- 1Department of Ecology and Evolution, Biophore, University of Lausanne, Lausanne, Switzerland
- 2Aquatic Ecology and Toxicology Group, Center of Organismic Studies, University of Heidelberg, Heidelberg, Germany
Salmonids are a socioeconomically and ecologically important group of fish that are often managed by stocking. Little is known about potential sex-specific effects of stocking, but recent studies found that the sexes differ in their stress tolerances already at late embryonic stage, i.e., before hatchery-born larvae are released into the wild and long before morphological gonad formation. It has also been speculated that sex-specific life histories can affect juvenile growth and mortality, and that a resulting sex-biassed demography can reduce population growth. Here we test whether juvenile brown trout (Salmo trutta) show sex-specific life histories and whether such sex effects differ in hatchery- and wild-born fish. We modified a genetic sexing protocol to reduce false assignment rates and used it to study the timing of sex differentiation in a laboratory setting, and in a large-scale field experiment to study growth and mortality of hatchery- and wild-born fish in different environments. We found no sex-specific mortality in any of the environments we studied. However, females started sex differentiation earlier than males, and while growth rates were similar in the laboratory, they differed significantly in the field depending on location and origin of fish. Overall, hatchery-born males grew larger than hatchery-born females while wild-born fish showed the reverse pattern. Whether males or females grew larger was location-specific. We conclude that juvenile brown trout show sex-specific growth that is affected by stocking and by other environmental factors that remain to be identified.
Introduction
Intersexual differences in life history strategies exist across diverse unrelated taxa (Brooks and Garratt, 2017; Li and Kokko, 2019) and can affect population dynamics and extinction risks (Hendry et al., 2018; Cally et al., 2019). Despite this, intersexual differences in early life history strategies receive little attention when studying the fitness of individuals and/or assessing population viability (Tarka et al., 2018). Stock enhancement practices primarily focus on the release of early life stages but sex effects are only rarely considered [but see for example Huertas and Cerdà (2006), Lenz et al. (2007), and Svobodová et al. (2020)]. Sex-specific early life history strategies, and their relationship with stock enhancement practices, may thus be a hidden driver of population dynamics and need to be studied in more detail.
Sex can affect physiology (Grilo et al., 2018; Millington and Rideout, 2018; Selmoni et al., 2019), development (Maitre et al., 2017; Gurley et al., 2018) and behaviour (Magurran and Garcia, 2000; Klemetsen et al., 2003; Pearse et al., 2019) well before gonad formation (Tsai et al., 2009; Mousavi et al., 2021). Divergent selection pressures may thus act on the sexes even at early developmental stages (Bolund et al., 2013; Schroeder et al., 2013; Forsman, 2018) leading to the development of distinct life history strategies and sometimes even sex-specific mortality (Badyaev et al., 2002; Wiklund et al., 2003; Husby et al., 2006; but see Dietrich et al., 2003; Altwegg et al., 2007). Consequently, operational sex ratios within populations can be biassed, and this can affect population dynamics and extinction risks through a reduced effective population size (Donald, 2007; Geffroy and Wedekind, 2020), behavioural changes (Eberhart-Phillips et al., 2018) and altering the direction of evolution (Forsman, 2018; Gomes et al., 2018). While sex-specific effects can be profound, they are generally understudied in ecology, evolution and conservation biology (e.g., Einum and Fleming, 2001; Nislow and Armstrong, 2012; Skov et al., 2012; Tsuboi et al., 2013; Louison and Stelzer, 2016; Wilkins and Marsden, 2021).
Salmonid species exhibit diverse life-history strategies and thus form excellent models to study the importance and consequences of sex-specific life history strategies at early developmental stages (Birnie-Gauvin et al., 2021). Sex differences have been reported for growth (Yamamoto, 2004; Maitre et al., 2017), aggression (Rgen et al., 2001), migration strategies (Kelson et al., 2019; Nevoux et al., 2019; Eldøy et al., 2021) and size- and age-at-maturation (Young, 2005; McKinney et al., 2020; Mobley et al., 2021; Tréhin et al., 2021). During the embryonic phase, male lake char (Salvelinus umbla) were found to hatch earlier and be less susceptible to environmental stressors (Nusbaumer et al., 2021). In contrast, sex did not seem to influence emergence time, or morphological and physiological traits in newly emerged brown trout (Salmo trutta) (Régnier et al., 2015). However, male-specific mortality has been reported in the first month of development of brown trout leading to female-biassed sex ratios (Morán et al., 2016). Growth during the juvenile phase has been reported to be higher for males in masu salmon (Oncorhynchus masou) (Yamamoto, 2004), chinook salmon (Oncorhynchus tshawytscha) (Mizzau et al., 2013) and European grayling (Thymallus thymallus) (Maitre et al., 2017). The latter study showed that differences in growth rates in grayling can be attributed to a differential resource allocation between sexes. That is, gonadal differentiation is delayed in males which instead invest more in growth (Maitre et al., 2017). These intersexual differences could be large enough to cause female-biassed juvenile mortality and hence male-biassed adult sex ratios (Wedekind et al., 2013). Differences in sexual differentiation have also been reported for sea migrating brown trout but no sex-specific differences were recorded for growth (Dziewulska and Domagala, 2004). While sex-specific early life history strategies may be common in salmonids, they remain understudied which may partly be because of the challenges involved in sexing early life stages.
In Salmoninae and Thymallinae (two salmonid subfamilies), sex has been shown to be under the control of a conserved master sex-determining gene (i.e., sdY) (Yano et al., 2012, 2013). This allowed for the development of genetic sexing methods. Initially a simple PCR-based presence-absence test was proposed (Yano et al., 2013) which was later modified to a multiplex PCR allowing for high-throughput genetic sexing and genotyping (Quéméré et al., 2014). However, the use of a presence-absence of the sdY gene appears to be prone to false positive male identifications with the percentage of females misidentified as males ranging from 1 to 71% (Ueda et al., 2021). Various reasons for such misidentifications have been proposed (Ayllon et al., 2020). Firstly, mislabelling errors or low levels of between sample cross-contamination could result in a positive detection of the sdY gene in females. Second, discrepancies between genetic and phenotypic sex can arise due to the genetic silencing of the sdY gene (e.g., Yano et al., 2014) or environmentally driven sex reversal (e.g., Magerhans et al., 2009; Weber et al., 2020). Finally, non-functional copies of the sdY gene may be present in female salmonids and lead to the observation of high error rates in the genetic identification of phenotypic females (Ayllon et al., 2020; Bertho et al., 2021). While genetic sexing of early life stages of salmonid species is now feasible, improvements to genetic sexing methods are still needed to reduce misidentifications.
Brown trout are a keystone species within their natural range, have been translocated across the globe (Halverson, 2008; Hunt and Jones, 2018; Hasegawa, 2020), and have great socio-economical value (Elliott, 1989; Liu et al., 2019). Because of their value and often high exploitation rates, restocking and stock-enhancement are a common management practice for brown trout populations (Aas et al., 2018; Cucherousset et al., 2021). A large body of literature is thus available focussing on the effectiveness of these management practises (Pinter et al., 2018; Dieterman et al., 2020), the intraspecific competition between wild- and hatchery-born individuals (Einum and Fleming, 2001; Araki et al., 2008) and the overall population-level consequences (Meier et al., 2011; Leitwein et al., 2018; Klütsch et al., 2019). However, to the best of our knowledge, sex-specific effects have not been considered yet when evaluating the demographic and genetic consequences of restocking and stock-enhancement in this or any other salmonid fish.
Here we combine a large-scale field experiment with a controlled rearing of males and females under laboratory conditions to test for sex-specific growth and survival under various ecological conditions. The laboratory population also enabled continuous sampling for histological analyses to study possible sex differences in the timing of sex differentiation. The field experiment allows us to test whether sex-specific effects on growth and survival differ for hatchery- and wild-born fish that occupy the same habitat, and whether such differences are habitat specific.
Materials and Methods
Study Populations
We focus here on different brown trout populations within the Aare catchment between Lake Thun and the city of Bern (Switzerland) (Figure 1). Within the study system, multiple genetically and phenotypically different brown trout populations occupy the different tributaries (Stelkens et al., 2012). Individuals used in this study are either wild-caught or originate from the yearly stock-enhancement programmes (F1 fish) conducted only by the Fishery Inspectorate of the Bern canton (continuously for more than 25 years). Stocking fish without their consent would be illegal and is unlikely in the study area, especially in the case of 0 + brown trout.
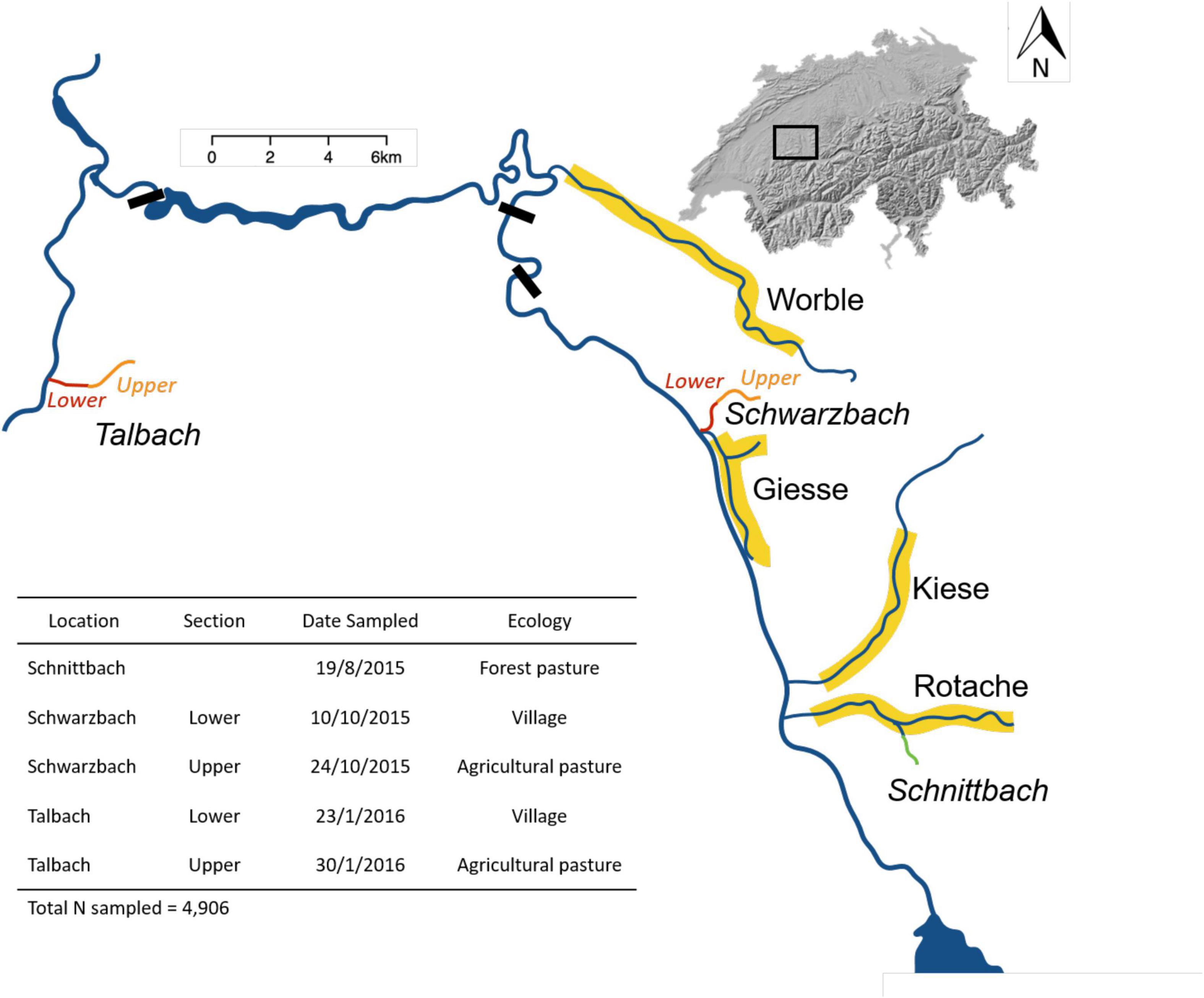
Figure 1. Overview of the study area with the key stream locations used in the current study. Areas highlighted in yellow were the source populations of mature fish used for experimental breeding. Orange, red, and green colours represent stream locations stocked with hatchery-born fish and sampled for the field-based study. The ecology type of each of the latter stream locations is given in the table.
Laboratory Study
For the laboratory study, breeding adults were collected around the beginning of the spawning season from the river Kiese through backpack electrofishing and kept in a hatchery (i.e., Fischereistützpunkt in Reutigen) until ovulation allowed the stripping of eggs. On the 28th November and 4th December 2018, 15 males and 20 females were haphazardly selected for block-wise full-factorial breeding. On the respective dates, one 8 × 10 breeding block (number of females crossed with number of males) and one 7 × 10 block were created. Adult breeders were narcoticized using Tricaine Methanesulfonate (i.e., 0.075 g/L buffered with 0.15 g/L NaHCO3), stripped for gametes for full-factorial breeding, photographed, measured (i.e., standard length and wet weight) and fin clips were collected and stored in 70% ethanol. Fertilised eggs were held in the hatchery using typical hatchery-rearing methods while adult fish were released in their stream of origin after breeding. Post-hatching, fish were kept in a common environment in the hatchery in large tanks with a circulating water current, with a water temperature of 9°C, and fed ad libitum with size-appropriate commercial dry fish food. The peak of hatching occurred on 17th February 2019 (i.e., ca. 77 days post-fertilisation). Further reports of fish age are based on this hatching date.
A sample of 816 hatchery-raised fish were moved to the laboratory at the University of Lausanne at 190 days post-hatching to study the effects of sympatric diclofenac pollution and proliferative kidney disease infection (Palejowski et al., in preparation). They were randomly divided into 12 groups, with each group containing 68 fish in a 120 L aquaria filled with tap water. Each aquarium was constantly pump-filtered, the water was aerated, a water current was applied, and to reduce stress to the fish a structure was provided to hide under and the front of the aquarium was obscured by an opaque cover. Aquaria were kept in a temperature-controlled room with a 12:12 light-dark cycle at a water temperature of 9°C for 3 weeks to allow the fish to acclimatise to the laboratory environment. The temperature was then gradually raised from 9°C to a final mean of 14.6°C (SD = 0.49°C). Water was changed daily for the first 35 days in the laboratory and every weekday from then on, changing 15 L for the first 70 days then 40 L per change for the following 55 days. Fish were fed ad libitum with size-appropriate commercial dry fish food and water quality was monitored weekly using JBL Pro Scan® tests (JBL GmbH, Germany) and JBL Denitrol® was added to correct excessive nitrate levels if necessary. As population density is known to affect salmonid health, and differences in densities may therefore result in differences in development strategy (Pickering and Pottinger, 1987; Mazur and Iwama, 1993) the number of fish was equalised between aquaria once a week if mortality occurred. Population densities were reduced to the level of the least dense aquaria. This was done by dividing the aquaria into a top-down two-dimensional grid, choosing a grid square using a random number generator and removing and euthanising the nearest individual.
The timing of sexual differentiation was studied by monthly sampling of 24 fish from 129 days post-hatching onward for a period of 5 months (Table 1). During each of timespoints 1–3, 24 fish were haphazardly taken from the fish that had been left at Fischereistützpunkt Reutigen, while all later samples were taken from the laboratory population (all originating from the same breeding experiment). For sampling timespoints 4 and 5, 2 fish were randomly selected from each of the 12 aquaria and euthanised. Fish were euthanised with an overdose of KoiMed® Sleep (KOI&BONAI, Bühlertann, Germany) at a concentration of 0.7 ml/L. For all fish in all timespoints, total length and weight measures were taken and fin clips were stored in 70% ethanol at −20°C for genetic sexing. The heads (caudal of the operculum) and tails (rostral of the anus) were removed, and the remainder of the bodies were immersed in histological tissue fixative (Hartmann’s Fixative, H0290, Sigma-Aldrich, Germany) for 2 weeks to be used for phenotypical sexing and analysis of sexual differentiation. Fixative solution was changed after 1 week for better bone decalcification. After immersion for 2 weeks in histological tissue fixative the bodies were embedded in cassettes and dehydrated using a LEICA TP1020 Tissue Processor (LEICA, Tempe, AZ, United States). After 48 h of dehydration, the tissues were cast in paraffin wax (Histoplast P, Serva, Heidelberg, Germany) with a paraffin dispenser insert (Leica EG1150H, Leica, Tempe, AZ, United States) and cooled to obtain solid paraffin blocks. Using a microtome, 5μm cuts were made in a coronal plane, with a ventral to dorsal direction. Cuts were then floated in a 35°C water bath and collected on glass slides (Thermo Scientific™ SuperFrost Plus™). The sections were deparaffinised, using a xylene substitute (X-tra-solv, Medite, Burgdorf, Germany) dehydrated and stained with Mayer’s haematoxylin and eosin in a two-stage procedure according to standard HE-staining protocols (Aescht et al., 2010). Sections were then covered by applying drops of glue (X-tra-Kit, Medite, Burgdorf, Germany) followed by a cover glass (Sail brand™). An average of 12 slides per fish were made and checked for the presence of gonads. All sections containing gonads were analysed for the presence and developmental stage of the gonads (Supplementary Material). Phenotypical sex was determined by analysing cellular composition of the gonads with fish being classified using four categories: (i) undeveloped genetic male (only immature germ cells are present in the gonad), (ii) phenotypically differentiated male, (iii) undeveloped genetic female, (iv) phenotypically differentiated female (Supplementary Material). One individual was removed before final analysis as phenotypic and genotypic sex were inconsistent likely due to a labelling error during histological slide preparation. To study sex-specific growth and mortality, from timespoints 4 onward this dataset was expanded, randomly sampling five fish per aquaria once every 2 weeks for a total of 3 months. Fish to sample were randomly selected via the method used to equalise aquaria densities as described above. All fish sampled were euthanised and fin clips were taken for genetic sexing, but histological analyses were only performed on two of the five fish per aquaria collected at timespoints 4 and 5. Over time, the number of aquaria reduced due to mortality making it impossible to continuously collect five fish per timespoints (see Supplementary Material for full details).
Field Experiment
In autumn 2014, adult brown trout were caught and used to further optimise genetic sexing methods and create offspring used in the field experiment. Breeding males (♂n = 358) and females (♀n = 493) were collected around the beginning of the spawning season from different spawning tributaries representing six genetically different populations (Stelkens et al., 2012) in the context of a parallel study (Bylemans et al., in preparation). Adult fish were caught using backpack electrofishing and kept at the hatchery until ovulation allowed the stripping of eggs. All adults were narcoticised when they were ready to spawn and gametes were stripped for block-wise full-factorial in vitro fertilisations (Marques da Cunha et al., 2019). Photographs were taken for all adult fish as well as measurements (i.e., standard length and wet weight) and fin clips for molecular analyses. Offspring of the full factorial breeding designs from three different populations were used as the basis for the field experiment here. Breeders from the Giesse were used to create 7 full factorial breeding blocks (i.e., three 2 × 2, one 2 × 3, two 5 × 5 and one 6 × 6 block) producing 14,812 fertilised eggs. A total of 2,659 fertilised eggs were produced from 6 full factorial breeding blocks with breeders from the Rotache (i.e., three 2 × 2, one 3 × 2 and two 5 × 5 blocks). Breeders originating from the Worble were used in 13 full factorial breeding blocks (i.e., nine 2 × 2 and four 5 × 5 blocks) and generated a total of 13,643 fertilised eggs. Breeding was done on Nov 19th and 26th 2014 (Giesse and Worble) and on December 3rd 2014 (Rotache). A sample of 24 eggs per each sibgroup was taken to the laboratory for parallel studies (Marques da Cunha et al., 2019). The remaining eggs were incubated under standard hatchery conditions until hatching and released into the streamlets Schwarzbach (offspring from Giesse), Schnittlauch (offspring from Rotache) and Talbach (offspring from Worble) (Figure 1). No other brown trout were stocked into these streamlets in 2015. Stocking was done in early March 2015 about 17 to 18 days at 8°C after peak hatching, i.e., at the developmental stage that corresponds to the stage at emergence from gravel (yolk sacs are not fully used up yet and the larvae start to take up food items).
Between August 2015 and January 2016, juvenile fish were sampled from the three streams stocked with hatchery-born fish. Only one stream section was sampled for Schnittlauch while a lower and upper stream section was sampled for Schwarzbach and Talbach (Table 1 and Figure 1). All five locations differ in ecology and anthropogenic pressures (Figure 1). Backpack electrofishing was used to sample locations and for all fish with a body length below 18 cm. Fish were narcoticized with Tricaine Methanesulfonate as described above, photographed on a scale to later take weight and fork length, and fin clip were stored in 70% ethanol for molecular analyses. All fish were released into the wild after they had recovered from the narcosis. A subset of tissue samples was randomly selected and used for further molecular analyses (Table 1).
Molecular Analyses
Tissue samples from both the laboratory and field experiments were used in further molecular analyses. For all samples, DNA was extracted using the BioSprint® 96 robot following the manufacturer’s protocol (Qiagen GmbH, Hilden, Germany). A total of 94 samples and two negative controls were included in each batch extraction to assess potential cross-contamination. DNA extracts were quantified using a HS dsDNA assay on a Qubit® 2.0 Fluorometer (Life Technologies, Carlsbad, CA, United States). DNA concentrations were standardised to 20 ng/μL where possible and samples with lower DNA concentrations were left undiluted for further analyses.
Standardised DNA extracts were sent to Ecogenics GmbH (Balgach, Switzerland) for microsatellite typing at 13 loci (i.e., Brun13, Brun25, BS131, MST-15, Mst543, MST-591, Ssa-197, Ssa-85, SSOSL438, Str12INRA, Str2INRA, Str58CNRS, and T3-13) using in house protocols (see Supplementary Material for full details). Amplification of microsatellite loci was conducted in three multiplex reactions. Multiplex one and two, each containing primers for the amplification of four microsatellites, was performed using 2X QIAGEN® Multi-plex PCR Master Mix (Qiagen GmbH, Hilden, Germany). Multiplex three included primers for the amplification of five microsatellite loci and was amplified using 2X HotStarTaq Mastermix (Qiagen GmbH, Hilden, Germany). Before thermal cycling, a prolonged denaturation step (95°C for 15 min) was included followed by 35 cycles with 94°C for 30 s, 58°C for 90 s, 72°C for 60 s and a final elongation step of 30 min at 72°C. Fragment analyses were performed on a 3730XL DNA Analyser (Applied Biosystems, Foster City, CA, United States) with a GeneScan LIZ500 size standard (Applied Biosystems) and allele calling was performed using the GeneMarker V2.6.4 software (SoftGenetics LLC, State College, PA, United States).
Genetic sexing of all samples was achieved through a modification of the protocol from Quéméré et al. (2014). As previously mentioned, presence-absence detections of the male specific sdY gene fragment can be prone to errors. While errors due to mislabelling, gene silencing and environmentally driven sex reversal are challenging to detect, the potential influences of small levels of cross contamination and the presence of pseudogenes could be mitigated through a more quantitative approach. In a XY sex-determination system, the abundance of the sdY fragment in male individuals should be approximately half that of any autosomal marker (i.e., a relative abundance of 0.5) while in females the sdY fragment should be absent (i.e., a relative abundance of 0). Small levels of cross contamination, especially DNA transfer from males to females, may result in the detection of the sdY fragment in females but the abundance of the sdY fragment relative to autosomal markers should stay well below 0.5 and close to 0. The presence of non-functional sdY pseudogenes, which can be amplified with the target specific primers but possibly with a reduced efficiency, will also result in shifts in the relative abundances of the sdY fragment. Nonetheless, in the majority of cases relative abundance measures of the sdY fragment should always be higher in phenotypic males (see Ayllon et al., 2020). Here we achieve a relative quantification of the sdY fragment by incorporating the target specific primers (Quéméré et al., 2014) into the first microsatellite multiplex assay (Supplementary Material). Genetic sexing was then achieved by calculating peak area ratios between the sdY fragment and the autosomal MST-591 microsatellite marker to obtain a more quantitative measure. Under a perfect XY sex-determining system it can be expected that peak area ratios are 0 for females and 0.5 for males. This protocol was applied to all 851 adult breeders collected in the context of this and a parallel study (Bylemans et al., in preparation), whose phenotypic sex was accurately determined through gamete collections, for validation and determining optimal threshold value for assigning genetic sexing. The validated and optimised protocol was subsequently used for the genetic sexing of all juvenile tissue samples from the laboratory study and field experiment.
Data Analyses
Statistical analyses were performed in R version 3.6.1 (R Development Core Team, 2010). Individuals from the laboratory (n = 1) and field experiment (n = 17) whose sex could not be determined (e.g., due to a failed amplification of the MST-591RB reference) were removed prior to analyses. Statistical significance was evaluated using an alpha value of 0.05.
Validation of the modified genetic sexing protocol was performed by comparing genetic sexing results based on the presence-absence of the sdY fragment and the semi-quantitative analyses. For each approach, the replicability was determined based on 19 males and 13 females for which molecular analyses were performed twice and the false assignment rates were calculated based on all the data from the 351 males and 490 females (Bylemans et al., in preparation) returning high quality genotyping and sexing results (i.e., percentage of missing loci <50% and the percentage of loci containing tri- and tetra-allelic loci <25%). The threshold value used for genetic sex determination using the peak area ratios was determined by evaluating a range (i.e., from 0.1 to 0.4 with steps of 0.025) of threshold values to minimise the false assignment rates.
Differences in the timing of sexual differentiation and growth rates in the laboratory study were assessed using a generalised linear regression model (glm) and a standard linear regression model (lm), respectively. Analyses of sexual differentiation included a total of 100 individual length measurements over five timespoints (i.e., 1–5) (Supplementary Material) with a binomial response variable indicating the differential status (i.e., one for individuals with differentiated gonads and zero for undifferentiated gonads). Sampling timespoints, genetic sex and the two-way interaction were included as categorical explanatory variables. Variable significance was assessed using the Anova function from the car package (Fox and Weisberg, 2019) conducting Kenwood–Roger F-tests with Satterthwaite degrees of freedom, applying a heteroscedasticity-corrected coefficient covariance matrix [based on Long and Ervin (2000)]. Type 3 sums of squares were calculated, then type 2 were calculated to interpret the final model if no significant effect of the interaction was found. Length was used as a proxy for growth rates as all sampled fish were approximately the same age. Total length data of 349 individuals (Supplementary Material) was included as continuous response variable with timespoints, genetic sex and the two-way interaction as categorical explanatory variables. Model assumptions were checked via visual inspection of residuals and quantile-quantile plots, and a heteroscedasticity-corrected coefficient covariance matrix was applied to correct for heteroscedasticity. Variable significance was tested as described above. Finally, sex-specific mortality in the laboratory study was assessed by testing for an association between sex ratios and sampling date, as a significant relationship would indicate a higher rate of mortality in one of the sexes. The data used consisted of all individual fish sampled as part of the laboratory study (Supplementary Material). A glm model with sampling date (converted to Julian date) as a continuous explanatory variable was used with the genetic sex (i.e., value of zero for females and one for males) as a binomial response variable, again conducting Kenwood-Roger F-tests. Model analysis was carried out with the Anova function from the car package as described above, calculating only type 2 sums of squares.
Prior to the analyses of the field experiment, juvenile fish representing the young-of-year (0 +) age class were selected based on density plots of the distribution of fork lengths, with all individuals with length less than a determined threshold value being characterised as 0 + (Supplementary Material). Individuals were further characterised as being hatchery- or wild-born through parental assignments using microsatellite data (see Supplementary Material for further details). The effects of sex and hatchery raising on growth and mortality were assessed using a standard linear regression model and Chi-squared goodness of fit tests, respectively. Fork length data from 748 individuals was modelled as a continuous response variable, including genetic sex, rearing origin, location, all two-way interactions and the three-way interaction as categorical explanatory variables. Model assumptions were checked via visual inspection of residuals and quantile-quantile plots, and a heteroscedasticity-corrected coefficient covariance matrix was applied to correct for heteroscedasticity. Variable significance was assessed using the Anova function from the car package, applying a heteroscedasticity-corrected coefficient covariance matrix. No evidence was found for a significant effect of the three-way interaction term so it was removed and the model was re-analysed. Type 3 sums of squares were calculated, reverting to type 2 to analyse the final model if no significant effect of the interaction was found. Analyses of sex-specific mortality were conducted testing for significant deviations from a 1:1 sex ratio in the entire study area, in each of the three streams, and in each of the five locations, using the chisq.test function from the stats package. Deviations from a 1:1 sex ratio were then also assessed within laboratory and wild populations within each of these groups using the same function.
Results
Molecular Sexing
Presence-absence analyses of the sdY fragment showed two distinct peaks around a 166 and 177 base-pair (bp) length roughly corresponding to the previously reported two haplotypes [i.e., Quéméré et al. (2014) reported two haplotypes with a length of 167 and 177 bp]. Replicability of genetic sexing results based on the presence-absence approach was 100 and 61.5% for the phenotypic males and females, respectively. False assignment rates for the presence-absence approach were 0 and 42.4% for phenotypic males and females, respectively. Of all cases testing positive for the sdY fragment, the majority (i.e., 98.1%) showed only a positive signal for the 177 bp haplotype. In the phenotypic males, 2.5% of the cases showed a positive detection for the 166 bp fragment with 1.9% showing only a signal for this haplotype and 0.5% testing positive for both haplotypes. All phenotypic females testing positive for the 166 bp haplotype (i.e., 0.9% of cases) did not show a signal at a 177 bp length. For the modified protocol, an initial evaluation of a range of threshold values (i.e., from 0.1 to 0.4) for the peak area ratio revealed that an intermediate value of 0.25 (i.e., between the 0 and 0.5 values expected for females and males, respectively) reduced the false assignment rate to 0 and 0.2% for the phenotypic males and females, respectively (Supplementary Material). Finally, replicability of the genetic sexing using the semi-quantitative approach was 100% for both phenotypic sexes.
Laboratory Study
Histological analysis showed that females started sex differentiation earlier than males (Table 2A). This was evident at timespoints 1, while from timespoints 2 on both sexes showed similar and high rates of sex differentiation that gradually increased to 100% at timespoints 4 (220 days post-hatching) (Figure 2). There was no significant sex difference in growth during this period of sex differentiation (Table 2A and Supplementary Figure 3). Despite some non-explained mortality in the laboratory population, sex ratio did not change over time (F = 1.7, d.f. = 1, p = 0.20).
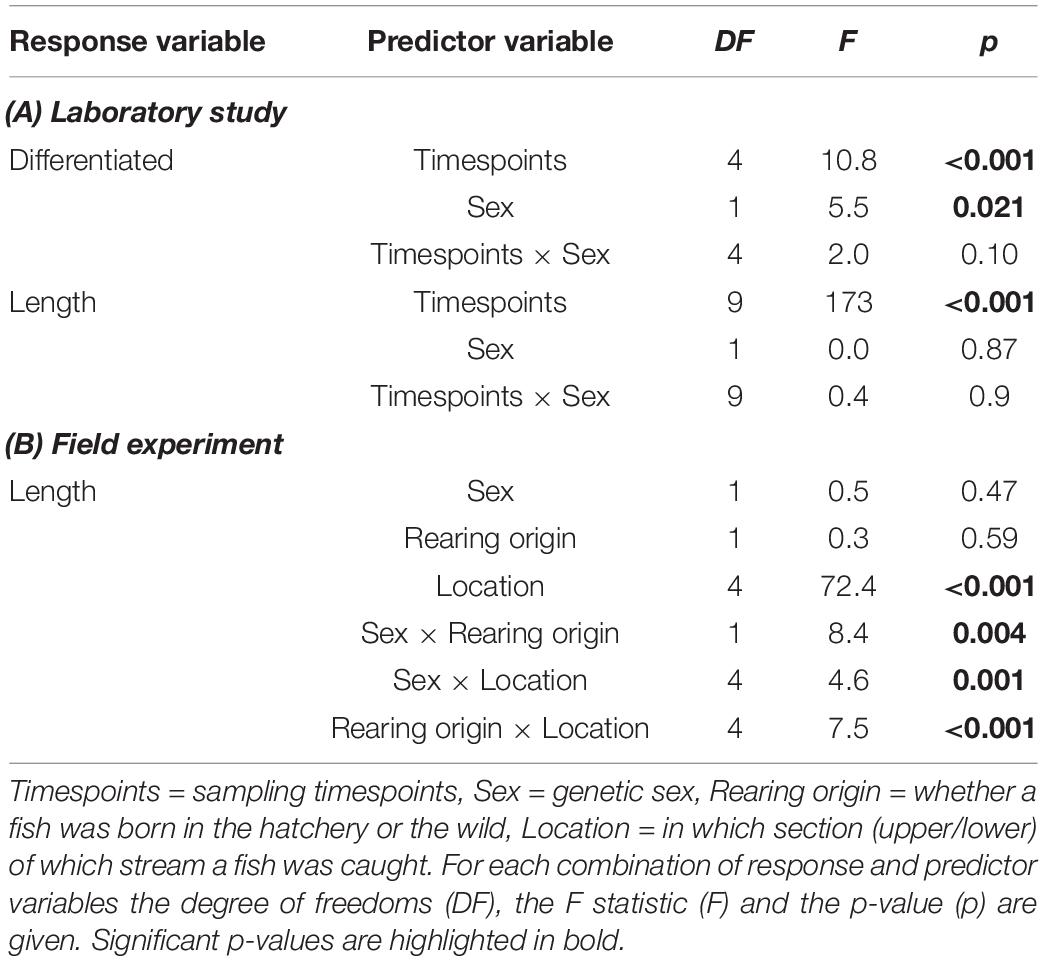
Table 2. Summary table of the statistical analyses for both the laboratory and field experiments, conducting analysis of variance on linear regression models.
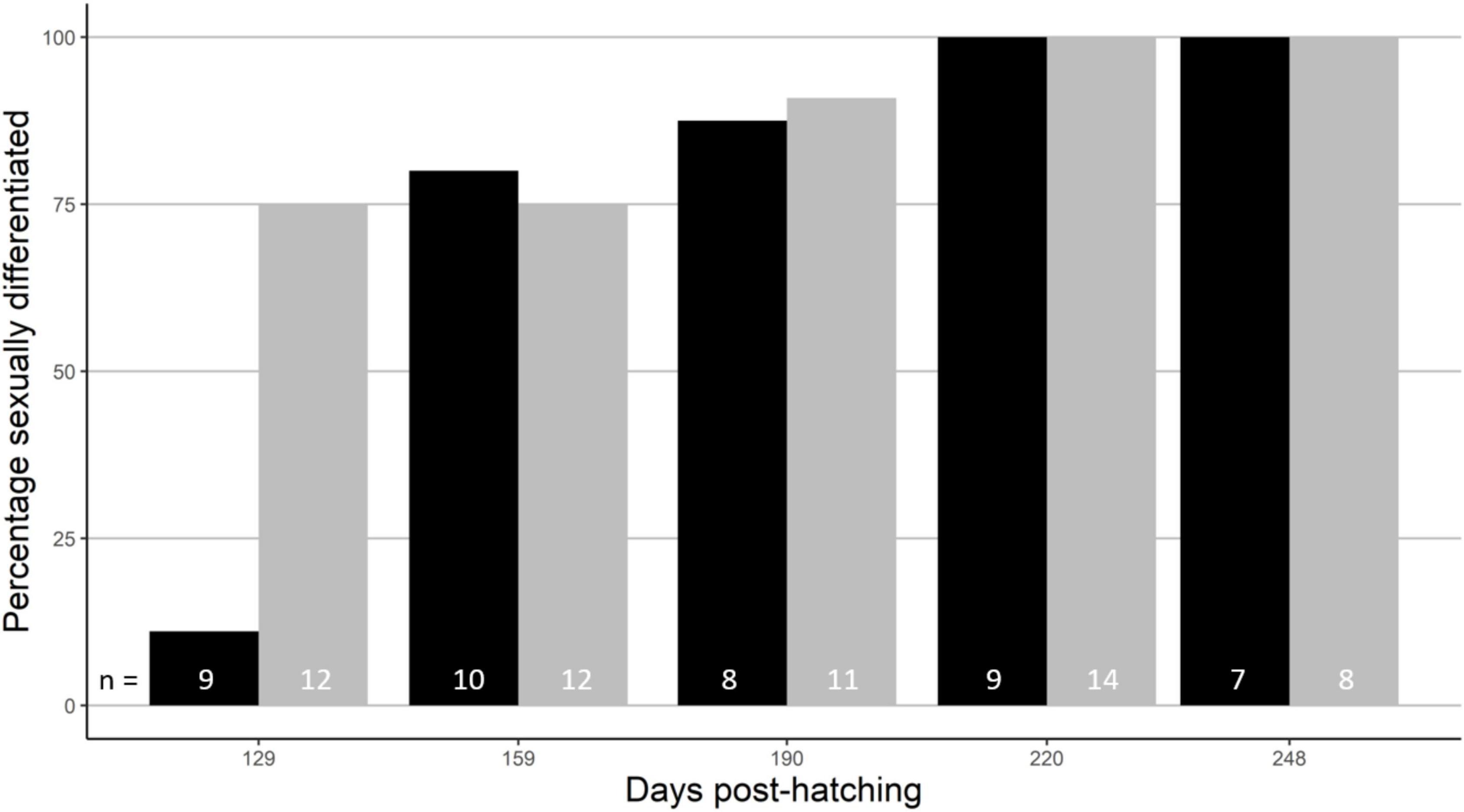
Figure 2. Timing of sex differentiation in males (black) and females (grey). The sample sizes are given at the bottom. See Table 2A for statistics.
Field Experiment
A total of 4,906 juvenile fish were sampled from the Schnittlauch, Schwarzbach, and Talbach streams. Of these 4,367 were assessed as being 0 + fish based on length thresholds as detailed above. A total of 762 0 + fish could be categorised as hatchery- or wild-born of which 748 fish could be genetically sexed (Table 1).
As expected, the samples differed in average length (see factor “location” in Table 2B that also includes effects of the timing of sampling). Overall, there seemed to be no effects of sex or rearing origin on growth (main effects in Table 2B). Importantly, however, the sexes grew differently depending on whether they were hatchery-born or wild born (interaction sex × rearing origin in Table 2B). Hatchery-born males were on average larger than hatchery-born females, while wild-born females were on average slightly larger than wild-born males (Figure 3A).
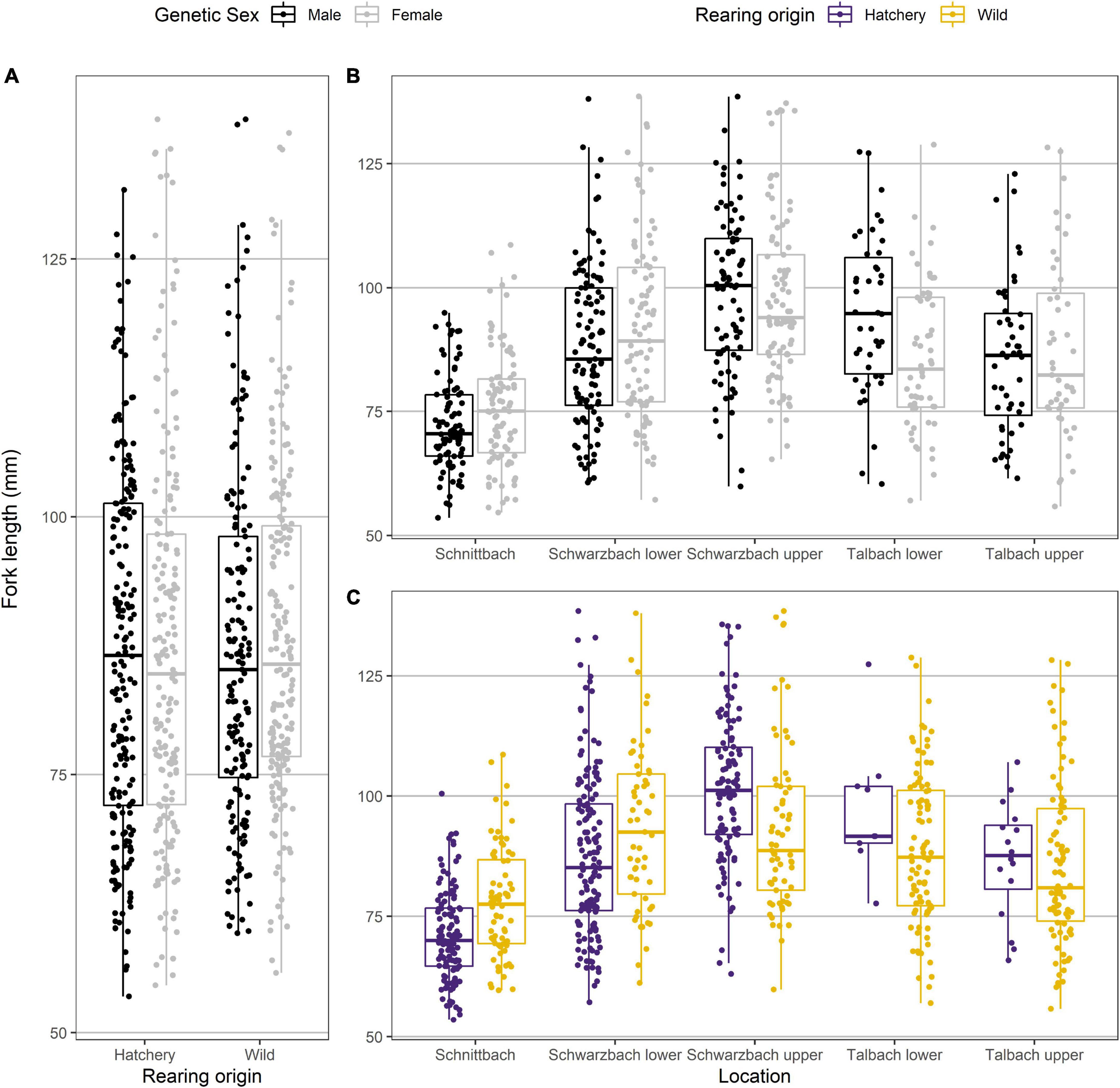
Figure 3. Boxplots showing the effects of the interactions between (A) genetic sex and rearing origin, (B) genetic sex and location, and (C) rearing origin and location on length in fish in the field experiment. See Table 2B for statistics.
Sexes also grew differently in the different locations (interaction sex × location in Table 2B). Females tended to be larger than males in the Schnittlauch sample, while males tended to the larger than females in the Schwarzbach and Talbach samples (Figure 3B). And while hatchery-born fish grew larger in the Talbach and upper Schwarzbach, wild-born ones were larger in the lower Schwarzbach and the Schnittlauch (Table 2B and Figure 3C). See Supplementary Material for the respective pairwise comparisons.
There seemed to be no sex-biassed mortality in the total studied area, in each stream, or in each location (no significant deviations from a 1:1 ratio). There was also no significant sex-biassed mortality in either hatchery- or wild-born fish. All relevant pairwise comparisons are given in the Supplementary Material.
Discussion
We modified an existing protocol to improve the accuracy of genetic sexing and used it to test for sex-specific life histories in early life stages of brown trout. We found that females start sex differentiation on average earlier than males, as previously observed in grayling, another salmonid (Maitre et al., 2017). However, the period of sex differences in differentiation seemed much shorter in brown trout than what was observed in grayling. Moreover, while Maitre et al. (2017) found that males grew larger than females during these juvenile stages, suggesting a trade-off between the investment into gonad formation and growth, we found no such sex-specific differences under laboratory conditions. This was different from what we observed in the field: Females were on average larger than males in two locations that were sampled first, while males were larger than females in the three locations that were sampled last. It remains to be shown whether these sex differences reveal effects of time or effects of environmental differences between the locations. The location-specific sex differences in growth seemed to not be dependent on whether fish were hatchery-born or wild-born (the corresponding three-way interaction was not significant). We conclude that there are sex-specific life histories in juvenile brown trout that affect the timing of sex differentiation and also growth.
The rate of hatchery-born fish varied between the different locations, ranging from 9.2 to 71.8%. These rates of success may reveal location-specific mortalities of hatchery-born fish, but if so, the mortalities did not seem to be sex-specific as sex ratios remained about equal in all locations (including the laboratory population). We found that hatchery-born 0 + trout were smaller than wild-born ones in the two locations that were sampled first, and tended to be larger than wild-born ones in the locations that were sampled later. Moreover, wild-born females grew on average slightly larger than wild-born males while in hatchery-born trout females grew less than males (even if Figure 3A suggests only a marginal effect, the model that takes all effects into account revealed a significant interaction between offspring sex and rearing origin (p = 0.004). We conclude that the origin of fish (hatchery or wild) affects growth and sex-specific life histories in brown trout. It is therefore likely that growth finally depends on the interplay between origin, sex, and location, and that we missed the corresponding three-way interaction due to a of lack of statistical power. The nature of potential environmental effects on sex-specific life histories will require data from more diverse sampling sites that differ, for example, in temperature, river slope, or riparian land use.
In salmonids, female length is tightly linked to fecundity (Lobon-Cervia et al., 1997; Einum and Fleming, 1999; Olsen and Vøllestad, 2003; Gregersen et al., 2009) and sometimes to offspring fitness (Hutchings, 1991; Ojanguren et al., 1996). Furthermore, early life female growth rates have been reported to influence reproduction in brown trout populations, with slower growing females generally delaying maturation and producing smaller clutches with overall larger eggs (Lobon-Cervia et al., 1997; Olsen and Vøllestad, 2003) that affect the life history of the offspring (Taborsky, 2006; Burton et al., 2013; Carim et al., 2017) and may eventually influence population viability (Carim et al., 2017). Our field experiment demonstrates that stocking can affect female growth relative to male growth. Reduced early growth rates in hatchery-born females may thus reveal different life history strategies compared to their wild conspecifics (e.g., mature later and produce smaller clutches with overall larger eggs). The overall effects of these observation on the viability of the studied brown trout population need to be better understood. We conclude that sex can be a factor that affects the impacts of restocking and stock-enhancement programmes (e.g., affects female life history and hence fecundity per age class).
Higher growth rates have often been reported in juvenile males relative to females of various salmonid fishes (Yamamoto, 2004; Mizzau et al., 2013; Maitre et al., 2017), and wild-born fish often differ in growth rates to hatchery-born fish (Einum and Fleming, 2001; Skov et al., 2012; Wilkins and Marsden, 2021). We found that such sex and origin effects are not a general rule but are context dependent in the case of brown trout. In our study, the context was defined by the location and the origin of fish. It is also possible that what we observed is partly the result of stocking-induced evolution, i.e., the long stock-enhancement history (several decades) of the study population could have caused evolutionary shifts in early growth rates.
Maitre et al. (2017) proposed that sex-specific life histories in early life-stage European grayling could be strong enough to result in sex-biassed mortality and may explain the highly male-biassed sex ratio seen in a pre-Alpine population of European grayling (Wedekind et al., 2013). The water temperature that juveniles are exposed to during their first summer best explained these unequal sex ratio (Wedekind et al., 2013). Because temperature effects on sex determination could be excluded (Pompini et al., 2013; Maitre et al., 2017), sex-specific sensitivity to temperature-related stress, and subsequent sex-biassed mortality, caused by sex-specific life history strategies has been proposed to be a likely cause for male-biassed sex ratios in this population and its subsequent decline. Similar drivers may potentially explain the comparable declines in other salmonid populations such as brown trout (Burkhardt-Holm et al., 2002). However, no sex-biassed mortality was observed in either the laboratory and or different streamlets we studied.
Restocking and stock enhancement is a taxonomically and geographically widespread. For conservation purposes, stocking is frequently done at larval or early juvenile stages (Halverson, 2008; Hunt and Jones, 2018), and a growing body of work provides evidence for sex-specific life histories in salmonid larvae and juveniles. We demonstrated that this is also true in the case in brown trout, with females starting sex differentiation earlier than males when raised under laboratory conditions. It is therefore possible that stocking affects sex-specific life histories in this species. We found that growth in the field was indeed sex-specific and depended on further factors that remain to be identified. One contributing factor that we identified here is whether fish were hatchery- or wild-born. We therefore conclude that stocking affects sex-specific life histories. This is a further aspect that needs to be considered when discussing the potential impacts of stocking on population viability and of hatchery-induced evolution.
Data Availability Statement
The data used in this study have been deposited on the Dryad repository: https://doi.org/10.5061/dryad.7h44j0zwj and https://doi.org/10.5061/dryad.pzgmsbcpj.
Ethics Statement
The animal study was reviewed and approved by the Veterinary Office of the Bern Canton and Veterinary Office of the Vaud Canton.
Author Contributions
LM, DN, IC, AU, and CW managed the breeding, sampling, and data extraction for the field experiment. KM developed the genotyping protocol. LM and JB managed the genetic sexing and parental assignments. HP, LM, DN, and CW bred the fish for the laboratory study, which were then raised and sampled by HP and VA. VA conducted the histology for phenotypic sexing, supervised by SK. HP, JB, and CW analysed the data and wrote the manuscript with input from all authors. All authors contributed to the article and approved the submitted version.
Funding
The project was funded by grants from the Swiss Federal Office for the Environment and the Swiss National Science Foundation (31003A_159579 and 31003A_182265).
Conflict of Interest
The authors declare that the research was conducted in the absence of any commercial or financial relationships that could be construed as a potential conflict of interest.
Publisher’s Note
All claims expressed in this article are solely those of the authors and do not necessarily represent those of their affiliated organizations, or those of the publisher, the editors and the reviewers. Any product that may be evaluated in this article, or claim that may be made by its manufacturer, is not guaranteed or endorsed by the publisher.
Acknowledgments
We thank U. Gutmann and B. Bracher (Fischereistützpunkt in Reutigen) who collected the fish from the wild, assisted in the experimental breeding, raised the fish in the hatchery, and stocked them into the wild. We also thank L. Cornetti, O. Darbellay, J. Ehinger, L. Garaud, F. Glauser, E. Longange, D. Maitre, S. Mandra, E. Pereira-Alvarez, L. Wilkins, and D. Zeugin, and the members of the fishery associations FV Aaretal, FV Rotache, FV Saane/Sense and FI Bern for their assistance in the field experiments, T. Bösch, J. Kast, and the ecogenics team for genotyping the fish, and T. Braunbeck, A. Hertig, C. Küng, and T. Vuille for support and discussion.
Supplementary Material
The Supplementary Material for this article can be found online at: https://www.frontiersin.org/articles/10.3389/fevo.2022.869925/full#supplementary-material
References
Aas, O., Cucherousset, J., Fleming, I. A., Wolter, C., Hojesjo, J., Buoro, M., et al. (2018). Salmonid stocking in five North Atlantic jurisdictions: Identifying drivers and barriers to policy change. Aquat. Conserv. Mar. Freshw. Ecosyst. 28, 1451–1464. doi: 10.1002/AQC.2984
Aescht, E., Büchl-Zimmermann, S., Burmester, A., Dänhardt-Pfeiffer, S., Desel, C., Hamers, C., et al. (2010). Romeis Mikroskopische Technik, 18th Edn. Heidelberg: Spektrum Akademischer Verlag, doi: 10.1007/978-3-8274-2254-5
Altwegg, R., Schaub, M., and Roulin, A. (2007). Age-specific fitness components and their temporal variation in the barn owl. Am. Nat. 169, 47–61. doi: 10.1086/510215
Araki, H., Berejikian, B. A., Ford, M. J., and Blouin, M. S. (2008). Fitness of hatchery-reared salmonids in the wild. Evol. Appl. 1, 342–355. doi: 10.1111/j.1752-4571.2008.00026.x
Ayllon, F., Solberg, M. F., Besnier, F., Fjelldal, P. G., Hansen, T. J., Wargelius, A., et al. (2020). Autosomal sdY pseudogenes explain discordances between phenotypic sex and DNA marker for sexiIdentification in Atlantic salmon. Front. Genet. 11:544207. doi: 10.3389/fgene.2020.544207
Badyaev, A. V., Hill, G. E., Beck, M. L., Dervan, A. A., Duckworth, R. A., McGraw, K. J., et al. (2002). Sex-biased hatching order and adaptive population divergence in a passerine bird. Science 295, 316–318. doi: 10.1126/science.1066651
Bertho, S., Herpin, A., Jouanno, E., Yano, A., Bobe, J., Parrinello, H., et al. (2021). A non-functional copy of the salmonid sex determining gene (sdY) is responsible for the “apparent” XY females in Chinook salmon, Oncorhynchus tshawytscha. bioRxiv 2021:454148. doi: 10.1101/2021.07.28.454148
Birnie-Gauvin, K., Bordeleau, X., Cooke, S. J., Davidsen, J. G., Eldøy, S. H., Eliason, E. J., et al. (2021). Life-history strategies in salmonids: the role of physiology and its consequences. Biol. Rev. 96, 2304–2320. doi: 10.1111/BRV.12753
Bolund, E., Bouwhuis, S., Pettay, J. E., and Lummaa, V. (2013). Divergent selection on, but no genetic conflict over, female and male timing and rate of reproduction in a human population. Proc. R. Soc. B Biol. Sci. 280:2002. doi: 10.1098/rspb.2013.2002
Brooks, R. C., and Garratt, M. G. (2017). Life history evolution, reproduction, and the origins of sex-dependent aging and longevity. Ann. N. Y. Acad. Sci. 1389, 92–107. doi: 10.1111/NYAS.13302
Burkhardt-Holm, P., Peter, A., and Segner, H. (2002). Decline of fish catch in switzerland: Project fishnet: a balance between analysis and synthesis. Aquat. Sci. 64, 36–54. doi: 10.1007/s00027-002-8053-1
Burton, T., Mckelvey, S., Stewart, D. C., Armstrong, J. D., and Metcalfe, N. B. (2013). Early maternal experience shapes offspring performance in the wild. Ecology 94, 618–626. doi: 10.1890/12-0462.1
Cally, J. G., Stuart-Fox, D., and Holman, L. (2019). Meta-analytic evidence that sexual selection improves population fitness. Nat. Commun. 2019 101, 1–10. doi: 10.1038/s41467-019-10074-7
Carim, K. J., Vindenes, Y., Eby, L. A., Barfoot, C., and Vøllestad, L. A. (2017). Life history, population viability, and the potential for local adaptation in isolated trout populations. Glob. Ecol. Conserv. 10, 93–102. doi: 10.1016/J.GECCO.2017.02.001
Cucherousset, J., Lassus, R., Riepe, C., Millet, P., Santoul, F., Arlinghaus, R., et al. (2021). Quantitative estimates of freshwater fish stocking practices by recreational angling clubs in France. Fish. Manag. Ecol. 28, 295–304. doi: 10.1111/FME.12471
Dieterman, D. J., Hoxmeier, R. J. H., Roloff, J., and Staples, D. F. (2020). Use of long-term (40+ year) trend data to evaluate management actions on brown trout, Salmo trutta, populations in groundwater-fed streams. Fish. Manag. Ecol. 27, 551–566. doi: 10.1111/FME.12431
Dietrich, V. C. J., Schmoll, T., Winkel, W., and Lubjuhn, T. (2003). Survival to first breeding is not sex-specific in the Coal Tit (Parus ater). J. Ornithol. 144, 148–156. doi: 10.1007/bf02465643
Donald, P. F. (2007). Adult sex ratios in wild bird populations. Ibis 149, 671–692. doi: 10.1111/J.1474-919X.2007.00724.X
Dziewulska, K., and Domagala, J. (2004). Testicular development in the sea trout (Salmo trutta morpha trutta L.) after sex differentiation, with a reference to precocious maturation. J. Appl. Ichthyol. 20, 282–289. doi: 10.1111/j.1439-0426.2004.00553.x
Eberhart-Phillips, L. J., Küpper, C., Carmona-Isunza, M. C., Vincze, O., Zefania, S., Cruz-López, M., et al. (2018). Demographic causes of adult sex ratio variation and their consequences for parental cooperation. Nat. Commun. 9, 1–8. doi: 10.1038/s41467-018-03833-5
Einum, S., and Fleming, I. A. (1999). Maternal effects of egg size in brown trout (Salmo trutta): Norms of reaction to environmental quality. Proc. R. Soc. B Biol. Sci. 266, 2095–2100. doi: 10.1098/rspb.1999.0893
Einum, S., and Fleming, I. A. (2001). Implications of Stocking: ecological interactions between wild and released salmonids. Nord. J. Freshw. Res. 75, 56–70.
Eldøy, S. H., Bordeleau, X., Lawrence, M. J., Thorstad, E. B., Finstad, A. G., Whoriskey, F. G., et al. (2021). The effects of nutritional state, sex and body size on the marine migration behaviour of sea trout. Mar. Ecol. Prog. Ser. 665, 185–200. doi: 10.3354/MEPS13670
Elliott, J. M. (1989). Wild brown trout Salmo trutta: an important national and international resource. Freshw. Biol. 21, 1–5. doi: 10.1111/j.1365-2427.1989.tb01343.x
Forsman, A. (2018). On the role of sex differences for evolution in heterogeneous and changing fitness landscapes: insights from pygmy grasshoppers. Philos. Trans. R. Soc. B Biol. Sci. 373:429. doi: 10.1098/RSTB.2017.0429
Fox, J., and Weisberg, S. (2019). An R Companion to Applied Regression. Third Edition. Thousand Oaks, CA: Sage.
Geffroy, B., and Wedekind, C. (2020). Effects of global warming on sex ratios in fishes. J. Fish Biol. 97, 596–606. doi: 10.1111/JFB.14429
Gomes, B. V., Guimarães, D. M., Szczupak, D., and Neves, K. (2018). Female dispersion and sex ratios interact in the evolution of mating behavior: A computational model. Sci. Rep. 8, 1–8. doi: 10.1038/s41598-018-20790-7
Gregersen, F., Vøllestad, L. A., Olsen, E. M., and Haugen, T. O. (2009). Sibling-size variation in brown trout Salmo trutta in relation to egg size and stream size. J. Fish Biol. 74, 1259–1268. doi: 10.1111/J.1095-8649.2009.02194.X
Grilo, T. F., Lopes, A. R., Sampaio, E., Rosa, R., and Cardoso, P. G. (2018). Sex differences in oxidative stress responses of tropical topshells (Trochus histrio) to increased temperature and high pCO2. Mar. Pollut. Bull. 131, 252–259. doi: 10.1016/J.MARPOLBUL.2018.04.031
Gurley, B., Finger, J. W., and Wada, H. (2018). Sex-specific effects of incubation temperature on embryonic development of zebra finch (Taeniopygia guttata) Embryos. Physiol. Biochem. Zool. 91, 1036–1045. doi: 10.1086/699741
Halverson, M. A. (2008). Stocking Trends: A quantitative review of governmental fish stocking in the United States, 1931 to 2004. Fisheries 33, 69–75. doi: 10.1577/1548-8446-33.2.69
Hasegawa, K. (2020). Invasions of rainbow trout and brown trout in Japan: A comparison of invasiveness and impact on native species. Ecol. Freshw. Fish 29, 419–428. doi: 10.1111/EFF.12534
Hendry, A. P., Schoen, D. J., Wolak, M. E., and Reid, J. M. (2018). The Contemporary Evolution of Fitness. Annu. Rev. Ecol. Evol. Syst. 49, 457–476. doi: 10.1146/ANNUREV-ECOLSYS-110617-062358
Huertas, M., and Cerdà, J. (2006). Stocking density at early developmental stages affects growth and sex ratio in the European eel (Anguilla anguilla). Biol. Bull. 211, 286–296. doi: 10.2307/4134550
Hunt, T. L., and Jones, P. (2018). Informing the Great Fish Stocking Debate: An Australian Case Study. Rev. Fish. Sci. Aquac. 26, 275–308. doi: 10.1080/23308249.2017.1407916
Husby, A., Saether, B.-E., Jensen, H., and Ringsby, T. H. (2006). Causes and consequences of adaptive seasonal sex ratio variation in house sparrows. J. Anim. Ecol. 75, 1128–1139. doi: 10.1111/j.1365-2656.2006.01132.x
Hutchings, J. A. (1991). Fitness consequences of variation in egg size and food abundance in brook trout Salvelinus fontinalis. Evolution 45, 1162–1168. doi: 10.1111/j.1558-5646.1991.tb04382.x
Kelson, S. J., Miller, M. R., Thompson, T. Q., O’rourke, S. M., and Carlson, S. M. (2019). Do genomics and sex predict migration in a partially migratory salmonid fish, Oncorhynchus mykiss? Can. J. Fish. Aquat. Sci. 76, 2080–2088. doi: 10.1139/CJFAS-2018-0394/SUPPL_FILE/CJFAS-2018-0394SUPPLA.DOCX
Klemetsen, A., Amundsen, P. A., Dempson, J. B., Jonsson, B., Jonsson, N., O’Connell, M. F., et al. (2003). Atlantic salmon Salmo salar L., brown trout Salmo trutta L. and Arctic charr Salvelinus alpinus (L.): A review of aspects of their life histories. Ecol. Freshw. Fish 12, 1–59. doi: 10.1034/j.1600-0633.2003.00010.x
Klütsch, C. F. C., Maduna, S. N., Polikarpova, N., Forfang, K., Aspholm, P. E., Nyman, T., et al. (2019). Genetic changes caused by restocking and hydroelectric dams in demographically bottlenecked brown trout in a transnational subarctic riverine system. Ecol. Evol. 9, 6068–6081. doi: 10.1002/ece3.5191
Leitwein, M., Gagnaire, P. A., Desmarais, E., Berrebi, P., and Guinand, B. (2018). Genomic consequences of a recent three-way admixture in supplemented wild brown trout populations revealed by local ancestry tracts. Mol. Ecol. 27, 3466–3483. doi: 10.1111/mec.14816
Lenz, T. L., Jacob, A., and Wedekind, C. (2007). Manipulating sex ratio to increase population growth: the example of the Lesser Kestrel. Anim. Conserv. 10, 236–244. doi: 10.1111/J.1469-1795.2007.00099.X
Li, X. Y., and Kokko, H. (2019). Sex-biased dispersal: a review of the theory. Biol. Rev. 94, 721–736. doi: 10.1111/BRV.12475
Liu, Y., Bailey, J. L., and Davidsen, J. G. (2019). Social-cultural ecosystem services of sea trout recreational fishing in Norway. Front. Mar. Sci. 6:178. doi: 10.3389/FMARS.2019.00178/BIBTEX
Lobon-Cervia, J., Utrilla, C. G., Rincón, P. A., and Amezcua, F. (1997). Environmentally induced spatio-temporal variations in the fecundity of brown trout Salmo trutta L.: Trade-offs between egg size and number. Freshw. Biol. 38, 277–288. doi: 10.1046/j.1365-2427.1997.00217.x
Long, J. S., and Ervin, L. H. (2000). Using heteroscedasticity consistent standard errors in the linear regression model. Am. Stat. 54, 217–224. doi: 10.1080/00031305.2000.10474549
Louison, M. J., and Stelzer, R. S. (2016). Use of first-order tributaries by brown trout (Salmo trutta) as nursery habitat in a cold water stream network. Ecol. Freshw. Fish 25, 133–140. doi: 10.1111/EFF.12197
Magerhans, A., Müller-Belecke, A., and Hörstgen-Schwark, G. (2009). Effect of rearing temperatures post hatching on sex ratios of rainbow trout (Oncorhynchus mykiss) populations. Aquaculture 294, 25–29. doi: 10.1016/j.aquaculture.2009.05.001
Magurran, A. E., and Garcia, C. M. (2000). Sex differences in behaviour as an indirect consequence of mating system. J. Fish Biol. 57, 839–857. doi: 10.1111/J.1095-8649.2000.TB02196.X
Maitre, D., Selmoni, O. M., Uppal, A., Marques, Da Cunha, L., Wilkins, L. G. E., et al. (2017). Sex differentiation in grayling (Salmonidae) goes through an all-male stage and is delayed in genetic males who instead grow faster. Sci. Rep. 7, 1–11. doi: 10.1038/s41598-017-14905-9
Marques da Cunha, L., Uppal, A., Seddon, E., Nusbaumer, D., Vermeirssen, E. L. M., et al. (2019). No additive genetic variance for tolerance to ethynylestradiol exposure in natural populations of brown trout (Salmo trutta). Evol. Appl. 12, 940–950. doi: 10.1111/eva.12767
Mazur, C. F., and Iwama, G. K. (1993). Handling and crowding stress reduces number of plaque-forming cells in Atlantic salmon. J. Aquat. Anim. Health 5, 98–101. doi: 10.1577/1548-86671993005<0098:HACSRN<2.3.CO;2
McKinney, G. J., Seeb, J. E., Pascal, C. E., Schindler, D. E., Gilk-Baumer, S. E., and Seeb, L. W. (2020). Y-chromosome haplotypes are associated with variation in size and age at maturity in male Chinook salmon. Evol. Appl. 13, 2791–2806. doi: 10.1111/EVA.13084
Meier, K., Hansen, M. M., Bekkevold, D., and Skaala Mensberg, K. L. D. (2011). An assessment of the spatial scale of local adaptation in brown trout (Salmo trutta L.): footprints of selection at microsatellite DNA loci. Heredity 106, 488–499. doi: 10.1038/hdy.2010.164
Millington, J. W., and Rideout, E. J. (2018). Sex differences in Drosophila development and physiology. Curr. Opin. Physiol. 6, 46–56. doi: 10.1016/J.COPHYS.2018.04.002
Mizzau, T. W., Garner, S. R., Marklevitz, S. A. C., Thompson, G. J., and Morbey, Y. E. (2013). A genetic test of sexual size dimorphism in pre-emergent chinook salmon. PLoS One 8:e78421. doi: 10.1371/JOURNAL.PONE.0078421
Mobley, K. B., Aykanat, T., Czorlich, Y., House, A., Kurko, J., Miettinen, A., et al. (2021). Maturation in Atlantic salmon (Salmo salar, Salmonidae): a synthesis of ecological, genetic, and molecular processes. Rev. Fish Biol. Fish. 313, 523–571. doi: 10.1007/S11160-021-09656-W
Morán, P., Labbé, L., and Garcia de Leaniz, C. (2016). The male handicap: male-biased mortality explains skewed sex ratios in brown trout embryos. Biol. Lett. 12:20160693. doi: 10.1098/rsbl.2016.0693
Mousavi, S. E., Purser, G. J., and Patil, J. G. (2021). Embryonic Onset of Sexually Dimorphic Heart Rates in the Viviparous Fish. Gambusia holbrooki. Biomed. 165:165. doi: 10.3390/BIOMEDICINES9020165
Nevoux, M., Finstad, B., Davidsen, J. G., Finlay, R., Josset, Q., Poole, R., et al. (2019). Environmental influences on life history strategies in partially anadromous brown trout (Salmo trutta. Salmonidae). Fish Fish. 20, 1051–1082. doi: 10.1111/faf.12396
Nislow, K. H., and Armstrong, J. D. (2012). Towards a life-history-based management framework for the effects of flow on juvenile salmonids in streams and rivers. Fish. Manag. Ecol. 19, 451–463. doi: 10.1111/J.1365-2400.2011.00810.X
Nusbaumer, D., Garaud, L., Ançay, L., and Wedekind, C. (2021). Sex-specific stress tolerance in embryos of lake char (Salvelinus umbla). Front. Ecol. Evol. 9:768263. doi: 10.3389/FEVO.2021.768263/PDF
Ojanguren, A. F., Reyes-Gavilán, F. G., and Braña, F. (1996). Effects of egg size on offspring development and fitness in brown trout. Salmo trutta L. Aquaculture 147, 9–20. doi: 10.1016/S0044-8486(96)01398-1
Olsen, E. M., and Vøllestad, L. A. (2003). Microgeographical variation in brown trout reproductive traits: Possible effects of biotic interactions. Oikos 100, 483–492. doi: 10.1034/j.1600-0706.2003.11900.x
Pearse, D. E., Barson, N. J., Nome, T., Gao, G., Campbell, M. A., Abadía-Cardoso, A., et al. (2019). Sex-dependent dominance maintains migration supergene in rainbow trout. Nat. Ecol. Evol. 312, 1731–1742. doi: 10.1038/s41559-019-1044-6
Pickering, A. D., and Pottinger, T. G. (1987). Crowding causes prolonged leucopenia in salmonid fish, despite interrenal acclimation. J. Fish Biol. 30, 701–712. doi: 10.1111/j.1095-8649.1987.tb05799.x
Pinter, K., Weiss, S., Lautsch, E., and Unfer, G. (2018). Survival and growth of hatchery and wild brown trout (Salmo trutta) parr in three Austrian headwater streams. Ecol. Freshw. Fish 27, 146–157. doi: 10.1111/eff.12332
Pompini, M., Buser, A. M., Thali, M. R., Von Siebenthal, B. A., Nusslé, S., Guduff, S., et al. (2013). Temperature-induced sex reversal is not responsible for sex-ratio distortions in grayling Thymallus thymallus or brown trout Salmo trutta. J. Fish Biol. 83, 404–411. doi: 10.1111/JFB.12174
Quéméré, E., Perrier, C., Besnard, A. L., Evanno, G., Baglinière, J. L., Guiguen, Y., et al. (2014). An improved PCR-based method for faster sex determination in brown trout (Salmo trutta) and Atlantic salmon (Salmo salar). Conserv. Genet. Resour. 6, 825–827. doi: 10.1007/s12686-014-0259-8
R Development Core Team (2010). R : A Language and Environment for Statistical Computing. Vienna: R Found. Stat. Comput.
Régnier, T., Labonne, J., Chat, J., Yano, A., Guiguen, Y., and Bolliet, V. (2015). No early gender effects on energetic status and life history in a salmonid. R. Soc. Open Sci. 2:150441. doi: 10.1098/rsos.150441
Rgen, J., Johnsson, I., Sernland, E., Blixt, J. M., Sernland, J. I, and Blixt, E. (2001). Sex-specific aggression and antipredator behaviour in young brown trout. Ethology 107, 587–599. doi: 10.1046/J.1439-0310.2001.00682.X
Schroeder, J., Cleasby, I., Dugdale, H. L., Nakagawa, S., and Burke, T. (2013). Social and genetic benefits of parental investment suggest sex differences in selection pressures. J. Avian Biol. 44, 133–140. doi: 10.1111/j.1600-048X.2012.00010.x
Selmoni, O. M., Maitre, D., Roux, J., Wilkins, L. G. E., Marques, da Cunha, L., et al. (2019). Sex-specific changes in gene expression in response to estrogen pollution around the onset of sex differentiation in grayling (Salmonidae). BMC Genomics 201, 1–12. doi: 10.1186/S12864-019-5955-Z
Skov, C., Koed, A., Baastrup-Spohr, L., and Arlinghaus, R. (2012). Dispersal, growth, and diet of stocked and wild orthern pike fry in a shallow natural lake, with implications for the management of stocking programs. Chang. Publ. 31, 1177–1186. doi: 10.1080/02755947.2011.646452
Stelkens, R. B., Jaffuel, G., Escher, M., and Wedekind, C. (2012). Genetic and phenotypic population divergence on a microgeographic scale in brown trout. Mol. Ecol. 21, 2896–2915. doi: 10.1111/j.1365-294X.2012.05581.x
Svobodová, J., Pinkasová, H., Hyršl, P., Dvořáčková, M., Zita, L., and Kreisinger, J. (2020). Differences in the growth rate and immune strategies of farmed and wild mallard populations. PLoS One 15:e0236583. doi: 10.1371/journal.pone.0236583
Taborsky, B. (2006). Mothers determine offspring size in response to own juvenile growth conditions. Biol. Lett. 2, 225–228. doi: 10.1098/rsbl.2005.0422
Tarka, M., Guenther, A., Niemelä, P. T., Nakagawa, S., and Noble, D. W. A. (2018). Sex differences in life history, behavior, and physiology along a slow-fast continuum: a meta-analysis. Behav. Ecol. Sociobiol. 72, 1–13. doi: 10.1007/s00265-018-2534-2
Tréhin, C., Rivot, E., Lamireau, L., Meslier, L., Besnard, A. L., Gregory, S. D., et al. (2021). Growth during the first summer at sea modulates sex-specific maturation schedule in Atlantic salmon. Can. J. Fish. Aquat. Sci. 78, 659–669. doi: 10.1139/cjfas-2020-0236
Tsai, H. W., Grant, P. A., and Rissman, E. F. (2009). Sex differences in histone modifications in the neonatal mouse brain. Epigenetics 4, 47–53. doi: 10.4161/EPI.4.1.7288
Tsuboi, J. I., Iwata, T., Morita, K., Endou, S., Oohama, H., and Kaji, K. (2013). Strategies for the conservation and management of isolated salmonid populations: lessons from Japanese streams. Freshw. Biol. 58, 908–917. doi: 10.1111/FWB.12096
Ueda, R., Takeshima, H., and Sato, T. (2021). Difficulty in sex identification of two local populations of red-spotted masu salmon using two salmonid male-specific molecular markers. Ichthyol. Res. 1, 1–5. doi: 10.1007/S10228-021-00837-Y/TABLES/1
Weber, G. M., Leeds, T. D., and Schneider, R. P. (2020). Sex reversal of female rainbow trout by immersion in 17α-methyltestosterone. Aquaculture 528:735535. doi: 10.1016/J.AQUACULTURE.2020.735535
Wedekind, C., Evanno, G., Székely, T., Pompini, M., Darbellay, O., and Guthruf, J. (2013). Persistent unequal sex ratio in a population of grayling (Salmonidae) and possible role of temperature Increase. Conserv. Biol. 27, 229–234. doi: 10.1111/j.1523-1739.2012.01909.x
Wiklund, C., Gotthard, K., and Nylin, S. (2003). Mating system and the evolution of sex-specific mortality rates in two nymphalid butterflies. Proc. R. Soc. London. Ser. B Biol. Sci. 270, 1823–1828. doi: 10.1098/rspb.2003.2437
Wilkins, P. D., and Marsden, J. E. (2021). Spatial and seasonal comparisons of growth of wild and stocked juvenile lake trout in Lake Champlain. J. Great Lakes Res. 47, 204–212. doi: 10.1016/J.JGLR.2020.11.007
Yamamoto, T. (2004). Sex-specific growth pattern during early life history in masu salmon, Oncorhynchus masou. Ecol. Freshw. Fish 13, 203–207. doi: 10.1111/j.1600-0633.2004.00051.x
Yano, A., Guyomard, R., Nicol, B., Jouanno, E., Quillet, E., Klopp, C., et al. (2012). An immune-related gene evolved into the master sex-determining gene in rainbow trout, Oncorhynchus mykiss. Curr. Biol. 22, 1423–1428.
Yano, A., Nicol, B., Jouanno, E., and Guiguen, Y. (2014). Heritable targeted inactivation of the rainbow trout (Oncorhynchus mykiss) master sex-determining gene using zinc-finger nucleases. Mar. Biotechnol. 16, 243–250. doi: 10.1007/S10126-013-9546-8/FIGURES/4
Yano, A., Nicol, B., Jouanno, E., Quillet, E., Fostier, A., Guyomard, R., et al. (2013). The sexually dimorphic on the Y-chromosome gene (sdY) is a conserved male-specific Y-chromosome sequence in many salmonids. Evol. Appl. 6, 486–496. doi: 10.1111/eva.12032
Keywords: sex, life history, genetic sexing, Salmo trutta, growth, survival, stock-enhancement, anthropogenic effects
Citation: Palejowski H, Bylemans J, Ammann V, Marques da Cunha L, Nusbaumer D, Castro I, Uppal A, Mobley KB, Knörr S and Wedekind C (2022) Sex-Specific Life History Affected by Stocking in Juvenile Brown Trout. Front. Ecol. Evol. 10:869925. doi: 10.3389/fevo.2022.869925
Received: 05 February 2022; Accepted: 22 March 2022;
Published: 02 May 2022.
Edited by:
Amanda Wilson Carter, The University of Tennessee, Knoxville, United StatesReviewed by:
Ondřej Slavík, Czech University of Life Sciences Prague, CzechiaKurt Pinter, University of Natural Resources and Life Sciences Vienna, Austria
Copyright © 2022 Palejowski, Bylemans, Ammann, Marques da Cunha, Nusbaumer, Castro, Uppal, Mobley, Knörr and Wedekind. This is an open-access article distributed under the terms of the Creative Commons Attribution License (CC BY). The use, distribution or reproduction in other forums is permitted, provided the original author(s) and the copyright owner(s) are credited and that the original publication in this journal is cited, in accordance with accepted academic practice. No use, distribution or reproduction is permitted which does not comply with these terms.
*Correspondence: Claus Wedekind, Y2xhdXMud2VkZWtpbmRAdW5pbC5jaA==
†ORCID: Claus Wedekind, orcid.org/0000-0001-6143-4716; Kenyon B. Mobley, orcid.org/0000-0003-2843-6407
‡These authors have contributed equally to this work and share first authorship
§Present address: Kenyon B. Mobley, Norwegian College of Fishery Science, UiT The Arctic University of Norway, Tromsø, Norway