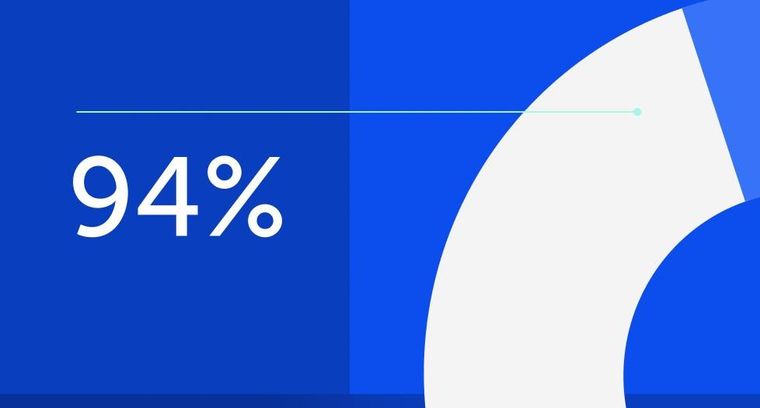
94% of researchers rate our articles as excellent or good
Learn more about the work of our research integrity team to safeguard the quality of each article we publish.
Find out more
ORIGINAL RESEARCH article
Front. Ecol. Evol., 07 April 2022
Sec. Ecophysiology
Volume 10 - 2022 | https://doi.org/10.3389/fevo.2022.871370
Much research has focused on visual system evolution in bony fishes. The capacity of visual systems to perceive and respond to external signals is integral to evolutionary success. However, integrated research on the mechanisms of adaptive evolution based on corneal structure and related genes remains limited. In this study, scanning electron microscopy (SEM) was used to assess the microstructure and adaptation of corneal epithelial cells. Then, the evolution of the cornea-related COL8A2 gene was investigated. We found various projections (microridges, microplicae, microholes, and microvilli) on the corneal epithelial cells of amphibious mudskippers. Compared with those of fully aquatic fishes, these microstructures were considered adaptations to the variable environments experienced by amphibious mudskippers, as they can resist dryness in terrestrial environments and infection in aquatic environments. Moreover, strong purifying selection was detected for COL8A2. In addition, some specific amino acid substitution sites were also identified in the COL8A2 sequence in mudskippers. Interestingly, the evolutionary rate of the COL8A2 gene was significantly and positively correlated with maximum diving depth in our dataset. Specifically, with increasing diving depth, the evolutionary rate of the COL8A2 gene seemed to gradually accelerate. The results indicated that the cornea of bony fishes has evolved through adaptation to cope with the different diving depths encountered during the evolutionary process, with the corneal evolution of the amphibious mudskipper group showing a unique pattern.
Independent adaptation to different aquatic niche across fishes has been accompanied by widespread changes in the visual system for coping with different light conditions (Reuter and Peichl, 2008). Due to adaptation to different water depths, the structure of fish eyes and their vision-related genes have undergone adaptive evolution accordingly. For example, the visual system of the Mariana lionfish (Pseudoliparis swirei) has been severely degraded due to its adaptation to darkness (Jiang et al., 2019). In many deep-diving groups, the rhodopsin RH1 gene, which mainly mediates scotopic vision (Lythgoe and Dartnall, 1970; Yokoyama et al., 2008), has not only evolved rapidly but also mutated in amino acid (AA) sequences with similar functional properties, resulting in adaptive evolution of protein functions (Xia et al., 2021). In addition, in the color vision system, cone cells and related genes (SWS1, LWS, etc.) also show adaptive evolutionary patterns (Levenson et al., 2006; Meredith et al., 2013; Emerling et al., 2015). Generally, the visual systems of fishes adapted to different living conditions have revealed new features of divergent structures and mechanisms of visual perception in various environments (Subramanian et al., 2008; Simmich et al., 2012; Hauser et al., 2017; Alexander et al., 2021).
The cornea is the outermost tissue of the eye and is an essential for animal vision, functioning in refraction and protection of the internal structure of the eye (Maurice, 1957; Bard et al., 1988). In contrast to the cone and retinal rod, the cornea may have different adaptation mechanisms due to its unique structure and physiological function in the eye (Maurice, 1957; Bard et al., 1988). Corneal morphology also shows complex diversity under different environmental conditions among different mammals and fishes (Mass and Supin, 2007; Subramanian et al., 2008), and the internal structure of the cornea has different cell types, densities, and microstructures, such as microvilli, microplicae, microridges and microholes (Collin and Collin, 2006; Simmich et al., 2012). A study on the corneas of 51 vertebrates revealed that corneal epithelial cells are mainly irregularly pentagonal or hexagonal in shape and show many microscopic structures that vary according to habitat (Collin and Collin, 2006). These structures are thought to be resistant to desiccation and abrasion in terrestrial environments and to infection in aquatic environments (Subramanian et al., 2008). In addition, Hu et al. (2016) found significant differences in corneal structure between juveniles and adults in Gobiinae due to their habitat differences (Hu et al., 2016). Generally, the cornea has undergone adaptive evolution in response to different water environments.
Belonging to a transitional group, most mudskipper species are amphibious fish (with both aquatic and semiterrestrial habitats) (Graham, 1997). They are members of family Oxudercidae and the subfamily Oxudercinae. They are divided into four main genera, including Boleophthalmus, Periophthalmodon, Periophthalmus, and Scartelaos (Ishimatsu and Gonzales, 2011). Their eyes placed on top of the head on short stalks and capable of being elevated or retracted, are well adapted for vision in air (Nelson et al., 2016).
Phylogenetic and comparative analyses revealed that the terrestrial transitions of mudskipper lineages are were independent of each other (Steppan et al., 2022). These species are mainly distributed in the intertidal mangrove or muddy beach areas of tropical, subtropical and temperate seas and exhibit a wide range of extreme adaptations to an amphibious lifestyle (Nursall, 1981; Burggren, 1997; Sayer, 2005; Ishimatsu and Gonzales, 2011). Because of the special habitat selection of this group, their visual evolution has always attracted much attention. Studies on corneal epithelial cells have shown that cell density in gobies usually decreases with decreasing corneal osmotic stress, and corneal epithelial cells also adapt to different degrees (Hu et al., 2016). To cope with amphibious conditions, mudskippers have evolved a broader range of color sensitivities between LWS1 and LWS2 than other teleosts and a shift toward violet rather than ultraviolet light (Shi and Yokoyama, 2003; You et al., 2014). The cornea is an important structure for vision, and the COL8A2 gene encodes the alpha 2 chain of type VIII collagen. This protein is a major component of the basement membrane of the corneal endothelium and forms homo- or heterotrimers with alpha 1 (VIII)-type collagens (GeneCard, 2021). In the normal cornea, there is a smooth monolayer of corneal endothelial cells (CECs), and the primary component is collagen type VIII (COL8), a non-fibrillar, short-chain collagen secreted by CECs (Meng et al., 2013). Investigations of the COL8A2 gene in bony fish are rare. However, missense mutations in the gene encoding the α2 (VIII) subunit of collagen VIII are associated with an early onset form of Fuchs dystrophy in human (Biswas et al., 2001). Additionally, experiments on different mouse models with COL82A mutations recapitulated some of the findings for endothelial cells and Descent’s membrane in humans (Jun et al., 2012). However, the variation in adaptive mechanisms among different diving depths, especially in amphibious species, remains unclear. In this study, some goby species were selected to dissect the morphology and structure of corneal epithelial cells, and their structures were compared with those of strictly aquatic fish to reveal the adaptation of fish corneas to different habitats.
Specimens sampled from Xiapu, China, on December 2, 2014, were divided into three groups: Scartelaos histophorus (six individuals), Boleophthalmus pectinirostris (seven individuals), and Periophthalmus magnuspinnatus (six individuals). The examined specimens are listed in Supplementary Table 1. All individuals were mature and in good health without ocular disease or abnormality prior to euthanasia. Specimens were euthanized in accordance with ethical guidelines. After observing the location and shape of the eyes through a ZEISS STEISV II microscope, the right eye of each specimen was completely removed, and the cornea of each enucleated eye was pretreated. Higher-magnification images were recorded digitally (Hu et al., 2016).
In this study, five species (i.e., P. magnuspinnatus, Periophthalmodon schlosseri, S. histophorus, B. pectinirostris, and Mugilogobius chulae) from Gobiiformes were used for phylogenetic and evolutionary analyses (Supplementary Table 2). To attain broad and balanced depth range coverage for analysis, 13 full-length mitochondrial DNA (mtDNA) and COL8A2 sequences were obtained from Clupeiformes, Pleuronectiformes, Scombriformes, Acanthuriformes, Carangiformes, and Perciformes (35 species in total), which are able to dive to various depths (Supplementary Table 2).
Multiple sequence alignments (MSAs) of the 13 mtDNA sequences and COL8A2 sequences of 35 species were obtained by using webPRANK1 based on codons. Afterward, the gaps and non-homologous regions were deleted by using GBLOCKS (Talavera and Castresana, 2007). The specific AA sites of COL8A2 MSA file were manually inspected by eye in MEGA 7 (Kumar et al., 2016). The domains of the protein secondary structure were identified by using UniProt.2
We obtained the 3D structure of the COL8A2 protein from UniProt (see text footnote “2”). Here, the COL8A2 homolog of zebra fish (Danio rerio) was used as the model. To provide further insights into the functional significance of these specific AA sites, they were mapped onto the 3D structure of the COL8A2 gene using PYMOL.3
The 13 mtDNA sequences were assembled by SequenceMatrix (Vaidya et al., 2011). The PhyloSuite platform (Zhang et al., 2020) was used to construct a phylogenetic tree. Maximum likelihood (ML) and Bayesian inference (BI) phylogenies were inferred by using IQ-TREE (Lam-Tung et al., 2015) and MrBayes 3.2.6 (Ronquist et al., 2012), respectively. The best model for each analysis was GTR + R5 + F. The ML tree was constructed with 1000 bootstrap replications. For BI analysis, Markov chain Monte Carlo (MCMC) chains for 10 million generations, with sampling every 1,000 generations. The phylogeny generated in PhyloSuite was visualized in Figtree v1.4.4 software4 and then used for subsequent evolutionary analyses.
To determine whether adaptive evolution of the COL8A2 gene might have occurred in bony fishes with different diving depths and the selection model of mudskipper, we used the PAML 4.9 package (Yang, 2007), which implements an ML method to calculate the ratio of non-synonymous (dN) to synonymous (dS) substitutions (ω = dN/dS). ω > 1, = 1 and <1 indicate positive selection, neutrality and negative selection, respectively.
To test for possible heterogeneity of ω ratios along independent branches, we used the free-ratio branch model, which allows each branch to have a separate ω value. The null model is the strict one-ratio model (M0, all branches have one estimated ω). To explore potential relationships between gene evolution and maximum diving depth values obtained from FISHBASE5 (see Supplementary Table 2), we examined the association between these two factors according to the method described by Montgomery et al. (2011). The evolutionary rates of the COL8A2 gene in the dataset were estimated by the root-to-tip ω values, which were computed in the CODEML program (Yang, 2007). Root-to-tip ω, which is regarded as a signal of an evolutionary trajectory and calculated through regression analysis against phenotypic data from extant species (Montgomery et al., 2011; Xu et al., 2017), is more inclusive of the evolutionary history of a locus than other metrics (Wolf et al., 2009). The mean ω value includes dN/dS ratios from an ancestral root to a terminal tip in a phylogenetic tree. Here, the value was calculated by the free-ratio model (S*dS = 0 and N*dN = 0 were not considered). Then, phylogenetic generalized least squares (PGLS) analysis was implemented in the R program (Orme et al., 2012). This method included the parameter lambda (λ), which was evaluated by the ML method. The parameter provided a quantitative estimate of the phylogenetic signal, which ranged from 0 (no phylogenetic signal) to 1 (significant phylogenetic signal).
To better understand the discrepancy in evolutionary rates between amphibious and fully aquatic fishes, a two-ratio model was implemented, in which the mudskipper clade was regarded as the foreground branch (Figure 2A). Then, the six-ratio model was further applied to uncover differences among fishes with different diving depths (Figures 1, 2B). For all the analyses, the nested models were compared by using a likelihood ratio test (LRT) with various degrees of freedom. All analyses were run twice to ensure convergence. A general overview of this study is shown in Figure 3.
Figure 1. Division of different photic layers in the ocean according to reference (Arístegui et al., 2009). Mudskippers are mainly distributed in the intertidal zone marked in green boxes.
Figure 2. (A,B) Graphical representation of branch models implemented to test for a possible role of selection across different teleost lineages with different diving abilities.
The epithelial cell microstructures were observed under a scanning electron microscope (Figures 4–6). In B. pectinirostris, the internal cells were differentiated into diverse structures, such as the ridge type (Figures 4A,B), reticular type (Figure 4C), and ridge-reticular type (Figure 4D). The border of corneal epithelial cells was clear, with many small projections (Figure 4C). The microplicae, microvilli, microholes (Figure 4C) and microridges (Figure 4A) were observed under high magnification.
Figure 4. (A–D) Corneal epithelial cells of B. pectinirostris under a scanning electron microscope. The types and microstructures of the epithelial cells are shown (mp, microplicae; mv, microvilli; mh, microholes; mr, microridges).
Figure 5. (A–D) Corneal epithelial cells of P. magnuspinnatus under a scanning electron microscope. The type and microstructure of the epithelial cells are shown (mp, microplicae; mv, microvilli; mh, microholes; mr, microridges).
Figure 6. (A–D) Corneal epithelial cells of S. histophorus under a scanning electron microscope. The type and microstructure of the epithelial cells are shown (mp, microplicae; mv, microvilli; mr, microridges).
In P. magnuspinnatus, the ridge cell type was observed (Figure 5A), and the border was clear with many small projections (Figures 5B,D). The corneal epithelial cells also consisted of four types of microstructures (Figure 5): microridges, microplicae, microvilli (Figures 5B,D) and microholes (Figure 5C). Compared those in B. pectinirostris, the microridges of corneal epithelial cells in P. magnuspinnatus were rough, with more microplicae and microvilli. The corneal epithelial cell surface was characterized by fragmentation and a compact structure.
In S. histophorus, corneal epithelial cells were of the ridge type (Figure 6). The border of the cells was clear (Figure 6C). Microplicae, microvilli and microridges (but not microholes) (Figure 6D) were observed under high magnification.
Based on the study of Collin and Collin (2006), we further compared the corneal microstructures of mudskippers and fully aquatic fishes. Specifically, as shown in Table 1, corneal epithelial cell microstructure and type were compared. Four kinds of microstructures were present in B. pectinirostris and P. magnuspinnatus, while only microridges were recorded in A. butcheri and only microholes and microvilli were observed in N. forsteri. In B. pectinirostris, there were three corneal epithelial cell types, but only the ridge type was recorded in A. butcheri, P. magnuspinnatus and S. histophorus. In short, the mudskippers examined in our study may have richer cellular microstructures and cell types than fully aquatic fishes.
Table 1. Corneal epithelial cell microstructure and type comparison among different species and mudskippers.
We constructed phylogenetic trees using IQ-TREE based on 13 mitochondrial coding genes. The relationships shown by the gene trees largely reflected known species relationships with high bootstrap support (Supplementary Figure 1).
The results showed that the free-ratio model (Model C) fit the data significantly better than the one-ratio model (Model A) for the COL8A2 gene (Table 2), which suggested divergent selection pressures on different teleostean branches. Further two-ratio model analysis revealed that the evolutionary rate of mudskipper fishes was significantly lower than that of other groups, and the whole mudskipper branch showed evidence of strong negative selection (Table 2). The results of the six-ratio model showed significant differences in evolutionary rates among fishes with various maximum diving depths (Table 2). Moreover, with increasing diving depth, the evolutionary rate of the COL8A2 gene seemed to gradually accelerate, except in the bathypelagic group (Figure 7).
Table 2. Likelihood and omega values estimated under branch models of selection pressures on the COL8A2 gene.
Corneal morphology is influenced by diving depth, and the rate of evolution of related genes is directly related to morphology. To explore the relationship between the evolutionary rate of the COL8A2 gene and maximum diving depth, we implemented PGLS analysis to estimate the association between the root-to-tip ω of the COL8A2 gene and maximum diving depth. The λ value was 0.699; however, P > 0.05, which indicated no phylogenetic signal in our analysis (Supplementary Table 3). Therefore, only the ordinary least squares (OLS) approach was implemented. The results showed a significant positive regression between the root-to-tip ω and maximum diving depth (Figure 8).
Further sequence comparison revealed many AA site substitutions and deletions in the COL8A2 AA sequence of mudskipper fishes (Figure 9). Some sites were located in important functional sites or domains (Figure 10). In particular, the absent region (sites 385–402) was located in the triple-helical region, and sites 5 and 13 belong to the signal peptide.
Figure 10. Specific amino acid sites (red) and absent region (blue) mapped on the 3D structure of COL8A2 protein in mudskippers. The numbers represent the positions of these specific amino acid sites and regions.
Mudskippers are the largest group of amphibious teleost fish, most of which are uniquely adapted to live on mudflats (Graham, 1997). During their successful transition from aquatic to terrestrial living, they evolved morphological and physiological modifications in some systems, e.g., olfaction, vision, and aerial respiration (You et al., 2018). Regarding vision, for amphibious habitats lifestyle adaptation, some vision-related genes are differentially lost or mutated, such as SWS1, which exhibits a loss of function (You et al., 2014). In this study, adaptive characters of corneal physiological structure were found in mudskippers (Figures 4–6 and Table 1). There were many types of corneal epithelial cells in mudskippers. For example, B. pectinirostris has three types of cells and mainly migrates to low tidal regions without vegetation, which suggests that it has various ecomorphologies to adapt to a diversity of habitats.
The main surface features of the corneal epithelium of terrestrial vertebrates are usually microplicae and microvilli, while the corneal epithelium of aquatic vertebrates usually has microridges (Beuerman and Pedroza, 1996). The results in Table 1 show that amphibious mudskippers generally have microplicae, microvilli and microridges, while aquatic fish have only microridges. The presence of a variety of cellular microstructures seems to be characteristic of corneal epithelial cells of fishes with amphibious lifestyles.
Microvilli are usually vertical extensions of the surface of epithelial cells and are believed to be the most effective way to increase the surface area of cells, which facilitates the uptake of oxygen and nutrients by the cell and the excretion of water and metabolites from the cell (Beuerman and Pedroza, 1996). These structures are generally found on the corneal surface of species that spend adequate periods out of an aquatic environment (Beuerman and Pedroza, 1996). Our study confirms the existence of microvilli in mudskippers. Phylogenetic work revealed molecular evidence to support the division of family Gobiidae into two major clades, family Oxudercidae (= Gobionellidae by some authors) and Gobiidea. Four formerly recognized gobiid subfamilies-Gobionellinae, Oxudercinae (including the mudskippers), Amblyopinae, and Sicydiinae, are included within family Oxudercidae (Thacker, 2003; Thacker, 2009; Thacker et al., 2011; Agorreta et al., 2013). Mudskippers (Teleostei: Gobiidae: Oxudercinae: Periophthalmini) are gobies that are “fully terrestrial for some portion of the daily cycle.” They belong to four genera: Boleophthalmus, Periophthalmodon, Periophthalmus, and Scartelaos (Murdy, 1989). The diversity of both sensory structures and visually mediated behaviors of fish reflects the complexity of their environment. Visual stimuli vary in both physical and biological components (Evans, 2004). Aquatic and terrestrial environments typically present very different optical, osmotic and physico-mechanical problems to the cornea. In aquatic environments, the cornea contributes little to the refractive power of the eye due to the similarity of the refractive indices of water (Kröger, 1992). In terrestrial environments, the refractive index of air is 1.00 under standard temperature and pressure conditions. Based on our previous observation, terrestrial mudskippers tend to have eyes on the top of their heads, while aquatic mudskippers have eyes on both sides of their heads, similar to those of ordinary fish. These fishes inhabit the intertidal zone and mangrove respiratory root areas, and their eyes are exposed to air for a long time in tidal flats or shallow waters. Our results combined with those previously reported for N. forsteri (which lives in terrestrial habitats for a long time) (Collin and Collin, 2006) suggest that the corneal epithelium of amphibious fish has evolved adaptively in response to the relatively arid terrestrial environment.
Microplicae are mainly found in cartilaginous and jawless fishes and amphibians. They may represent a transitional form between microvilli and microridge that increases the osmotic exchange function of the cornea and the supporting strength of the cornea. All 30 mudskipper species are resident intertidal fishes, and are strictly found in temperate, subtropical and tropical mudflat, mangrove and lower fluvial ecosystems (Murdy, 1989; Jaafar and Larson, 2008). Mudskippers spend part of their lives out of water to feed, mate, and avoid capture by terrestrial predators. Therefore, they have developed behavioral and physiological specializations for adaptation to amphibious life. The most significant morphological and functional modifications are the trend to have closely set and moveable protuberant eyes located high on the head for escaping from predators on land and they are structurally adapted for accurate vision in both air and water (Sayer, 2005). Microplicae are observed in the corneal epithelial cells of amphibious fish, which may correspond to their amphibious lifestyle.
Among the microstructure types, the one that increases the cell membrane area the least is microridges. Previous studies suggested that microridges are found only on corneal surfaces exposed to hypertonic (mainly marine) environments, as commonly recorded in aquatic habitats (Hu et al., 2016). Microridges increase corneal strength and the surface area of the mucus layer, and the microridge structure helps stabilize the tear film of teleost fishes (Hu et al., 2016). Well-developed microridges occur only in bony fishes that live in hypertonic environments, such as marine or estuarine habitats (Hu et al., 2016). As shown in Table 1, the microstructures of the three mudskipper species were dominated by microridges, which is consistent with amphibious mudskippers having evolved adaptations to high-osmolarity seawater, similar to other marine fishes.
In aquatic species, unlike the pores found in most terrestrial species, microholes are found only in species that burrow into the substrate and may be exposed to air, e.g., Geotria australis, Mordacia mordax, Ambystoma mexicanum (Collin and Collin, 2000), and Limnichthyes fasciatus (Collin and Collin, 1997). Mudskippers usually nest in mudflats, building burrows where they can wait for an opportunity to surprise prey outside, as well as avoid predators and breed (Ishimatsu et al., 1998). In our study, B. pectinirostris and P. magnuspinnatus showed microholes. This reflects a great degree of adaptability to their habitats.
In addition, the evolutionary history of the cornea-related gene COL8A2, which plays key roles in the development of the corneal endothelium (GeneCard, 2021), also includes adaptive changes. We found that COL8A2 has undergone strong purifying selection in some goby clades (Table 2 Mudskipper Store Air in Their Burrows). Moreover, some specific AA substitution sites were found in the COL8A2 AA sequence, some of which were located in and/or near important functional sites and domains (Figure 9). For example, sites 385–402 are located in the triple-helical region; however, they are absent in some goby clades. Mutation or deletion of these sites will lead to conformational changes in the three-dimensional structure of the COL8A2 protein, thereby affecting its overall function. Our results revealed that the cornea of gobies has evolved at the molecular level to enable adaptation to an amphibious lifestyle.
Due to the absorption and scattering of light in water, the photic environment of the aquatic system changes rapidly with depth, and the phenomenon of wavelength absorption leads to a blueshift with increasing water depth (Davies et al., 2012; Hauser et al., 2017; Hauser and Chang, 2017). In addition, due to the different levels of refraction between water and land, there are adaptive changes in the structure of fish eyes (Sayer, 2005). The cornea is the outermost structure of the eye and functions in refraction and protection of the internal structure of the eye. Its morphological changes largely reflect the adaptation of fish to an aquatic environment (Mass and Supin, 2007). In addition, genes might have undergone adaptive evolution. Our results revealed a significant correlation between COL8A2 evolution and maximum diving depth (Figure 8), which indicated selection on genes imposed by diving depth. This conclusion is consistent with the finding of a previous study on RH1 that water depth adaptation has a significant impact on the evolution of vision (Sugawara et al., 2005; Dungan et al., 2016; Xia et al., 2021). In addition, the analysis of differential selection further confirmed that COL8A2 was subject to differential selection among different aquatic environments (Table 2 and Figure 7). Through the comparison of anatomical data, obvious differences in the corneal structure of fishes in different habitats were also found. Meanwhile, the evolution of the COL8A2 gene exhibits a special pattern. However, the relationship and mechanisms of these two types of evolutionary processes are unclear. Thus, further research is needed to confirm the mechanisms by which diving depth drives corneal adaptation.
This study provides new insight into the evolutionary mechanisms of visual adaptation in teleosts based on corneas. In this study, two parallel lines of evidence for visual adaptations in goby fish were uncovered. Phenotypically, the cornea of this clade might have evolved structures adapted to both aquatic (marine) and terrestrial habitats, i.e., microvilli and microridges. Furthermore, strong purifying selection was detected for the cornea-related COL8A2 gene. In addition, some specific AA substitution sites were also identified in the COL8A2 sequence in gobies, which might be associated with their amphibious lifestyle. Moreover, there was a significant and positive correlation between the evolution of the COL8A2 gene and maximum diving depth. Further research is needed to determine the mechanisms explaining the relationship between morphological adaptation and functional genes.
The original contributions presented in the study are included in the article/Supplementary Material, further inquiries can be directed to the corresponding authors.
Ethical review and approval was not required for the animal study because the experimental materials involved in this study are lower vertebrates, and there are few experimental materials. There are no inhumane procedures in the experiment.
WH participated in the determination of research and writing ideas, designed and completed the experiment of fish physiology and scanning electron microscopy, completed the data collection and manuscript writing of this part, and completed the manuscript submission. YM participated in the determination of research and writing ideas, and completed the data collection, and manuscript writing on the molecular part. JZ overall guidance to the research and writing ideas, and participated in the writing of the whole manuscript. FL participated in the collection of fish samples. XL participated in the writing and modification of part of the manuscript. All authors contributed to the article and approved the submitted version.
Grants supporting this research were received awards from the Chinese Academy of Sciences (XDA19050202) to JZ, and from the Erhai Watershed Ecological Environment Quality Testing Engineering Research Center of Yunnan provincial Provincial Universities to WH, and from the start-up projects on high-level talent introduction of Dali University to YM (No. KY1916101940).
The authors declare that the research was conducted in the absence of any commercial or financial relationships that could be construed as a potential conflict of interest.
All claims expressed in this article are solely those of the authors and do not necessarily represent those of their affiliated organizations, or those of the publisher, the editors and the reviewers. Any product that may be evaluated in this article, or claim that may be made by its manufacturer, is not guaranteed or endorsed by the publisher.
We thank Shengguo Chen for helping collect specimens and for support in the field. We also thank Yunlin Gai (China West Normal University) for valuable suggestions.
The Supplementary Material for this article can be found online at: https://www.frontiersin.org/articles/10.3389/fevo.2022.871370/full#supplementary-material
Supplementary Figure 1 | Phylogenetic tree of 13 mtDNA constructed by IQ-TREE.
Agorreta, A., San Mauro, D., Schliewen, U., Van Tassell, J. L., Kovačić, M., Zardoya, R., et al. (2013). Molecular phylogenetics of Gobioidei and phylogenetic placement of European gobies. Mol. Phylogenet. Evol. 69, 619–633. doi: 10.1016/j.ympev.2013.07.017
Alexander, V. N., Castiglione, G. M., Gutierrez, E. D., Lovejoy, N. R., and Chang, B. S. W. (2021). Recreated ancestral opsin associated with marine to freshwater croaker invasion reveals kinetic and spectral adaptation. Mol. Biol. Evol. 38, 2076–2087. doi: 10.1093/molbev/msab008
Arístegui, J., Gasol, J. M., Duarte, C. M., and Herndld, G. J. (2009). Microbial oceanography of the dark ocean’s pelagic realm. Limnol. Oceanogr. 54, 1501–1529. doi: 10.1016/j.ejop.2020.125721
Bard, J. B., Bansal, M. K., and Ross, A. S. (1988). The extracellular matrix of the developing cornea: diversity, deposition and function. Development 103, 195–205. doi: 10.1242/dev.103.Supplement.195
Beuerman, R. W., and Pedroza, L. (1996). Ultrastructure of the human cornea. Microsc. Res. Tech. 33, 320–335. doi: 10.1002/(sici)1097-0029(19960301)33:4<320::aid-jemt3>3.0.co;2-t
Biswas, S., Munier, F. L., Yardley, J., Hart-Holden, N., Perveen, R., Cousin, P., et al. (2001). Missense mutations in COL8A2, the gene encoding the alpha2 chain of type VIII collagen, cause two forms of corneal endothelial dystrophy. Hum. Mol. Genet. 10, 2415–2423. doi: 10.1093/hmg/10.21.2415
Burggren, W. W. (1997). Air-Breathing Fishes: Evolution, Diversity, and Adaptation. San Diego, CA: Academic Press.
Collin, H. B., and Collin, S. P. (2000). The corneal surface of aquatic vertebrates: microstructures with optical and nutritional function? Philos. Trans. R. Soc. Lond. B Biol. Sci. 355, 1171–1176. doi: 10.1098/rstb.2000.0661
Collin, S. P., and Collin, H. B. (1997). The head and eye of the sand lance, limnichthyes fasciatus-a field emission scanning electron microscopy study. Clin. Exp. Optom. 80, 133–138. doi: 10.1111/j.1444-0938.1997.tb04868.x
Collin, S. P., and Collin, H. B. (2006). The corneal epithelial surface in the eyes of vertebrates: environmental and evolutionary influences on structure and function. J. Morphol. 267, 273–291. doi: 10.1002/jmor.10400
Davies, W., Collin, S. P., and Hunt, D. M. (2012). Molecular ecology and adaptation of visual photopigments in craniates. Mol. Ecol. 21, 3121–3158. doi: 10.1111/j.1365-294X.2012.05617.x
Dungan, S. Z., Alexander, K., and Chang, B. S. W. (2016). Spectral tuning of killer whale (Orcinus orca) rhodopsin: evidence for positive selection and functional adaptation in a cetacean visual pigment. Mol. Biol. Evol. 33, 323–336. doi: 10.1093/molbev/msv217
Emerling, C. A., Huynh, H. T., Nguyen, M. A., Meredith, R. W., and Springer, M. S. (2015). Spectral shifts of mammalian ultraviolet-sensitive pigments (short wavelength-sensitive opsin 1) are associated with eye length and photic niche evolution. Proc. Biol. Sci. 282:20151817. doi: 10.1098/rspb.2015.1817
Evans, B. I. (2004). “A fish’s eye view of habitat change,” in The Senses of Fish: Adaptations for the Reception of Natural Stimuli, eds G. von der Emde, J. Mogdans, and B. G. Kapoor (New Delhi: Narosa Publishing House), 1–30. doi: 10.1007/978-94-007-1060-3_1
GeneCard (2021). COL8A2 Gene. Available online at: https://www.genecards.org/cgi-bin/carddisp.pl?gene=COL8A2 (accessed December 27, 2021).
Graham, J. B. (1997). Air Breathing Fishes: Evolution, Diversity and Adaptation. San Diego, CA: Academic Press.
Hauser, F. E., and Chang, B. S. (2017). Insights into visual pigment adaptation and diversity from model ecological and evolutionary systems. Curr. Opin. Genet. Dev. 47, 110–120. doi: 10.1016/j.gde.2017.09.005
Hauser, F. E., Ilves, K. L., Schott, R. K., Castiglione, G. M., López-Fernández, H., and Chang, B. S. W. (2017). Accelerated evolution and functional divergence of the dim light visual pigment accompanies cichlid colonization of central America. Mol. Biol. Evol. 34, 2650–2664. doi: 10.1093/molbev/msx192
Hu, W. X., Zhang, J., and Kang, B. (2016). Structure and function of corneal surface of mudskipper fishes. Fish Physiol. Biochem. 42, 1481–1489. doi: 10.1007/s10695-016-0234-2
Ishimatsu, A., and Gonzales, T. T. (2011). “Mudskippers: front runners in the modern invasion of land,” in The Biology of Gobies, eds R. A. Patzner, J. L. Van Tassell, M. Kovačić, and B. G. Kapoor (Enfield, NH: Science Publishers Inc), 609–638.
Ishimatsu, A., Hishida, Y., Takita, T., Kanda, T., Oikawa, S., Takeda, T., et al. (1998). Mudskippers store air in their burrows. Nature 391, 237–238. doi: 10.1038/34560
Jaafar, Z., and Larson, H. K. (2008). A new species of mudskipper, Periophthalmus takita (: Gobiidae: Oxudercinae), from Australia, with a key to the genus. Zoolog. Sci. 25:947. doi: 10.2108/zsj.25.946
Jiang, H. F., Du, K., Gan, X. N., Yang, L. D., and He, S. P. (2019). Massive loss of olfactory receptors but not trace amine-associated receptors in the world’s deepest-living fish (Pseudoliparis swirei). Genes 10:910. doi: 10.3390/genes10110910
Jun, A. S., Meng, H., Ramanan, N., Matthaei, M., Chakravarti, S., Bonshek, R., et al. (2012). An alpha 2 collagen VIII transgenic knock-in mouse model of Fuchs endothelial corneal dystrophy shows early endothelial cell unfolded protein response and apoptosis. Hum. Mol. Genet. 21, 384–393. doi: 10.1093/hmg/ddr473
Kröger, R. H. H. (1992). Methods to estimate dispersion in vertebrate ocular media. J. Opt. Soc. Am. A 9, 1486–1490. doi: 10.1364/josaa.9.001486
Kumar, S., Stecher, G., and Tamura, K. (2016). MEGA7: molecular evolutionary genetics analysis version 7.0 for bigger datasets. Mol. Biol. Evol. 33, 1870–1874. doi: 10.1093/molbev/msw054
Lam-Tung, N., Schmidt, H. A., Arndt, V. H., and Quang, M. B. (2015). IQ-TREE: a fast and effective stochastic algorithm for estimating maximum-likelihood phylogenies. Mol. Biol. Evol. 32, 268–274. doi: 10.1093/molbev/msu300
Levenson, D. H., Ponganis, P. J., Crognale, M. A., Deegan, J. F., Dizon, A., and Jacobs, G. H. (2006). Visual pigments of marine carnivores: pinnipeds, polar bear, and sea otter. J. Comp. Physiol. A Neuroethol. Sens. Neural Behav. Physiol. 192, 833–843. doi: 10.1007/s00359-006-0121-x
Lythgoe, J. N., and Dartnall, H. J. A. (1970). A “deep sea rhodopsin” in a mammal. Nature 227, 955–956. doi: 10.1038/227955a0
Mass, A. M., and Supin, A. Y. (2007). Adaptive features of aquatic mammals’ eye. Anat. Rec. (Hoboken) 290, 701–715. doi: 10.1002/ar.20529
Maurice, D. M. (1957). The structure and transparency of the cornea. J. Physiol. 136, 263–286. doi: 10.1113/jphysiol.1957.sp005758
Meng, H., Matthaei, M., Ramanan, N., Grebe, R., Chakravarti, S., Speck, C. L., et al. (2013). L450W and Q455K COL8A2 knock-in mouse models of Fuchs endothelial corneal dystrophy show distinct phenotypes and evidence for altered autophagy. Invest. Ophthalmol. Vis. Sci. 54, 1887–1897. doi: 10.1167/iovs.12-11021
Meredith, R. W., Gatesy, J., Emerling, C. A., York, V. M., and Springer, M. S. (2013). Rod monochromacy and the coevolution of cetacean retinal opsins. PLoS Genet. 9:e1003432. doi: 10.1371/journal.pgen.1003432
Montgomery, S. H., Isabella, C., Chris, V., Barton, R. A., and Mundy, N. I. (2011). Adaptive evolution of four microcephaly genes and the evolution of brain size in anthropoid primates. Mol. Biol. Evol. 28, 625–638. doi: 10.1093/molbev/msq237
Murdy, E. O. (1989). A taxonomic revision and cladistic analysis of the oxudercine gobies (Gobiidae: Oxudercinae). Rec. Aust. Mus. Suppl. 11, 1–93. doi: 10.3853/j.0812-7387.11.1989.93
Nelson, J. S., Grande, T. C., and Wilson, M. V. H. (2016). “Phylum chordata,” in Fishes of the World, (Hoboken, NJ: John Wiley & Sons, Inc), 13–526. doi: 10.1002/9781119174844.ch2
Nursall, J. R. (1981). Behavior and habitat affecting the distribution of five species of sympatric mudskippers in Queensland. Bull. Mar. Sci. 31, 730–735.
Orme, D., Freckleton, R., Thomas, G., Petzoldt, T., Fritz, S., Isaac, N., et al. (2012). CAPER: Comparative Analyses of Phylogenetics and Evolution in R. R Package Version 1.0.1. Available online at: https://cran.r-project.org/package=caper.
Reuter, T., and Peichl, L. (2008). “Structure and function of the retina in aquatic tetrapods,” in Sensory Evolution on the Threshold: Adaptations in Secondarily Aquatic Vertebrates, eds J. G. M. Thewissen and S. Nummela (Berkeley: University of California Press), 149–172. doi: 10.1525/9780520934122-011
Ronquist, F., Teslenko, M., van der Mark, P., Ayres, D. L., Darling, A., Höhna, S., et al. (2012). MrBayes 3.2: efficient Bayesian phylogenetic inference and model choice across a large model space. Syst. Biol. 61, 539–542. doi: 10.1093/sysbio/sys029
Sayer, M. D. J. (2005). Adaptations of amphibious fish for surviving life out of water. Fish Fish. 6, 186–211. doi: 10.1111/jfb.12270
Shi, Y. S., and Yokoyama, S. (2003). Molecular analysis of the evolutionary significance of ultraviolet vision in vertebrates. Proc. Natl. Acad. Sci. U S A. 100, 8308–8313. doi: 10.1073/pnas.1532535100
Simmich, J., Temple, S. E., and Collin, S. P. (2012). A fish eye out of water: epithelial surface projections on aerial and aquatic corneas of the ‘four-eyed fish’ anableps anableps. Clin. Exp. Optom. 95, 140–145. doi: 10.1111/j.1444-0938.2011.00701.x
Steppan, S., Meyer, A. A., Barrow, L. N., Alhajeri, B. H., Al-Zaidan, A. S. Y., Gignac, P. M., et al. (2022). Phylogenetics and the evolution of terrestriality in mudskippers (Gobiidae: Oxudercinae). Mol. Phylogenet. Evol. 169:107416. doi: 10.1016/j.ympev.2022.107416
Subramanian, S., Ross, N. W., and MacKinnon, S. L. (2008). Comparison of antimicrobial activity in the epidermal mucus extracts of fish. Comp. Biochem. Physiol. B Biochem. Mol. Biol. 150, 85–92. doi: 10.1016/j.cbpb.2008.01.011
Sugawara, T., Terai, Y., Imai, H., Turner, G. F., Koblmueller, S., Sturmbauer, C., et al. (2005). Parallelism of amino acid changes at the RH1 affecting spectral sensitivity among deep-water cichlids from lakes Tanganyika and Malawi. Proc. Natl. Acad. Sci. U S A. 102, 5448–5453. doi: 10.1073/pnas.0405302102
Talavera, G., and Castresana, J. (2007). Improvement of phylogenies after removing divergent and ambiguously aligned blocks from protein sequence alignments. Syst. Biol. 56, 564–577. doi: 10.1080/10635150701472164
Thacker, C. E. (2003). Molecular phylogeny of the gobioid fishes (Teleostei: Perciformes: Gobioidei). Mol. Phylogenet. Evol. 26, 354–368. doi: 10.1016/s1055-7903(02)00361-5
Thacker, C. E. (2009). Phylogeny of Gobioidei and placement within Acanthomorpha, with a new classification and investigation of diversification and character evolution. Copeia 2009, 93–104. doi: 10.1643/ci-08-004
Thacker, C. E., Thompson, A. R., and Roje, D. M. (2011). Phylogeny and evolution of Indo-Pacific shrimp-associated gobies (Gobiiformes: Gobiidae). Mol. Phylogenet. Evol. 59, 168–176. doi: 10.1016/j.ympev.2011.02.007
Vaidya, G., Lohman, D. J., and Meier, R. (2011). SequenceMatrix: concatenation software for the fast assembly of multi-gene datasets with character set and codon information. Cladistics 27, 171–180. doi: 10.1111/j.1096-0031.2010.00329.x
Wolf, J. B. W., Künstner, A., Nam, K., Jakobsson, M., and Ellegren, H. (2009). Nonlinear dynamics of nonsynonymous (dN) and synonymous (dS) substitution rates affects inference of selection. Genome Biol. Evol. 1, 308–319. doi: 10.1093/gbe/evp030
Xia, Y., Cui, Y. M., Wang, A. S., Liu, F. N., Chi, H., Potter, J. H. T., et al. (2021). Convergent phenotypic evolution of rhodopsin for dim-light sensing across deep-diving vertebrates. Mol. Biol. Evol. 38, 5726–5734. doi: 10.1093/molbev/msab262
Xu, S. X., Sun, X. H., Niu, X., Zhang, Z. P., Tian, R., and Ren, W. H. (2017). Genetic basis of brain size evolution in cetaceans: insights from adaptive evolution of seven primary microcephaly (MCPH) genes. BMC Evol. Biol. 17:206. doi: 10.1186/s12862-017-1051-7
Yang, Z. (2007). PAML 4: Phylogenetic analysis by maximum likelihood. Mol. Biol. Evol. 24, 1586–1591. doi: 10.1093/molbev/msm088
Yokoyama, S., Tada, T., Zhang, H., and Britt, L. (2008). Elucidation of phenotypic adaptations: molecular analyses of dim-light vision proteins in vertebrates. Proc. Natl. Acad. Sci. U S A. 105, 13480–13485. doi: 10.1073/pnas.0802426105
You, X. X., Bian, C., Zan, Q. J., Xu, X., Liu, X., Chen, J. M., et al. (2014). Mudskipper genomes provide insights into the terrestrial adaptation of amphibious fishes. Nat. Commun. 5:5594. doi: 10.1038/ncomms6594
You, X. X., Sun, M., Li, J., Bian, C., Chen, J. M., Yi, Y. H., et al. (2018). Mudskippers and their genetic adaptations to an amphibious lifestyle. Animals 8:24. doi: 10.3390/ani8020024
Keywords: mudskippers, cornea, microstructure, COL8A2, purifying selection
Citation: Hu W, Mu Y, Lin F, Li X and Zhang J (2022) New Insight Into Visual Adaptation in the Mudskipper Cornea: From Morphology to the Cornea-Related COL8A2 Gene. Front. Ecol. Evol. 10:871370. doi: 10.3389/fevo.2022.871370
Received: 08 February 2022; Accepted: 15 March 2022;
Published: 07 April 2022.
Edited by:
Lin Zhang, Hubei University of Chinese Medicine, ChinaReviewed by:
Inge Seim, Nanjing Normal University, ChinaCopyright © 2022 Hu, Mu, Lin, Li and Zhang. This is an open-access article distributed under the terms of the Creative Commons Attribution License (CC BY). The use, distribution or reproduction in other forums is permitted, provided the original author(s) and the copyright owner(s) are credited and that the original publication in this journal is cited, in accordance with accepted academic practice. No use, distribution or reproduction is permitted which does not comply with these terms.
*Correspondence: Yuan Mu, bXV5QGVhc3Rlcm4taGltYWxheWEuY24=; Jie Zhang, emhhbmdqaWVAaW96LmFjLmNu
Disclaimer: All claims expressed in this article are solely those of the authors and do not necessarily represent those of their affiliated organizations, or those of the publisher, the editors and the reviewers. Any product that may be evaluated in this article or claim that may be made by its manufacturer is not guaranteed or endorsed by the publisher.
Research integrity at Frontiers
Learn more about the work of our research integrity team to safeguard the quality of each article we publish.