- 1Behavioral Physiology, Institute of Biology, Humboldt-Universität zu Berlin, Berlin, Germany
- 2Department of Biology, McGill University, Montréal, QC, Canada
To understand animal ecology, observation of wildlife in the natural habitat is essential, but particularly challenging in the underwater realm. Weakly electric fishes provide an excellent opportunity to overcome some of these challenges because they generate electric organ discharges (EODs) to sense their environment and to communicate, which can be detected non-invasively. We tracked the EOD and swimming activity of two species of mormyrid weakly electric fishes (Marcusenius victoriae and Petrocephalus degeni) over diel cycles in the laboratory, and we recorded EODs and environmental dissolved oxygen (DO) concentration and temperature over several months in a naturally hypoxic habitat in Uganda. Under laboratory conditions, both species showed increases of activity and exploration behavior that were closely synchronized to the onset of the dark phase. In the wild, fish preferred structurally complex habitats during the day, but dispersed toward open areas at night, presumably to forage and interact. Nocturnal increase of movement range coincided with diel declines in DO concentration to extremely low levels. The fact that fish showed pronounced nocturnal activity patterns in the laboratory and in the open areas of their habitat, but not under floating vegetation, indicates that light intensity exerts a direct effect on their activity. We hypothesize that being dark-active and tolerant to hypoxia increases the resistance of these fish against predators. This study establishes a new technology to record EODs in the field and provides a window into the largely unknown behavior of mormyrids in their natural habitat.
Introduction
From the morning songs of birds to the seasonal migrations of salmon, rhythmic patterns of behavior are ubiquitous in nature across all taxa of animals (Wulund and Reddy, 2015; Häfker and Tessmar-Raible, 2020). Rhythmic behavior is driven by cyclical (abiotic) shifts in environmental conditions, such as the day/night cycle, the lunar cycle, and seasonal changes, e.g., in temperature and rainfall. The predictable nature of these cycles has made it possible for organisms to adapt to, and anticipate, environmental change in order to optimize the use of resources and environmental conditions (Häfker and Tessmar-Raible, 2020).
Many aquatic habitats are characterized by rhythmic changes in environmental conditions, although these may differ from terrestrial cycles. Light and temperature, reliable terrestrial diel time cues (Hut et al., 2013), can be less predictable underwater. In deep and/or turbid water bodies, ambient light levels may reach values where day and night are indistinguishable. The large heat capacity of water and other factors, such as rainfall or vertical mixing, can affect daily temperature cycles (Hut et al., 2013; Häfker and Tessmar-Raible, 2020). Fluctuations of dissolved oxygen (DO) concentration on the other hand, are mostly negligible on land but can be subject to pronounced rhythms in water bodies. Reduced input of photosynthetically produced oxygen during the night affects diel shifts in DO concentration. Seasonal effects, such as ice cover and rainfall can shift DO concentration over a longer timescale (e.g., Greenbank, 1945; Talling, 1957; Kannan and Job, 1980; Townsend, 1999). Alongside DO levels, pH and CO2 concentration can fluctuate substantially on a diel and seasonal timescale (Semesi et al., 2009; Baumann et al., 2015). In tropical ecosystems, increased rainfall during the wet season can change inundation levels, flush nutrients and pollutants into water bodies and thereby change their hydrochemistry drastically (Adebisi, 1981; Hamilton, 2010).
Adaptive behavioral rhythms are just as commonly found in marine and freshwater habitats as on land (Naylor, 2010; Häfker and Tessmar-Raible, 2020). Among these, rhythms that occur on a diel timescale have been studied most intensively. Many aspects of fish behavior follow such diel rhythms, for example, shelter use (Vanderpham et al., 2012), swimming activity (Tabata et al., 1989; Hurd et al., 1998), food intake (Boujard and Leatherland, 1992; Sánchez-Vázquez et al., 1995), foraging behavior and migration (Hobson, 1973; Neilson and Perry, 1990; Ibbotson et al., 2006), and the formation of fish assemblages (Rooker and Dennis, 1991; Arrington and Winemiller, 2003). On a longer timescale, migration and reproductive behavior have been found in many species to be linked to lunar, seasonal, and annual rhythms (reviewed in Volpato and Trajano, 2005).
The study of activity rhythms in the field is challenging, and this is particularly true for the study of aquatic animals, mostly due to the logistical challenges and limitations of underwater sampling methods that are associated with them. For example, most studies on fish activity rhythms that are carried out in tropical regions are based on direct observation (Volpato and Trajano, 2005; Frehse et al., 2020, 2021). These studies are subject to a sampling bias that favors species that are diurnal and live in clear water. Hence, natural behavioral patterns of more elusive fish species, such as nocturnal fishes, remain largely unknown. In this respect, weakly electric fishes offer unique opportunities for behavioral observations in their natural habitat. They generate an electric field around their body by emitting species-specific electric organ discharges (EODs, Lissmann, 1951), which they use to navigate and forage (Lissmann, 1958; Lissmann and Machin, 1958), and to communicate with conspecifics (Moller, 1970; Moller and Bauer, 1973; Carlson, 2002). By recording their EODs with electrodes, the presence, activity state, number, and (if the EOD waveform is known) species of weakly electric fish can be detected in a water body without the need for visual or acoustic sampling (e.g., Lissmann and Schwassmann, 1965; Henninger et al., 2018, 2020; Madhav et al., 2018; Migliaro et al., 2018).
Weakly electric fishes are widespread in tropical freshwater habitats in Central and South America and throughout Africa where they constitute important food web components (Lundberg et al., 1987; Moller, 1995; Crampton, 1996). They are generally considered nocturnal animals, and this has been documented in some species that show nocturnal increases of locomotor and EOD activity in the laboratory (Harder et al., 1964; Bässler et al., 1979; Cobert, 1984; Franchina and Stoddard, 1998; Markham et al., 2009; Migliaro and Silva, 2016). Field studies generally support the notion of nocturnality in weakly electric fishes (Lissmann, 1961; Lissmann and Schwassmann, 1965; Kruger, 1973; Moller et al., 1979; Henninger et al., 2018, 2020; Madhav et al., 2018; Migliaro et al., 2018). However, a close examination of this body of research shows that there are examples of weakly electric fish that remain active during the day (e.g., in dark habitats, Kruger, 1973; Hopkins, 1980), and that nocturnal activity patterns have often been observed in shallow and/or clear water habitats, where light conditions change drastically from day to night (Lissmann, 1961; Lissmann and Schwassmann, 1965; Henninger et al., 2018, 2020). Thus, it seems that the activity of weakly electric fishes in the wild corresponds more closely to ambient light levels than to the phase of the diel cycle. It is therefore likely that activity patterns of many of the >450 species of weakly electric fishes (Crampton, 2019) depend on light conditions among other exogenous and endogenous factors, and that these animals might not always classify as nocturnal. Previous studies have shown that fishes can display extraordinary plasticity in their diel and circadian activity rhythms (reviewed in Reebs, 2002). Other factors than illumination, such as social stimuli (Franchina et al., 2001; Silva et al., 2007) and temperature (Dunlap et al., 2000; Ardanaz et al., 2001), can affect activity, and the interplay of these and other, hitherto unknown environmental influences, might shape natural behavioral patterns that differ from those observed in the laboratory. An environmental factor whose influence on diel behavior has been studied little in the field is ambient DO (Pollock et al., 2007). Many weakly electric fishes experience seasonal, diel, or chronic hypoxia (Chapman et al., 1996b; Crampton, 1998). Usually, DO reaches the lowest levels during the night due to respiration and lack of photosynthesis. The degree to which weakly electric fish can tolerate hypoxia might be limited by the energetic costs for generation and sensing of EODs (Salazar and Stoddard, 2008; Salazar et al., 2013; Lewis et al., 2014; Sukhum et al., 2016). With the large ionic currents underlying EOD generation (Markham et al., 2009), electric organs are considered to be energetically similarly expensive tissue as brains, but make up a larger proportion of body mass than brain tissue (Markham et al., 2016). Little is known about how environmental rhythms, such as diel patterns of hypoxia, affect the activity of weakly electric fish, and how representative laboratory studies are of natural activity patterns of fish.
The aim of this study was to investigate and compare activity and habitat use of mormyrid weakly electric fish in the laboratory and in the wild. Marcusenius victoriae and Petrocephalus degeni occur in a severely hypoxic swamp habitat in Uganda and show a high degree of hypoxia tolerance (Chapman and Chapman, 1998; Clarke et al., 2020; Moulton et al., 2020). They belong to different subfamilies of the Mormyridae, Mormyrinae and Petrocephalinae, respectively, and differ in their electrosensory anatomy, overall morphology, and social behavior (Carlson, 2016). Marcusenius victoriae are medium sized fish (usually 7–15 cm standard length) that are found predominantly alone in nature and show a high degree of intraspecific competition for shelters (Carlson, 2016). Petrocephalus degeni are shorter fish (usually 5–9 cm standard length) that are often found in groups and show pronounced social affiliation behavior (Carlson, 2016). Both species produce very short (ca. 300–500 μs), bi- or triphasic EOD pulses with a species-specific waveform (Supplementary Figure 1A). The diel variation of DO in their swamp habitat has not been quantified yet, and no detailed description of their diel habitat use and activity rhythms exists.
We conducted laboratory and field work to assess behavioral patterns under controlled and natural conditions. We captured fish from their swamp habitat and acclimated them to standardized laboratory housing conditions for one year before measuring diel patterns of EOD and locomotor activity in a 12:12 h light:dark environment under constant temperature and DO. To assess the behavioral patterns of M. victoriae and P. degeni in their habitat, we developed and built autonomous EOD recording devices. We measured the diel cycling of environmental temperature and DO, and recorded EODs in three habitats within a swamp lagoon system between July and September 2019 to document diel patterns of activity and habitat use, and abiotic environmental cycles.
Materials and Methods
Laboratory Activity Measurements
Activity of laboratory-housed Marcusenius victoriae and Petrocephalus degeni was measured at Humboldt-Universität zu Berlin, Germany. The fish were wild caught in the Lwamunda Swamp that surrounds Lake Nabugabo in Uganda (Figure 1) and transferred to the animal housing facility of the Institut für Biologie of Humboldt-Universität zu Berlin, where they were held under controlled conditions for at least one year prior to experiments. Marcusenius victoriae were housed individually in 70 l tanks, and P. degeni were kept in groups of up to three fish in 70 l tanks. The light regime was set to a 12:12 h light:dark cycle with 30 min dusk/dawn periods during which light levels gradually transitioned between light and dark. All fish were in non-breeding condition.
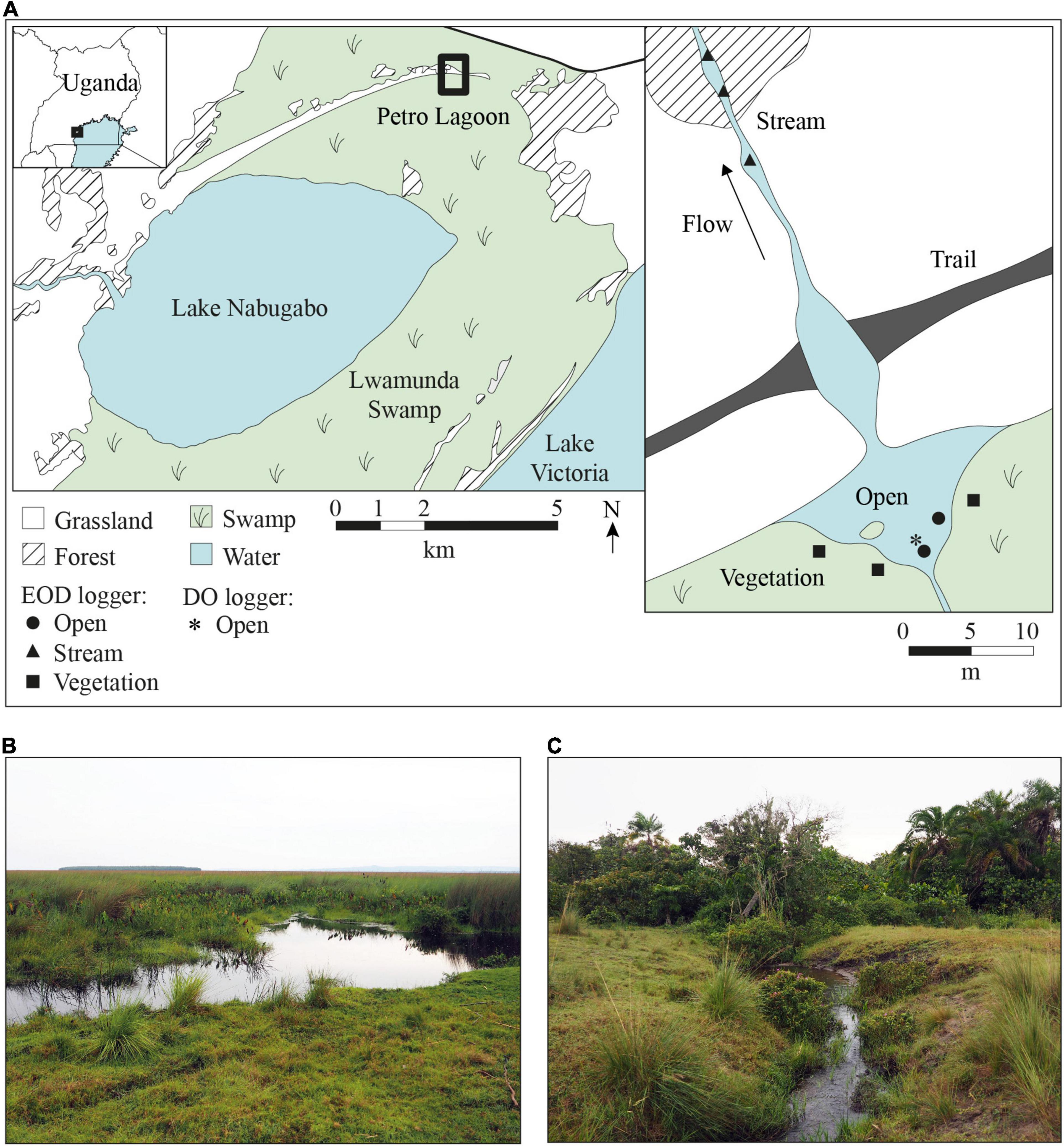
Figure 1. Map of Lake Nabugabo and Petro Lagoon, Uganda. (A) Lake Nabugabo is separated from Lake Victoria by the extensive Lwamunda Swamp, which surrounds Lake Nabugabo to the north, east and south. To the west, Lake Nabugabo is bordered by forest and grassland. The map on the right shows a close up of Petro lagoon, where water from the swamp collects in the pond area and flows outwards from the swamp to seasonally inundated grassland. The outflow is intersected by a trail that crosses through a shallow section of the stream. EODs were recorded in three habitats in the lagoon (open, stream and vegetation). Positions of EOD loggers are indicated by filled symbols. The asterisk marks the position of the DO and temperature logger. (B) The pond area of Petro Lagoon where the open and vegetation habitat are located. (C) The small stream-like area of the lagoon where water flows seasonally from the wetland to the inland. Map data were obtained from satellite images (Google Earth, https://earth.google.com/web/, accessed 26.07.2021) and our own measurements at Petro Lagoon.
Activity was quantified in a shuttle-box system (Figure 2; Loligo Systems Inc., Viborg, Denmark). The shuttle-box system consisted of two circular compartments (diameter of 50 cm each), that were connected by a central passage (8.5 cm wide and 14 cm long). Two opaque PVC tubes (5.5 cm inner diameter, 16 cm length) were placed symmetrically, one per compartment, to serve as shelter for the fish during the experiments. A piece of plexiglas was glued to the bottom of the shelter tubes to secure their position against disturbances. Water temperature was maintained by a thermostat (ITC-308, Shenzhen Inkbird Technology Co., Ltd., Shenzhen, China) that controlled the power supply to two silicone rubber heating mats on either side of the shuttle-box system and one heat radiator that was placed near the setup. The temperature sensor of the thermostat was submerged in the passage between the two compartments of the shuttle-box system. Two pumps (Universal Pumpe 1048, EHEIM GmbH & Co., KG, Deizisau, Germany) created a weak circular current in both compartments to homogenize temperature gradients in the system. The shuttle-box system rested on a self-constructed LED panel, which illuminated the system from below with white light during the day and infrared light during the night. The LED panel was controlled by a programmable LED timer (TC420, Shenzhen Leynew Technology Co., Ltd., Shenzhen, China). The light:dark cycle was set to synchronize with the light regime in the animal housing facility. Light intensity in the shuttle-box was ca. 48 lux during the light phase and 0 lux during the dark phase (Supplementary Figure 2). During the dark phase, the setup was illuminated with infrared light (940 nm wavelength) to enable video tracking of the animals. A camera (Grasshopper3 GS3-U3-41S4C-C 1, Teledyne FLIR LLC., Wilsonville, OR, United States) was mounted above the shuttle-box to record swimming activity of the fish. Videos were recorded at sample rates of 25–40 frames per second using Matlab R2018. To minimize disturbance, the setup was visually isolated from the surrounding area with lightproof fabric that was mounted on an aluminum frame around the shuttle-box system, LED panel, and camera. A Faraday cage made of steel mesh was mounted on the same aluminum frame and connected to an electric ground to reduce electric noise. EODs were recorded with carbon rod electrodes that were submerged in both compartments of the shuttle-box. During experiments with M. victoriae, one pair of electrodes was used in each compartment. Due to the smaller peak-to-peak amplitude of EODs of P. degeni, two pairs of electrodes in each compartment were necessary to reliably record their EODs during activity measurements. EODs were band-pass filtered (100 Hz – 20 kHz), amplified (10 x gain, DPA-2FS, NPI, Germany), digitized at a sample rate of 50 kHz (NI-PCI 6259, National Instruments, Austin, TX, United States), and stored on a computer using Matlab R2018. Videos and EOD recordings were synchronized using a LED light on the side of the LED panel, which signaled the beginning of each new EOD recording.
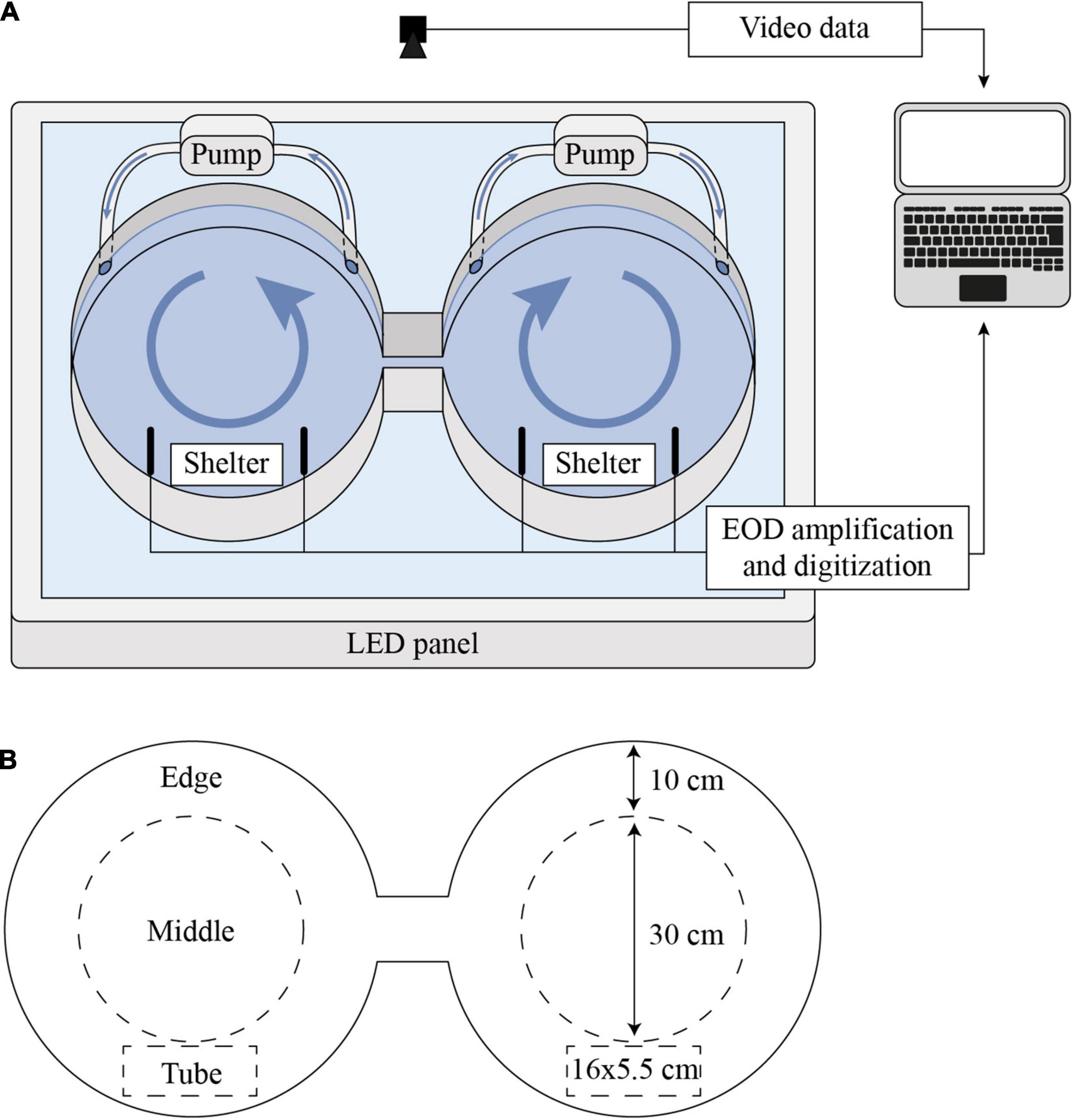
Figure 2. Schematic of shuttle-box setup for behavioral activity measurements. (A) Experimental setup. The setup was illuminated from below by a LED panel and videos were recorded from above. EODs were recorded with carbon rod electrodes in both compartments that were arranged to both sides of each PVC shelter tube and, in experiments with P. degeni, with an additional pair of electrodes per compartment that was arranged on a perpendicular axis. Pumps created a slow circular current in both compartments. The heating system is not shown here for better clarity. (B) Definition of zones in shuttle-box tank. Edge: within 10 cm of shuttle-box wall; middle: more than 10 cm away from shuttle-box wall; tube: in PVC tube.
For each trial, one fish was introduced in a randomly chosen compartment of the shuttle-box in the late afternoon, before the onset of the dark phase, and left undisturbed for 42 h. Over the duration of the trial, movements and EOD activity were recorded continuously. After conclusion of the trial, the fish was removed from the shuttle-box system, weighed and measured, and returned to its home tank. Between two trials, the shuttle-box was cleaned with salt water and rinsed to remove residues from the previous trial. All experiments were approved by the German Landesamt für Gesundheit und Soziales (project number G0278/17).
Shuttle-Box Data Analysis
Videos recorded from the shuttle-box were overlaid with a mask to obscure the parts of the image that did not show the shuttle-box system and increase tracking speed using FFMPEG ver. 4.41. Fish position was then tracked using the Biotracker 3 software (Mönck et al., 2018). The resulting dataset was further processed using R. High-velocity jumps of the track position (>400 cm/s) that occurred, e.g., when fish traversed their opaque shelter tubes or when the tracking position was transiently lost, were corrected by connecting the two positions before and after the jump with a series of equally interspaced steps. Small jitters of the track position were then smoothed using a second-order Savitzky–Golay filter (Savitzky and Golay, 1964). Tracking data were annotated with categorical information about the position of the fish based on the x and y coordinates of the track position. For this, three zones in the shuttle-box tank were defined: shelter tube (tube), within 10 cm of the edge (edge), more than 10 cm away from the edge (middle, Figure 2B). To quantify swimming behavior, the mean swimming speed and the percentage of time spent in the tube, middle, and/or edge of the shuttle-box system were summarized for every 10 min of the trial.
EODs were extracted offline from shuttle-box recordings using Python ver. 3.8.82 with a custom-written Python script and peak-finding functions from the Thunderfish library3. Signals were identified using a peak-finding algorithm, which detected the positive and negative peak of each EOD pulse above a preset amplitude threshold of 0.1 V. A short data fragment (1 ms) centered around the zero crossing of each EOD was extracted, and sample rate was interpolated to 200 kHz for waveform analysis. Several EOD features were extracted from each EOD: peak-to-peak duration, peak-to-peak amplitude, amplitude ratio of positive/negative peak, and the frequency of the highest power of the FFT-transformed pulse. To quantify changes in electric signaling during the trial, EOD rate, inter-pulse-interval coefficient of variation (IPI CV), EOD amplitude CV, and EOD waveform features were summarized for every second and every 10 min of the trial.
For analysis of location preference, resting EOD rate, and the correlation of EOD rate and velocity, only data from the last 24 h of each trial were used to reduce the effect of acclimation to the shuttle-box system. Location preference was computed as the percentage of total time that fish spent at different locations of the shuttle-box. Resting EOD rate was calculated from sequences of the recording when fish spent at least 5 s in their shelter tube and EOD amplitude remained constant (coefficient of variation < 0.12), suggesting low locomotor activity (Silva et al., 2007).
In situ Recordings
Study Site
We recorded EOD activity in the natural habitat of M. victoriae and P. degeni between July and September 2019 in the Lwamunda Swamp, a wetland surrounding Lake Nabugabo in Uganda (Figure 1). Separated from Lake Victoria about 5000 years ago (Stager et al., 2005), Lake Nabugabo is a small satellite lake (surface area = 33 km2, mean depth = 3.13 m, Nyboer and Chapman, 2013) with a unique composition of fish species, such as a small number of haplochromine cichlid species that are endemic to Lake Nabugabo and nearby satellite lakes (Trewavas, 1933; Greenwood, 1965; Kaufman and Ochumba, 1993) and four species of mormyrid weakly electric fishes (Ogutu-Ohwayo, 1993; Chapman et al., 2002). In the years following the introduction of the predatory Nile Perch in the 1960s (Ogutu-Ohwayo, 1993; Pringle, 2005), Lake Nabugabo suffered a dramatic decline or disappearance of several native species from the waters of the main lake (Ogutu-Ohwayo, 1993; Chapman et al., 2003). The lake is surrounded by an extensive wetland area (Lwamunda Swamp), which is dominated by the emergent grass Miscanthidium violaceum that transitions to water lilies (Nymphaea lotus and N. carulea) or hippo grass (Vossia cuspidata) at the lake edge (Chapman et al., 1996a; Chrétien and Chapman, 2016). Various fish species occur deep within the wetland (see species list in Chapman et al., 1996b), including P. degeni and M. victoriae (Chapman et al., 1996b,2002; Chapman and Hulen, 2001; Moulton et al., 2020). These fishes are protected from predation by Nile perch, as this species does not penetrate the dense swamp and is sensitive to hypoxia (Chapman et al., 2002). Inundation levels, water temperature and DO in the wetland show seasonal and diel variation, though DO remains generally low (previously reported diurnal ranges: 1.06–2.63 mg O2 L-1, Reardon and Chapman, 2008; 0.69–0.82 mg O2 L-1, Moulton et al., 2020), and water conductivity is very low (10–20 μS cm1, measured manually during sampling). All research conducted in Uganda was approved by the Uganda National Council for Science and Technology (research clearance nr. 10601).
Electric Fish Loggers
We developed an autonomous data-logging device to record electric signals in the natural habitat of weakly electric fishes. The electric fish logger consists of a Teensy 3.5 microcontroller board (PJRC.COM, LLC., Sherwood, OR, United States) that is connected to a DS3231 real-time clock module (AZ-Delivery Vertriebs GmbH, Deggendorf, Germany) for timekeeping functionality, and powered by four AA batteries. The electronic components of the logger are housed in a PVC tube with an inner diameter of 45 mm, that is sealed on one side with a glued-on lid and on the other side with a screw lid, which allows replacement of batteries and the microSD card. Two small carbon disks (1 cm diameter, 4 mm long) serve as electrodes to measure EODs. The disks are glued into the lids of the logger housing using aquarium silicone and connected to the analog-digital converters of the Teensy board via copper wire.
The logger continuously records differential voltage through its electrodes with a dynamic input range between –3.3 V and +3.3 V. Voltage data were digitized at a sample rate of 100 kHz with a 12-bit resolution, and stored on a microSD card. The program for the logger was written using the Arduino IDE4 and the Teensyduino plugin5. The code for the logger program has been archived and is maintained online on GitHub, alongside a more detailed documentation6.
The detection range of the electric fish logger depends on the amplitude of the signal that is generated by the fish. Due to the dipole character of the electric field, the recorded EOD amplitude depends on the distance and the position of the fish relative to the electrodes (see Benda, 2020 for a comprehensive review of electric field geometry). As the two poles of the electric field are located on the head-to-tail axis of the fish, EOD amplitude is largest when the measurement electrodes align with this axis, and practically reaches zero when the fish is perpendicular to the line connecting the two measurement electrodes. Thus, there is no fixed detection range for the loggers, and we rather estimated detection probability. To do this, we recorded freely swimming specimens of each species (n = 4 per species, see Supplementary Material) in a rectangular tank (200 cm × 100 cm). Detection probability decreased with distance, following an exponential decay function, and was overall lower for P. degeni, approaching zero at 50 cm radial distance from the logger (Supplementary Figure 3). Detection probability for EODs of M. victoriae reached about 10% at the maximum distance of 180 cm. All fish used for detection probability estimation in the lab were in non-breeding condition.
In situ Electric Organ Discharges Sampling
Eight electric fish loggers were deployed in a small lagoon (Petro Lagoon, 0°19′07′′S, 31°56′48′′E) in the Lwamunda Swamp in three different habitats: under floating vegetation with ca. 1 m distance to the edge of vegetation cover (vegetation, n = 3), under the open water surface of the lagoon with at least 1 m distance to the nearest vegetation (open, n = 2), and in the middle of the small stream-like area of the lagoon where water flows seasonally from the wetland to the inland (stream, n = 3). These habitats were chosen to capture a variety of environmental conditions that is representative of the conditions experienced by fish in the lagoon (Table 1). To secure the logger position against perturbations, each logger was attached to a stick that was driven firmly into the ground. Each logger was placed approximately in the middle of the water column and with at least 3 m distance to the nearest logger.
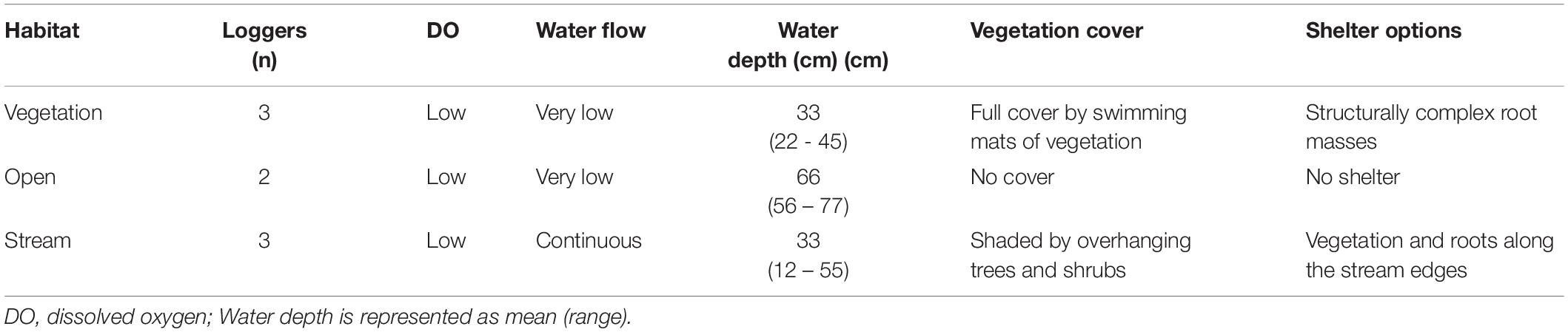
Table 1. Characteristics of different habitats in Petro Lagoon situated in the Lwamunda Swamp of the Lake Nabugabo System.
In situ recordings were made on six sampling sessions in 2019 (July: 23–24 and 29–30, August: 8–9 and 21–22, September: 2–3 and 19–20). Electric fish loggers were deployed in the morning and left to record continuously for at least 24 h. After retrieval of the loggers, EODs were extracted from recordings as described above, using Python ver. 3.8.8 (see footnote 2, accessed December 2021) with a custom-written Python script and functions from the Thunderfish library (see footnote 3, accessed December 2021). Only EODs with peak-to-peak amplitudes above 0.066 V (1% of the dynamic range of the logger) were extracted. EODs were then grouped together into encounters based on their zero-crossing timestamp using R ver. 4.1.07. An encounter was defined as a coherent EOD pulse train with at least 10 pulses and a maximum inter-pulse interval (IPI) of 5 s. These selection criteria served to eliminate very brief and/or erroneous fish detections that occurred due to electrical noise, e.g., through lightning discharges. For each encounter, EODs were assigned to either M. victoriae or P. degeni based on their EOD waveform, using mixture discriminant analysis (MDA) and EOD features. To create MDA models, EOD features from fish that had been recorded under laboratory conditions were used (see Supplementary Material). Three features, peak-to-peak duration, peak 1/peak 2 amplitude ratio, and the frequency of the highest power of the FFT-transformed pulse, were sufficient for robust species discrimination with a fidelity of 98.3% (Supplementary Table 1). The MDA model was then applied to predict the species for each EOD pulse in the field recordings. After species prediction via MDA, the EOD pulse train of each encounter was inspected visually to determine the number and verify the species of the fish. Encounters with single fish that could not be assigned with confidence (i.e., less than 70% of pulses could be assigned to either species) were removed from analysis.
As individual fish of a given species could not be identified unambiguously based on their EOD features, it is likely that some animals were encountered multiple times. This is even more likely for M. victoriae, which have been described as territorial (Carlson, 2016). Although, in theory, absolute animal densities may be estimated despite double counting of individuals (Campos-Candela et al., 2018; Follana-Berná et al., 2020), the data required to satisfy the assumptions underlying such methods (e.g., fish must display home ranging behavior and home range centers must be homogeneously distributed, Campos-Candela et al., 2018) are not available for this study system. Thus, data on fish distribution are presented as frequency of encounters, which depends on fish activity as well as fish density. Frequency of encounters was calculated as the total number of fish that were encountered per logger over intervals of 30 min. Duration of encounters (from first to last EOD) and EOD rate during encounters were extracted to compare encounter characteristics between habitats and periods of the diel cycle. For the comparison of encounter characteristics, only events with a single fish were used, as they occurred most consistently across time and space.
In situ Temperature and Dissolved Oxygen Sampling
One oxygen and temperature logger with optical oxygen sensor (miniDOT, PME, Vista, CA, United States) was placed in the open habitat of the lagoon to record water conditions on the same sampling days as in situ EOD recordings were made. Water temperature and DO concentration were sampled at least every 30 min for the duration of EOD recordings. After each sampling day, data were collected from the logger, and the first and last 30 min of measurements were deleted to remove disturbances in the measurements that were caused by placing and retrieving the logger. Previous manual measurements that were taken during the daytime at this site between 2005 and 2018 showed that temperature varies on average by 0.2 ± 0.5°C and air saturation varies by 1.8 ± 7.9% between the water surface and at a water depth of 50 cm (n = 690 measurements). As we took the measurements for this study in the middle of the water column, we expect our values to be representative of large parts of the water column where the fish are located. In addition to continuous measurements, diurnal temperature and DO were recorded in all habitats twice per sample day using a handheld meter (Handy Polaris, OxyGuard, Farum, Denmark).
Results
All values are represented as mean ± standard deviation (SD) if not otherwise stated.
Activity Patterns in the Laboratory
The activity patterns of M. victoriae and P. degeni in the lab corresponded closely to ambient light levels, with long phases of inactivity and strong shelter preference during the light phase and increased activity and exploration behavior during the dark phase of the trial (Figure 3).
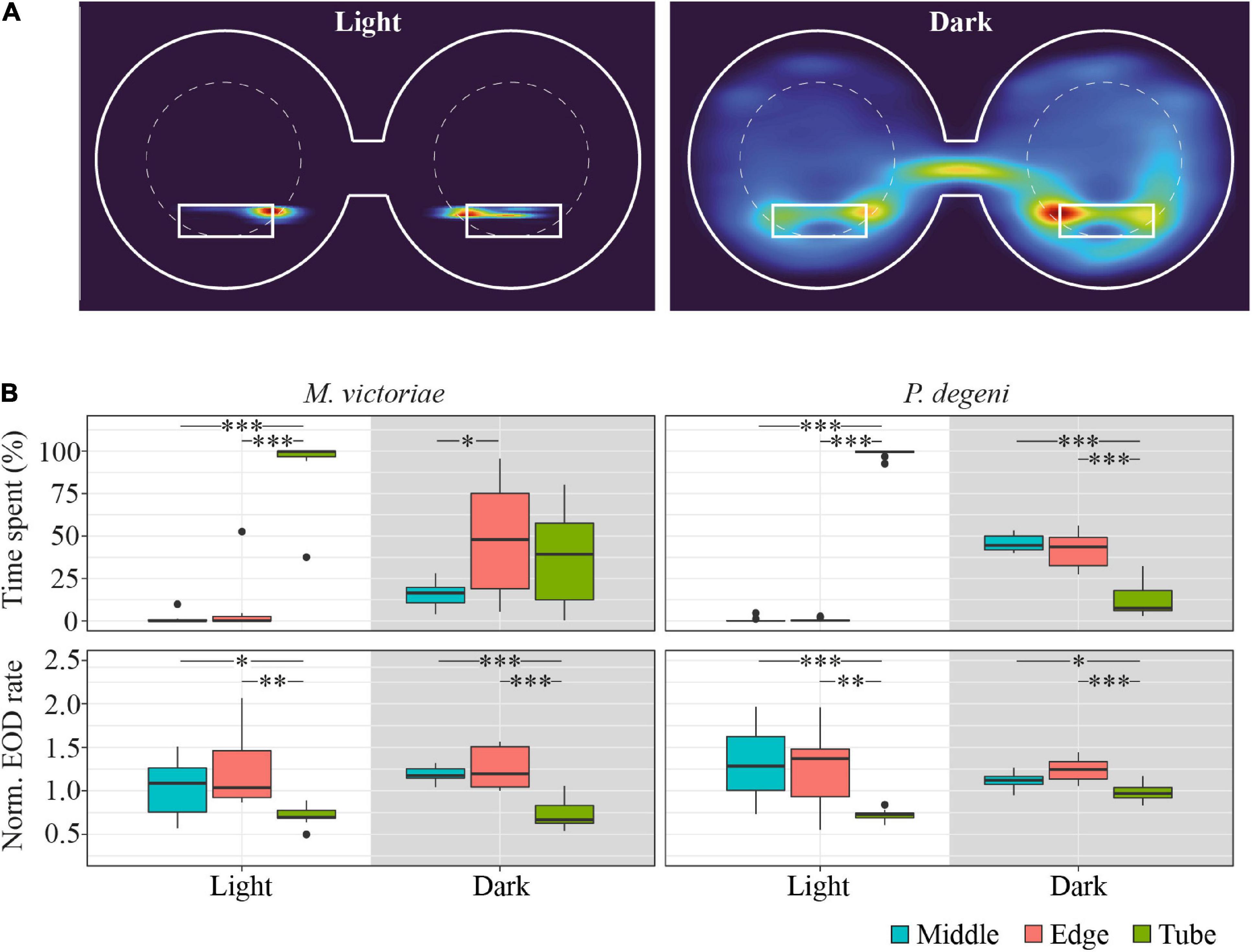
Figure 3. Example heatmap, location preference and EOD rates in the shuttle-box. (A) Heatmap of swim activity of one P. degeni during the light and the dark phase. Warmer colors indicate that the fish spent more time at this location. The margins of the shuttle-box tank and the two shelter tubes are indicated with solid white lines, the area boundaries between edge and middle are indicated with dashed white lines. Two-dimensional kernel density estimation was used to convert X-Y coordinates from tracked video data (downscaled to 1 frame per second) into a probability density function for the location of the fish. (B) Top row: proportion of time fish spent in different locations in the shuttle-box as percentage of total time. Bottom row: normalized EOD rates, averaged per fish, period of the light cycle, and area of the shuttle-box (M. victoriae: n = 11; P. degeni: n = 10; light phase EOD rates: Conover test; rest: Wilcoxon test: p < 0.05*, p < 0.01**, p < 0.001***). Overall M. victoriae emitted EODs with a rate of 8.0 ± 0.9 Hz (day) and 11.1 ± 1.1 Hz (night); P. degeni with 8.0 ± 0.4 Hz (day) and 12.3 ± 0.3 Hz (night).
During the light phase of the trial, Marcusenius victoriae spent 93.1 ± 18.5% and P. degeni spent 98.7 ± 2.4% of the time in a shelter tube, which decreased during the dark phase to 36.4 ± 27.5% and 12.5 ± 10.0% percent, respectively. During the dark phase, M. victoriae showed no preference for either shelter tube or unsheltered areas of the shuttle-box (edge and middle). However, when fish were located outside of the shelter tube, they spent significantly more time close to the edge of the shuttle-box than in the middle. Throughout the light cycle, M. victoriae emitted EODs at lower rates in the shelter tube than in the unsheltered areas of the shuttle-box. Petrocephalus degeni spent significantly more time in the unsheltered areas of the shuttle-box during the dark phase without a preference for the edge or the middle of the shuttle-box tank. Similar to M. victoriae, P. degeni emitted EODs at lower rates in the shelter tube during the light phase. However, and in contrast to M. victoriae, there was no difference in EOD rates between tank areas during the dark phase (Figure 3B).
Swim speed and EOD rate of both species increased sharply during the 30-min dusk period, peaked at the onset of complete darkness, and remained at elevated levels for the entire duration of the dark phase (Figure 4A). The dark phase proportion of the total distance that fish swam within 24 h was 96.3 ± 7.8% for M. victoriae and 97.7 ± 4.6% for P. degeni. There was no evidence of anticipation of the dark phase (i.e., no increase of EOD rate or swim activity before the dusk period). During the light phase, EOD rate decreased steadily in contrast to swim speed, which dropped quickly close to 0 body lengths per second (BL s–1) at the onset of the light phase and remained there until the dusk period. Despite this difference, EOD rate was overall positively correlated with swim speed throughout the light and dark phases for both species (linear regressions: p < 0.001; Figure 4B).
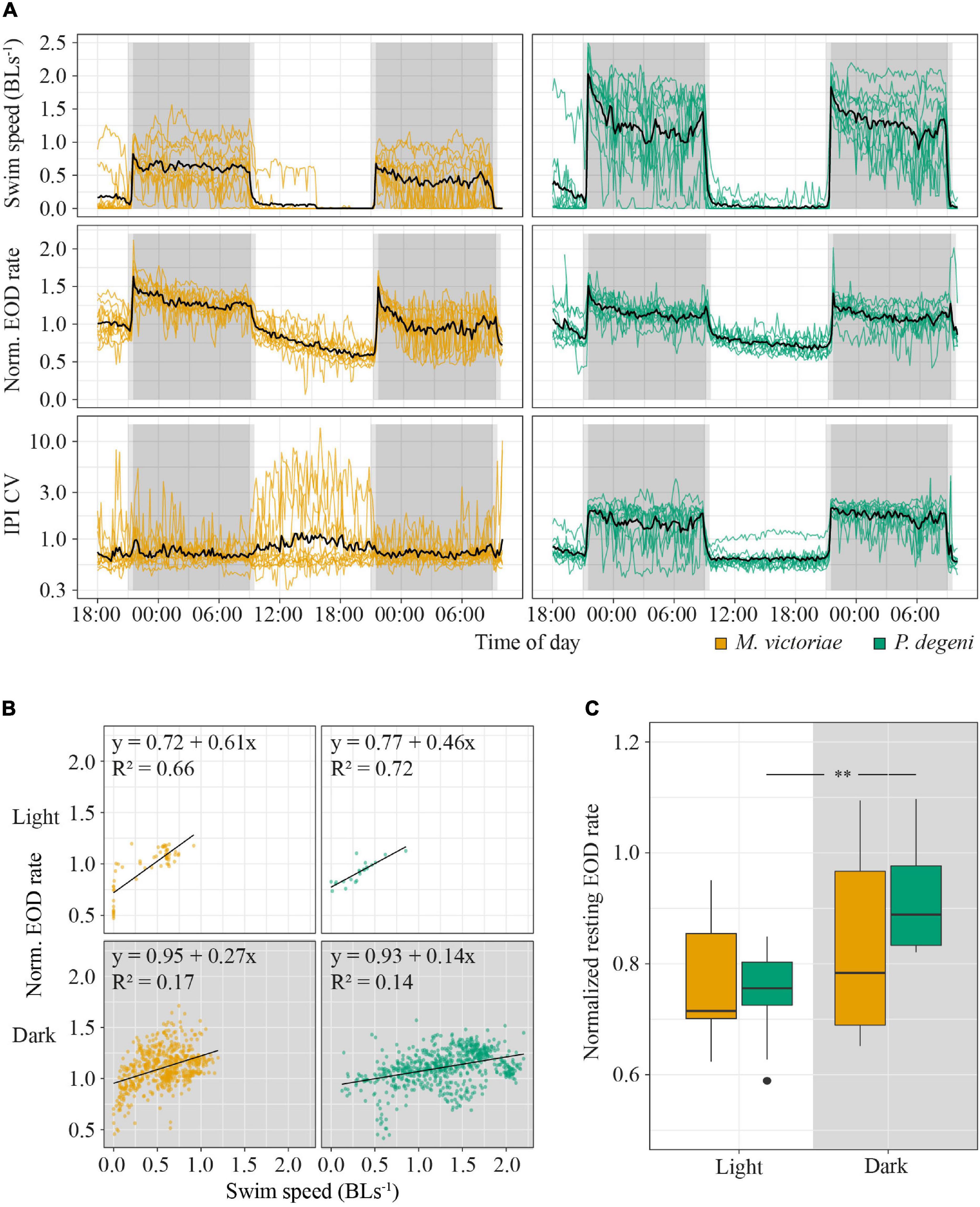
Figure 4. Behavioral activity of M. victoriae (n = 11) and P. degeni (n = 10) under laboratory conditions. (A) Swim speed in body lengths per second (BL s– 1, top row), normalized EOD rate (middle row), and coefficient of variation of inter-pulse intervals (IPI CV, bottom row) of M. victoriae (left) and P. degeni (right) in the shuttle-box. All variables were averaged per fish over 10-min intervals. Colored lines represent individual fish, black lines represent the average over all fish, gray shaded areas represent periods of dusk/dawn (light gray) and complete darkness (dark gray). Notice that IPI CV is shown on a logarithmic y-axis. (B) Linear regressions of swim speed and normalized EOD rate. Each point represents a measurement from an actively swimming fish that was averaged over a 10-min interval. Points from different fish are not distinguished. (C) Box plot of normalized EOD rate of fish that rested in their shelter tubes during the day and night. Resting EOD rate was averaged per fish (t-test: **p < 0.01).
There were some differences between species in swim and EOD activity. Swim activity of P. degeni was significantly higher than that of M. victoriae during the dark phase (1.30 ± 0.40 vs. 0.44 ± 0.28 BLs–1, Wilcoxon test: p < 0.001), and P. degeni increased swim speed and EOD rate at the end of the dark phase, an effect that was not as prominent in M. victoriae. IPI coefficient of variation (IPI CV) of P. degeni showed a clear diel pattern indicating that EOD rate was more variable during their active phase. By contrast, IPI CV of M. victoriae did not differ between light and dark phase, and some individuals showed greatly irregular IPIs during the inactive phase, mostly due to longer cessations of EOD generation. The resting EOD rate of P. degeni was significantly higher at night than during the day (t-test: t = –3.57, df = 9, p < 0.01; Figure 4C). This effect was not observed in M. victoriae.
Environmental and Behavioral Patterns at Petro Lagoon
Temperature and Dissolved Oxygen
Temperature and DO in the open habitat of the Petro Lagoon fluctuated over the diel cycle (Figure 5A). DO reached minimum values at night (5.1 ± 5.4% air saturation at 11.5 h after sunset) and increased in the morning. Rainfall and/or disturbance of the water surface is visible as peaks in DO values and contributed to the increase of mean DO in the morning. Water temperature followed a more pronounced oscillation than DO, reaching its maximum in the late afternoon (24.9 ± 1.1°C at 3.5 h before sunset) and its minimum in the early morning (21.6 ± 0.7°C at 0.5 h after sunset). Day length remained constant throughout the sampling period (12:06 – 12:07 h, Sunrise: 06:47 – 06:47 am, Sunset: 06:50 – 07:03 pm8).
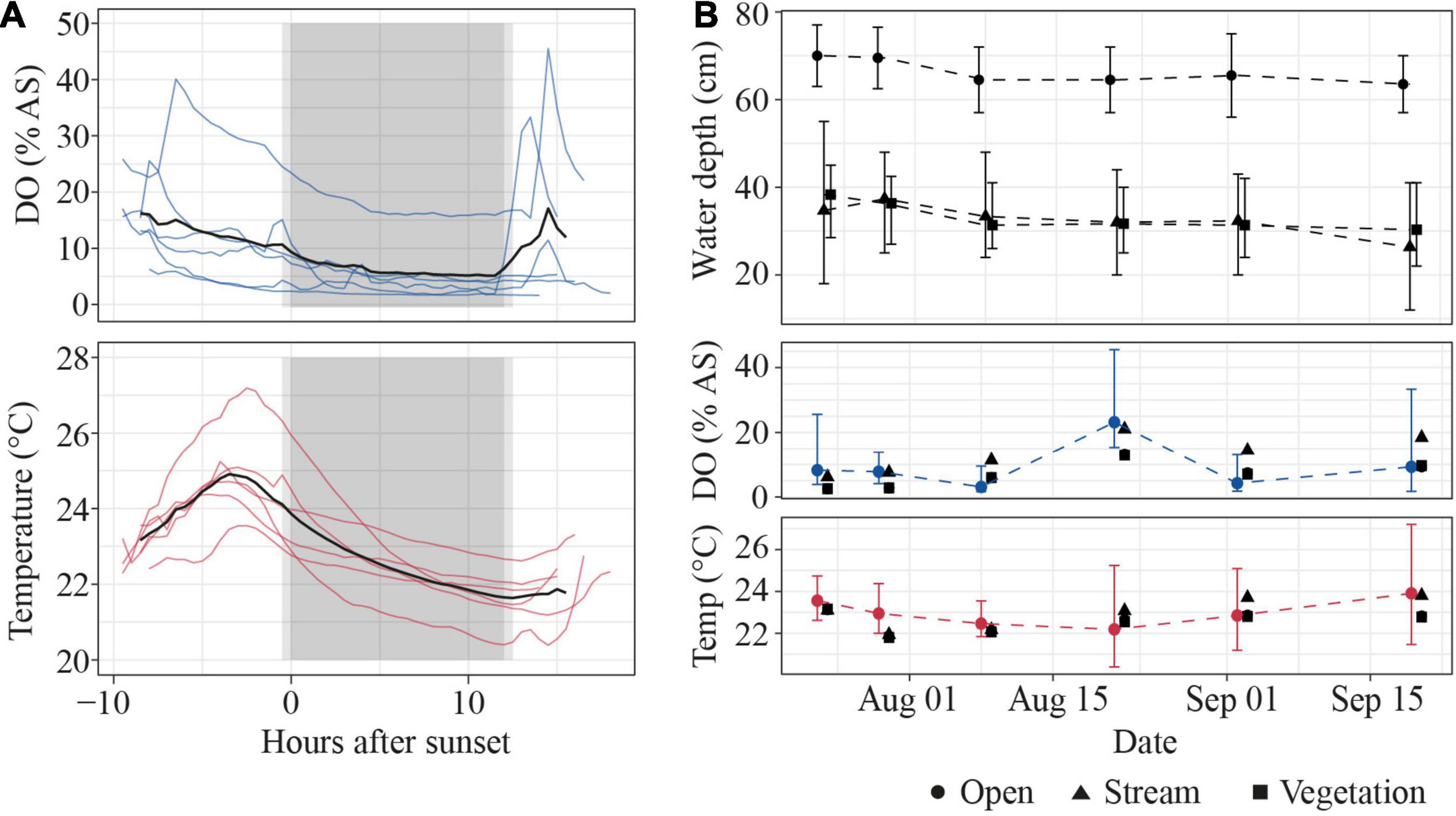
Figure 5. Environmental conditions at Petro Lagoon. (A) Daily changes in DO (in% air saturation, top) and water temperature (bottom). Thin lines indicate individual sampling days, the thick black line shows the mean over all sampling days (n = 6). Light gray boxes indicate 30-min dusk/dawn periods, dark gray boxes indicate night periods. (B) Variation of water level (top), DO (% air saturation, middle), and temperature (Temp., bottom) across sampling days. Symbols represent mean values, error bars represent the range of values. Manual measurements that were taken during the daytime are shown as black symbols in the DO and temperature panel. The shape of the symbols represents the different habitats.
Across sampling days, DO was highest on August 21st, due to continuous rainfall on that day, but remained low on average (9.2 ± 7.3% air saturation) and was extremely low on August 8th (2.7 ± 1.4%, Figure 5B). Daily water temperature remained at a relatively constant average value (23.0 ± 0.6°C) despite diel fluctuations. Water depth also remained relatively constant in all habitats and was highest in the open habitat. Manual measurements that were taken during the day showed little difference in temperature and DO between the open and vegetation habitat. However, DO and temperature tended to be slightly but systematically higher in the stream habitat (by 6.4 ± 2.4% air saturation and 0.4 ± 0.7°C, Figure 5B) than other areas of the lagoon.
Presence of Electric Fish in the Different Habitats
We collected more than 1,122 h of electric recordings in the open, stream and vegetation habitat at Petro Lagoon between July 23rd and September 20th 2019. We registered 2849 encounters with at least one M. victoriae and/or P. degeni, most of which happened during the night (2218). Both species were encountered equally often (M. victoriae: 1433, P. degeni: 1416). By far the highest number of encounters was recorded in the vegetation habitat (1137 and 852, respectively), followed by the open habitat (581 and 78, respectively), and the stream (0 and 201, respectively).
The frequency with which encounters occurred in the different habitats varied between species and periods of the diel cycle (Table 2 and Figure 6A). In the open habitat, both M. victoriae and P. degeni were encountered only at night, and M. victoriae accounted for the majority of the nocturnal encounters. Under the floating vegetation, both species were encountered throughout the diel cycle. However, the frequency of encounters with M. victoriae increased more than fivefold from daytime to nighttime, whereas P. degeni were encountered more continuously during the day and the night under the floating vegetation (Table 2). In the stream habitat, only P. degeni were encountered, and primarily at night.
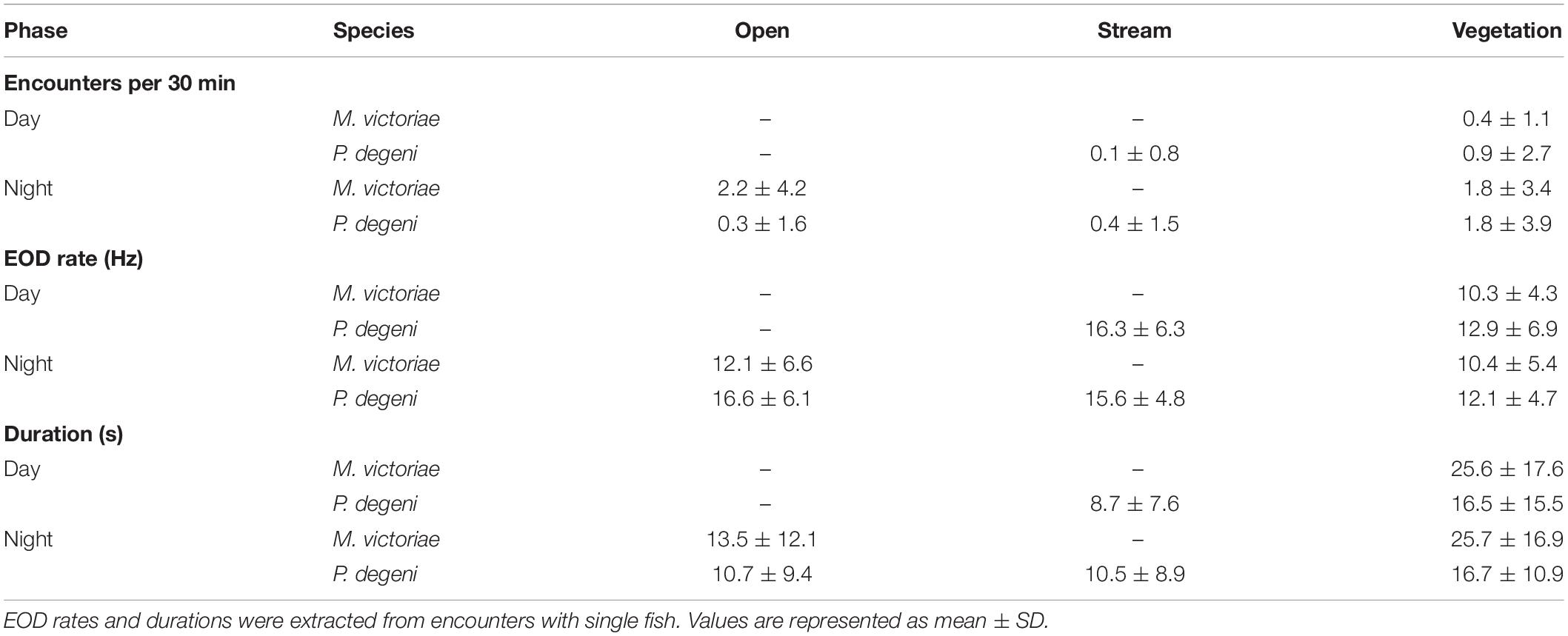
Table 2. Average number of encounters, EOD rates, and durations, grouped by species, habitat and phase of the diel cycle.
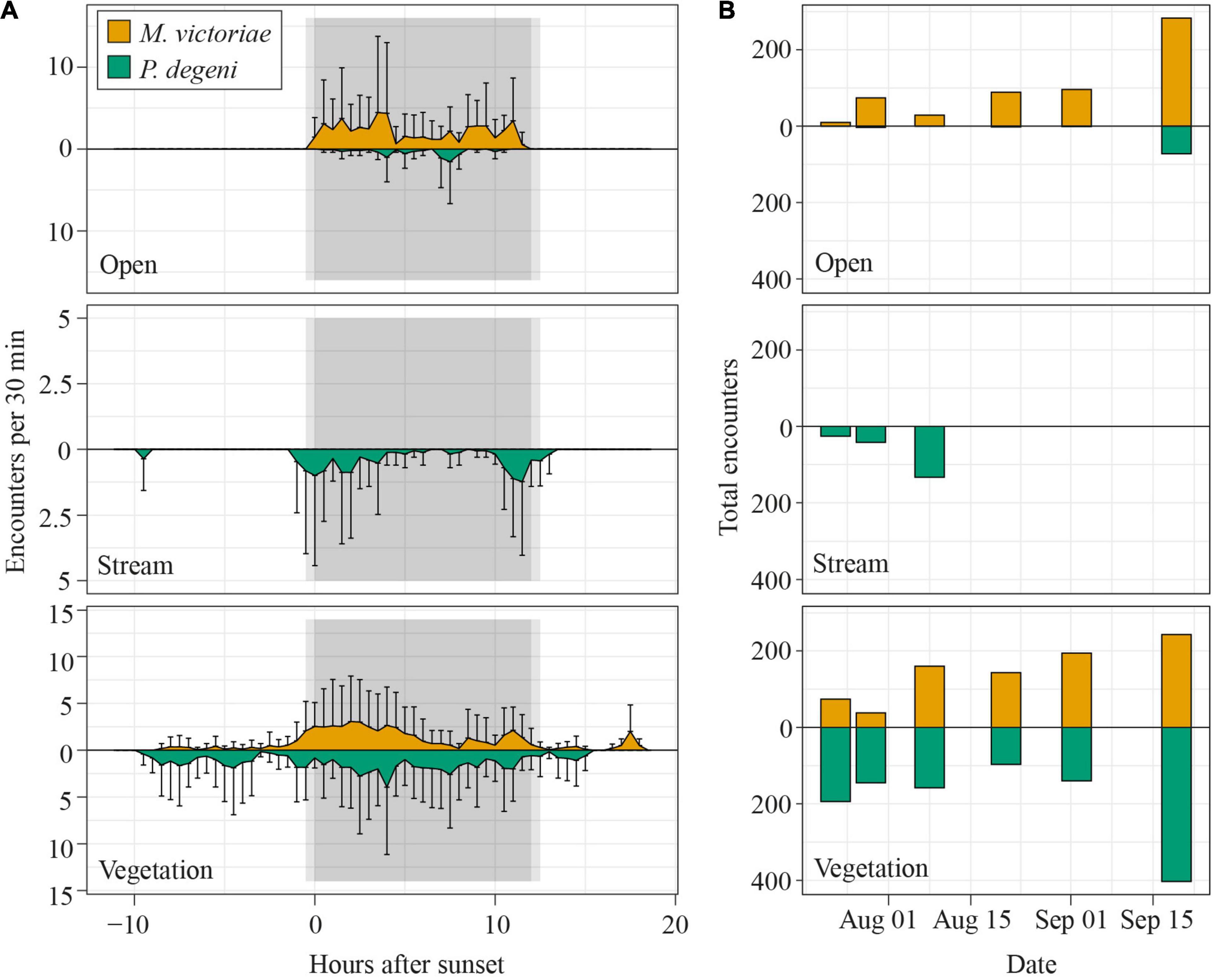
Figure 6. Diel patterns of EOD activity in Petro Lagoon. (A) Average number of fish encounters per 30 min in the open (top), stream (middle) and vegetation (bottom) habitat. To calculate the average number of fish encounters per 30 min, the number of fish was extracted from each encounter, summed up for each logger over intervals of 30 min and averaged over the number of loggers per habitat (open: 2, stream: 3, vegetation: 3) and all sampling days (n = 6). Error bars representing SD extend only into one direction for better readability. Notice that the y-axis scaling differs between the different panels. (B) Total number of fish that were encountered per sample day in the open (top), stream (middle), and vegetation (bottom) habitat. Data for M. victoriae are shown above the x-axis, those for P. degeni below.
The number of daily fish encounters varied considerably across sampling days (Figure 6B). Encounters with M. victoriae increased in the open habitat and vegetation habitat from 84 to 526 encounters. Numbers of encounters with P. degeni remained relatively constant under the floating vegetation except for a peak of 403 encounters on the last sampling day. In the stream habitat, P. degeni was encountered only during the first three sampling days, and in the open habitat only on the last sampling day. Overall, 35% of all fish encounters were made on the last sampling day.
Co-occurrence of Fish
In 18.7% of encounters, more than one fish was detected (co-occurrence; Figure 7A). On average, the proportion of encounters during which multiple M. victoriae were detected simultaneously was higher at night (17.4 ± 10.9% of encounters) than during the day (2.0 ± 2.8%; U-test: U = 2, p < 0.05). Petrocephalus degeni, likewise, co-occurred more often at night (10.8 ± 8.4%) than during the day (2.4 ± 4.1%; U-test: U = 3, p < 0.05). There was no difference between the two species in the percentage of encounters that included more than one conspecific. In 50.9% of co-occurrences, fish from both species were present.
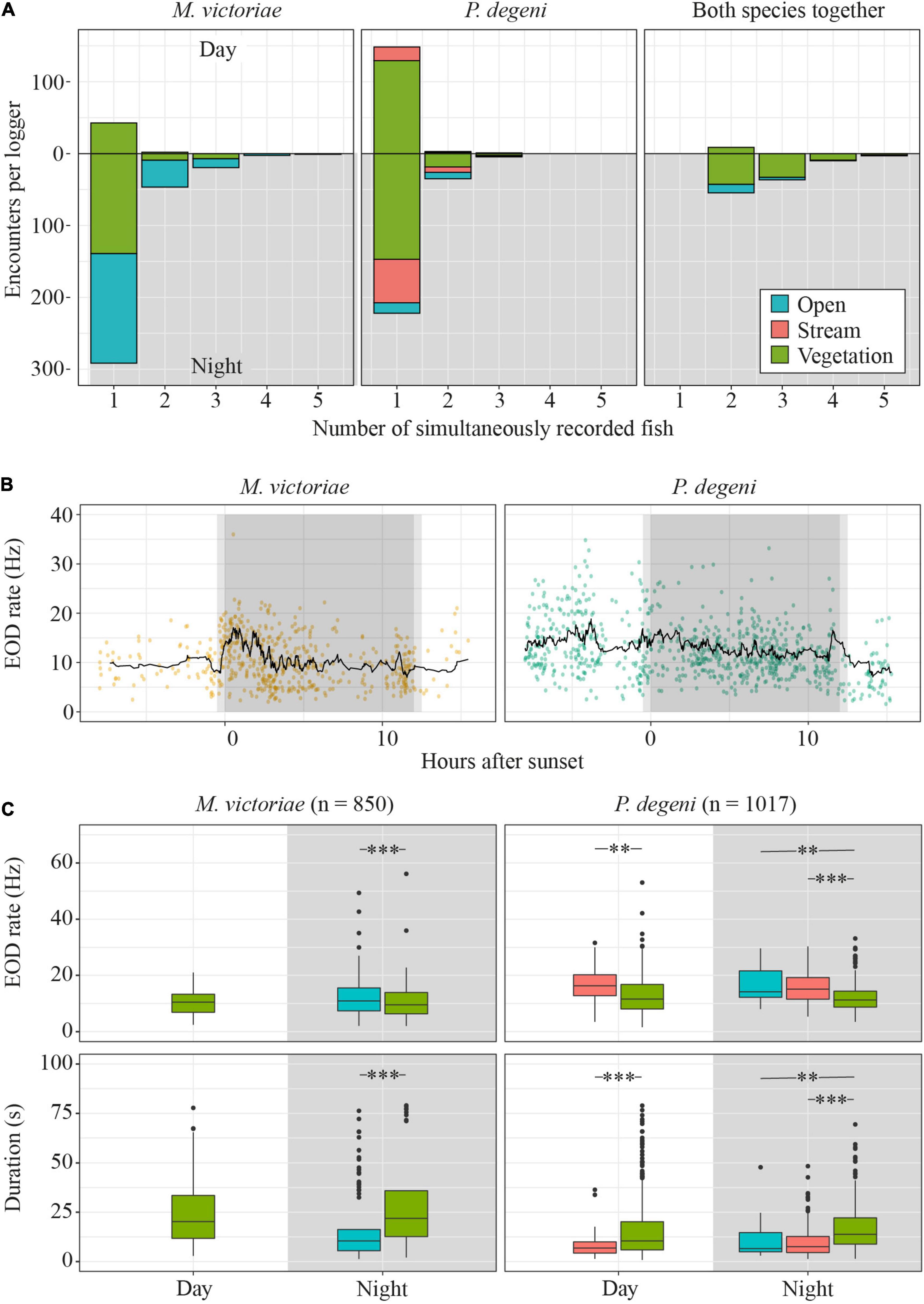
Figure 7. Diel differences in encounter characteristics. (A) Total number of encounters by species, habitat, daytime and number of fish that were present during encounter. (B) EOD rates of encounters with single M. victoriae (left) and P. degeni (right) in the vegetation habitat. Each encounter is represented by a dot, the black line represents the rolling mean over 20 encounters. (C) Boxplots of EOD rate (top row) and duration (bottom row) of encounters by phase of the diel cycle, species and habitat. Extreme outliers (more than 3 × interquartile range above the 3rd quantile or below the 1st quantile) were removed from the duration plot to improve readability. Outlier removal did not affect the outcome of statistical testing (t-test: **p < 0.01; ***p < 0.001).
Encounter Duration and Electric Organ Discharges Rate
Encounter duration and EOD rate differed among habitats and periods of the diel cycle, suggesting that fish displayed higher activity in the open and stream habitat and around sunset and sunrise (Table 2 and Figures 7B,C).
In the vegetation habitat, where most encounters were detected throughout the diel cycle, EOD rates of M. victoriae peaked within one hour of sunset and again shortly before sunrise. EOD rates of P. degeni were highest during the day but showed a small peak before sunrise (Figure 7B). These changes were transient and did not lead to elevated nocturnal EOD rates, as were observed in the laboratory. Both species emitted EODs at a lower rate under the vegetation than in the other habitats, both during the day and at night, and encounters lasted longer under the vegetation than in the other habitats (Figure 7C). Overall, the EOD rates of M. victoriae in the field were comparable to those from the laboratory (day: 10.3 ± 4.3 Hz vs. 8.0 ± 2.3 Hz; night: 11.1 ± 6.0 Hz vs. 11.1 ± 3.3 Hz). EOD rates of P. degeni were significantly higher in the field than in the laboratory during the day (13.0 ± 6.8 Hz vs. 7.7 ± 2.8 Hz, U-test: U = 3069, p < 0.01), but not during the night (13.6 ± 6.8 Hz vs. 11.3 ± 4.8 Hz).
Discussion
In this study, we tracked the activity of two species of mormyrid weakly electric fishes in the laboratory and recorded their diel activity patterns in a chronically hypoxic habitat using newly developed recording devices. In both settings, M. victoriae and P. degeni showed distinct, daylight-dependent behavioral patterns that were characterized by increased activity and exploration behavior under dark conditions. Diel patterns of co-occurrence suggest that the study species interact socially primarily during the night in their habitat. Although the behavior observed in the laboratory and in the wild was largely congruent, P. degeni showed higher daytime activity in naturally dark habitats than in the laboratory, and the increase of activity during the dark phase was much more pronounced and consistent in the laboratory than in the wild for both species. These findings suggest that the activity of the study species depends more on the environmental light conditions than on the phase of the diel cycle. It is likely that sinking nocturnal DO and temperature further affect activity patterns that are more varied in the wild than what is observed in the laboratory.
Spatial and Temporal Patterns of Behavior
Habitat Use and Swim Behavior
In the laboratory, fish spent nearly 100% of the time in their shelters during the light phase and increased their behavioral activity with the onset of the dark phase, which resulted in higher swim speed and a change in their movement range from sheltering to active exploration of their environment. In the wild, fish were almost exclusively detected under floating vegetation during the day and dispersed into the open areas of the lagoon at night. Interestingly, P. degeni showed a stronger preference for open areas in the shuttle-box during the dark phase than M. victoriae, whereas in the wild, M. victoriae were encountered more often in the open area at night, and P. degeni seemed to prefer the vegetation habitat both during day and night. As the sheltered areas in the shuttle-box were limited to two tubes, it is possible that this was an effect of the overall higher swim activity of P. degeni compared to M. victoriae. The different activity levels of the two species could also help to explain the fish dispersion patterns at Petro Lagoon. Given that DO was overall low but highest in the stream habitat, it could have been expected that fish move into the stream at night to reduce hypoxic stress. Thus, it came as a surprise to find that only P. degeni were encountered here, and only on the first three sampling days. It is possible that their higher activity level makes P. degeni more vulnerable to hypoxia and/or better adapted to an environment with flowing water. The more sluggish M. victoriae, on the other hand, might be better adapted to stagnant environments. Another possible explanation for the lack of fish in the stream is that fish had to cross a shallow section of the lagoon in order to reach it. Due to its low water depth, this section may have represented a movement barrier for the larger M. victoriae in the beginning of the sampling period. Over the sampling duration, water depth decreased slightly throughout the lagoon, which could have kept P. degeni from venturing into the stream on the later sampling days.
The temporal patterns of fish encounters suggest that fish actively moved into the open and stream areas of the lagoon at night and returned to the floating vegetation around sunrise. The transient increase of EOD rates under the vegetation during sunset and sunrise could be a result of this movement activity. This seems to align well with the activity peaks that were observed in the laboratory at the onset and toward the end of the dark phase. The question is, for what purpose do fish emerge at night and venture into unprotected areas? We suggest that fish use the open habitats to forage, interact, and carry out ASR at night at a lower risk of predation (e.g., Hobson, 1973; Nagelkerken et al., 2000; Hammerschlag et al., 2010; Sikkel et al., 2017). Although the low DO in the swamp protects fish from large predators, such as the Nile perch (Lates niloticus, Chapman and Chapman, 1998; Chapman et al., 2002), there is some risk of predation during the daytime due to the presence of visually oriented piscivorous birds, such as the hamerkop (Scopus umbretta) and the pied kingfisher (Ceryle rudis, Figure 8). These predators hunt predominantly in the open and shallow regions of the lagoon (pers. observation), turning them into dangerous territories for fish during the day. Further, the complete absence of mormyrids in the open and stream habitat during the day indicates that there is little diurnal foraging activity in these areas. At night, fish can exploit the untapped resources at a lower risk of predation. The non-visual sensory modality of M. victoriae and P. degeni facilitates prey capture in the dark (Emde and Bleckmann, 1998; Emde, 1999), and gives them an edge over other visually oriented nocturnal foraging species. Encounter duration and EOD rates suggest that the activity level of fish was higher in the open and stream area than in the vegetation, which aligns well with foraging behavior as increased EOD rates increase the temporal acuity of electrosensory information, and can aide in the localization of prey and/or avoidance of predators. Similar nocturnal exploration and foraging activity has been described before in weakly electric fishes (Lissmann and Schwassmann, 1965; Kruger, 1973; Moller et al., 1979; Friedman and Hopkins, 1996; Henninger et al., 2018; Madhav et al., 2018; Henninger et al., 2020). For example, Henninger et al. (2020) observed in a creek in Panama that three gymnotiform species, Apteronotus rostratus, Eigenmannia humboldtii and Sternopygus dariensis, move actively only during the night, traveling upstream after sunset and downstream in the second half of the night. In an early study in Brazil, Lissmann and Schwassmann (1965) demonstrated that sandfish (Gymnorhamphichthys hypostomus) rest, buried in the sand, during the day and emerge at night to forage and interact. In South Africa, Kruger (1973) found that Marcusenius macrolepidotus (formerly Gnathonemus macrolepidotus), a close relative to M. victoriae, moves at night from bottom waters into the littoral zone, presumably to feed on chironomids. Moller et al. (1979) recorded mormyrids in the Swashi River in Nigeria, which hid during the day in a river inlet and moved actively in and out of the river at night. The behavior of M. victoriae and P. degeni described here matches these observations well.
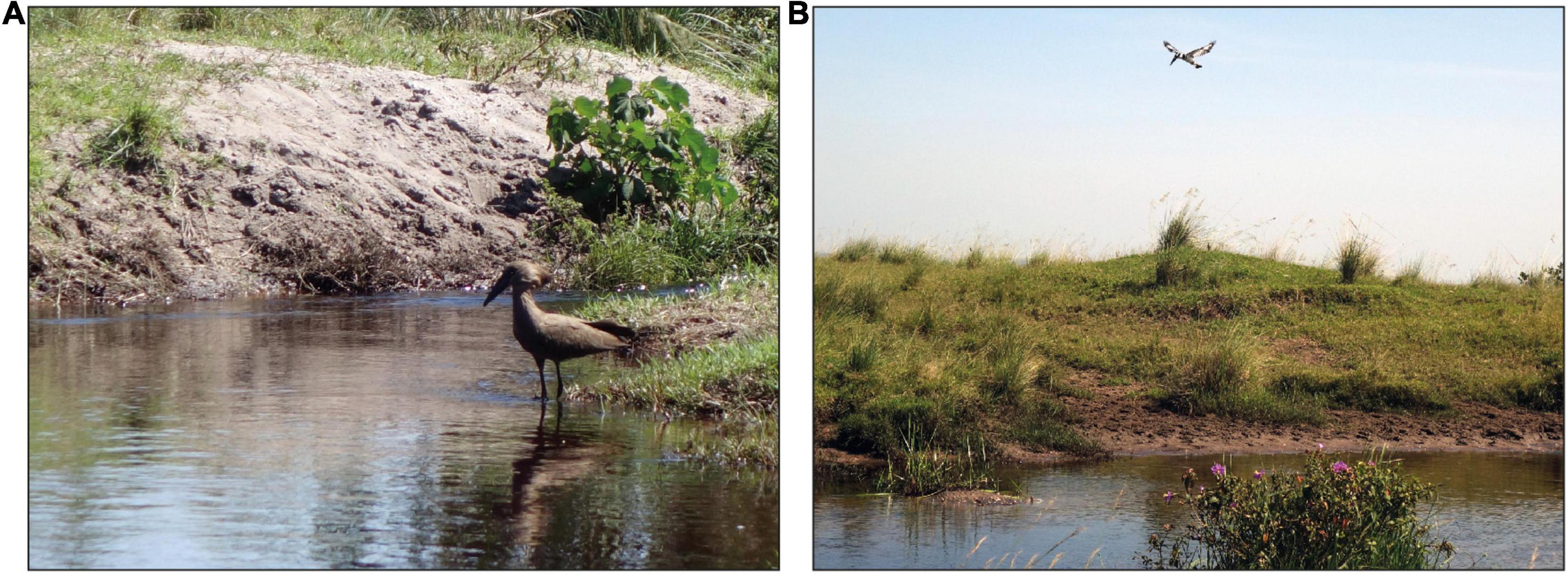
Figure 8. Piscivorous birds that forage at Petro Lagoon during the day. (A) A hamerkop (Scopus umbretta) lurks for prey at a shallow area of the lagoon. (B) A pied kingfisher (Ceryle rudis) hovers over the open area of the lagoon. Photo credit: Stefan Mucha.
We saw an increase in total fish encounters toward the end of the sampling period, especially on the last sampling day. Our last measurements were made just at the onset of the short rainy season, and there was a slight upwards trend in DO and temperature on the three last measurement days. The increase of encounters might be a result of higher behavioral activity due to higher energy availability and temperature. Another intriguing possibility is that the increase of fish encounters was caused by seasonal breeding migrations. Some mormyrid species breed at the onset of the rainy season (Hopkins and Bass, 1981; Kirschbaum, 1995). Decreasing water conductivity, increasing water levels, and artificial rainfall on the surface all promote gonad growth in those species (e.g., Kirschbaum and Schugardt, 2002). Although not much is known about the natural breeding behavior of our study species, it is possible that the increase of encounters with M. victoriae and the high number of encounters with P. degeni on the last sampling day indicate breeding behavior. This would be an exciting finding, as it would mean that these fish reproduce under severely hypoxic conditions. A longer-term survey of the fish distribution in the Lake Nabugabo system during the rainy seasons, e.g., with electric fish loggers, should give better insights into this important aspect of the ecology of P. degeni and M. victoriae.
Electric Activity
In the laboratory, EOD rate was closely correlated to swim activity in both species and showed clear diel rhythms. The two species differed in their temporal patterns of EOD generation. Petrocephalus degeni emitted EODs at more regular intervals during their inactive phase and became more irregular during their active phase. This was not the case in M. victoriae. In contrast, some M. victoriae showed greatly irregular IPIs with pauses that exceeded 10 s and could reach up to > 120 s during their inactive phase. Similar differences in EOD generation have been described by Carlson (2016) who found that M. victoriae respond to EOD playback with pauses of electric signaling whereas P. degeni tend to generate EOD bursts. These differences in pulsing behavior likely reflect a variety of differences in electrosensory anatomy and social behavior between these two species (Carlson, 2016). For example, cessations of EOD generation have been associated with aggressive and submissive behavior (Carlson, 2002), traits that might be more typical of M. victoriae (Carlson, 2016). Pausing EOD generation during their inactive phase could reduce their detectability by electrosensory predators and/or aggressive conspecifics.
In the field, EOD rates of M. victoriae increased after sunset, however, this effect was much more transient than in the laboratory. The EOD rates of P. degeni were highest during the day and showed only a transient increase toward the end of the night. Overall, the clear pattern that was observed in the laboratory could not be reproduced in the field. We identified a variety of factors that may have influenced EOD activity patterns. First, the lower water temperatures likely reduced EOD rates during the night (e.g., Toerring and Serrier, 1978). Second, nocturnal hypoxia could have led to reduced EOD rates (Clarke et al., 2020; Moulton et al., 2020), if it was not compensated for, e.g., by aquatic surface respiration (ASR), during which fish ventilate the well-oxygenated surface water layer (Lewis, 1970; Chapman and Chapman, 1998). Third, P. degeni maintained a higher level of activity during the day under the floating vegetation than in the tubes of the shuttle-box. This effect could not be discerned in M. victoriae. It appears that the availability of an extensive sheltered habitat stimulated diurnal activity (e.g., exploration and foraging) of P. degeni. A possible factor for the species difference in diurnal activity could be their different social behavior, with the group-living P. degeni exhibiting higher diurnal activity than the solitary M. victoriae, although this remains speculative.
Social Interaction
Unlike many other fishes, which rely primarily on visual cues for social interaction (e.g., reviewed by Rowland, 1999), weakly electric fishes can use their active electrosense to communicate and interact with conspecifics, and thus are well equipped to do so at night (Moller et al., 1989; Scheffel and Kramer, 1997; Carlson and Hopkins, 2004). The sampling design we used did not allow detailed observation of social interactions, and an in-depth analysis of EOD recordings from co-occurrences was beyond the scope of this study, which focused on the temporal and spatial dynamics of fish behavior. However, some conclusions can be drawn from the statistics of recorded co-occurrences of fish. Here, it is important to consider the different detectability of the two species. While co-occurring P. degeni had to be within approximately 1 m of each other to be detected simultaneously in a logger recording, M. victoriae could be more than 3 m apart from each other and still be detected together. Thus, based on co-occurrences, the degree of social interaction is likely somewhat overestimated in M. victoriae (co-occurrence without interaction) and underestimated in P. degeni (detection of one fish out of a group). Notwithstanding these limitations, the fact that co-occurrences increased by 850% (M. victoriae) and 450% (P. degeni) strongly suggests that fish encountered and interacted with conspecifics more frequently at night. Nocturnal bouts of social interaction have also been described in the phylogenetically distant gymnotiforms and could be a common characteristic of weakly electric fishes (Silva et al., 2007; Stoddard et al., 2007). Marcusenius victoriae has been described as a territorial species, which is solitary and displays high intraspecific competition for shelters (Carlson, 2016). Our recordings suggest that territoriality and competition for shelters is most prominent during the daytime, when M. victoriae were recorded exclusively in the vegetation, and were alone in ca. 98% of those encounters. During the night, M. victoriae seem to show a higher degree of social affiliation and/or acceptance of conspecifics in their proximity. Interestingly, most co-occurrences of M. victoriae were recorded in the open habitat, which may suggest that they favor interacting in a more “neutral zone” than in the habitat where fish occupy their shelters during the day. Petrocephalus degeni, on the other hand, has been described as a gregarious species (Carlson, 2016), and can be kept in group tanks more easily than M. victoriae (pers. observation). There was no difference between the two species in the proportion of conspecific co-occurrences, however, given the considerable difference in detectability, this finding supports the notion that P. degeni occurs naturally in social groups.
During most encounters with multiple fish, both species were present. In some cases, the low-amplitude EODs of a P. degeni were only revealed due to the presence of another fish whose EOD amplitude crossed the detection threshold, which was frequently M. victoriae. Nevertheless, this implies that both species occur in spatial proximity and likely are connected through interactions and/or competition for resources. More detailed behavioral observations, e.g., using a grid of electrodes (Henninger et al., 2018, 2020; Madhav et al., 2018), could give interesting insights into the nocturnal social behavior of these sympatric species.
Environmental and Endogenous Rhythms
Influence of Light
Behavioral rhythms can be governed by environmental cues and endogenous biological clocks, and the question, which one of these two components drives an observed behavioral rhythm cannot be answered without thorough study of the endogenous circadian rhythms of an organism (Aschoff, 1960). In the laboratory, where temperature and DO were kept constant, behavioral activity of M. victoriae and P. degeni corresponded closely to ambient light levels: locomotor activity and EOD rate peaked precisely at the onset of darkness. Although activity level was not directly quantified in the field, the patterns of fish encounters corroborate this finding. In the open area where day-night changes in illumination are most pronounced, the number of fish encounters followed a clear diel pattern. In the vegetation habitat where light conditions remain mostly dark throughout the diel cycle, this pattern was less obvious, and P. degeni showed the same level of activity during the day and the night. Both observations suggest that light intensity is an important factor for behavioral activity, however, they are insufficient to disentangle the effects of endogenous clocks that are entrained by light and the direct effect of light as environmental cue on the behavior of M. victoriae and P. degeni.
There was no evidence that fish anticipated the dark phase, which could have served to suggest the existence of a circadian clock (Reebs, 1994). Two findings, however, could indicate the effect of an endogenous rhythm on behavioral activity in P. degeni. First, they showed an increase in swim speed and EOD rate toward the end of the dark phase, both in the laboratory and in the field. A similar phenomenon has been documented in G. petersii (Bässler et al., 1979). Second, their EOD rate at rest was significantly higher during the night than during the day. A similar increase in nocturnal EOD baseline rate has been found in the pulse-type gymnotiform Gymnotus omarorum, which lives under naturally constant darkness (Migliaro et al., 2018). This phenomenon has been hypothesized to be under endogenous control, although this has yet to be confirmed under laboratory conditions. The presence of a circadian rhythm could be tested by subjecting fish to constant light and/or dark conditions for several days while assessing whether free-running behavioral rhythms can be detected (Aschoff, 1960). In a study with the pulse-type gymnotiform Brachyhypopomus pinnicaudatus, Stoddard et al. (2007) found circadian rhythms in electric signal parameters that are likely caused by melanocortin peptides, which act directly on electrocytes, and through serotonergic projections to neuronal pacemaker structures. However, in a recent field study, Henninger et al. (2020) also found no evidence for anticipation of the active phase in wild gymnotiforms.
Temperature and Dissolved Oxygen
Interestingly, the nocturnal increase of activity and movement range co-occurred with extreme nocturnal hypoxia at Petro Lagoon. In line with previous observations and surveys, which have consistently found both species in this site since the 1990s under hypoxic conditions (Chapman et al., 1996b,2002; Chapman and Chapman, 1998; Carlson, 2016; Clarke et al., 2020; Moulton et al., 2020), the continuous in situ measurements of EODs, DO and temperature show for the first time that these fish remain present, and likely are most active, throughout the most extreme nocturnal hypoxia periods. This is an intriguing finding as, although both species investigated here have been found to be hypoxia-tolerant (Chapman and Chapman, 1998; Clarke et al., 2020; Moulton et al., 2020), the degree of nocturnal hypoxia found here far exceeded their respective physiological thresholds of oxyregulation (Pcrit). Pcrit is defined as the oxygen partial pressure at which an organism can no longer maintain a stable rate of oxygen consumption (oxyregulation) and begins to conform to environmental DO (oxyconformation; Beamish, 1964; Ultsch et al., 1978). In the study site, air saturation dropped as low as 2.7 ± 1.4% (on August 8, 2019), which is well below any Pcrit measured in swamp-dwelling P. degeni (Chapman and Chapman, 1998: ca. 5.8%, Clarke et al., 2020: ca. 7.0%) and M. victoriae (Moulton et al., 2020: ca. 9.5%). In fact, mean PO2 at this site remained below the lowest published Pcrit for more than 50% of the diel cycle on three of the six sampling days. Ackerly et al. (2018) showed that a DO concentration of ca. 17.5% already negatively affected the critical swim speed and EOD rate of M. victoriae. Temperature decreased during the night at Petro Lagoon, which could reduce the rate of metabolic processes and somewhat buffer hypoxic stress. However, it seems unlikely that this effect would suffice to compensate for extremely low nocturnal DO, which suggests that environmental hypoxia significantly limited the performance of both species, particularly during their most active phase. This raises the question as to how these fish manage to maintain and even ramp up their levels of electrical and locomotor activity during extreme hypoxia. One possible explanation is that fish compensate for the nighttime hypoxia by using ASR (Chapman and Chapman, 1998). Since ASR requires that fish have free access to the water surface, fish have to emerge from the swimming vegetation to perform ASR. This might drive the nocturnal migration of both species into the open habitats. At night, ASR could be particularly well suited to increase oxygen supply as the absence of diurnal predators reduces the otherwise high risk of predation (Kramer et al., 1983). A similar behavior has been identified in Amazonian fishes that emerge from their macrophyte cover to perform ASR during periods of severe nocturnal hypoxia (Saint-Paul and Soares, 1987).
The diel fluctuation of DO and temperature could entrain endogenous circadian rhythms, and therefore affect behavioral activity on another level. The circadian clock has been shown to be closely related to the hypoxia-signaling pathway in mammals (e.g., Adamovich et al., 2017) and zebrafish (Danio rerio, Egg et al., 2013; Pelster and Egg, 2018; Sandbichler et al., 2018). Hypoxia-inducible factor 1α (HIF-1α) is a key regulator of the physiological hypoxia response. It dimerizes with HIF-1β under hypoxic conditions and regulates the transcription of genes by binding to hypoxia-responsive elements (Wenger et al., 2005; Schödel et al., 2011). The expression of HIF-1α is tightly controlled by the circadian clock and follows a circadian rhythm (Egg et al., 2013). On the other hand, hypoxic conditions lead to decreased expression levels of clock genes such as period1 (Egg et al., 2013; Sandbichler et al., 2018). Adamovich et al. (2017) suggest that, mediated through HIF-1α, hypoxia is a resetting cue for circadian clocks. In fish that have adapted to chronic environmental hypoxia, such as M. victoriae and P. degeni, an interesting question for further studies would be, whether adaptation to hypoxia has affected this interaction, or whether the expression of clock genes remains constantly dampened by hypoxia.
Many fish species seem to possess peripheral clocks in their tissues that are light sensitive without the need for specialized light sensing organs such as eyes or the pineal gland (Whitmore et al., 2000; Frøland Steindal and Whitmore, 2019). The finding of such peripheral clocks illustrates that the concept of a centralized “master-clock” that governs rhythmic behavior does not extend to fish, which rather possess a full-body light sensitivity (Frøland Steindal and Whitmore, 2019). As oxygen levels are not homogeneously distributed throughout body tissues, it is likely that these peripheral clocks are differentially affected by hypoxia. Although this has not been shown in electric fish yet, their well-understood neural circuits and non-invasively detectable EODs make electric fishes promising subjects for future studies of biological rhythms and the influence of light, DO and temperature.
Recording Natural Activity Patterns With Electric Fish Loggers
Mormyrids are often considered nocturnal animals, however, this view is at least partially based on laboratory studies (Harder et al., 1964; Moller, 1970; Bässler et al., 1979; Cobert, 1984). As we show here, natural activity patterns can deviate from laboratory activity patterns. A closer look into the literature demonstrates that mormyrids exhibit a variety of natural activity patterns that would not all classify as nocturnal. Using capture by gill net in Loskop Dam, South Africa, Kruger (1973) found that M. macrolepidotus migrate at night from bottom waters to the littoral zone. However, based on stomach content analysis, he also concluded that they feed predominantly during the day at the bottom of the reservoir. In the Ivindo River district in Gabon, Hopkins (1980) recorded EODs of 23 mormyrid species in the field and noted that mormyrids in shallow water remained sheltered during the day and made occasional foraging excursions at night whereas fish that lived in deeper waters appeared to move around during the day, as well. Our study shows that P. degeni remain active during the day under floating vegetation. In all of these studies, mormyrid activity seems most clearly determined by ambient light levels, and might not follow a diel pattern in continuously dark habitats. This differentiation may seem trivial, however, it has far-reaching biological implications. Nocturnality suggests a fixed and recurring behavioral rhythm that is likely to be under the control of endogenous oscillations whereas direct light-sensitivity facilitates more opportunistic behavioral patterns and might be independent of endogenous clocks. Understanding the mechanisms that underlie natural activity patterns of fishes is a crucial step to understanding the dynamics of aquatic ecosystems. Weakly electric fishes offer great opportunities in this regard. Their EODs give insight into the activity, habitat use and social interaction of elusive, tropical fishes, that are impossible to gain through visual observation methods. Previous studies have used electrode arrays (Henninger et al., 2018, 2020; Madhav et al., 2018), or single/handheld electrodes (Lissmann and Schwassmann, 1965; Moller et al., 1979; Hopkins, 1980; Friedman and Hopkins, 1996; Migliaro et al., 2018) to record EOD activity of weakly electric fishes in the wild. The methods that these studies employed necessitated the supervision of a researcher, which naturally limited the timespan over which observations were made. Here, we introduce a new and unsupervised method to continuously record the EODs of weakly electric fish. Our approach is based on the deployment of autonomously operating logging devices. In comparison with the previously used electrode arrays, observations from individual loggers lack the spatial resolution that is necessary to track animals and document detailed behavioral interactions. Nevertheless, there are certain benefits to this approach. Distribution of individual loggers allowed us to sample from different habitats and cover a larger area than would have been possible using an electrode array, and it enabled us to make observations over longer timespans than previously possible. The low price per unit (ca. 70 €) makes this technology accessible and reduces the financial risk of loss of equipment in the field. Open source microcontroller technology, such as the Teensy, and the Arduino programming language are used by a large community of scientists and non-scientists. These features make the EOD logger a promising and open tool for studies that focus on longer-term temporal patterns, or that are conducted in challenging environments that are difficult to access, such as swamps and lakes. For future applications, it is conceivable that the recording program can be adapted to allow the connection of multiple EOD loggers to record with higher spatial resolution from areas of interest.
Conclusion
This study shows nocturnal behavior of two mormyrid fishes that persist in a habitat with extreme nocturnal hypoxia. Activity increases corresponded directly to ambient light levels indicating that light is an important environmental cue and/or zeitgeber for endogenous clocks. Compared to laboratory observations, nocturnal activity increases were less pronounced in the field, which is possibly due to lower nocturnal water temperatures, hypoxia, and a higher environmental complexity that fosters a higher diurnal activity level. Habitat use shifted between a strong preference for complex habitats during the day to increased activity in open and unprotected areas during the night, when DO concentrations are lowest. Co-occurrences suggest that social interactions take place in both species mostly during the active phase at night, and in the case of M. victoriae mostly in the open areas of the habitat, although further investigation is needed for a detailed description of the nocturnal social behavior of the study species. Given their preference for structurally complex habitats, freshwater ecosystems with dense vegetation are likely to play an important role in the ecology of the study species.
Data Availability Statement
The original data presented in the study are publicly available at: doi: 10.6084/m9.figshare.19526155.v3.
Ethics Statement
The animal study was reviewed and approved by Landesamt für Gesundheit und Soziales, Berlin, Germany.
Author Contributions
All authors participated in the design of this study. SM developed the EOD logger, performed the field work and laboratory experiments with P. degeni, and data analyses. FO conducted the laboratory experiments with M. victoriae. SM drafted the manuscript and all authors took part in its revision.
Funding
Research funding was provided by Cluster of Excellence NeuroCure and by Humboldt-Universität zu Berlin to RK. We acknowledge support by the German Research Foundation (DFG) and the Open Access Publication Fund of Humboldt-Universität zu Berlin.
Conflict of Interest
The authors declare that the research was conducted in the absence of any commercial or financial relationships that could be construed as a potential conflict of interest.
Publisher’s Note
All claims expressed in this article are solely those of the authors and do not necessarily represent those of their affiliated organizations, or those of the publisher, the editors and the reviewers. Any product that may be evaluated in this article, or claim that may be made by its manufacturer, is not guaranteed or endorsed by the publisher.
Acknowledgments
We would like to thank D. Twinomugisha and P. Omeja for logistical support and assistance with field work. We also thank assistants J. Kiberu, F. Sseguya, G. Miiro and J. Mutebi, and members of both the Chapman and Krahe labs, particularly Leon Marquardt who contributed to the preliminary code of the EOD logger.
Supplementary Material
The Supplementary Material for this article can be found online at: https://www.frontiersin.org/articles/10.3389/fevo.2022.870043/full#supplementary-material
Footnotes
- ^ https://ffmpeg.org/ (accessed December 2021).
- ^ https://www.python.org/ (accessed December 2021).
- ^ https://github.com/bendalab/thunderfish (accessed December 2021).
- ^ https://www.arduino.cc/ (accessed December 2021).
- ^ https://www.pjrc.com/ (accessed December 2021).
- ^ https://github.com/muchaste/EOD-Logger (accessed December 2021).
- ^ https://www.r-project.org/ (accessed December 2021).
- ^ https://www.worldweatheronline.com/masaka-weather-history/masaka/ug.aspx (accessed January 2022).
References
Ackerly, K. L., Krahe, R., Sanford, C. P., and Chapman, L. J. (2018). Effects of hypoxia on swimming and sensing in a weakly electric fish. J. Exp. Biol. 2018:221. doi: 10.1242/jeb.172130
Adamovich, Y., Ladeuix, B., Golik, M., Koeners, M. P., and Asher, G. (2017). Rhythmic oxygen levels reset circadian clocks through HIF1α. Cell Metab. 25, 93–101. doi: 10.1016/j.cmet.2016.09.014
Adebisi, A. A. (1981). The physico-chemical hydrology of a tropical seasonal river - upper Ogun river. Hydrobiologia 79, 157–165. doi: 10.1007/bf00006123
Ardanaz, J. L., Silva, A., and Macadar, O. (2001). Temperature sensitivity of the electric organ discharge waveform in Gymnotus carapo. J. Comp. Physiol. A 187, 853–864. doi: 10.1007/s00359-001-0256-8
Arrington, D. A., and Winemiller, K. O. (2003). Diel changeover in sandbank fish assemblages in a neotropical floodplain river. J. Fish Biol. 63, 442–459. doi: 10.1046/j.1095-8649.2003.00167.x
Aschoff, J. (1960). Exogenous and endogenous components in circadian rhythms. Cold Spring Harb. Symp. Quant. Biol. 25, 11–28. doi: 10.1101/sqb.1960.025.01.004
Bässler, G., Hilbig, R., and Rahmann, H. (1979). Untersuchungen zur circadianen Rhythmik der elektrischen und motorischen Aktivität von Gnathonemus petersii (Mormyridae. Pisces). Z. Tierpsychol. 49, 156–163. doi: 10.1111/j.1439-0310.1979.tb00284.x
Baumann, H., Wallace, R. B., Tagliaferri, T., and Gobler, C. J. (2015). Large natural pH, CO2 and O2 fluctuations in a temperate tidal salt marsh on diel, seasonal, and interannual time scales. Estuaries Coasts 38, 220–231. doi: 10.1007/s12237-014-9800-y
Beamish, F. W. H. (1964). Respiration of fishes with special emphasis on standard oxygen consumption: II. Influence of weight and temperature on respiration of several species. Can. J. Zool. 42, 177–188. doi: 10.1139/z64-016
Benda, J. (2020). “The Physics of Electrosensory Worlds,” in The Senses: A Comprehensive Reference, ed. B. Fritzsch (Cambridge, MA: Academic Press), 228–254. doi: 10.1016/b978-0-12-805408-6.00016-6
Boujard, T., and Leatherland, J. F. (1992). Circadian rhythms and feeding time in fishes. Environ. Biol. Fish. 35, 109–131. doi: 10.1007/bf00002186
Campos-Candela, A., Palmer, M., Balle, S., and Alós, J. (2018). A camera-based method for estimating absolute density in animals displaying home range behaviour. J. Anim. Ecol. 87, 825–837. doi: 10.1111/1365-2656.12787
Carlson, B. A. (2002). Electric signaling behavior and the mechanisms of electric organ discharge production in mormyrid fish. J. Physiol. Paris 96, 405–419. doi: 10.1016/S0928-4257(03)00019-6
Carlson, B. A. (2016). Differences in electrosensory anatomy and social behavior in an area of sympatry between two species of mormyrid electric fishes. J. Exp. Biol. 219, 31–43. doi: 10.1242/jeb.127720
Carlson, B. A., and Hopkins, C. D. (2004). Stereotyped temporal patterns in electrical communication. Anim. Behav. 68, 867–878. doi: 10.1016/j.anbehav.2003.10.031
Chapman, L. J., and Chapman, C. A. (1998). Hypoxia tolerance of the mormyrid Petrocephalus catostoma. implications for persistence in swamp refugia. Copeia 1998:762. doi: 10.2307/1447812
Chapman, L. J., and Hulen, K. G. (2001). Implications of hypoxia for the brain size and gill morphometry of mormyrid fishes. J. Zool. 254, 461–472. doi: 10.1017/s0952836901000966
Chapman, L. J., Chapman, C. A., Ogutu-Ohwayo, R., Chandler, M., Kaufman, L., and Keiter, A. E. (1996b). Refugia for endangered fishes from an introduced predator in Lake Nabugabo, Uganda. Conserv. Biol. 10, 554–561. doi: 10.1046/j.1523-1739.1996.10020554.x
Chapman, L. J., Chapman, C. A., and Chandler, M. (1996a). Wetland ecotones as refugia for endangered fishes. Biol. Conserv. 78, 263–270. doi: 10.1016/s0006-3207(96)00030-4
Chapman, L. J., Chapman, C. A., Nordlie, F. G., and Rosenberger, A. E. (2002). Physiological refugia: swamps, hypoxia tolerance and maintenance of fish diversity in the Lake Victoria region. Comp. Biochem. Physiol. A Mol. Integr. Physiol. 133, 421–437. doi: 10.1016/s1095-6433(02)00195-2
Chapman, L. J., Chapman, C. A., Schofield, P. J., Olowo, J. P., Kaufman, L., Seehausen, O., et al. (2003). Fish faunal resurgence in Lake Nabugabo, East Africa. Conserv. Biol. 17, 500–511. doi: 10.1046/j.1523-1739.2003.01519.x
Chrétien, E., and Chapman, L. J. (2016). Habitat heterogeneity facilitates coexistence of native fishes with an introduced predator. the resilience of a fish community 5 decades after the introduction of Nile perch. Biol. Invasions 18, 3449–3464. doi: 10.1007/s10530-016-1235-x
Clarke, S. B., Chapman, L. J., and Krahe, R. (2020). The effect of normoxia exposure on hypoxia tolerance and sensory sampling in a swamp-dwelling mormyrid fish. Comp. Biochem. Physiol. A Mol. Integr. Physiol. 2020:240. doi: 10.1016/j.cbpa.2019.110586
Cobert, S. J. (1984). Environmental control of rhythmic behavior in the weak-electric fish, Gnathonemus petersii (Mormyridae). Ph.D. thesis, New York, NY.
Crampton, W. G. R. (1996). Gymnotiform fish. An important component of Amazonian floodplain fish communities. J. Fish Biol. 48, 298–301. doi: 10.1006/jfbi.1996.0029
Crampton, W. G. R. (1998). Effects of anoxia on the distribution, respiratory strategies and electric signal diversity of gymnotiform fishes. J. Fish Biol. 53, 307–330. doi: 10.1111/j.1095-8649.1998.tb01034.x
Crampton, W. G. R. (2019). Electroreception, electrogenesis and electric signal evolution. J. Fish Biol. 95, 92–134. doi: 10.1111/jfb.13922
Dunlap, K. D., Smith, G. T., and Yekta, A. (2000). Temperature dependence of electrocommunication signals and their underlying neural rhythms in the weakly electric fish. Apteronotus leptorhynchus. Brain Behav. Evol. 55, 152–162. doi: 10.1159/000006649
Egg, M., Köblitz, L., Hirayama, J., Schwerte, T., Folterbauer, C., Kurz, A., et al. (2013). Linking oxygen to time: the bidirectional interaction between the hypoxic signaling pathway and the circadian clock. Chronobiol. Int. 30, 510–529. doi: 10.3109/07420528.2012.754447
Emde, G. (1999). Active electrolocation of objects in weakly electric fish. J. Exp. Biol. 202, 1205–1215. doi: 10.1242/jeb.202.10.1205
Emde, G., and Bleckmann, H. (1998). Finding food: senses involved in foraging for insect larvae in the electric fish Gnathonemus petersii. J. Exp. Biol. 201(Pt 7), 969–980. doi: 10.1242/jeb.201.7.969
Follana-Berná, G., Palmer, M., Lekanda-Guarrotxena, A., Grau, A., and Arechavala-Lopez, P. (2020). Fish density estimation using unbaited cameras: accounting for environmental-dependent detectability. J. Exp. Mar. Biol. Ecol. 527:151376. doi: 10.1016/j.jembe.2020.151376
Franchina, C. R., and Stoddard, P. K. (1998). Plasticity of the electric organ discharge waveform of the electric fish Brachyhypopomus pinnicaudatus. I. Quantification of day-night changes. J. Comp. Physiol. A 183, 759–768. doi: 10.1007/s003590050299
Franchina, C. R., Salazar, V. L., Volmar, C. H., and Stoddard, P. K. (2001). Plasticity of the electric organ discharge waveform of male Brachyhypopomus pinnicaudatus. II. Social effects. J. Comp. Physiol. A 187, 45–52. doi: 10.1007/s003590000176
Frehse, F. D. A., Weyl, O. L. F., and Vitule, J. R. S. (2020). Comparison of visual census and underwater video for fish sampling in Neotropical reservoirs. Environ. Biol. Fish 103, 1269–1277. doi: 10.1007/s10641-020-01021-3
Frehse, F. D. A., Weyl, O. L. F., and Vitule, J. R. S. (2021). Differential use of artificial habitats by native and non-native fish species in Neotropical reservoirs. Hydrobiologia 848, 2355–2367. doi: 10.1007/s10750-021-04564-3
Friedman, M. A., and Hopkins, C. D. (1996). Tracking individual mormyrid electric fish in the field using electric organ discharge waveforms. Anim. Behav. 51, 391–407. doi: 10.1242/jeb.226340
Frøland Steindal, I. A., and Whitmore, D. (2019). Circadian clocks in fish-what have we learned so far? Biology 2019:8. doi: 10.3390/biology8010017
Greenbank, J. (1945). Limnological conditions in ice-covered lakes, especially as related to winter-kill of fish. Ecol. Monogr. 15, 343–392. doi: 10.2307/1948427
Häfker, N. S., and Tessmar-Raible, K. (2020). Rhythms of behavior: are the times changin’? Curr. Opin. Neurobiol. 60, 55–66. doi: 10.1016/j.conb.2019.10.005
Hamilton, S. K. (2010). Biogeochemical implications of climate change for tropical rivers and floodplains. Hydrobiologia 657, 19–35. doi: 10.1007/s10750-009-0086-1
Hammerschlag, N., and Heithaus Serafy, J. E. (2010). Influence of predation risk and food supply on nocturnal fish foraging distributions along a mangrove–seagrass ecotone. Mar. Ecol. Prog. Ser. 414, 223–235. doi: 10.3354/meps08731
Harder, W., Schief, A., and Uhlemann, H. (1964). Zur Funktion des elektrischen Organs von Gnathonemus petersii (Gthr. 1862) (Mormyriformes, Teleostei). Z. vergl. Physiologie 48, 302–331. doi: 10.1007/bf00339459
Henninger, J., Krahe, R., Kirschbaum, F., Grewe, J., and Benda, J. (2018). Statistics of natural communication signals observed in the wild identify important yet neglected stimulus regimes in weakly electric fish. J. Neurosci. 38, 5456–5465. doi: 10.1523/JNEUROSCI.0350-18.2018
Henninger, J., Krahe, R., Sinz, F., and Benda, J. (2020). Tracking activity patterns of a multispecies community of gymnotiform weakly electric fish in their Neotropical habitat without tagging. J. Exp. Biol. 2020:223. doi: 10.1242/jeb.206342
Hobson, E. S. (1973). Diel feeding migrations in tropical reef fishes. Helgoländer wiss. Meeresunters 24, 361–370. doi: 10.1007/bf01609526
Hopkins, C. D. (1980). Evolution of electric communication channels of mormyrids. Behav. Ecol. Sociobiol. 7, 1–13. doi: 10.1007/bf00302513
Hopkins, C. D., and Bass, A. H. (1981). Temporal coding of species recognition signals in an electric fish. Science 212, 85–87. doi: 10.1126/science.7209524
Hurd, M., DeBruyne, J., Straume, M., and Cahill, G. M. (1998). Circadian rhythms of locomotor activity in zebrafish. Physiol. Behav. 65, 465–472. doi: 10.1016/s0031-9384(98)00183-8
Hut, R. A., Paolucci, S., Dor, R., Kyriacou, C. P., and Daan, S. (2013). Latitudinal clines: an evolutionary view on biological rhythms. Proc. R. Soc. B 280:20130433. doi: 10.1098/rspb.2013.0433
Ibbotson, A. T., Beaumont, W. R. C., Pinder, A., Welton, S., and Ladle, M. (2006). Diel migration patterns of Atlantic salmon smolts with particular reference to the absence of crepuscular migration. Ecol. Freshw. Fish 15, 544–551. doi: 10.1111/j.1600-0633.2006.00194.x
Kannan, V., and Job, S. V. (1980). Diurnal depth-wise and seasonal changes of physico-chemical factors in Sathiar reservoir. Hydrobiologia 70, 103–117. doi: 10.1007/bf00015496
Kaufman, L., and Ochumba, P. (1993). Evolutionary and conservation biology of cichlid fishes as revealed by faunal remnants in northern Lake Victoria. Conserv. Biol. 7, 719–730. doi: 10.1046/j.1523-1739.1993.07030719.x
Kirschbaum, F. (1995). “Reproduction and devlopment in mormyriform and gymnotiform fishes,” in Electric fishes: history and behavior, ed. P. Moller (London: Chapman and Hall), 267–301.
Kirschbaum, F., and Schugardt, C. (2002). Reproductive strategies and developmental aspects in mormyrid and gymnotiform fishes. J. Physiol. 96, 557–566. doi: 10.1016/S0928-4257(03)00011-1
Kramer, D. L., Manley, D., and Bourgeois, R. (1983). The effect of respiratory mode and oxygen concentration on the risk of aerial predation in fishes. Can. J. Zool. 61, 653–665. doi: 10.1139/z83-087
Kruger, E. J. (1973). Autumn feeding cycle of the bull-dog fish, Gnathonemus macrolepidotus (Pisces, Mormyridae). Afr. Zool. 8, 25–34. doi: 10.1080/00445096.1973.11447463
Lewis, J. E., Gilmour, K. M., Moorhead, M. J., Perry, S. F., and Markham, M. R. (2014). Action potential energetics at the organismal level reveal a trade-off in efficiency at high firing rates. J. Neurosci. 34, 197–201. doi: 10.1523/JNEUROSCI.3180-13.2014
Lewis, W. M. (1970). Morphological adaptations of cyprinodontoids for inhabiting oxygen deficient waters. Copeia 1970, 319–326. doi: 10.2307/1441653
Lissmann, H. W. (1951). Continuous electrical signals from the tail of a fish. Gymnarc. Niloticus Cuv. 167, 201–202. doi: 10.1038/167201a0
Lissmann, H. W. (1958). On the function and evolution of electric organs in fish. J. Exp. Biol. 35, 156–191. doi: 10.1242/jeb.35.1.156
Lissmann, H. W. (1961). “Ecological studies on gymnotids,” in Bioelectrogenesis. A comparative survey of its mechanisms with particular emphasis on electric fishes, eds C. Chagas and A. P. de Carvalho (Amsterdam: Elsevier), 215–226.
Lissmann, H. W., and Machin, K. E. (1958). The mechanism of object location in Gymnarchus niloticus and similar fish. J. Exp. Biol. 35, 451–486. doi: 10.1242/jeb.35.2.451
Lissmann, H. W., and Schwassmann, H. O. (1965). Activity rhythm of an electric fish, Gymnorhamphichthys hypostomus, Ellis. J. Comp. Physiol. A 51, 153–171. doi: 10.1007/bf00299291
Lundberg, J. G., Lewis, W. M., Saunders, J. F., and Mago-Leccia, F. (1987). A major food web component in the orinoco river channel: evidence from planktivorous electric fishes. Science 237, 81–83. doi: 10.1126/science.237.4810.81
Madhav, M. S., Jayakumar, R. P., Demir, A., Stamper, S. A., Fortune, E. S., and Cowan, N. J. (2018). High-resolution behavioral mapping of electric fishes in Amazonian habitats. Sci. Rep. 8:5830. doi: 10.1038/s41598-018-24035-5
Markham, M. R., Ban, Y., McCauley, A. G., and Maltby, R. (2016). Energetics of sensing and communication in electric fish: a blessing and a curse in the anthropocene? Integr. Comp. Biol. 56, 889–900. doi: 10.1093/icb/icw104
Markham, M. R., McAnelly, M. L., Stoddard, P. K., and Zakon, H. H. (2009). Circadian and social cues regulate ion channel trafficking. PLoS Biol. 7:e1000203. doi: 10.1371/journal.pbio.1000203
Migliaro, A., and Silva, A. C. (2016). Melatonin regulates daily variations in electric behavior arousal in two species of weakly electric fish with different social structures. Brain Behav. Evol. 87, 232–241. doi: 10.1159/000445494
Migliaro, A., Moreno, V., Marchal, P., and Silva, A. C. (2018). Daily changes in the electric behavior of weakly electric fish naturally persist in constant darkness and are socially synchronized. Biol. Open 2018:7. doi: 10.1242/bio.036319
Moller, P. (1970). ‘Communication’ in weakly electric fish, Gnathonemus niger (Mormyridae) I. Variation of electric organ discharge (EOD) frequency elicited by controlled electric stimuli. Anim. Behav. 18, 768–786. doi: 10.1016/0003-3472(70)90026-6
Moller, P., and Bauer, R. (1973). ‘Communication’ in weakly electric fish, Gnathonemus petersii (Mormyridae) II. Interaction of electric organ discharge activities of two fish. Anim. Behav. 21, 501–512. doi: 10.1016/s0003-3472(73)80010-7
Moller, P., Serrier, J., and Bowling, D. (1989). Electric Organ Discharge Displays during Social Encounter in the Weakly Electric Fish Brienomyrus niger L. (Mormyridae). Ethology 82, 177–191. doi: 10.1111/j.1439-0310.1989.tb00498.x
Moller, P., Serrier, J., Belbenoit, P., and Push, S. (1979). Notes on ethology and ecology of the Swashi River mormyrids (Lake Kainji, Nigeria). Behav. Ecol. Sociobiol. 4, 357–368. doi: 10.1007/bf00303242
Mönck, H. J., Jorg, A., Falkenhausen, T. V., Tanke, J., Wild, B., Dormagen, D., et al. (2018). BioTracker: an open-source computer vision framework for visual animal tracking. arXiv 1803:07985v1.
Moulton, T. L., Chapman, L. J., and Krahe, R. (2020). Effects of hypoxia on aerobic metabolism and active electrosensory acquisition in the African weakly electric fish Marcusenius victoriae. J. Fish Biol. 96, 496–505. doi: 10.1111/jfb.14234
Nagelkerken, I., Dorenbosch, M., Verberk, W., La Cocheret, de Morinière, E., and van der Velde, G. (2000). Day-night shifts of fishes between shallow-water biotopes of a Caribbean bay, with emphasis on the nocturnal feeding of Haemulidae and Lutjanidae. Mar. Ecol. Prog. Ser. 194, 55–64. doi: 10.3354/meps194055
Neilson, J. D., and Perry, R. I. (1990). Diel Vertical Migrations of Marine Fishes: an Obligate or Facultative Process? in Advances in Marine Biology, Vol. 26. Amsterdam: Elsevier, 115–168.
Nyboer, E. A., and Chapman, L. J. (2013). Movement and home range of introduced Nile perch (Lates niloticus) in Lake Nabugabo, Uganda: implications for ecological divergence and fisheries management. Fish. Res. 137, 18–29. doi: 10.1016/j.fishres.2012.08.003
Ogutu-Ohwayo, R. (1993). The effects of predation by Nile perch, Lates niloticus L., on the fish of Lake Nabugabo, with suggestions for conservation of endangered endemic cichlids. Conserv. Biol. 7, 701–711. doi: 10.1046/j.1523-1739.1993.07030701.x
Pelster, B., and Egg, M. (2018). Hypoxia-inducible transcription factors in fish: expression, function and interconnection with the circadian clock. J. Exp. Biol. 2018:221. doi: 10.1242/jeb.163709
Pollock, M. S., Clarke, L. M. J., and Dubé, M. G. (2007). The effects of hypoxia on fishes. from ecological relevance to physiological effects. Environ. Rev. 15, 1–14. doi: 10.1139/a06-006
Pringle, R. M. (2005). The origins of the Nile perch in Lake Victoria. Bioscience 55, 780–787. doi: 10.1641/0006-3568(2005)055[0780:tootnp]2.0.co;2
Reardon, E. E., and Chapman, L. J. (2008). Reproductive seasonality in a swamp-locked African cichlid. Ecol. Freshw. Fish 17, 20–29. doi: 10.1111/j.1600-0633.2007.00251.x
Reebs, S. G. (1994). The anticipation of night by fry-retrieving convict cichlids. Anim. Behav. 48, 89–95. doi: 10.1006/anbe.1994.1214
Reebs, S. G. (2002). Plasticity of diel and circadian activity rhythms in fishes. Rev. Fish Biol. Fish. 12, 349–371. doi: 10.1023/A:1025371804611
Rooker, J. R., and Dennis, G. D. (1991). Diel, lunar and seasonal changes in a mangrove fish assemblage off southwestern Puerto Rico. Bull. Mar. Sci. 49, 684–698.
Rowland, W. J. (1999). Studying visual cues in fish Bbhavior: A review of ethological techniques. Environ. Biol. Fish. 56, 285–305. doi: 10.1023/a:1007517720723
Saint-Paul, U., and Soares, G. M. (1987). Diurnal distribution and behavioral responses of fishes to extreme hypoxia in an Amazon floodplain lake. Environ. Biol. Fish. 20, 91–104. doi: 10.1007/bf00005289
Salazar, V. L., and Stoddard, P. K. (2008). Sex differences in energetic costs explain sexual dimorphism in the circadian rhythm modulation of the electrocommunication signal of the gymnotiform fish Brachyhypopomus pinnicaudatus. J. Exp. Biol. 211, 1012–1020. doi: 10.1242/jeb.014795
Salazar, V. L., Krahe, R., and Lewis, J. E. (2013). The energetics of electric organ discharge generation in gymnotiform weakly electric fish. J. Exp. Biol. 216, 2459–2468. doi: 10.1242/jeb.082735
Sánchez-Vázquez, F. J., Madrid, J. A., and Zamora, S. (1995). Circadian rhythms of feeding activity in sea bass, Dicentrarchus labrax L.: dual phasing capacity of diel demand-feeding pattern. J. Biol. Rhythms 10, 256–266. doi: 10.1177/074873049501000308
Sandbichler, A. M., Jansen, B., Peer, B. A., Paulitsch, M., Pelster, B., and Egg, M. (2018). Metabolic plasticity enables circadian adaptation to acute hypoxia in zebrafish cells. Cell. Physiol. Biochem. 46, 1159–1174. doi: 10.1159/000489058
Savitzky, A., and Golay, M. J. E. (1964). Smoothing and differentiation of data by simplified least squares procedures. Anal. Chem. 36, 1627–1639. doi: 10.1021/ac60214a047
Scheffel, A., and Kramer, B. (1997). Electrocommunication and social behaviour in Marcusenius senegalensis (Mormyridae, Teleostei). Ethology 103, 404–420. doi: 10.1111/j.1439-0310.1997.tb00156.x
Schödel, J., Oikonomopoulos, S., Ragoussis, J., Pugh, C. W., Ratcliffe, P. J., and Mole, D. R. (2011). High-resolution genome-wide mapping of HIF-binding sites by ChIP-seq. Blood 117, e207–e217. doi: 10.1182/blood-2010-10-314427
Semesi, I. S., Beer, S., and Björk, M. (2009). Seagrass photosynthesis controls rates of calcification and photosynthesis of calcareous macroalgae in a tropical seagrass meadow. Mar. Ecol. Prog. Ser. 382, 41–47. doi: 10.3354/meps07973
Sikkel, P. C., Welicky, R. L., Artim, J. M., McCammon, A. M., Sellers, J. C., Coile, A. M., et al. (2017). Nocturnal migration reduces exposure to micropredation in a coral reef fish. Bull. Mar. Sci. 93, 475–489. doi: 10.5343/bms.2016.1021
Silva, A. C., Perrone, R., and Macadar, O. (2007). Environmental, seasonal, and social modulations of basal activity in a weakly electric fish. Physiol. Behav. 90, 525–536. doi: 10.1016/j.physbeh.2006.11.003
Stager, J. C., Westwood, J., Grzesik, D., and Cumming, B. F. (2005). A 5500-year environmental history of Lake Nabugabo, Uganda. Palaeogeogr. Palaeoclimatol. Palaeoecol. 218, 347–354. doi: 10.1016/j.palaeo.2004.12.025
Stoddard, P. K., Markham, M. R., Salazar, V. L., and Allee, S. (2007). Circadian rhythms in electric waveform structure and rate in the electric fish Brachyhypopomus pinnicaudatus. Physiol. Behav. 90, 11–20. doi: 10.1016/j.physbeh.2006.08.013
Sukhum, K. V., Freiler, M. K., Wang, R., and Carlson, B. A. (2016). The costs of a big brain. Extreme encephalization results in higher energetic demand and reduced hypoxia tolerance in weakly electric African fishes. Proc. R. Soc. B 2016:283. doi: 10.1098/rspb.2016.2157
Tabata, M., Minh-Nyo, M., Niwa, H., and Oguri, M. (1989). Circadian rhythm of locomotor activity in a teleost, Silurus asotus. Zool. Sci. 6, 367–375.
Talling, J. F. (1957). Diurnal changes of stratification and photosynthesis in some tropical African waters. Proc. R. Soc. B 147, 57–83. doi: 10.1098/rspb.1957.0036
Toerring, M. J., and Serrier, J. (1978). Influence of water temperature on the electric organ discharge (EOD) of the weakly electric fish Marcusenius cyprinoides (Mormyridae). J. Exp. Biol. 74, 133–150. doi: 10.1242/jeb.74.1.133
Townsend, S. A. (1999). The seasonal pattern of dissolved oxygen, and hypolimnetic deoxygenation, in two tropical Australian reservoirs. Lakes Reserv. 4, 41–53. doi: 10.1046/j.1440-1770.1999.00077.x
Trewavas, E. (1933). Scientific results of the Cambridge Expedition to the East African Lakes, 1930-1.-11. The cichlid fishes. Zool. J. Linn. Soc. 38, 309–341. doi: 10.1111/j.1096-3642.1933.tb00062.x
Ultsch, G. R., Boschung, H., and Ross, M. J. (1978). Metabolism, critical oxygen tension, and habitat selection in darters (Etheostoma). Ecology 59, 99–107. doi: 10.2307/1936635
Vanderpham, J. P., Nakagawa, S., and Closs, G. P. (2012). Diel variation in use of cover and feeding activity of a benthic freshwater fish in response to olfactory cues of a diurnal predator. Environ. Biol. Fish. 93, 547–556. doi: 10.1007/s10641-011-9949-1
Volpato, G. L., and Trajano, E. (2005). Biological Rhythm in The Physiology of Tropical Fishes. Cambridge, MA: Academic Press, 101–153.
Wenger, R. H., Stiehl, D. P., and Camenisch, G. (2005). Integration of oxygen signaling at the consensus HRE. Sci. 2005:re12. doi: 10.1126/stke.3062005re12
Whitmore, D., Foulkes, N. S., and Sassone-Corsi, P. (2000). Light acts directly on organs and cells in culture to set the vertebrate circadian clock. Nature 404, 87–91. doi: 10.1038/35003589
Keywords: behavioral activity, habitat use, electric organ discharge, hypoxia, swim behavior
Citation: Mucha S, Oehlert F, Chapman LJ and Krahe R (2022) A Spark in the Dark: Uncovering Natural Activity Patterns of Mormyrid Weakly Electric Fish. Front. Ecol. Evol. 10:870043. doi: 10.3389/fevo.2022.870043
Received: 05 February 2022; Accepted: 14 April 2022;
Published: 15 June 2022.
Edited by:
Daniel Marques Almeida Pessoa, Federal University of Rio Grande do Norte, BrazilReviewed by:
Philip K. Stoddard, Florida International University, United StatesPeter Moller, Hunter College (CUNY), United States
Copyright © 2022 Mucha, Oehlert, Chapman and Krahe. This is an open-access article distributed under the terms of the Creative Commons Attribution License (CC BY). The use, distribution or reproduction in other forums is permitted, provided the original author(s) and the copyright owner(s) are credited and that the original publication in this journal is cited, in accordance with accepted academic practice. No use, distribution or reproduction is permitted which does not comply with these terms.
*Correspondence: Rüdiger Krahe, cnVlZGlnZXIua3JhaGVAaHUtYmVybGluLmRl
†ORCID: Stefan Mucha, orcid.org/0000-0001-9862-2497; Lauren J. Chapman, orcid.org/0000-0002-2802-5889; Rüdiger Krahe, orcid.org/0000-0003-1669-6121