanded scale of stopover. PLoS One 6:e27054. doi: 10.1371/journal.pone.0027054
Toledo, S., Shohami, D., Schiffner, I., Lourie, E., Orchan, Y., Bartan, Y., et al. (2020). Cognitive map–based navigation in wild bats revealed by a new high-throughput tracking system. Science 369, 188–193. doi: 10.1126/science.aax6904
Williams, M. R., Lamont, B. B., and He, T. (2022). Dealing with ‘the spectre of “spurious” correlations’: Hazards in comparing ratios and other derived variables with a randomization test to determine if a biological interpretation is justified. Oikos 2022:e08575. doi: 10.1111/oik.08575
Keywords: optimal migration strategies, bird stopover ecology, departure fuel load, body mass measurements, ATLAS biotelemetry system, Hula Valley Israel, Red-backed Shrike
Citation: Zinßmeister D, Troupin D and Sapir N (2022) Autumn migrating passerines at a desert edge: Do birds depart for migration after reaching a threshold fuel load or vary it according to the rate of fuel deposition? Front. Ecol. Evol. 10:874923. doi: 10.3389/fevo.2022.874923
Received: 13 February 2022; Accepted: 20 July 2022;
Published: 17 August 2022.
Edited by:
Nikita Chernetsov, Zoological Institute (RAS), RussiaReviewed by:
Arseny Tsvey, Zoological Institute (RAS), RussiaYolanda E. Morbey, Western University, Canada
Copyright © 2022 Zinßmeister, Troupin and Sapir. This is an open-access article distributed under the terms of the Creative Commons Attribution License (CC BY). The use, distribution or reproduction in other forums is permitted, provided the original author(s) and the copyright owner(s) are credited and that the original publication in this journal is cited, in accordance with accepted academic practice. No use, distribution or reproduction is permitted which does not comply with these terms.
*Correspondence: Nir Sapir, bmlyc0BzY2kuaGFpZmEuYWMuaWw=