- Department of Biology, University of South Dakota, Vermillion, SD, United States
Seasonal changes, such as alterations in food availability or type and cold conditions, present challenges to free-living birds living in highly seasonal climates. Small birds respond to such challenges through seasonal metabolic flexibility, which better matches seasonal metabolic phenotypes to environmental conditions and can improve fitness. To better understand the mechanistic basis of this metabolic flexibility, we conducted a large-scale metabolic profiling of pectoralis muscle in black-capped chickadees (Poecile atricapillus) and American goldfinches (Spinus tristis), which are small, year-round bird species of temperate-zones. We analyzed muscle samples using non-biased, global metabolomics profiling technology based on UHLC/MS/MS2 platforms. A total of 582 metabolites was characterized for summer and winter season samples. Chickadees showed greater seasonal separation of global metabolite profiles than goldfinches, which is consistent with previous transcriptomic studies of pectoralis muscle in these two species. Reduced levels of amino acids during winter occurred in both species and might reflect decreasing dietary protein intake, amino acid shuttling to other pathways for thermogenesis and/or elevated rates of protein turnover in the pectoralis muscle. Concomitant decreased abundances in tricarboxylic acid cycle (TCA) metabolites suggest faster cycling of the oxidative phosphorylation pathway in winter to meet the metabolic demands of thermogenesis. Accordingly, chickadees displayed shifts toward lipid oxidation in winter, whereas goldfinches showed winter declines in ketone bodies, which suggests increased energy demand or subtle changes in substrate availability. Beyond the winter-specific changes in metabolite abundances, integration of the metabolomic and the transcriptomic data revealed a landscape of gene–metabolite associations related to the winter-adaptive metabolic response. This landscape of gene–metabolite pairs was overrepresented by pathways associated with transport of small molecules, metabolism of amino acids and derivatives, activation and biosynthesis of fatty acid derivatives, and biosynthesis and metabolism of nicotinate and nicotinamide derivatives. Collectively, our results suggest that increased levels of NADH and its derivatives in the pectoralis muscle are a potential novel mechanism for increasing winter metabolic output, fueled by lipids, for thermogenesis during winter.
Introduction
Phenotypically flexible responses to season are ubiquitous among birds inhabiting seasonally variable climates, with those that live in cold winter climates adopting a strategy of metabolic upregulation and increased energy consumption in winter (Marsh and Dawson, 1989; Swanson, 2010). Cold winter climates typically result in upregulation of both basal (BMR) and summit (peak cold induced metabolic rates, Msum) metabolic rates, although seasonal responses are more variable for birds in milder winter climates (McKechnie, 2008; Wells and Schaeffer, 2012; McKechnie et al., 2015). This seasonal metabolic flexibility allows birds to better match their metabolic phenotypes to prevailing environmental conditions (e.g., Vézina et al., 2020; Le Pogam et al., 2021). Because elevated Msum in birds is associated with enhanced cold tolerance (Swanson, 2001; Swanson and Liknes, 2006), it represents a functional link between metabolic rates and performance that could influence survival over winter. Indeed, such matching of metabolic and cold tolerance phenotypes to seasonal conditions can have fitness consequences (Nilsson and Nilsson, 2016; Petit et al., 2017; Latimer et al., 2018).
The physiological, biochemical, and molecular mechanisms underlying metabolic flexibility in birds are incompletely understood (Cheviron and Swanson, 2017; Stager et al., 2021). Different bird species can employ or emphasize different mechanisms for generating elevated metabolic rates in cold winter climates (Liknes and Swanson, 2011a,b; Petit and Vézina, 2014; Petit et al., 2014; Dubois et al., 2016). In addition, within year-round temperate-zone resident populations, birds may show different metabolic and mechanistic responses to temperature variation across seasons (Swanson et al., 2020). Common pathways contributing to metabolic upregulation include muscle and digestive system growth, fat catabolism and transport capacities, cellular metabolic intensity, and oxygen transport capacity. However, seasonal adjustments to specific regulatory steps in each of these pathways is far from uniform among birds in cold winter climates (Swanson, 2010).
Because Msum in birds is primarily a function of shivering in skeletal muscle, especially pectoralis muscle (Hohtola, 1982; Swanson et al., 2013; Petit and Vézina, 2014), flexibility in organismal Msum may result from changes in muscle mass or cellular metabolic intensity (Swanson and Vézina, 2015). Winter increments of Msum in birds are consistently supported by greater pectoralis muscle mass (Swanson and Vézina, 2015, but see Swanson et al., 2014; Milbergue et al., 2019) but less consistently by elevated cellular metabolic intensity [see Swanson (2010) for review; but also Liknes and Swanson (2011b), Peña-Villalobos et al. (2014), Zheng et al. (2014), Wang et al. (2019)].
Seasonal changes in the transport of oxygen and substrates (especially lipids, McWilliams et al., 2004; Guglielmo, 2010) also regularly contribute to seasonal metabolic variation in birds (Swanson, 2010; Petit and Vézina, 2014; Zhang et al., 2015a). Increases in lipid transport and catabolism are also often positively correlated with higher winter Msum in birds (Swanson, 2010; Zhang et al., 2015b). Moreover, acute cold and exercise training increased lipid transport and cellular metabolic intensity in house sparrows, Passer domesticus, especially in terms of intracellular lipid transport via cytosolic fatty acid binding protein, FABPc (Zhang et al., 2015c). Such changes are particularly important to birds because lipids serve as the primary fuel for shivering and birds rely on exogenous lipids to fuel this activity (McWilliams et al., 2004; Vaillancourt et al., 2005).
Metabolic flexibility and its mechanistic underpinnings are likely mediated, at least in part, through transcriptional changes in underlying gene expression networks (Ayroles et al., 2009; Stager et al., 2015; Cheviron and Swanson, 2017). Cheviron and Swanson (2017) studied seasonal transcriptomic variation pectoralis muscle, the primary thermogenic organ (Hohtola, 1982; Marsh and Dawson, 1989) in American goldfinches (Spinus tristis) and black-capped chickadees (Poecile atricapillus), two North American year-round resident species in cold winter climates. Interestingly, chickadees showed greater summer-to-winter variation in differentially expressed genes than did goldfinches, although both species showed similar overall patterns of differential gene expression between seasons (Cheviron and Swanson, 2017). Some genes and pathways associated with muscle growth and remodeling showed differential seasonal expression in both chickadees and goldfinches (Cheviron and Swanson, 2017). The substrate metabolism pathways exhibiting significant increases in transcript abundance in winter included “fatty acid metabolism,” “tricarboxylic acid cycle,” and “oxidative phosphorylation” in chickadees and “glycerolipid metabolism” in both species (Cheviron and Swanson, 2017). These changes suggests that seasonal modifications to fat metabolism are an important component of winter acclimatization in both species.
The quantities and types of metabolites are downstream products of gene expression, protein interactions and other regulatory processes, and thus provide a functional snapshot of the phenotype under specific conditions (Guijas et al., 2018). Because gene expression does not necessarily indicate or correlate with protein expression or metabolic pathway regulation, including during seasonal acclimatization or temperature acclimation in birds (e.g., King et al., 2015; Stager et al., 2015; Zhang et al., 2015b), integration of ‘omics data sets can provide a robust, comprehensive picture of organismal responses to changing energy demands that can reveal pathway-level changes that are not apparent through individual analytical methods (Tung et al., 2019; Savva et al., 2022). Few studies have attempted integration of different ‘omics data sets. In the present study, however, we generated a metabolomics data set for the same individual birds from which Cheviron and Swanson (2017) developed a transcriptomic data set. We then integrated the metabolomic and transcriptomic data sets to compare black-capped chickadees and American goldfinches in summer and winter. We hypothesize that chickadees would show greater seasonal differences in metabolites relative to goldfinches, as they did for transcript abundances (Cheviron and Swanson, 2017). We also hypothesize that metabolites related to skeletal muscle function and growth and those that function in fat metabolism pathways will vary seasonally in a manner consistent with the increased abundances of related transcripts in winter in both species. We anticipate that integration of these ‘omics data sets might also elucidate additional important mechanistic pathways that could contribute to seasonal metabolic phenotypes in small passerine birds.
Materials and Methods
All birds for the present study were collected using mist nets near Vermillion, Clay County, South Dakota (approximately 42°47′N 96°55′W) in summer (June to August) and winter (December to February) in 2010–2012 as described in Swanson et al. (2014) and Cheviron and Swanson (2017). All American goldfinches used in this study (Summer n = 10, Winter n = 7) were adults. All black-capped chickadees collected in winter (n = 7) were adults, but summer-collected chickadees consisted of six adults and four fully grown independent hatch-year birds that were likely 1.5–2 months old (Cheviron and Swanson, 2017). Hatch-year summer chickadees did not differ significantly in body size or pectoralis muscle mass from adults (Cheviron and Swanson, 2017). After capture, we transported the birds back to the laboratory and euthanized them within 2 h by cervical dislocation. Following euthanasia, we rapidly dissected out pectoral muscle (one side only) on ice and weighed the sample to the nearest 0.1 mg before flash-freezing in liquid nitrogen. We stored muscle samples at –80°C until metabolomic assays, except that we briefly partially thawed the muscle sample to remove a sub-sample for another study (Swanson et al., 2014), but immediately re-froze the sample in liquid nitrogen after removal of the sub-sample. Some of the muscle samples did not have sufficient tissue for metabolic profiling, hence a slightly smaller subset was used compared to the transcriptomic set used in the Cheviron and Swanson (2017) study. The resulting tissue sample sizes used for profiling were Summer n = 9, Winter n = 7 for chickadees and Summer n = 7, Winter n = 7 for goldfinches, where transcriptomic data were available (Cheviron and Swanson, 2017) for these samples. We captured birds under active state (permit #10-2, #11-7, and #12-2) and federal (permit #MB758442) scientific collecting permits and all procedures were approved by the University of South Dakota Institutional Animal Care and Use Committee (Protocol # 79-01-11-14).
For metabolite extractions, we first ground pectoral muscle to a fine powder under liquid nitrogen and about 20 mg of powdered muscle was used for the unbiased global metabolic profiling performed by Metabolon (Durham, NC, United States). Briefly, each 20-mg muscle tissue sample was extracted at a 1:5 ratio in methanol plus recovery standards using an automated MicroLab STAR system (Hamilton Company, Salt Lake City, UT, United States). To remove protein, dissociate small molecules bound to protein or trapped in the precipitated protein matrix, and to recover chemically diverse metabolites, proteins were precipitated with methanol under vigorous shaking for 2 min (Glen Mills GenoGrinder 2000) followed by centrifugation. The resulting extract was divided into five fractions: two for analysis by two separate reverse phase (RP)/UPLC-MS/MS methods with positive ion mode electrospray ionization (ESI), one for analysis by RP/UPLC-MS/MS with negative ion mode ESI, and one for analysis by HILIC/UPLC-MS/MS with negative ion mode ESI.
When a metabolite was below the threshold of detection, data were imputed as the minimum detected quantity for that metabolite in this project. Resulting data were log (base 2)-transformed to normalize and then initially analyzed using a two-way ANOVA, with season and species as factors. Reported significant differences from the two-way ANOVA are p < 0.05 and q < 0.10, where q is the False Discovery Rate (FDR; Storey, 2002). We then mapped the detected metabolites onto the biochemical pathways to which they belonged and categorized pathways into related groups (e.g., glucose mapped onto the glycolysis pathway and grouped into carbohydrate metabolism). We used principal component analysis (PCA) to transform the detected metabolites into a smaller number of orthogonal variables (i.e., component 1 and component 2) to visualize the overall variation in metabolite profiles among species and seasons and to provide a global overview of the dataset.
We used the web-based metabolomics data processing tool, MetaboAnalyst 5.01 to provide overviews of metabolite data (i.e., fold-change analysis and comparative cluster analysis). Briefly, univariate fold-change analyses were performed using the fold-change analysis module for each species with fold-change threshold set at 2.0. For an overview of hierarchical cluster analysis (HCA), a heat map visualization of metabolite abundances was generated from hierarchical clustering using Ward’s linkage and Euclidean distance. We focused our interpretations of metabolite analyses on substrate (carbohydrates, amino acids, and lipids) metabolism pathways and on intermediates of key metabolic pathways related to energy production [e.g., tricarboxylic Acid Cycle (TCA), glycolysis, and NAD/NADH metabolism], which are relevant to previously documented seasonal variation in organismal metabolic capacities in the two study species (Cooper and Swanson, 1994; Liknes et al., 2002).
To evaluate whether the relationships between transcript and metabolites differ by species and season, we used a linear model approach (Siddiqui et al., 2018) to integrate the current metabolomics data with the previously published transcriptomics data for the same tissues of black-capped chickadees and American goldfinches (Cheviron and Swanson, 2017). This linear model approach can identify transcript-metabolite associations that are specific to a particular phenotype (Patt et al., 2019; Banerjee et al., 2020). Significant transcript-metabolite pairs were clustered by the direction of association (i.e., Winter correlated and Winter anti-correlated). Winter correlated means that certain combinations of both transcripts and metabolites increase in winter relative to summer, whereas Winter anti-correlated means that certain combinations of transcripts increase and metabolites decrease (or vice versa) in winter relative to summer. To reveal the underlying seasonal pathways in each species (i.e., Winter-specific and Summer-specific) from the integration of the metabolomic and transcriptomic data, we conducted pathway overrepresentation analyses on significant transcript-metabolite pairs using the web-based ‘omics integration tool, IMPaLA: Integrated Molecular Pathway Level Analysis (Kamburov et al., 2011)2.
Results
Metabolome Composition of Black-Capped Chickadee and American Goldfinch
A total of 582 metabolites was characterized in samples from birds collected in summer or winter to determine patterns of seasonal shifts in metabolites of black-capped chickadees and American goldfinches (Figure 1A). Lipids (50%) were the most prevalent, followed by amino acids (21%), carbohydrates (8%), nucleotides (7%), cofactors (5%), peptides (4%), and xenobiotics (3%). Energy-related metabolites were the least (2%) prevalent. PCA indicated that these samples formed distinct groupings by species and season, where Component 1 separated the two species and Component 2 separated between Summer and Winter samples (Figure 1B). Chickadees showed the greatest separation between the Summer and Winter samples, whereas goldfinches showed much less separation between Summer and Winter samples. HCA indicated a primary split in the bottom third of the heatmap that separated chickadee Winter samples from Summer samples, with a secondary split separating goldfinch Winter samples from Summer samples (Figure 2A).
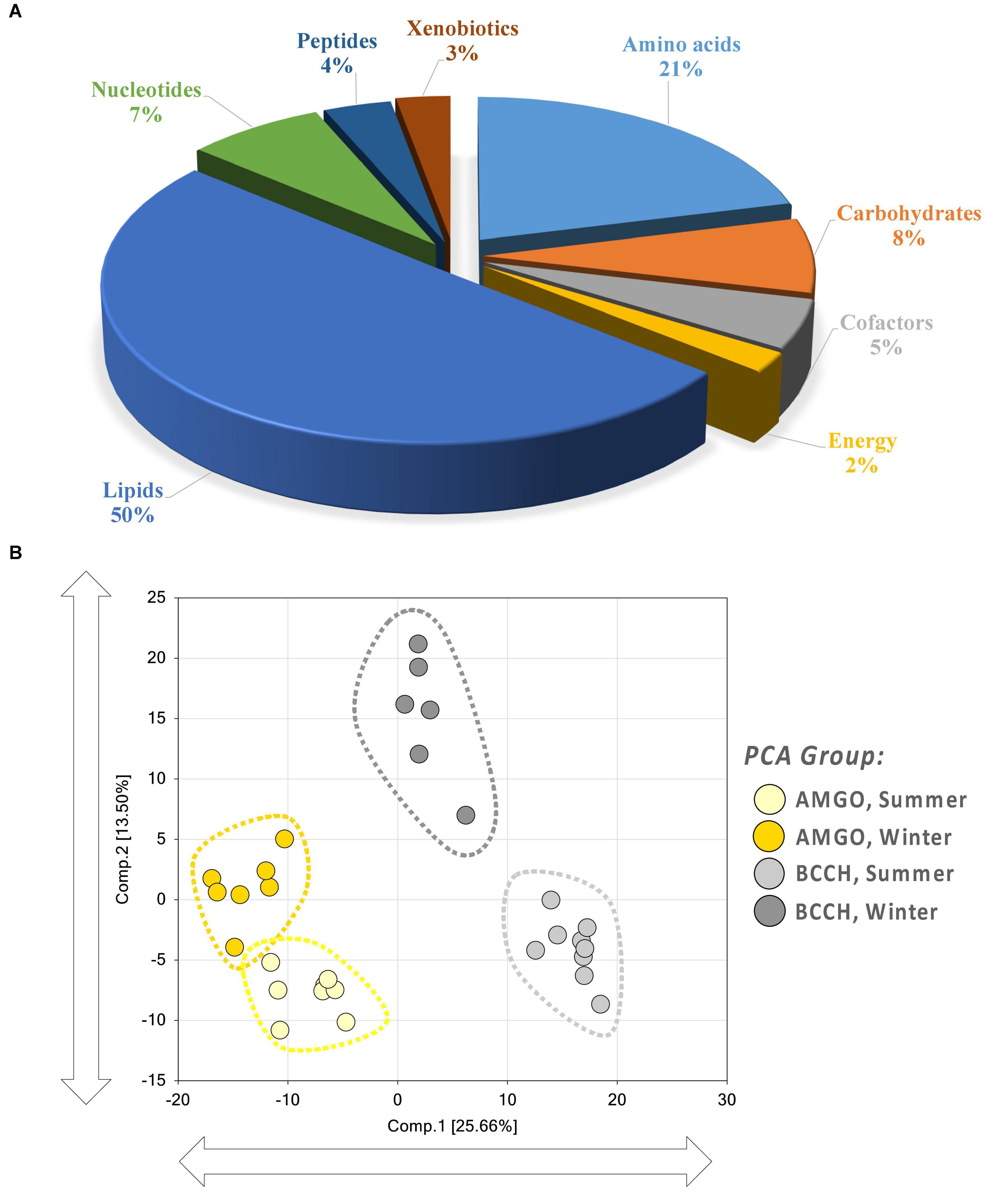
Figure 1. Global metabolome of pectoralis muscle in Black-capped Chickadee (BCCH) and American Goldfinch (AMGO) during Summer and Winter. (A) Functional categorization of 582 identified metabolites detected. (B) Pectoralis muscle sample distribution as determined by Principal Component Analysis (PCA). Ovoids highlight species/season combinations.
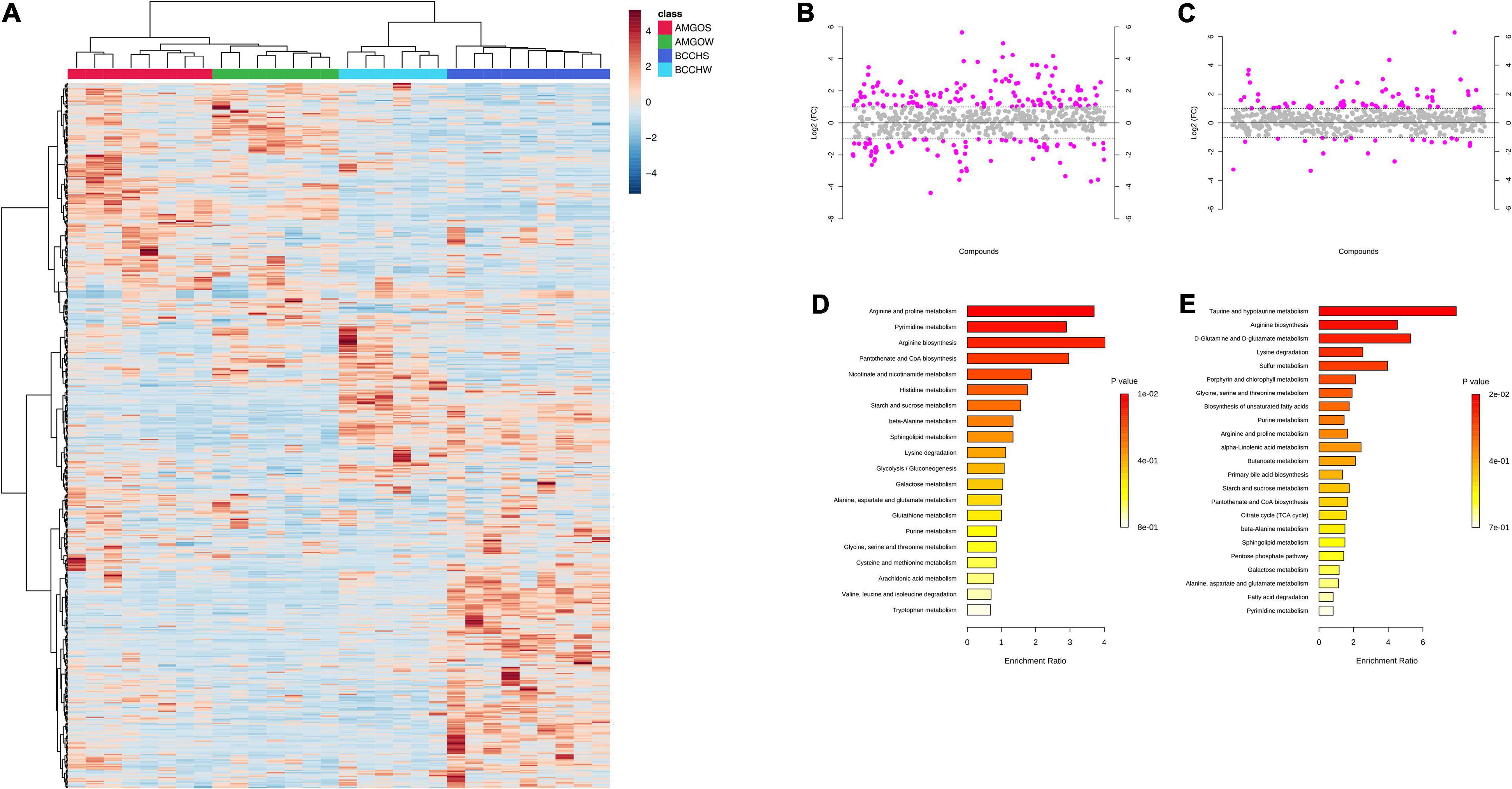
Figure 2. Metabolite abundances in pectoralis muscle in Black-Capped Chickadee (BCCH) and American Goldfinch (AMGO) during Summer and Winter. (A) Clustered by seasons, where class color at the top of the heatmap represents samples/individuals, and the vertical dendrogram indicates individual metabolites and their relations to each other; blue = BCCHS – Black-Capped Chickadee Summer; light blue = BCCHW –Black-Capped Chickadee Winter, red = AMGOS – American Goldfinch Summer, green = AMGOW – American Goldfinch Winter. Color in the heatmaps reflects the relative metabolite abundance: red represents metabolite abundances higher than the mean and blue represents metabolite abundances lower than the mean. Metabolites with greater than twofold increase in the (B) black-capped chickadee (Winter compared to Summer, Supplementary Table 1) and the (C) American Goldfinch (winter vs. summer; Supplementary Table 2). Significantly enriched (P < 0.05) metabolic pathways according to metabolites with greater than twofold increase in the (D) black-capped chickadee (Winter compared to Summer) and the (E) American goldfinch (Winter compared to Summer).
Seasonal Shifts in Metabolic Profiles of Pectoral Muscle
More distinct significant changes were detected in the metabolic profiles of pectoral muscles of chickadees between Summer and Winter compared to pectoral muscles of goldfinches between Summer and Winter (Figure 2A). Specifically, 198 metabolites exhibited more than a twofold significant change in abundance between Summer and Winter profiles in chickadees, whereas only 85 metabolites exhibited more than a twofold significant change in abundance between Summer and Winter profiles in goldfinches (Figures 2B,C and Supplementary Tables 1, 2). In winter, chickadees showed greater than twofold enrichment in arginine and proline metabolism, pyrimidine metabolism, arginine biosynthesis, pantothenate and CoA biosynthesis, nicotinate and nicotinamide metabolism, and histidine metabolism, whereas goldfinches showed greater than twofold enrichment in taurine and hypotaurine metabolism, arginine biosynthesis, D-glutamine and D-glutamate metabolism, lysine, deregulation, sulfur metabolism, biosynthesis of unsaturated fatty acids (FAs), and alpha-linolenic metabolism (Figures 2D,E).
Seasonal Shifts in Amino Acids
The abundance of amino acids tended to decrease in Winter for both species. Abundances of several amino acids (e.g., Ala, alanine; Glu, glutamate; His, histidine; Lys, lysine; Phe, phenylalanine; Tyr, tyrosine; Trp, tryptophan; Leu, leucine; Ile, isoleucine; Val, valine; Met, methionine; Arg, arginine) and their catabolites tended to decrease in Winter, though these shifts did not achieve significance for comparisons in either species (Figure 3A). While Arg abundance tended to decrease in Winter, creatine abundance was elevated in Winter for both species, with a significant increase in creatinine only for chickadees. The abundance of creatine phosphate decreased in Winter for both species (Figure 3B) but did not vary significantly with season in goldfinches.
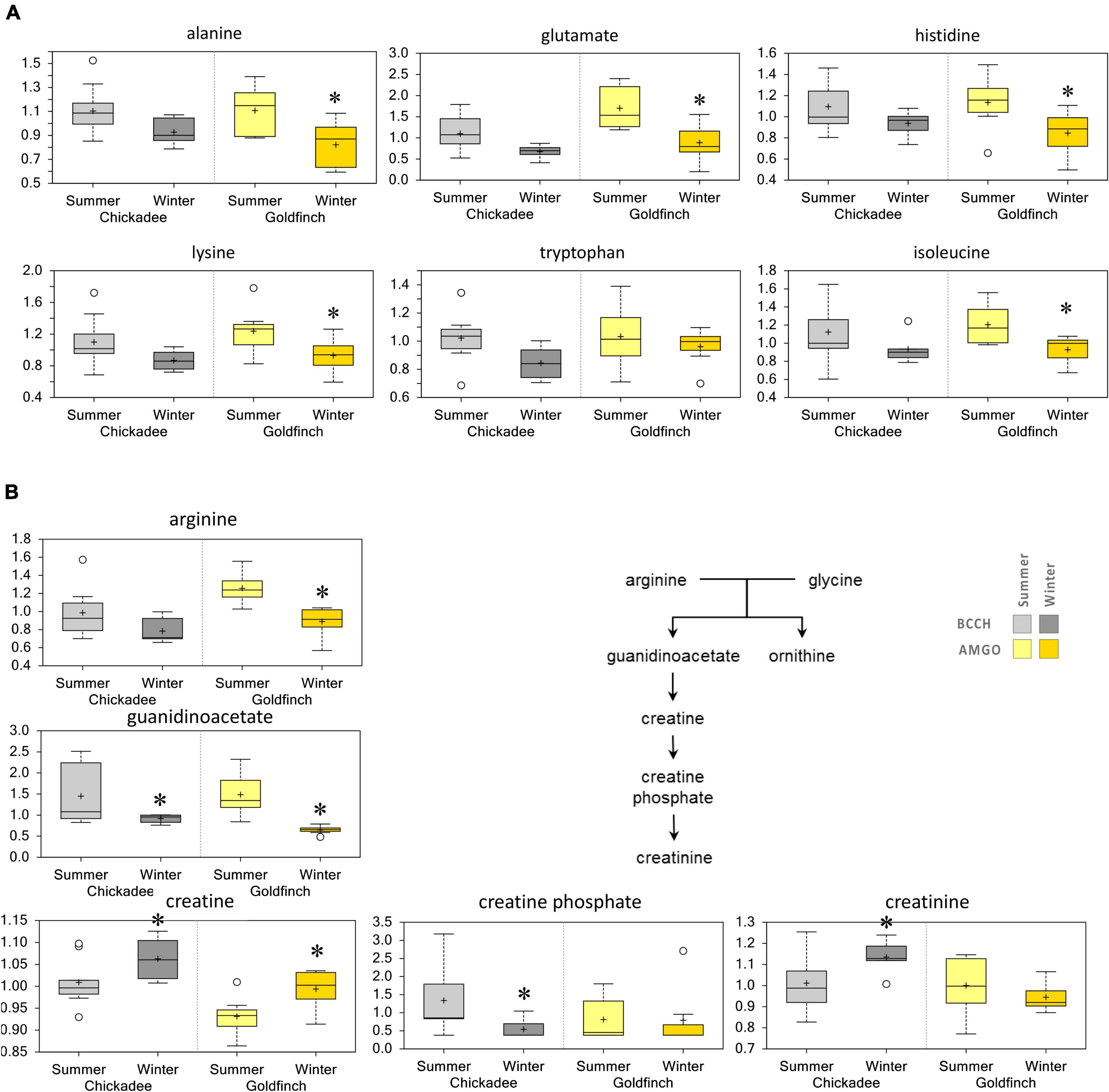
Figure 3. Differences in the abundances of (A) amino acids and (B) amino acid derivatives in pectoralis muscle of Black-Capped Chickadee (BCCH) and American Goldfinch (AMGO). The X-axis represents the species and the seasons sampled. The Y-axis box plots indicate the scaled intensity mean (+) and median (–) values: upper and lower ranges of boxes indicate upper and lower quartiles, respectively; upper and lower whiskers indicate the maximum and minimum distributions of the data; small circles represent outlying data points. *Significantly (p < 0.05 and q < 0.10) different compared to the other season within the species.
Seasonal Shifts in Lipid Metabolites
Fatty acids are a critical source of energy for mitochondrial oxidation and cellular ATP generation, as well as being precursors for phospholipids and storage lipids. While the abundance of long-chain FAs did not significantly differ between Winter and Summer for goldfinches (Figure 4), the abundance of longer-chain FAs (e.g., C20-C24 FAs behenate, erucate, and nervonate) was increased in Winter for chickadees, whereas the abundances of several other FAs (e.g., myristate, margarate, and 1-heptadecenoate) decreased. Interestingly, the abundance of omega-3 polyunsaturated fatty acids (PUFAs), such as eicosapentaenoate and docosapentaenoate were also lower in Winter for both species, whereas the abundance omega-6 PUFAs (e.g., linoleate, dihomo-linoleate) were elevated in Winter for chickadees. Also, the abundance of 1-stearoyl-2-arachidonoyl-GPC tended to increase in Winter for both species.
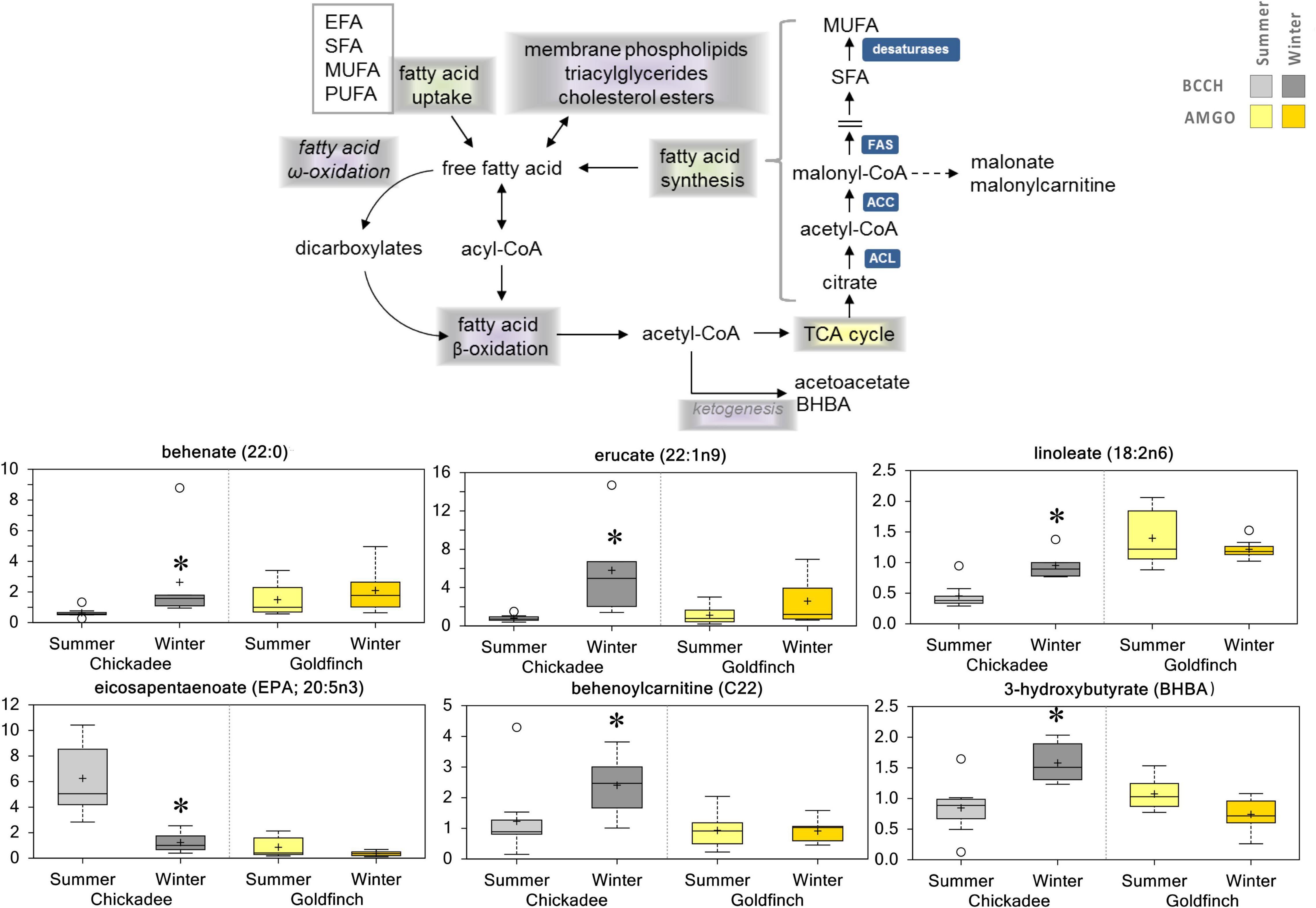
Figure 4. Differences in the abundances of metabolites involved in lipid metabolism in pectoralis muscle of Black-Capped Chickadee (BCCH) and American Goldfinch (AMGO). The X-axis represents the species and the seasons sampled. The Y-axis box plots indicate the scaled intensity mean (+) and median (–) values: upper and lower ranges of boxes indicate upper and lower quartiles, respectively; upper and lower whiskers indicate the maximum and minimum distributions of the data; small circles represent outlying data points. *Significantly (p < 0.05 and q < 0.10) different compared to the other season within the species.
Seasonal Shifts in Carbohydrate Metabolites
Few significant changes in carbohydrate metabolism were detected in Winter for goldfinches. However, the abundance of 3-carbon glycolytic intermediates (2-phosohoglycerate, 3-phoshoglycerate, and phosphoenolpyruvate) and pyruvate decreased in Winter chickadees but not goldfinches (Figure 5).
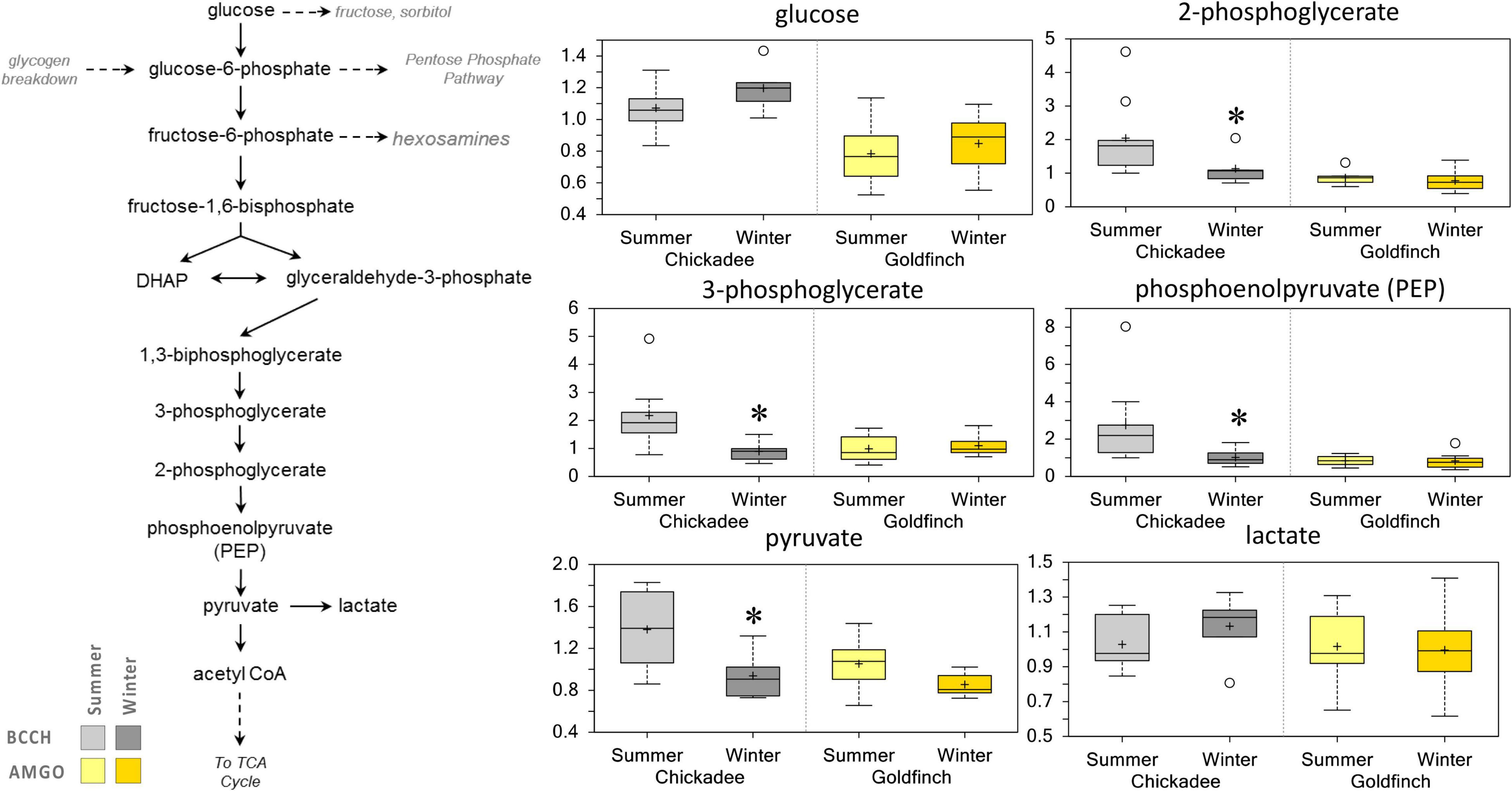
Figure 5. Differences in the abundances of metabolites involved in glucose metabolism in pectoralis muscle of Black-Capped Chickadee (BCCH) and American Goldfinch (AMGO). The X-axis represents the species and the seasons sampled. The Y-axis box plots indicate the scaled intensity mean (+) and median (-) values: upper and lower ranges of boxes indicate upper and lower quartiles, respectively; upper and lower whiskers indicate the maximum and minimum distributions of the data; small circles represent outlying data points. *Significantly (p < 0.05 and q < 0.10) different compared to the other season within the species.
Seasonal Shifts in Tricarboxylic Acid Cycle Metabolites
Carbohydrates and lipids are among several sources of carbon for the TCA cycle. Other carbon sources could include, via conversion of acetyl-CoA to citrate, glutamine entering as alpha-ketoglutarate, and branched-chain amino acids entering as citrate and succinyl-CoA. Decreased abundances of citrate, isocitrate, and alpha-ketoglutarate were observed in Winter for goldfinches and the abundance of succinate decreased in Winter for chickadees (Figure 6).
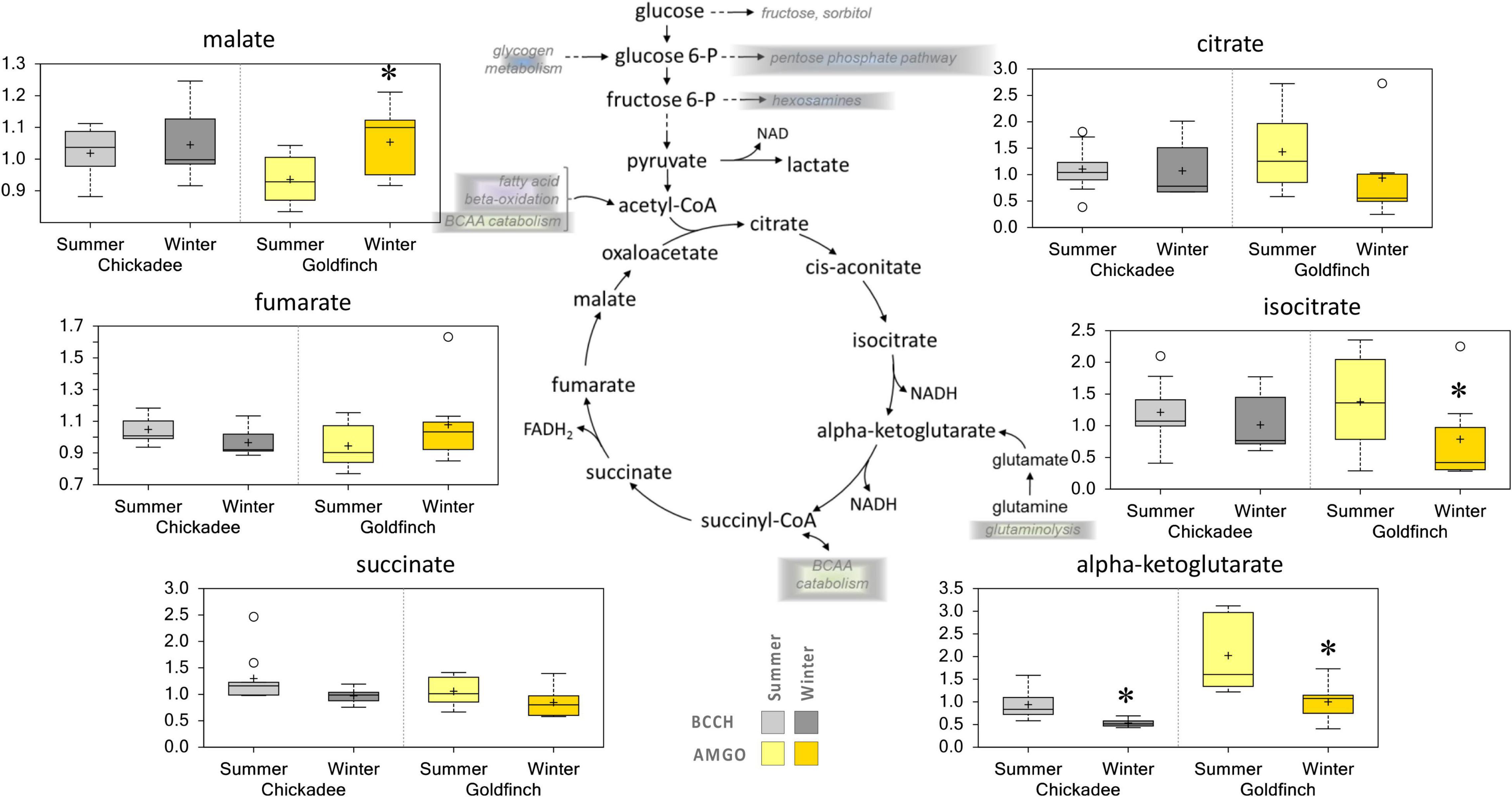
Figure 6. Differences in the abundances of metabolites involved in TCA cycle metabolism in pectoralis muscle of Black-Capped Chickadee (BCCH) and American Goldfinch (AMGO). The X-axis represents the species and the seasons sampled. The Y-axis box plots indicate the scaled intensity mean (+) and median (-) values: upper and lower ranges of boxes indicate upper and lower quartiles, respectively; upper and lower whiskers indicate the maximum and minimum distributions of the data; small circles represent outlying data points. *Significantly (p < 0.05 and q < 0.10) different compared to the other season within the species.
Seasonal Shifts in Other Metabolites
The abundance of several metabolites showed trends toward increasing levels in Winter for one or both species. The abundance of tocopherols increased in Summer for both species, whereas the abundances of biliverdin, taurocholate, and taurochenodeoxycholate were elevated in Winter only for goldfinches. The abundances of serotonin, and tryptophan betaine increased in Winter, but only for chickadees (Figure 7). The abundance of NADH was significantly increased in Winter for both species, whereas the abundances of nicotinamide riboside was significantly elevated in Winter only for goldfinches (Figure 8).
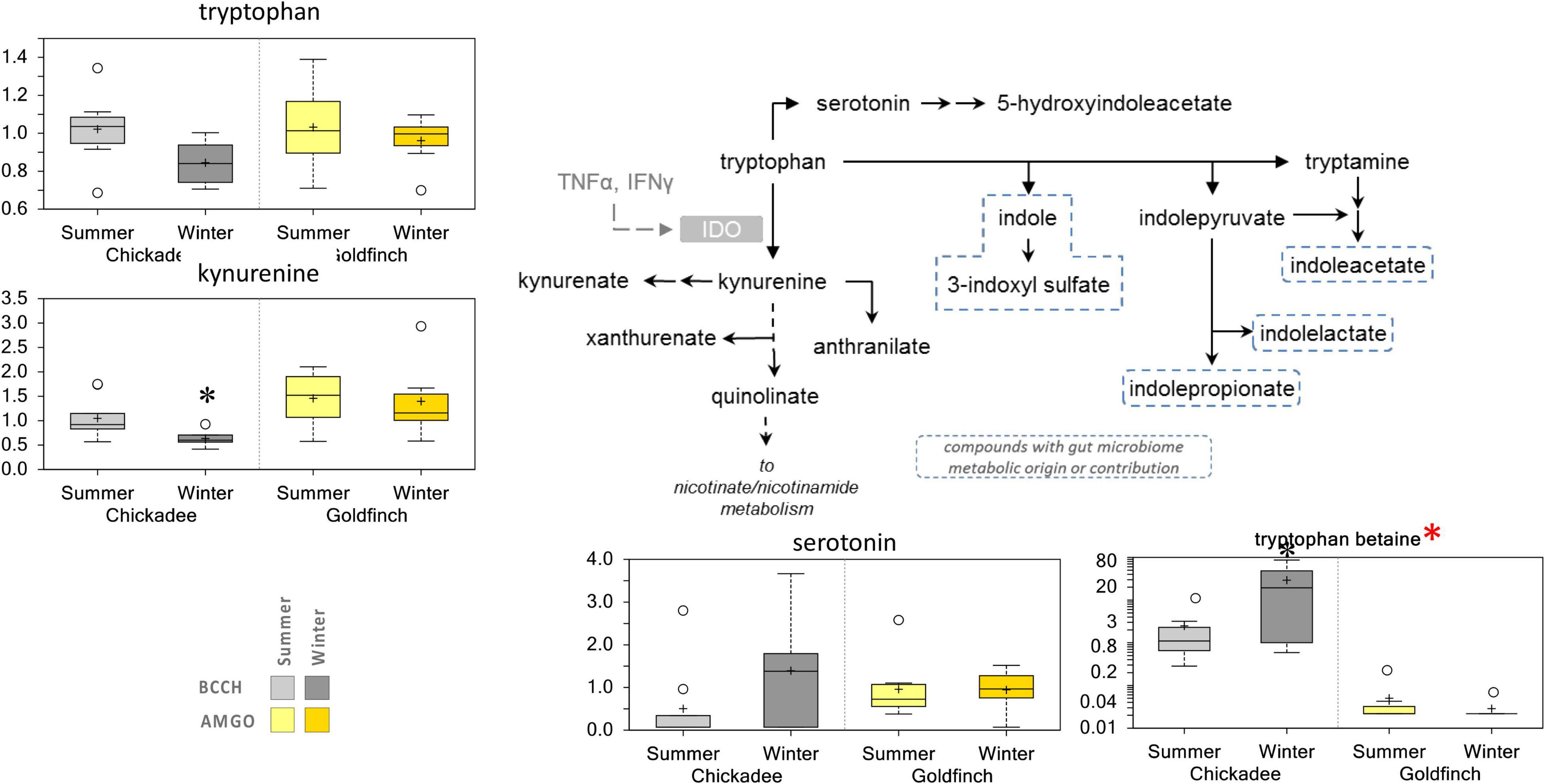
Figure 7. Differences in the abundances of metabolites involved tryptophan metabolism in pectoralis muscle of Black-Capped Chickadee (BCCH) and American Goldfinch (AMGO). The X-axis represents the species and the seasons sampled. The Y-axis box plots indicate the scaled intensity mean (+) and median (-) values: upper and lower ranges of boxes indicate upper and lower quartiles, respectively; upper and lower whiskers indicate the maximum and minimum distributions of the data; small circles represent outlying data points. Red asterisk—The Y-axis of this plot has been transformed to a log-scale to better show population differences. *Significantly (p < 0.05 and q < 0.10) different compared to the other season within the species.
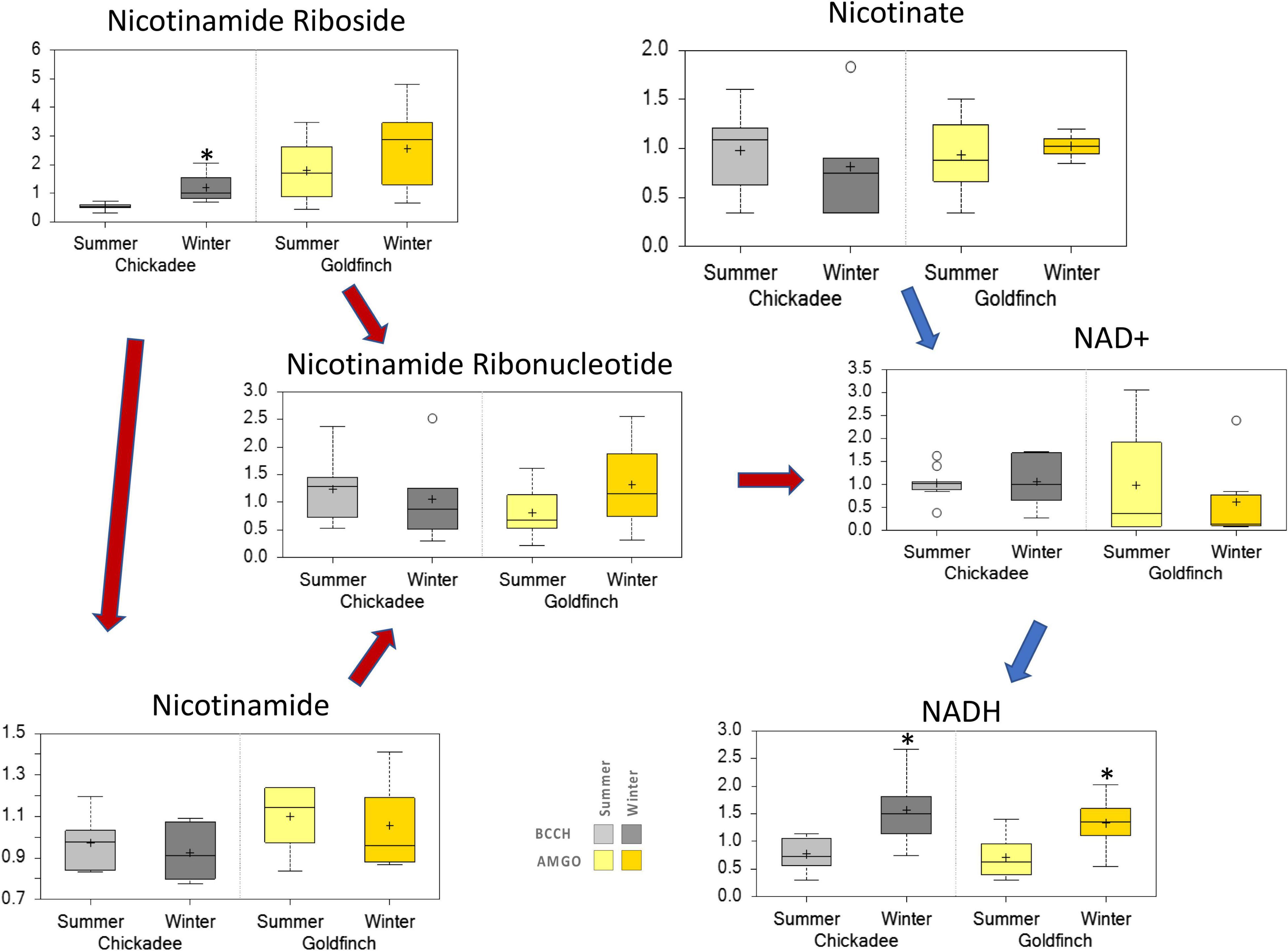
Figure 8. Differences in the abundances of metabolites involved nicotinate and nicotinamide metabolism in pectoralis muscle of Black-Capped Chickadee (BCCH) and American Goldfinch (AMGO). The X-axis represents the species and the seasons sampled. The Y-axis box plots indicate the scaled intensity mean (+) and median (-) values: upper and lower ranges of boxes indicate upper and lower quartiles, respectively; upper and lower whiskers indicate the maximum and minimum distributions of the data; small circles represent outlying data points; *Significantly (p < 0.05 and q < 0.10) different compared to the other season within the species. Blue arrows—NAD+ de novo via Preiss–Handler pathway; Red arrows—NAD+ salvage pathway.
Landscape of Gene-Metabolite Associations
Changes in season-specific transcript-metabolite pairs were much more pronounced in black-capped chickadee compared to American goldfinch (Figure 9). Linear model analysis identified 3,019 Winter-specific transcript-metabolite associations in chickadees (Benjamini-Hochberg FDR, Benjamini and Hochberg, 1995; adjusted interaction p-value < 0.10, and a Spearman correlation difference > | ± 0.5|, Supplementary Table 3) involving 1,651 genes and 269 metabolites. In contrast, the analysis identified only 69 Winter-specific transcript-metabolite associations in goldfinches (Benjamini-Hochberg FDR- adjusted interaction p-value < 0.10, and a Spearman correlation difference > | ± 0.5|; Supplementary Table 4) involving 53 genes and 38 metabolites. Clustering of these pairs by the positive (i.e., Winter correlated) or negative (i.e., Winter anti-correlated) direction of association within each season revealed two major clusters for both chickadees (Figure 9A) and goldfinches (Figure 9B). For chickadees, the Winter correlated cluster of 1,373 transcript-metabolite pairs (816 unique genes and 185 unique metabolites) showed relatively high positive correlations in Winter samples and low or mostly negative correlations in Summer samples, whereas the Winter anti-correlated cluster of 1,646 transcript-metabolite pairs (908 unique genes and 180 unique metabolites) showed relatively high negative correlations in Winter samples and positive or low negative correlations in Summer samples (Supplementary Table 3). In contrast, the Winter correlated cluster in goldfinches consisted of 26 transcript-metabolite pairs (20 unique genes and 18 unique metabolites) with relatively high positive correlations in Winter samples and mostly negative correlations in Summer samples. The Winter anti-correlated cluster in goldfinches was composed of 43 transcript-metabolite pairs (33 unique genes and 28 unique metabolites) with relatively high negative correlations in Winter samples and relatively high positive correlations in Summer samples (Supplementary Table 4).
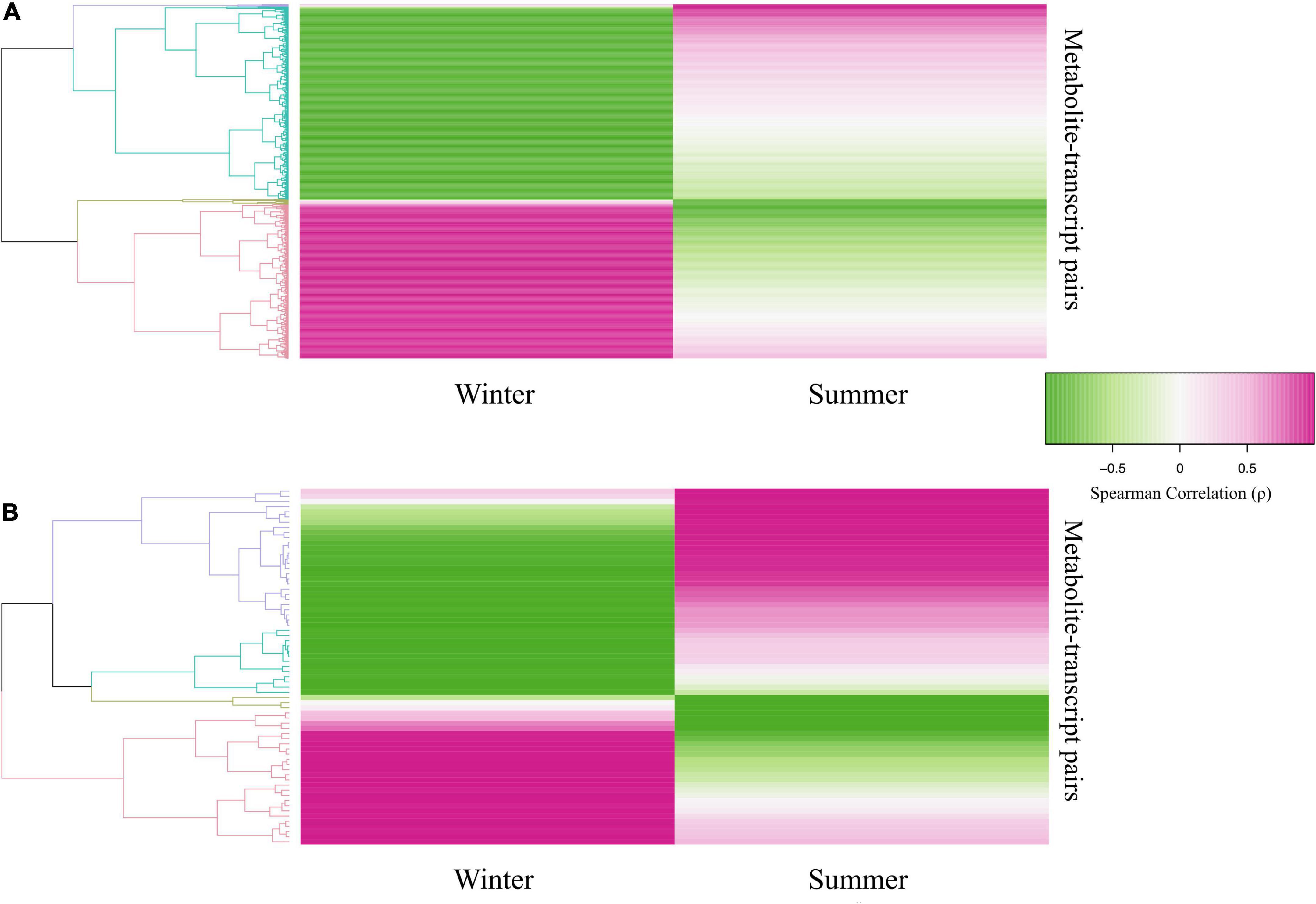
Figure 9. Results of the integration through linear model approach (IntLIM; Siddiqui et al., 2018) applied to the transcriptomic and metabolomic data sets of pectoralis muscle from black-capped chickadee and American goldfinch. Clustering of identified transcript-metabolites pairs (Benjamini-Hochberg FDR—adjusted interaction p-value < 0.10, and a Spearman correlation difference > | ± 0.5|) in (A) Black-capped chickadee and (B) American goldfinch. Fuchsia, Winter correlated; Green, Winter anti-correlated.
Integrated molecular pathway level analysis of the 1,373 transcript-metabolite pairs (mappable genes and metabolites) in the chickadee Winter correlated cluster identified 1,065 pathways that were overrepresented (Supplementary Table 5), with 781 pathways shared with the Winter anti-correlated cluster and 284 pathways unique to the Winter correlated cluster. In the Winter correlated cluster (i.e., 1,065 pathways), the top 25 overrepresented transcript-metabolite pathways fall within pathways associated with transport of small molecules, metabolism of amino acids and derivatives, and activation and biosynthesis of fatty acid derivatives. The top 25 unique (i.e., 284 pathways) overrepresented transcript-metabolite pathways included beta oxidation of FAs and biosynthesis of amino acids and carnitine derivatives (Supplementary Table 9). Of the chickadee Winter anti-correlated cluster transcript-metabolite pairs (N = 1,686), 983 pathways were overrepresented (Supplementary Table 6) and 202 pathways were unique to the Winter anti-correlated cluster. Like the Winter correlated cluster, the top 25 overrepresented transcript-metabolite pathways of the Winter anti-correlated cluster (i.e., 983 pathways) included pathways associated with transport of the small molecules, metabolism of amino acids and nucleotides, and biosynthesis of FAs. The top 25 unique (i.e., 202 pathways) overrepresented transcript-metabolite pathways in chickadees included communication and release of neurotransmitters and related signaling molecules (Supplementary Table 9). Interestingly, overrepresented transcript-metabolite pathways common to both Winter correlated and anti-correlated clusters are metabolism of polyamines, and biosynthesis of putrescine, spermine, and spermidine, as well as their precursor ornithine (Supplementary Tables 5, 6).
In contrast to chickadees, integrated molecular pathway level analysis of the 26 transcript-metabolite pairs (mappable genes and metabolites) in the goldfinch Winter correlated cluster identified 40 pathways that were overrepresented (Supplementary Table 7). Of these 40 pathways, 28 were shared with the Winter anti-correlated cluster and 12 pathways were unique to the Winter correlated cluster. In the Winter correlated cluster (i.e., 40 pathways), the top 25 transcript-metabolite pathways overrepresented included metabolism of sphingolipids and osteoblast signaling. Only 12 unique overrepresented transcript-metabolite pathways were uncovered and these mainly included metabolism and biosynthesis of nicotinamides (Supplementary Table 10). Of the goldfinch Winter anti-correlated cluster of transcript-metabolite pairs (N = 43), 96 pathways were overrepresented (Supplementary Table 8), with 68 pathways unique to the Winter anti-correlated cluster. In the Winter anti-correlated cluster (i.e., 96 pathways), the top 25 transcript-metabolite pathways overrepresented include transport of nucleotides and vitamins, metabolism of sphingolipids and lipids. Overall, the top 25 unique (i.e., 68 pathways) transcript-metabolite overrepresented pathways include transport of nucleosides and vitamins and metabolism of nucleotides and FAs (Supplementary Table 10).
Discussion
The winter-adaptive response of small birds is a complex phenomenon influenced by environmental and genetic factors and involves many molecular/biochemical pathways in the primary thermogenic organ, the pectoralis muscle. Because the pectoralis muscle is also the largest organ in volant birds and is important to both locomotion and shivering, the pectoralis muscle is also an essential location for the metabolism of carbohydrates and lipids (Pedersen and Febbraio, 2012; Morales et al., 2017). Our metabolic profiling and integration of the metabolomic and transcriptomic data for the same muscle tissue not only highlighted molecular functions and pathways linked to the winter-adaptive response but revealed previously unknown winter-specific patterns of transcript-metabolite coordination that could contribute to the metabolic flexibility of temperate-zone resident birds during cold winter seasons.
The abundances of some complex polyunsaturated lipids (e.g., 1-stearoyl-2-arachidonoyl-GPC) tended to increase in both chickadees and goldfinches in Winter compared with Summer. In addition, omega-3 PUFAs tended to show decreases in winter, whereas omega-6 PUFAs increased in winter for chickadees. These differences could reflect seasonal differences in dietary intake. Goldfinches are thought to eat primarily a vegetarian diet year-round (McGraw and Middleton, 2020), and whereas chickadees eat predominantly insects in summer, their diet is supplemented with seeds and fruit in winter when insects are scarce (Foote et al., 2020). Seeds tend to be high in oleate and linoleate and other PUFAs (Collins and Horn, 2012; Maikhuri et al., 2021), whereas insects such as caterpillars can be high in omega-3 PUFAs (Lautenschläger et al., 2017; Guil-Guerrero et al., 2018; Mba et al., 2019). In addition, aquatic insects may represent an important dietary subsidy for woodland birds (Smith et al., 2007; Allen, 2019), including chickadees and goldfinches, if woodlands are located near water bodies, as many of our study sites were. Aquatic arthropods contain highly unsaturated omega-3 fatty acids from their algal-based diet and, therefore, differ from most terrestrial arthropods in fatty acid composition of body tissues (Twining et al., 2018, 2019, 2021). The low dietary intake of arthropods, especially aquatic insects, in winter by both species could potentially help explain reduced levels of dietary PUFAs and this could potentially impact metabolic performance. However, woodland birds like chickadees and goldfinches, consumed few aquatic arthropods, even in spring, late summer, and autumn in riparian areas in our study area (Liu, 2015; Wesner et al., 2020). Also, because goldfinches eat mostly seeds all year, aquatic resources probably aren’t very important dietary items. Both species showed similar seasonal trends in PUFA metabolites, suggesting that differences in availability of aquatic resources are likely not the explanatory factor for the seasonal differences documented in this study. The potential impacts of these seasonal differences in omega-3 and omega-6 FAs on thermogenic performance are uncertain. Highly unsaturated omega-3 FAs may positively impact breeding and flight performance in birds (Pierce and McWilliams, 2014; Martinez del Rio and McWilliams, 2016; Twining et al., 2018). Omega-6 FAs may also be associated with improved exercise metabolic performance in endotherms (Ayre and Hulbert, 1997; Pierce et al., 2005; Price et al., 2011; Pierce and McWilliams, 2014). Thus, lower omega-3, but higher omega-6, fatty acid levels in winter may have contrasting effects on thermogenic performance, but demonstration of such effects will require further study.
Interpreting the direction of seasonal variation in flux through metabolic pathways from single measurements of metabolite levels is difficult, but seasonal changes in metabolite levels do suggest alterations in flux through metabolic pathways. The abundances of complex long-chain lipids (e.g., 1,2-dipalmitoyl-GPC) tended to be lower in winter samples, but winter increases in several acylcarnitines (e.g., behenoylcarnitine, erucoylcarnitine) also occurred. Complex long-chain FAs might be reduced to shorter-chain FAs by peroxisomes (Poulos, 1995; Carter et al., 2019) for delivery to mitochondria, so we hypothesize that winter decreases in long-chain FAs might reflect increases in production of short-chain FAs for delivery to mitochondria for beta-oxidation. Winter increases in several acylcarnitines might be consistent with the increased production of shorter-chain FAs from complex long-chain FAs prior to transport into mitochondria, which could suggest increased demand for use to support beta-oxidation. Increases in beta-oxidation capacity are a common element of the winter phenotype in birds (Marsh and Dawson, 1989; Swanson, 2010). Such increases in fatty acid availability might support shifts in energy use toward lipid substrates in winter (Marsh and Dawson, 1989; Swanson, 2010). Moreover, the ketone body 3-hydroxybutyrate (BHBA) was elevated in Winter in chickadees but showed a trend toward a winter decrease for goldfinches. The abundance of other ketone bodies also showed winter decreases for goldfinches, which could reflect increased energy demand or subtle changes in substrate availability, perhaps due to a higher proportion of seeds in the winter diet of goldfinches than chickadees. Further studies assessing plasma and/or liver function could shed additional light on seasonal changes in lipid metabolism in these two small bird species.
The reduced abundances of many amino acids in both species from Winter samples suggest potential changes in protein turnover rates in winter birds associated with their higher metabolic rates (Cooper and Swanson, 1994; Liknes et al., 2002). We hypothesize that the generally lower amino acid levels might be associated with increased protein turnover rates in winter birds. Consistent with this hypothesis, cold exposure increased carbon turnover rates in pectoralis muscle of zebra finches (Taeniopygia guttata) relative to flight exercise-trained and control birds that were within their thermal neutral zone (Bauchinger et al., 2010). In addition, exercise-training in human subjects led to higher metabolic rates and higher rates of protein turnover, including both catabolism and synthesis (Rodriguez et al., 2007). Thus, our data showing generally reduced abundances of amino acids in Winter of both species could potentially be consistent with higher winter protein turnover. Further study focusing on seasonal measurement of protein turnover, however, is required to definitively determine how amino acid levels in our study might be related to protein turnover. Moreover, if protein turnover varies between seasons, as we hypothesize, how such variation in protein turnover relates specifically to general winter increases in muscle mass in winter for small birds in cold climates (Swanson, 2010) is uncertain and will also require further study.
Integration of the metabolomic and transcriptomic data not only corroborated the patterns observed from metabolic profiling of pectoralis muscle but helped identify transcripts and pathways whose expression or function might be coordinately regulated in the winter-adaptive phenotype. For example, in chickadees, integrated pathway analyses of significant transcript-metabolite pairs indicated that the Winter correlated cluster was overrepresented with pathways of amino acid and metabolism of carbohydrates, amino acid biosynthesis, fatty acid and beta-oxidation, nicotinate and nicotinamide metabolism (Supplementary Table 5), whereas the Winter anti-correlated cluster was overrepresented with pathways of nucleotide and amino acid derivatives metabolism, lipid metabolism, and serotonin signaling (Supplementary Tables 6, 9). Interestingly for goldfinches, integrated pathway analyses highlighted that the Winter correlated cluster was overrepresented mainly with pathways of sphingolipid metabolism, nicotinate and nicotinamide metabolism (Supplementary Table 7), whereas the Winter anti-correlated cluster was overrepresented with pathways of lipid metabolism (Supplementary Tables 8, 10). Collectively, the overrepresented pathways explained the reduced levels of amino acids, TCA cycle intermediates, and glycolytic intermediates observed in the metabolic profiling of the two species. Most importantly, our combined metabolomic and transcriptomic analyses revealed other key components of the winter-adaptive response in these two species. Specifically, the Winter correlated cluster was overrepresented with pathways of nicotinate and nicotinamide metabolism and degradation; fatty acid and carnitine derivatives biosynthesis; and ornithine, spermidine, and spermine biosynthesis (Supplementary Table 5), whereas the Winter anti-correlated cluster was enriched for nicotinate nicotinamide metabolism, and, ornithine biosynthesis and metabolism, spermidine and spermine biosynthesis in chickadee. As noted earlier for goldfinches, the Winter correlated cluster was overrepresented mainly with pathways of sphingolipid metabolism, and nicotinate and nicotinamide metabolism. Collectively, these results suggest that both chickadees and goldfinches not only ramp up their metabolism of lipids, but also the biosynthesis and metabolism of nicotinamide and carnitine derivatives. Indeed, elevated levels of nicotinamide adenine dinucleotide (NADH) and nicotinamide riboside were observed in Winter for both species, with NADH levels significantly higher in Winter (Figure 9). The metabolism of nicotinate and nicotinamide is generally increased in various tissues under conditions associated with elevated metabolic rates in vertebrates. For example, increased temperatures promoted higher growth rates in juvenile sea bass, Dicentrachus labrax, which resulted in an increased metabolism of nicotinate and nicotinamide derivatives (Zhou et al., 2021). This type of metabolism was also increased during growth in fast-growing chicken (Gallus gallus) strains compared to slow-growing strains (Willson et al., 2018). Moreover, the metabolism of nicotinate and nicotinamide derivatives was significantly decreased in kidneys of old mice compared to young mice (Ahn et al., 2021). Dietary supplementation of nicotinamide riboside (an NAD+ precursor) improved mitochondrial function and rejuvenated muscle stem cells in aged mice (Zhang et al., 2016). These data suggest a general pattern of positive association between metabolism of nicotinate and nicotinamide derivatives and organismal metabolic capacities in vertebrates. Our data showed the abundances of nicotinate and nicotinamide derivatives increased during Winter in pectoralis muscle of both goldfinches and chickadees, and together with the reported elevation of metabolic rates during winter in these species (Cooper and Swanson, 1994; Liknes et al., 2002), our data are consistent with a positive relationship between nicotinate and nicotinamide metabolism and organismal metabolic capacity.
The metabolic profiling together with the integrated metabolomic and transcriptomic analyses revealed key components of the winter-adaptive response common to both black-capped chickadees and American goldfinches. During winter, the metabolism of lipids is increased with a concomitant increase of nicotinate and nicotinamide metabolism to increase the abundance of NAD+/NADH. Higher levels of NADH and nicotinamide riboside (Figure 8) likely are associated with increases in thermogenic capacity of their pectoralis muscle, the primary thermogenic organ in small birds. Nicotinamide adenine dinucleotide (NAD+) and its reduced form NADH are important molecules involved in energy production, function, regeneration, adaption to exercise, aging, stress response, and adaptation to environmental changes in muscles (Li et al., 2009; Goody and Henry, 2018; Xie et al., 2020; White and Schenk, 2022). In addition to the widely recognized roles of NAD+ as a coenzyme in energy metabolism, we hypothesize that it also serves to increase metabolic output during winter to increase thermogenic capacity in small birds via increased metabolism of lipids, which would require higher amounts of NAD+/NADH (Lutkewitte et al., 2019; Yamaguchi et al., 2019). Although the increased metabolic output of small passerines living in regions with seasonal temperature fluctuations are well documented (Swanson and Vézina, 2015; Li et al., 2017; Wang et al., 2019), the mechanisms that underpin this increase are not fully understood. Our results suggest that increased levels of NAD+/NADH and their derivatives are a potential mechanism to facilitate the increased metabolic output of the pectoralis muscle for thermogenesis during winter. Although mammalian cells can generate NAD+ de novo from dietary Trp via the kynurenine pathway or from the Preiss–Handler pathway from nicotinate (Goody and Henry, 2018; Xie et al., 2020), we are not aware if avian cells can also perform these pathways. However, we suspect that avian cells can generate NAD+ de novo from dietary Trp as the abundance of kynurenine, an intermediate of Trp metabolism (Martin et al., 2020) is lower during Winter for both species, significantly so for chickadees (Figure 7). Similar to mammalian cells, the salvage pathway is the likely primary route given that its precursor, nicotinamide riboside levels are elevated during Winter for both species, where levels were significantly higher in chickadees (Figure 8). Although decreases in amino acid abundances might reflect decreased dietary protein intake during winter, another possible explanation is that amino acids are being shuttled, as precursors to maintain a relatively higher level of nicotinate and nicotinamide metabolism. Notably, transport of small molecules and metabolism of amino acids were among the most overrepresented pathways in both species, coincident with the decline in the abundance of amino acids as indicated by the metabolic profiling [Figures 3A, 5; Li et al. (2009)]. Future studies should address a causal role for upregulation of the nicotinate and nicotinamide metabolic pathway in promoting elevated winter thermogenic capacities in small birds.
Our major findings regarding the metabolic changes during winter for both black-capped chickadees and American goldfinches can be summarized as a model with four main hypotheses: (1) Metabolism of lipids is the apparent main source of energy during winter; (2) reduced amino acid levels in winter are consistent with seasonal changes in protein turnover that might contribute to seasonal variation in muscle mass; (3) accumulated NADH and nicotinamide riboside should facilitate the increased metabolic output needed for thermogenesis during winter; and (4) increased biosynthesis and metabolism of nicotinates and nicotinamides is a potential mechanistic adaption underpinning seasonal metabolic flexibility. These hypotheses offer new plausible directions for further research into the mechanism of the winter-adaptive response in small passerine birds.
Data Availability Statement
The original contributions presented in the study are included in the article/Supplementary Material, further inquiries can be directed to the corresponding author/s.
Ethics Statement
The animal study was reviewed and approved by the University of South Dakota Institutional Animal Care and Use Committee.
Author Contributions
BW and DS designed, conducted the research, and wrote the manuscript. BW analyzed the data and integrated the transcriptomic and metabolomic data. Both authors reviewed, edited, and approved the final manuscript.
Funding
The metabolic profiling was supported by startup funds from the College of Arts and Sciences, University of South Dakota to BW. Support for the study was also provided by NSF-IOS 1021218 to DS.
Conflict of Interest
The authors declare that the research was conducted in the absence of any commercial or financial relationships that could be construed as a potential conflict of interest.
Publisher’s Note
All claims expressed in this article are solely those of the authors and do not necessarily represent those of their affiliated organizations, or those of the publisher, the editors and the reviewers. Any product that may be evaluated in this article, or claim that may be made by its manufacturer, is not guaranteed or endorsed by the publisher.
Supplementary Material
The Supplementary Material for this article can be found online at: https://www.frontiersin.org/articles/10.3389/fevo.2022.866130/full#supplementary-material
Footnotes
References
Ahn, E., Lee, J., Han, J., Lee, S.-M., Kwon, K.-S., and Hwang, G.-S. (2021). Glutathione if an aging-related metabolic signature in the mouse kidney. Aging 13:17. doi: 10.18632/aging.203509
Allen, D. C. (2019). Nutritional hotspots? Prey from one ecosystem provide key fatty acids required for consumers in multiple ecosystems. Funct. Ecol. 33, 1816–1817. doi: 10.1111/1365-2435.13436
Ayre, K. J., and Hulbert, A. J. (1997). Dietary fatty acid profile affects endurance in rats. Lipids 32, 1265–1270. doi: 10.1007/s11745-006-0162-5
Ayroles, J., Carbone, M., Stone, E., Jordan, K., Lyman, R., Magwire, M., et al. (2009). System genetics of complex traits in drosophila melanogaster. Nat. Genet. 41, 299–307.
Banerjee, P., Carmelo, V. A. O., and Kadarmideen, H. N. (2020). Integrative analysis of metabolomic and transcriptomic profiles uncovers biological pathways of feed efficiency in pigs. Metabolites 10:275. doi: 10.3390/metabo10070275
Bauchinger, U., Keil, J., McKinney, R. A., Starck, J. M., and McWilliams, S. R. (2010). Exposure to cold but not exercise increases carbon turnover rates in specific tissues of a passerine. J. Exp. Biol. 213, 526–534. doi: 10.1242/jeb.037408
Benjamini, Y., and Hochberg, Y. (1995). Controlling the false discovery rate: a practical and powerful approach to multiple testing. J. R. Statist. Soc. Ser. B 57, 289–300. doi: 10.1111/j.2517-6161.1995.tb02031.x
Carter, W. A., Whiteman, J. P., Cooper-Mullin, C., Newsome, S. D., and McWilliams, S. R. (2019). Dynamics of individual fatty acids in muscle fat stores and membranes of a songbird and it functional and ecological importance. Physiol. Biochem. Zool. 92, 239–251. doi: 10.1086/702667
Cheviron, Z. A., and Swanson, D. L. (2017). Comparative transcriptomics of seasonal phenotypic flexibility in two species of North american songbirds. Int. Comput. Biol. 57, 1040–1054. doi: 10.1093/icb/icx118
Collins, K. A., and Horn, D. J. (2012). The role of oil content and size in seed selection by wild birds. illinois state academy of science. Transactions 105:107.
Cooper, S. J., and Swanson, D. L. (1994). Seasonal acclimatization of thermoregulation in the black-capped chickadee. Condor 96, 638–646. doi: 10.2307/1369467
Dubois, K., Hallot, F., and Vézina, F. (2016). Basal and maximal metabolic rates differ in their response to rapid temperature change among avian species. J. Comput. Physiol. B 186, 919–935. doi: 10.1007/s00360-016-1001-5
Foote, J. R., Mennill, D. J., Ratcliffe, L. M., and Smith, S. M. (2020). “Black-capped chickadee (poecile atricapillus), version 1.0,” in Cornell Lab of Ornithology, ed. A. F. Poole (Ithaca, NY), doi: 10.2173/bow.bkcchi.01
Goody, M. F., and Henry, C. A. (2018). A need for NAD+ in muscle development, homeostasis, and aging. Skeletal Muscle 8, 1–14. doi: 10.1186/s13395-018-0154-1
Guglielmo, C. G. (2010). Move that fatty acid: fuel selection and transport in migratory birds and bats. Int. Comput. Biol. 50, 336–345. doi: 10.1093/icb/icq097
Guijas, C., Montenegro-Burke, J. R., Warth, B., Spilker, M. E., and Siuzdak, G. (2018). Metabolomics activity screening for identifying metabolites that modulate phenotype. Nat. Biotechnol. 36, 316–320.
Guil-Guerrero, J. L., Ramos-Bueno, R. P., González-Fernández, M. J., Fabrikov, D., Sánchez-Muros, M. J., and Barroso, F. G. (2018). Insects as food: fatty acid profiles, lipid classes, and sn-2 fatty acid distribution of lepidoptera larvae. Eur. J. Lip. Sci. Technol. 120:1700391. doi: 10.1002/ejlt.201700391
Hohtola, E. (1982). Thermal and electromyographic correlates of shivering thermogenesis in the pigeon. Comput. Biochem. Physiol. 73A, 159–166. doi: 10.1016/0300-9629(82)90049-4
Kamburov, A., Cavill, R., Ebbels, T. M., Herwig, R., and Keun, H. C. (2011). Integrated pathway-level analysis of transcriptomics and metabolomics data with IMPaLA. Bioinformatics 27, 2917–2918. doi: 10.1093/bioinformatics/btr499
King, M. O., Zhang, Y., Carter, T., Johnson, J., Harmon, E., and Swanson, D. L. (2015). Phenotypic flexibility of skeletal muscle and heart masses and expression of myostatin and tolloid–like proteinases in migrating passerine birds. J. Comput. Physiol. B 185, 333–342. doi: 10.1007/s00360-015-0887-7
Latimer, C. E., Cooper, S. J., Karasov, W. H., and Zuckerberg, B. (2018). Does habitat fragmentation promote climate-resilient phenotypes? Oikos 127, 1069–1080. doi: 10.1111/oik.05111
Lautenschläger, T., Neinhuis, C., Kikongo, E., Henle, T., and Förster, A. (2017). Impact of different preparations on the nutritional value of the edible caterpillar imbrasia epimethea from northern angola. Eur. Food Res. Technol. 243, 769–778. doi: 10.1007/s00217-016-2791-0
Le Pogam, A., O’Connor, R. S., Love, O. P., Drolet, J., Régimbald, L., Roy, G., et al. (2021). Snow buntings maintain winter-level cold endurance while migrating to the high arctic. Front. Ecol. Evol. 9:724876.
Li, M., Sun, Y., Mao, H., Xu, J., Zheng, W., and Liu, J. (2017). Seasonal phenotypic flexibility in body mass, basal thermogenesis, and tissue oxidative capacity in the male silky starling (sturnus sericeus). Avian Res. 8, 1–12.
Li, Y., Dash, R. K., Kim, J., Saidel, G. M., and Cabrera, M. E. (2009). Role of NADH/NAD+ transport activity and glycogen store on skeletal muscle energy metabolism during exercise: in silico studies. Am. J. Physiol. Cell Physiol. 296, C25–C46. doi: 10.1152/ajpcell.00094.2008
Liknes, E. T., Scott, S. M., and Swanson, D. L. (2002). Seasonal acclimatization in the american goldfinch revisited: to what extent to metabolic rates vary seasonally? Condor 104, 548–557. doi: 10.1093/condor/104.3.548
Liknes, E. T., and Swanson, D. L. (2011b). Phenotypic flexibility of body composition associated with seasonal acclimatization of passerine birds. J. Therm. Biol. 36, 363–370. doi: 10.1016/j.jtherbio.2011.06.010
Liknes, E. T., and Swanson, D. L. (2011a). Phenotypic flexibility in passerine birds: seasonal variation of aerobic enzyme activities in skeletal muscle. J. Therm. Biol. 36, 430–436.
Liu, M. (2015). Physiological and Ecological Measures of Stopover Habitat Quality For Migrant Birds In Natural Riparian Corridor Woodlands And Anthropogenic Woodlots In Southeastern South Dakota. Ph. D, Thesis. Vermillion: University of South Dakota.
Lutkewitte, A. J., Burgess, S. C., and Finck, B. N. (2019). Fatty acid desaturation gets a NAD+ reputation. Cell Metab. 29, 790–792. doi: 10.1016/j.cmet.2019.03.007
Maikhuri, R. K., Parshwan, D. S., Kewlani, P., Negi, V. S., Rawat, S., and Rawat, L. S. (2021). Nutritional composition of seed kernel and oil of wild edible plant species from western himalaya, India. Int. J. Fruit Sci. 21, 609–618. doi: 10.1080/15538362.2021.1907009
Marsh, R. L., and Dawson, W. R. (1989). “Avian adjustments to cold,” in Advances in Comparative and Environmental Physiology 4: Animal Adaptation to Cold, ed. L. C. H. Wang (New York: Springer), 205–253. doi: 10.1007/978-3-642-74078-7_6
Martin, K. S., Azzolini, M., and Ruas, J. L. (2020). The kynurenine connection: how exercise shifts muscle tryptophan metabolism and affects energy homeostasis, the immune system, and the brain. Am. J. Physiol. Cell Physiol. 318, C818–C830.
Martinez del Rio, C., and McWilliams, S. R. (2016). How essential fats affect bird performance and link aquatic ecosystems to terrestrial consumers. Proc. Natl. Acad. Sci. U.S.A. 113, 11988–11990. doi: 10.1073/pnas.1614106113
Mba, A. R. F., Kansci, G., Viau, M., Rougerie, R., and Genot, C. (2019). Edible caterpillars of Imbrasia truncata and Imbrasia epimethea contain lipids and proteins of high potential for nutrition. J. Food Composit. Analy. 79, 70–79. doi: 10.1016/j.jfca.2019.03.002
McGraw, K. J., and Middleton, A. L. (2020). “American goldfinch (Spinus tristis), version 1.0,” in Cornell Lab of Ornithology, ed. P. G. Rodewald (Ithaca, NY), doi: 10.2173/bow.amegfi.01
McKechnie, A. E. (2008). Phenotypic flexibility in basal metabolic rate and the changing view of avian physiological diversity: a review. J. Comput. Physiol. B 178, 235–247. doi: 10.1007/s00360-007-0218-8
McKechnie, A. E., Noakes, M. J., and Smit, B. (2015). Global patterns of seasonal acclimatization in avian resting metabolic rates. J. Ornithol. 156, S367–S376.
McWilliams, S. R., Guglielmo, C., Pierce, B., and Klaassen, M. (2004). Flying, fasting, and feeding in birds during migration: a nutritional and physiological ecology perspective. J. Avian. Biol. 35, 377–393. doi: 10.1111/j.0908-8857.2004.03378.x
Milbergue, M. S., Blier, P. U., and Vézina, F. (2019). Large muscles are beneficial but not required for improving thermogenic capacity in small birds. Sci. Rep. 8:14009. doi: 10.1038/s41598-018-32041-w
Morales, P. E., Bucarey, J. L., and Espinosa, A. (2017). Muscle lipid metabolism: role of lipid droplets and perilipins. J. Diab. Res. 2017. doi: 10.1155/2017/1789395
Nilsson, J. F., and Nilsson, J.-A. (2016). Fluctuating selection on basal metabolic rate. Ecol. Evolu. 6, 1197–1202. doi: 10.1002/ece3.1954
Patt, A., Siddiqui, J., Zhang, B., and Mathé, E. (2019). Integration of Metabolomics and Transcriptomics to Identify Gene-Metabolite Relationships Specific to Phenotype. New York, NY: Humana Press, 441–468.
Pedersen, B. K., and Febbraio, M. A. (2012). Muscles, exercise and obesity: skeletal muscle as a secretory organ. Nat. Rev. Endocrinol. 8, 457–465. doi: 10.1038/nrendo.2012.49
Peña-Villalobos, I., Nuñez-Villegas, M., Bozinovic, F., and Sabat, P. (2014). Metabolic enzymes in seasonally acclimatized and cold acclimated rufous-collared sparrow inhabiting a chilean mediterranean environment. Curr. Zool. 60, 338–350. doi: 10.1093/czoolo/60.3.338
Petit, M., Clavijo-Baquet, S., and Vézina, F. (2017). Increasing winter maximal metabolic rate improves intrawinter survival in small birds. Physiol. Biochem. Zool. 90, 166–177. doi: 10.1086/689274
Petit, M., Lewden, A., and Vézina, F. (2014). How does flexibility in body composition relate to seasonal changes in metabolic performance in a small passerine wintering at northern latitude? Physiol. Biochem. Zool. 87, 539–549. doi: 10.1086/676669
Petit, M., and Vézina, F. (2014). Phenotype manipulations confirm the role of pectoral muscles and haematocrit in avian maximal thermogenic capacity. J. Exp. Biol. 217, 824–830. doi: 10.1242/jeb.095703
Pierce, B. J., and McWilliams, S. R. (2014). The fat of the matter: how dietary fatty acids can affect exercise performance. Int. Comput. Biol. 54, 903–912. doi: 10.1093/icb/icu098
Pierce, B. J., McWilliams, S. R., O’Connor, T. P., Place, A. R., and Guglielmo, C. (2005). Effect of dietary fatty acid composition on depot fat and exercise performance in a migrating songbird, the red-eyed vireo. J. Exp. Biol. 208, 1277–1285. doi: 10.1242/jeb.01493
Poulos, A. (1995). Very long chain fatty acids in higher animals – a review. Lipids 30, 1–14. doi: 10.1007/BF02537036
Price, E. R., Staples, J. F., Milligan, C. L., and Guglielmo, C. G. (2011). Carnitine palmitoyl transferase activity and whole muscle oxidation rates vary with fatty acid substrate in avian flight muscles. J. Comput. Physiol. B 181, 565–573. doi: 10.1007/s00360-010-0542-2
Rodriguez, N. R., Vislocky, L. M., and Gaine, P. C. (2007). Dietary protein, endurance exercise, and human skeletal-muscle protein turnover. Curr. Opin. Clin. Nutr. Metab. Care 10, 40–45. doi: 10.1097/MCO.0b013e3280115e3b
Savva, C., Helguero, L. A., Gonzalez-Granillo, M., Melo, T., Couto, D., Buyandelger, B., et al. (2022). Maternal high-fat diet programs white and brown adipose tissue lipidome and transcriptome in offspring in a sex- and tissue-dependent manner in mice. Int. J. Obesity. [Epub ahead of print], doi: 10.1038/s41366-021-01060-5
Siddiqui, J. K., Baskin, E., Liu, M., Cantemir-Stone, C. Z., Zhang, B., Bonneville, R., et al. (2018). IntLIM: integration using linear models of metabolomics and gene expression data. BMC Bioinform. 19:1–12. doi: 10.1186/s12859-018-2085-6
Smith, R. J., Moore, F. R., and May, C. A. (2007). Stopover habitat along the shoreline of northern lake huron, michigan: emergent aquatic insects as a food resource for spring migrating landbirds. Auk 124, 107–121. doi: 10.1093/auk/124.1.107
Stager, M., Senner, N. R., Swanson, D. L., Carling, M. D., Eddy, D. K., Grieves, T. J., et al. (2021). Temperature heterogeneity correlates with intraspecific variation in physiological flexibility in a small endotherm. Nat. Commun. 12:4401. doi: 10.1038/s41467-021-24588-6
Stager, M., Swanson, D. L., and Cheviron, Z. A. (2015). Regulatory mechanisms of metabolic flexibility in the dark-eyed junco (Junco hyemalis). J. Exp. Biol. 218, 767–777. doi: 10.1242/jeb.113472
Storey, J. D. (2002). A direct approach to false discovery rates. J. R. Statist. Soc. Ser. B 64, 479–498. doi: 10.1111/1467-9868.00346
Swanson, D. L. (2001). Are summit metabolism and thermogenic endurance correlated in winter acclimatized passerine birds? J. Comparat. Physiol. B 171, 475–481. doi: 10.1007/s003600100197
Swanson, D. L. (2010). Seasonal metabolic variation in birds: functional and mechanistic correlates. Curr. Ornithol. 17, 75–129. doi: 10.1007/978-1-4419-6421-2_3
Swanson, D. L., Agin, T. J., Zhang, Y., Oboikovitz, P., and DuBay, S. (2020). Within-season temperature variability and metabolic flexibility in house sparrows. Integr. Org. Biol. 2:obaa039. doi: 10.1093/iob/obaa039
Swanson, D. L., King, M. O., and Harmon, E. (2014). Seasonal variation in pectoralis muscle and heart myostatin and tolloid-like proteins in small birds: a regulatory role for seasonal phenotypic flexibility? J. Comput. Physiol. B 184, 249–258. doi: 10.1007/s00360-013-0798-4
Swanson, D. L., and Liknes, E. T. (2006). A comparative analysis of thermogenic capacity and cold tolerance in small birds. J. Exp. Biol. 209, 466–474. doi: 10.1242/jeb.02024
Swanson, D. L., and Vézina, F. (2015). Environmental, ecological and mechanistic drivers of avian seasonal metabolic flexibility in response to cold winters. J. Ornithol. 156, S377–S388.
Swanson, D. L., Zhang, Y., and King, M. O. (2013). Individual variation in thermogenic capacity is correlated with flight muscle size but not cellular metabolic capacity in American goldfinches, spinus tristis. Physiol. Biochem. Zool. 86, 421–431. doi: 10.1086/671447
Tung, Y.-T., Hsu, C.-C., Liao, S. T., Huang Ho, C.-C., and Huang, W.-C. (2019). Physiological and biochemical effects of intrinsically high and low exercise capacities through multiomics approaches. Front. Physiol. 10:1201.
Twining, C. W., Brenna, J. T., Lawrence, P., Winkler, D. W., Flecker, A. S., and Hairston, N. G. Jr. (2019). Aquatic and terrestrial resources are not nutritionally reciprocal for consumers. Funct. Ecol. 33, 2042–2052. doi: 10.1111/1365-2435.13401
Twining, C. W., Parmar, T. P., Mathieu-Resuge, M., Kainz, M. J., Shipley, J. R., and Martin-Creuzburg, D. (2021). Use of fatty acids from aquatic prey varies with foraging strategy. Front. Ecol. Evol. 9:735350.
Twining, C. W., Shipley, J. R., and Winkler, D. W. (2018). Aquatic insects rich in omega-3 fatty acids drive breeding success in a widespread bird. Ecol. Lett. 21, 1812–1820. doi: 10.1111/ele.13156
Vaillancourt, E., Prud’Homme, S., Haman, F., Guglielmo, C. G., and Weber, J.-M. (2005). Energetics of a long-distance migrant shorebird (Philomachus pugnax) during cold exposure and running. J. Exp. Biol. 208, 317–325. doi: 10.1242/jeb.01397
Vézina, F., Ruhs, E. C., O’Connor, E. S., Le Pogam, A., Régimbald, L., Love, O. P., et al. (2020). Consequences of being phenotypically mismatched with the environment: rapid muscle ultrastructural changes in cold-shocked black-capped chickadees (Poecile atricapillus). Am .J. Physiol. Regul. Int. Comput. Physiol. 318, R274–R283. doi: 10.1152/ajpregu.00203.2019
Wang, Y., Shan, S., Zhang, H., Dong, B., Zheng, W., and Liu, J. (2019). Physiological and biochemical thermoregulatory responses in male chinese hwameis to seasonal acclimatization: phenotypic flexibility in a small passerine. Zool. Stud. 58:e6. doi: 10.6620/ZS.2019.58-06
Wells, M. E., and Schaeffer, P. J. (2012). Seasonality of peak metabolic rate in non-migrant tropical birds. J. Avian. Biol. 43, 481–485. doi: 10.1111/j.1600-048x.2012.05709.x
Wesner, J., Swanson, D. L., Dixon, M. D., Yager, L., Warmbold, J., Oddy, E., et al. (2020). Loss of potential aquatic-terrestrial subsidies along the missouri river floodplain. Ecosystems 23, 111–123. doi: 10.1007/s10021-019-00391-9
White, A. T., and Schenk, S. (2022). NAD+/NADH and skeletal muscle mitochondrial adaptations to exercise. Am. J. Physiol. Endocrinol. Metab. 303, E308–E321. doi: 10.1152/ajpendo.00054.2012
Willson, N.-L., Forder, R. E. A., Tearle, R., Williams, J. L., Hughes, R. J., Nattrass, G. S., et al. (2018). Transcriptional analysis of liver from chickens with fast (meat bird), moderate (F1 layer x meat bird cross) and low (layer bird) growth potential. BMC Genom. 19:309. doi: 10.1186/s12864-018-4723-9
Xie, N., Zhang, L., Gao, W., Huang, C., Huber, P. E., Zhou, X., et al. (2020). NAD+ metabolism: pathophysiologic mechanisms and therapeutic potential. Signal Trans. Targeted Ther. 5, 1–37. doi: 10.1038/s41392-020-00311-7
Yamaguchi, S., Franczyk, M. P., Chondronikola, M., Qi, N., Gunawardana, S. C., Stromsdorfer, K. L., et al. (2019). Adipose tissue NAD+ biosynthesis is required for regulating adaptive thermogenesis and whole-body energy homeostasis in mice. Proc. Natl. Acad. Sci. U.S.A. 116, 23822–23828. doi: 10.1073/pnas.1909917116
Zhang, H., Ryu, D., Wu, Y., Gariani, K., Wang, X., Luan, P., et al. (2016). NAD+ repletion improves mitochondrial and stem cell function and enhances life span in mice. Science 352, 1436–1443. doi: 10.1126/science.aaf2693
Zhang, Y., King, M. O., Harmon, E., and Swanson, D. L. (2015a). Summer-to-winter phenotypic flexibility of fatty acid transport and catabolism in skeletal muscle and heart of small birds. Physiol. Biochem. Zool. 88, 535–549. doi: 10.1086/682154
Zhang, Y., King, M. O., Harmon, E., Eyster, K., and Swanson, D. L. (2015b). Migration-induced variation of fatty acid transporters and cellular metabolic intensity in passerine birds. J. Comput. Physiol. B 185, 787–810. doi: 10.1007/s00360-015-0921-9
Zhang, Y., Carter, T., Eyster, K., and Swanson, D. L. (2015c). Acute cold and exercise training increase similar aspects of fatty acid transport and catabolism in house sparrows, Passer domesticus. J. Exp. Biol. 218, 3885–3893.
Zheng, W.-H., Li, M., Liu, J.-S., Shao, S.-L., and Xu, X.-J. (2014). Seasonal variation of metabolic thermogenesis in eurasian tree sparrows (Passer montanus) over a latitudinal gradient. Physiol. Biochem. Zool. 87, 704–718. doi: 10.1086/676832
Keywords: phenotypic flexibility, birds, seasonal-adaptative response, metabolomics, winter, NAD+ metabolism, nicotinate and nicotinamide metabolism pathway
Citation: Wone BWM and Swanson DL (2022) Metabolic Profiling and Integration of Metabolomic and Transcriptomic Data From Pectoralis Muscle Reveal Winter-Adaptive Metabolic Responses of Black-Capped Chickadee and American Goldfinch. Front. Ecol. Evol. 10:866130. doi: 10.3389/fevo.2022.866130
Received: 30 January 2022; Accepted: 16 March 2022;
Published: 12 April 2022.
Edited by:
Todd Jason McWhorter, University of Adelaide, AustraliaReviewed by:
Sheldon Cooper, University of Wisconsin–Oshkosh, United StatesWales Carter, Great Hollow Nature Preserve and Ecological Research Center, United States
Copyright © 2022 Wone and Swanson. This is an open-access article distributed under the terms of the Creative Commons Attribution License (CC BY). The use, distribution or reproduction in other forums is permitted, provided the original author(s) and the copyright owner(s) are credited and that the original publication in this journal is cited, in accordance with accepted academic practice. No use, distribution or reproduction is permitted which does not comply with these terms.
*Correspondence: Bernard W. M. Wone, YmVybmllLndvbmVAdXNkLmVkdQ==; David L. Swanson, ZGF2aWQuc3dhbnNvbkB1c2QuZWR1