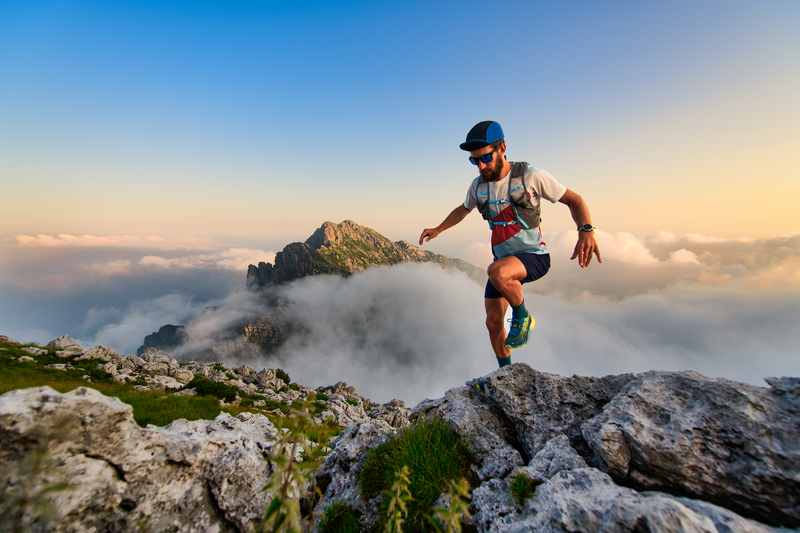
95% of researchers rate our articles as excellent or good
Learn more about the work of our research integrity team to safeguard the quality of each article we publish.
Find out more
ORIGINAL RESEARCH article
Front. Ecol. Evol. , 03 June 2022
Sec. Ecophysiology
Volume 10 - 2022 | https://doi.org/10.3389/fevo.2022.866102
This article is part of the Research Topic Avian Behavioral and Physiological Responses to Challenging Thermal Environments and Extreme Weather Events View all 12 articles
Small passerine birds in the north need to take advantage of several behavioral and physiological mechanisms to maintain energy balance during the winter characterized by low food supply, low ambient temperatures, and short days. Here we test if the breathing pattern of a non-migratory species, the great tit (Parus major), show seasonal variation that could help the species keeping a positive energy balance in the winter. To this aim, we measured oxygen consumption and ventilatory variables (tidal volume and respiratory frequency) in summer- and winter-acclimatized great tits exposed to ambient temperatures between –15 and 30°C. Winter-acclimatized great tits had a higher resting metabolic rate and a different breathing pattern compared to the summer-acclimatized birds. During the winter the great tits utilized a breathing pattern, consisting of an increased respiratory frequency to tidal volume ratio compared to summer-acclimatized birds at all temperatures. The higher oxygen uptake and the altered breathing pattern in the winter-acclimatized tits resulted in a higher lung oxygen extraction. However, during acute cold exposure neither the winter- nor summer-acclimatized great tits increased the oxygen extraction at low ambient temperature. The higher lung oxygen extraction in the winter-acclimatized tits implies that the birds will save on the minute ventilation, which reduces the evaporative water loss through respiration. The daily water loss saved can be more than 1 g of water per day. This is a substantial saving corresponding to a saving in evaporative heat loss corresponding to between 4 and 8% of the resting metabolic rate. This might be significant in keeping an energy balance, and the altered breathing pattern in the winter, ensuring an increased oxygen extraction, may therefore represents an additional physiological mechanism making it possible for small passerine birds to survive the northern winter.
Small passerine birds are, because of their high mass-specific metabolic rate and their high surface to volume ratio, especially vulnerable to environmental challenging conditions. Hence, those that are wintering at high latitudes, where they experience low ambient temperatures in combination with reduced food supply and only few hours of daylight, have evolved a suite of behavioral and physiological mechanisms to maintain an energetic balance (Irving, 1972; Dawson and Marsh, 1989; Dawson and O’Connor, 1996; Reinertsen, 1996; Bodin, 2007; Olson et al., 2010; Swanson, 2010; Swanson and Vézina, 2015). These mechanisms enable small passerine birds to be year-round resident even at very high latitudes.
One of the most important behavioral adaptations in northern birds keeping an energy balance during the winter, is the ability to hoard food. This has been described in a series of classical papers by Haftorn (1954, 1956a,1956b,1956c) for several small northern passerine species. Also, the ability to forage at lower light intensities has also been described as a way of increasing the total time during a day that can be used for foraging. This has been demonstrated in the arctic living redpolls (Carduelis flammea and C. hornemanni; Brooks, 1968; Pohl, 1989). Other ways of ensuring an energy balance during cold winter nights is to store more body fat. Hence many passerine birds increase their fat reserves during the winter, with the amount of fattening being directly influenced by the prevailing ambient temperatures (Gosler, 2002; Krams et al., 2010).
Another way of ensuring an optimal economic use of the available energy during the northern winter is to use nightly hypothermia (Reinertsen and Haftorn, 1986; Reinertsen, 1996; Cooper and Gessaman, 2005; Bodin, 2007). This involves a temporal decrease of the body temperature, thereby decreasing the temperature gradient from the body core to the environment, resulting in a reduced heat loss. This has been most clearly shown in the Willow tit (Poecile montanus), where the nightly body temperature may decrease to values of 33°C, with the decrease being dependent on the ambient temperature (Reinertsen and Haftorn, 1986).
One of the main characteristics of winter acclimatization of small passerines from the temperate region is an increased metabolic capacity. Hence, small birds wintering in the north typically increase their maximum metabolic capacity during cold exposure in the winter, a feature that has been demonstrated to increase the probability of survival (Petit et al., 2017). The increased thermogenetic capacity might be caused by increased muscle mass, muscle structure or aerobic enzyme activity, which drive an upregulation of the metabolic machinery of the whole body (Swanson, 2010; Liknes and Swanson, 2011; Petit et al., 2014; Jimenez et al., 2019). Even the mitochondria of the red blood cells have recently been shown to be more thermogenic in the winter, further adding to an increased heat producing capacity in small avian species living in the north (Nord et al., 2021).
In addition to these behavioral and physiological mechanisms, the respiratory system has, apart from being directly responsible for the uptake of oxygen, also been advocated as playing a potential role in cold acclimatization in vertebrates (Mortola and Frappell, 2000). It is normally assumed that for most animals an increased metabolic rate during cold exposure is directly followed by a proportional increased in lung ventilation (Mortola and Maskrey, 2011). However, in some species the increase in ventilatory volume is less than the increase in metabolic rate, implying that less air is ventilated for a given amount of oxygen taken up by the lungs. Such a decrease in the ventilation-oxygen uptake relationship (often in the range of 5–15%; Mortola and Maskrey, 2011), suggest an increased “efficiency” of the lung system during cold exposure, often expressed as an increased lung oxygen extraction (EO2). Because the evaporative heat loss is inevitably correlated to the volume of lung air being expired, an increased EO2 could be a mean of reducing the evaporative heat loss in the cold. Most studies investigating this have been conducted on birds experimentally exposed to decreased ambient temperatures, and mixed results have been reported. Although a few species actually increase EO2 when experimentally cold exposed (Bech et al., 1984; Brent et al., 1984; Johannesen and Nicol, 1990), most do not (e.g., Bech et al., 1985; Clemens, 1988; Bucher and Morgan, 1989; Morgan et al., 1992; Bech and Nicol, 1999). This also led Morgan et al. (1992) to conclude, after studying species living in the Antarctic, that increasing EO2, as a means of reducing ventilatory heat loss is not a general phenomenon in cold-adapted birds.
Although a changed lung efficiency may not play a large role during thermoregulating when acutely exposed to cold, it could still play a role on a more longer time scale such a seasonal acclimation to cold. Data to test this are, however, so far very scarce. Only two studies on birds have provided some evidence that there could be a seasonal effect on the ventilatory accommodation of oxygen uptake in the cold (Cooper and Same, 2000; Arens and Cooper, 2005b). It is therefore still an open question to what extent a change in lung ventilation play a role in the seasonal acclimatization of small northern birds, and no newer studies seem to have addressed this question.
The great tit (Parus major) is a stationary breeding species throughout most of Asia, North-West Africa, and Europe (Snow and Perrins, 1998). Being stationary throughout most of northern Europe, the species consequently experiences large seasonal changes in ambient temperatures, daylight, and availability of food. In the present study we asked the question, whether great tits, living close to the northern limit of their distribution, show seasonal changes in the ventilatory pattern and oxygen extraction, which may add to help keeping their energy balance. If changes in lung oxygen extraction is part of the physiological repertoire ensuring energy balance during the winter, we would expect to find increased values of EO2 in the winter-acclimatized great tits.
Birds used in the present study were all caught around Trondheim in mid Norway at 63°N. Average daily temperature in Trondheim is warmest in July with an average temperature of 14.8°C and coldest in February with an average of –1.2°C (Norwegian Centre for Climate Services [NCCS], 2021). However, temperatures down to –20°C during winter and up to 25°C in the summer are regularly recorded.
The summer-acclimatized (SA) individuals (N = 6) were captured and used during the period between 6 July and 16 September, while the winter-acclimatized (WA) individuals (N = 5) were caught and used between 30 November and 15 March. During captivity the birds were kept at ambient temperatures (Ta) and light-dark conditions which were close to the average outdoor conditions for the season. Hence, in the winter they were normally exposed to a Ta around –5°C, while in the summer Ta was normally kept between 15 and 20°C. Each bird had its own private cage (see Reinertsen, 1982, for a detailed description) with free access to food and water. The birds were mainly fed sunflower seeds with occasionally mealworms provided in addition. During the winter, at sub-zero ambient temperatures, they were daily given fresh snow as a source of water. To reduce the effect of captivity, each individual bird was kept in captivity for a maximum of 2 weeks, during which time all measurements were obtained. After the experiments all birds were released at the site of capture.
Metabolic rate was measured indirectly as rates of oxygen consumption obtained from birds resting in darkness during daytime, between 10:00 and 17:00 h. At the start of each run the birds were weighed and placed inside a 2.0 L metabolic chamber. The chamber had double walls through which an anti-freeze solution circulated, the temperature of which was controlled by a thermostat. An experimental run would last for 90–120 min, during which we normally would obtain values of VO2 and ventilatory parameters (see below) during the last 30 min. We calculated 10-min running averages of VO2 during the experiment, and the lowest value was used to represent the resting metabolic rate. The birds were not deprived of food prior to the metabolic measurements.
Oxygen consumption was measured by an open circuit respirometry system. Outside air was dried and pumped through the metabolic chamber (volume of 2,000 ml) at a constant flow of 250 ml/min. After removal of water vapor using silica-gel, a subsample of 100 ml/min of the excurrent air was directed through an O2 analyzer (Applied Electrochemistry S-3A), which measured fractional O2 content of the air. Reference measurements of incurrent air were taken at the beginning and at the end of each run. The actual air flow through the metabolic chamber was measured with a calibrated flowmeter (Flow control, model R-1, Applied Electrochemistry Inc., Sunnyvale, CA). The voltage output from the O2 analyzer was recorded at intervals of 30 s by a data logger (Grant Squirrel; type 1,200). Rates of oxygen consumption (VO2; ml O2/min) were calculated from the equation VO2 = VEx*(FiO2-FeO2)/[1-(1-RQ*FiO2)] (Lighton, 2008; Eq. 11.2), where FiO2 and FeO2 is the incurrent and excurrent oxygen fraction, respectively, and VEx is the measured excurrent air flow. The respiratory quotient (RQ) is assumed to be 0.8. Because of the relatively low airflow/volume ratio, FeO2 was calculated according to the instantaneous method (Bartholomew et al., 1981; Lighton, 2008) and used for calculating VO2. When calculating values of mass-specific oxygen consumption, we used the mean body mass obtained from measurements of body mass immediately before and after an experimental run.
Ambient temperature in the metabolic chamber was measured to the nearest 0.1°C using a copper-constantan thermocouple positioned in the outlet port of the chamber and connected to the Squirrel data logger. VO2 as well as ventilatory data were obtained at Ta’s between –15 and 30°C, guaranteeing measurements at thermoneutral conditions assumed to be near 25°C (Broggi et al., 2005). Birds were allowed 2–3 days in captivity before being used, and they were thereafter measured randomly at different Ta’s during the stay in captivity. In addition, on each experimental day only a single Ta was used for a given individual. Most birds were measured twice at each Ta and the average values were used in the subsequent analyses.
Simultaneously with the VO2 measurements, recordings of ventilation were made using the barometric method (Drorbaugh and Fenn, 1955; Mortola and Frappell, 2013). A differential pressure transducer (Lighton, 1988) was used to measure pressure changes inside the metabolic chamber. A computer recorded the voltage output of the pressure transducer at intervals of 0.02 s in bouts of 30 s, and values of VT and f were obtained from this period. During each run an injection of a known volume of air (0.50 ml) was used for calibration. The birds were always resting quietly and the oxygen uptake stable before any ventilation measurements were made. The mean amplitudes of the ventilation and calibration signals were used to obtain estimates of tidal volume (VT, ml BTPS) according to the formula given by Stahel and Nicol (1988). Ventilatory frequency (f, min–1) was obtained directly from the periodicity of the ventilation deflections. Minute volume (VE, ml min–1, expressed at BTPS conditions) was calculated as VT * f. The oxygen extraction efficiency (EO2,%; i.e., the amount of oxygen taken up as a percentage of the oxygen present in the inspired air) was calculated using the formula EO2 = (VO2*100)/(VE * FeO2), where VO2 is the oxygen uptake expressed as ml O2/min, VE is the minute ventilation expressed in STPD-conditions and FeO2 is the excurrent oxygen fraction from the metabolic chamber.
As an expression of the breathing pattern (BP), we chose to use the ratio f/VT (Milsom, 1989). Since the body mass changed significantly between seasons, and because tidal volume changes isometrically with body mass (de Souza et al., 2021), VT was expressed as mass-specific values, as volume of air (expressed at BTPS conditions) per 100 g body mass. Consequently, the BP was calculated as f/(VT/100 g).
Body temperature (Tb) was not measured during the metabolic experiments due to the stress this would impose on the birds. Consequently, in four birds from each season we measured body temperatures in separate experimental runs, which closely resembled the metabolic experiments except that the chamber was not completely sealed. This enabled us to remove the birds quickly and obtain a reading of Tb, usually within 60 s, using a Cu-Co-thermocouple inserted 10–15 mm into the rectum. Values of Tb were obtained in both WA and SA birds at four different ambient temperatures (–15, 0, 25, and 30°C). The overall mean Tb obtained from WA and SA individuals were used in calculating VT in WA and SA birds, respectively.
Each individual bird was often measured more than once at a given ambient temperature. Thus, for each individual bird a mean value for each parameter at each Ta was calculated and used in all statistical tests. Values are presented as mean ± 1 SD. For comparison of mean values, a t-test was used. For analyses of the effect of season on the relationship between ambient temperature and VO2 below thermoneutrality we used a one-way ANCOVA. Also, for analyzing whether season influenced the relationship between Ta and any respiratory parameter below thermoneutrality, we also use one-way ANCOVAs. In both cases body mass was included as a covariate and bird ID as a random factor. We used the same statistical approach when analyzing if season influenced how tidal volume or respiratory frequency influenced EO2. In the latter case data obtained at all temperatures between –15°C and 30°C were used. All statistical analyses were performed using IBM SPSS Statistics ver. 28 with the significance level set at 0.05, although we have adopted a more flexible use of this P-value threshold (Muff et al., 2022).
For each individual bird, an overall mean body mass was calculated. The mean body mass of the SA tits was 15.9 g (SD = 1.3 g, N = 6) and for the WA tits 17.9 g (SD = 1.0 g, N = 5). The body mass of the WA tits is higher than that of the SA tits (t-test, P = 0.025).
Body temperatures were measured in four WA and four SA great tits. The Tb was not related (P > 0.10) to Ta in either summer or winter. However, the mean Tb of WA birds (42.2 ± 0.4°C) was significantly higher (t-test, P < 0.001) than the mean Tb of SA birds (41.0 ± 0.6°C).
Within both SA and WA great tits there were no statistical differences between values of VO2 obtained at ambient temperatures of 20, 25, and 30°C. However, the values obtained at Ta of 15 and at 20°C differed significantly (P < 0.05) within both seasons. Consequently, we assumed the values obtained between 20 and 30°C to represent thermoneutral values and the mean oxygen consumption at these temperatures was used to represent the daytime resting metabolic rate (RMR). For WA tits thermoneutral VO2 was 5.03 ± 0.56 ml O2 g–1 h–1 and for the SA birds VO2 was 4.17 ± 0.37 ml O2 g–1 h–1. There was strong statistical evidence (P < 0.001; Table 1) that thermoneutral RMR was higher in the WA tits than in the SA tits.
Table 1. Mean values (± 1 SD) of resting oxygen (VO2) uptake and ventilatory parameters in summer- and winter-acclimatized great tits obtained at thermoneutrality, i.e., temperatures between 20 and 30°C (NS, non-significant).
Below the thermoneutral zone the oxygen consumption increase linearly with decreasing ambient temperature for both groups of birds (Figure 1A). The relationships are best described by the equations: VO2 (O2 g–1h–1, summer) = 7.016–0.143Ta (R2 = 0.79) and VO2 (O2 g–1h–1, winter) = 7.661–0.118Ta (R2 = 0.62). There is only weak statistical evidence that the slopes of the lines differ (ANCOVA, P = 0.051).
Figure 1. Oxygen consumption (VO2, A) and ventilatory parameters [(B) breathing pattern BP; (C) lung oxygen extraction EO2; (D) tidal volume VT; (E) respiratory frequency f; and (F) minute ventilation VE] as functions of ambient temperature in summer-acclimatized (open symbols) and winter-acclimatized (closed symbols) great tits. Shown are mean values with one SD at selected ambient temperature. Mean thermoneutral values and linear regression lines below thermoneutrality are shown for summer-acclimatized (stippled lines) and winter-acclimatized (solid lines) birds.
Thermoneutral values of respiratory parameters are shown in Table 1. Remarkably, the mean values of tidal volume obtained in the two seasons were practically identical (Table 1). Our data on respiratory frequency, on the other hand, show evidence of higher values in the winter than in summer (P < 0.01; Table 1). Hence, the breathing pattern showed a noticeable seasonal change, with the BP being significantly higher in the winter, due to a change solely in the breathing frequency. Despite the increase in respiratory frequency in the winter, we found no evidence that minute ventilation changed with season (P = 0.11; Table 1). That the great tits do not show a change in total ventilation volume between seasons, and yet had a much higher oxygen uptake in the winter, are evidence of a higher lung oxygen extraction in the WA birds during thermoneutral conditions (P < 0.05; Table 1).
When exposed to temperatures below the thermoneutral zone (TNZ), the total (minute) ventilation increased in both SA and WA birds due to an increase in both respiratory frequency and tidal volume (Figures 1D–F). However, the dependency of f or VT in increasing the minute ventilation varied between the seasons. While the respiratory frequency was lower at all Ta’s in the summer (Figure 1E), the opposite was the case for tidal volume (Figure 1D; ANCOVA, P < 0.001 in both cases). Hence, in both seasons the increase in total ventilation was mainly due to an increase in tidal volume. As a result, the WA and the SA great tits follow distinct trajectories for the relationship between f and VT when cold exposed (Figure 2). This also results in a clear difference in breathing pattern between season, with the WA great tits having a higher breathing pattern than the SA birds (Figure 1B). Using values for all tested ambient temperatures, season had a significant effect on the overall BP (ANCOVA; P < 0.001). There was strong evidence that Ta affected BP (P = 0.004) indicating a decrease in BP during cold exposure (Figure 1B). Season did not influence this decrease (P = 0.91 for the interaction season*Ta).
Figure 2. Relationship between respiratory frequency (presented as the respiratory time, i.e., the time between two respiratory cycles) and tidal volume in summer-acclimatized (open symbols) and winter-acclimatized (closed symbols) great tits. Each point represents (from thermoneutral temperatures (TNZ) through 10, 0, –5 to –15°C) the mean value at an experimental ambient temperature, except at TNZ which is the mean value obtained between 20 and 30°C. Lines show values of iso-ventilation, i.e., combinations of tidal volume and respiratory frequency producing a constant minute ventilation volume.
The thermoneutral values of EO2 differed significantly (P = 0.029) between SA and WA great tits, with a slightly higher mean lung oxygen extraction in the winter; 29.1 vs. 23.5% in the summer (Table 1). There was moderate evidence that EO2 decreased at lower ambient temperatures (ANCOVA; P = 0.021). However, the mean EO2 during cold exposure is significantly higher (ANCOVA; P < 0.001, Figure 1C) during the winter than during the summer (estimates of 27.73 and 20.35, respectively). The seasonal change in EO2 coincides with an overall change in breathing pattern, suggesting an association between the two variables. Indeed, there is very strong evidence of an overall relationship between BP and EO2 (Figure 3, linear regression: EO2 = 13.23 + 0.42*BP, R2 = 0.31, P < 0.001). Within both seasons there was a significant effect of changes in VT on EO2, with a decrease in lung oxygen extraction as tidal volume increased (Figure 4A). In both seasons the relationship between VT and EO2 was significant with the linear relationships being EO2 = 28.96–12.70*VT (R2 = 0.26, P < 0.001) for the summer and EO2 = 42.93–28.39*VT (R2 = 0.40, P < 0.001) for the winter. A significant interaction between season and VT (ANCOVA, P = 0.010) points to VT having different effects on EO2 depending on season. The increase in EO2 per unit decrease in tidal volume is thus higher in the winter. Respiratory frequency also affects EO2 significantly with a decrease in oxygen extraction as respiratory frequency increase (Figure 4B). Season does not influence this effect (ANCOVA; P = 0.87 for the interaction season*f). Overall, our results suggests that great tits during the winter, both at thermoneutrality and during cold exposure, have a higher lung oxygen extraction, caused by a seasonal change in their breathing pattern, with the winter-acclimatized tits breathing with a higher respiratory frequency to tidal volume ratio.
Figure 3. Relationship between breathing pattern and lung oxygen extraction (EO2) in summer- (open symbols) and winter-acclimatized (closed symbols) great tits. Each point shows the mean value for an individual bird at a single ambient temperature.
Figure 4. Relationship between tidal volume (A) and respiratory frequency (B) and lung oxygen extraction (EO2) in summer- (open symbols) and winter-acclimatized (closed symbols) great tits. Each point shows the mean value for an individual bird at a single ambient temperature. See text for statistics.
Our values of VO2 and ventilatory parameters at thermoneutral conditions are all higher than expected based on allometric equations (de Souza et al., 2021). This is to be expected since our birds were measured during daytime and were not in a postabsorptive phase, while allometric equations are based on “BMR-conditions.” Our values of thermoneutral VO2 are 55 and 22% higher than the expected BMR for winter- and summer-acclimatized tits, respectively. Likewise, all ventilatory parameters are similarly higher than expected, varying between only 1% (summer breathing frequency) and 42% (summer tidal volume). However, as all birds were treated alike during the experimental procedures, the relative changes described here should still be representative for what would be the natural response. In addition, the condition with a postabsorptive phase and thermoneutrality is rarely experienced by wild great tits, who during normal activity are more likely to experience conditions like that created during the experimental runs. Anyway, our results on VO2 conform to a general trend among high latitude birds in which there is a seasonal difference in BMR with a higher value during the winter (Swanson, 2010; McKechnie et al., 2015). Specifically in great tits, studies have demonstrated a higher metabolic rate in winter than in summer (e.g., Broggi et al., 2019). A higher resting metabolic rate in winter may indicate a need for higher metabolic capacity during the coldest months of the year (McKechnie et al., 2015).
The increase in minute ventilation with decreasing ambient temperature is a result of a simultaneous increase in both VT and f (Figures 1, 2), with a larger contribution from tidal volume in both seasons. This is similar to the results in two species of finches (Rosy finches Leucosticte arctoa and House finches Carpodacus mexicanus) studied during cold exposure (Clemens, 1988), as well as in many other bird species (Bernstein and Schmidt-Nielsen, 1974; Bucher, 1985; Kaiser and Bucher, 1985; Bech and Nicol, 1999). However, a few species mainly increase respiratory frequency in the cold (e.g., Bucher, 1981).
During acute cold exposure, the lung oxygen extraction of the great tits did not change, which are in accord with most other studies on the effect of cold exposure in birds (Bech et al., 1985; Clemens, 1988; Bucher and Morgan, 1989; Morgan et al., 1992; Bech and Nicol, 1999). This is also in line with most mammalian studies in which the accommodation of the increased oxygen uptake at low ambient temperatures are being met by ventilatory response rather than a change in EO2 (e.g., Chappell, 1992; Warnecke et al., 2010), although a few species increase EO2 in the cold (e.g., Chappell and Roverud, 1990).
Acclimatization to winter conditions, in contrast, elicited a generally higher lung oxygen extraction in the great tits irrespective of ambient temperatures. There are only two studies to which these results can be compared. In the house sparrow (Passer domesticus) exposed to low ambient temperatures Arens and Cooper (2005b) also reported a higher EO2 in winter-acclimatized individuals compared to summer-acclimatized individuals. The difference, however, was only observed during night-time. A stable EO2 regardless of season during BMR-conditions was confirmed in another study on the same species by the same authors (Arens and Cooper, 2005a). In another small passerine bird, the black-capped chickadee (Poephile atricapillus), winter-acclimatized individuals are also reported to have a significant higher EO2 during acute cold exposure compared to summer-acclimatized individuals (Cooper and Same, 2000), although EO2 at BMR-conditions apparently also did not differ between seasons. Our study therefore supports these few other studies in the demonstration of a higher EO2 during the winter compared to summer even at thermoneutral conditions.
Our results have demonstrated strong evidence of a negative association between tidal volume and lung oxygen extraction (Figure 4A). A similar “strong inverse correlation between EO2 and VT” was also described by Clemens (1988) for the two species of finches. Obviously, if tidal volume should be so small that the dead space contributes a large fraction of VT, EO2 would necessarily decrease. This indicates that there should be an optimal value of VT generating the highest oxygen extraction. Bucher (1985) advocated an interesting explanation for this, based on the assumptions that the bird lung has a constant volume with pulsative airflow (Maina, 2015; Powel, 2015). Bucher (1985) argued that an increase in VT above an optimal size would move the amount of air equal to the increase in VT past the gas exchanging tissue. The extraction of oxygen from this extra amount of air should therefore not be optimal. Under these circumstances, there should be a decrease in EO2 as the VT increases above the optimal size. This model was later challenged by Clemens (1988) who argued that the air flow through the avian parabronchial lungs was mainly continuous and not pulsative. More detailed studies on the avian lungs have demonstrated more complex structure with a “new” lung, the neopulmo, where air flow is indeed bidirectional, while being unidirectional in the “older” parabronchial parts of the lung (Maina, 2015; Powel, 2015). The distribution of the increased amount of inspired air within the avian lung with increased VT is still not known. Such detailed information could possibly explain the relationship between tidal volume and lung oxygen extraction. Also, one can only speculate on why the great tits are not utilizing another breathing pattern and consequently an increased lung oxygen extraction also in the summer. We can offer no answer to this. However, it is interesting that in a broader context, i.e., moving taxonomically from low metabolic animals to high metabolic animals the BP has been shown to increase in parallel to the increase in total ventilation (Milsom, 1989). Our results demonstrate that the same can be the case intraspecifically when species adapt to seasonal environmental changes.
How much would then the described seasonal change in breathing pattern, with a resulting increase in EO2, mean in saved evaporative water loss? Since the overall (both within and below TNZ) winter EO2 is 28.2% and summer EO2 is 21.4%, one can calculate the saving in volume of expired air in the winter, as the extra minute ventilation needed for uptake of the winter VO2 at –15°C (2.85 mlO2/min) assuming an EO2 of only 21.4%. Using the equation for EO2 we arrive at a saving of 19 ml/min. Using 51.1 mg/L as the saturated water content at a body temperature of 40°C (Hill et al., 2016), the saving of evaporative water loss (EWL) adds up to 57 mg H2O/h, which mean a potential saving of more than 1 g of water (body mass) per day. This is a substantial saving, considering that great tits may lose up to 2 g of body mass during a single night (Bednekoff et al., 1994). Using the value for the latent heat of vaporization at 40°C of 2.41 kJ/g H2O (Withers, 1992) the saved heat loss due to the reduced EWL amounts to 0.137 kJ/h, corresponding to 8% of our measured metabolic rate at thermoneutrality, or 4% of the metabolic rate at –15°C in winter-acclimatized great tits.
Although great tits may use mechanisms that alleviate their nightly energy loss through ventilation, such as lowering their body temperature (Reinertsen and Haftorn, 1986) and using overnight quarters with more suitable ambient temperatures (Velký et al., 2010), our calculation still demonstrate that increasing the efficiency of the lungs in winter could be of potential importance in keeping energy balance in the winter. Hence, together with food hoarding, the storing of fat, hypothermia, and a changed aerobic machinery, the altered breathing pattern, with a resultant change in oxygen extraction, may thus represents an additional physiological mechanism making it possible for small passerine birds to live through the cold and dark northern winter.
The raw data supporting the conclusions of this article will be made available by the authors, without undue reservation.
The animal study and the experimental conditions for birds at the department was reviewed and approved by the Norwegian Animal Research Authority (Permit number S-0028/01).
CM and CB designed and performed the experiments. CM wrote the first draft of the manuscript. CB wrote the final version with contributions from CM. Both authors contributed to the article and approved the submitted version.
This study received no specific funding, except that of the basic infrastructure provided by the Norwegian University of Science and Technology.
The authors declare that the research was conducted in the absence of any commercial or financial relationships that could be construed as a potential conflict of interest.
All claims expressed in this article are solely those of the authors and do not necessarily represent those of their affiliated organizations, or those of the publisher, the editors and the reviewers. Any product that may be evaluated in this article, or claim that may be made by its manufacturer, is not guaranteed or endorsed by the publisher.
Arens, J. R., and Cooper, S. J. (2005b). Seasonal and diurnal variation in metabolism and ventilation in house sparrows. Condor 107, 433–444. doi: 10.1093/condor/107.2.433
Arens, J. R., and Cooper, S. J. (2005a). Metabolic and ventilatory acclimatization to cold stress in House sparrows (Passer domesticus). Physiol. Biochem. Zool. 78, 579–589. doi: 10.1086/430235
Bartholomew, G. A., Vleck, D., and Vleck, C. M. (1981). Instantaneous measurements of oxygen consumption during pre-flight warm-up and post-flight cooling in sphingid and saturniid moths. J. Exp. Biol. 90, 17–32. doi: 10.1242/jeb.90.1.17
Bech, C., Johansen, K., Brent, R., and Nicol, S. (1984). Ventilation and circulatory changes during cold exposure in the pekin duck Anas platyrhynchos. Respir. Physiol. 57, 103–112. doi: 10.1016/0034-5687(84)90036-7
Bech, C., and Nicol, S. C. (1999). Thermoregulation and ventilation in the tawny frogmouth, Podargus strigoides: a low-metabolic avian species. Aust. J. Zool. 47, 143–153. doi: 10.1071/ZO98058
Bech, C., Rautenberg, W., and May, B. (1985). Ventilatory oxygen extraction during cold exposure in the pigeon (Columba livia). J. Exp. Biol. 116, 499–502. doi: 10.1242/jeb.116.1.499
Bednekoff, P. A., Biebach, H., and Krebs, J. (1994). Great tit fat reserves under unpredictable temperatures. J. Avian Biol. 25, 156–160. doi: 10.2307/3677035
Bernstein, M. H., and Schmidt-Nielsen, K. (1974). Ventilation and oxygen extraction in the crow. Respir. Physiol. 21, 393–401. doi: 10.1016/0034-5687(74)90069-3
Bodin, A. (2007). Theoretical models of adaptive energy management in small wintering birds. Philos. Trans. R. Soc. B 362, 1857–1871. doi: 10.1098/rstb.2006.1812
Brent, R., Pedersen, P. F., Bech, C., and Johansen, K. (1984). Lung ventilation and temperature regulation in the European coot Fulica atra. Physiol. Zool. 57, 19–25. doi: 10.1086/physzool.57.1.30155962
Broggi, J., Hohtola, E., Orell, M., and Nilsson, J. -Å (2005). Local adaptation to winter conditions in a passerine spreading north: a common-garden approach. Evolution 59, 1600–1603. doi: 10.1554/05-106
Broggi, J., Nilsson, J. F., Koivula, K., Hohtola, E., and Nilsson, J. -Å (2019). Mass or pace? Seasonal energy management in wintering boreal passerines. Oecologia 189, 339–351. doi: 10.1007/s00442-018-04332-6
Brooks, W. S. (1968). Comparative adaptations of the Alaskan redpolls to the Arctic environment. Wilson Bull. 80, 253–280.
Bucher, T. L. (1981). Oxygen consumption, ventilation and respiratory heat loss in a parrot, Bolborhynchus lineola, in relation to ambient temperature. J. Comp. Physiol. 142, 479–488. doi: 10.1007/BF00688979
Bucher, T. L. (1985). Ventilation and oxygen consumption in Amazona viridigenalis. A reappraisal of “resting” respiratory parameters in birds. J. Comp. Physiol. B 155, 269–276. doi: 10.1007/BF00687467
Bucher, T. L., and Morgan, K. R. (1989). The effect of ambient temperature on the relationship between ventilation and metabolism in a small parrot (Agapornis roseicollis). J. Comp. Physiol. B 159, 561–567. doi: 10.1007/BF00694380
Chappell, M. A. (1992). Ventilatory accommodation of changing oxygen demand in sciurid rodents. J. Comp. Physiol. B 162, 722–730. doi: 10.1007/BF00301622
Chappell, M. A., and Roverud, R. C. (1990). Temperature effects on metabolism, ventilation, and oxygen extraction in a neotropical bat. Respir. Physiol. 81, 401–412. doi: 10.1016/0034-5687(90)90120-n
Clemens, D. T. (1988). Ventilation and oxygen consumption in Rosy Finches and House Finches at sea level and high altitude. J. Comp. Physiol. B 158, 57–66. doi: 10.1007/BF00692729
Cooper, S. J., and Gessaman, J. A. (2005). Nocturnal hypothermia in seasonally acclimatized mountain chickadees and juniper titmice. Condor 107, 151–155. doi: 10.1093/condor/107.1.151
Cooper, S. J., and Same, D. R. (2000). Ventilatory accommodation under cold stress in seasonally acclimatized black-capped chickadees. Am. Zool. 40:980A. doi: 10.1093/icb/40.6.925
Dawson, W. R., and Marsh, R. L. (1989). “Metabolic acclimatization to cold and season in birds,” in Physiology of Cold Adaptation in Birds, eds C. Bech and R. E. Reinertsen (New York: Plenum Press), 83–94. doi: 10.1007/978-1-4757-0031-2_9
Dawson, W. R., and O’Connor, T. P. (1996). “Energetic features of avian thermoregulatory responses,” in Avian Energetics and Nutritional Ecology, ed. C. Carey (London, UK: Chapman & Hall), 85–124. doi: 10.1007/978-1-4613-0425-8_4
de Souza, R. B. B., Bonfim, V. M. G., Rios, V. P., and Klein, W. (2021). Allometric relations of respiratory variables in Amniota: effects of phylogeny, form, and function. Comp. Biochem. Physiol. 252:110845. doi: 10.1016/j.cbpa.2020.110845
Drorbaugh, J. E., and Fenn, W. O. (1955). A barometric method for measuring ventilation in newborn infants. Pediatrics 16, 81–87. doi: 10.1542/peds.16.1.81
Gosler, A. G. (2002). Strategy and constraint in the winter fattening response to temperature in the great tit Parus major. J. Anim. Ecol. 71, 771–779. doi: 10.1046/j.1365-2656.2002.00642.x
Haftorn, S. (1954). Contributions to the food biology of tits especially about storing of surplus food. Part I. The crested tit (Parus c. cristatus L.). Kgl. Norske Vidensk. Selsk. Skrifter 4, 1–122.
Haftorn, S. (1956a). Contributions to the food biology of tits especially about storing of surplus food. Part II. The coal-tit (Parus ater L.). Kgl. Norske Vidensk. Selsk. Skrifter 2, 1–52.
Haftorn, S. (1956b). Contributions to the food biology of tits especially about storing of surplus food. Part III. The willow tit (Parus atricapillus L.). Kgl. Norske Vidensk. Selsk. Skrifter 3, 1–80.
Haftorn, S. (1956c). Contributions to the food biology of tits especially about storing of surplus food. Part IV, A comparative analysis of Parus atricapillus L., P. cristatus L. and P. ater L. Kgl. Norske Vidensk. Selsk. Skrifter 4, 1–54.
Hill, R. W., Wyse, G. W., and Anderson, M. (2016). Animal Physiology, Fourth edition. Massachusetts: Sinauer Associates, Inc. Publishers.
Irving, L. (1972). Arctic life of birds and mammals. Berlin: Springer Verlag, doi: 10.1007/978-3-642-85655-6
Jimenez, A. G., O’Connor, E. S., Brown, K. J., and Briggs, C. W. (2019). Seasonal muscle ultrastructure plasticity and resistance of muscle structural changes during temperature increases in resident black-capped chickadees and rock pigeons. J. Exp. Biol. 222:jeb201855. doi: 10.1242/jeb.201855
Johannesen, H., and Nicol, S. C. (1990). Effects of cold exposure on oxygen consumption, ventilation and interclavicular air-sac gases in the Little penguin (Eudyptula minor). J. Exp. Biol. 154, 397–405. doi: 10.1242/jeb.154.1.397
Kaiser, T. J., and Bucher, T. L. (1985). The consequences of reverse sexual size dimorphism for oxygen consumption, ventilation, and water loss in relation to ambient temperature in the Prairie falcon. Falco mexicanus. Physiol. Zool. 58, 748–758. doi: 10.1086/physzool.58.6.30156078
Krams, I., Cirule, D., Suraka, V., Krama, T., Rantala, M., and Ramey, G. (2010). Fattening strategies of wintering great tits support the optimal body mass hypothesis under conditions of extremely low ambient temperature. Funct. Ecol. 24, 172–177. doi: 10.1111/j.1365-2435.2009.01628.x
Lighton, J. R. B. (1988). A simpel, sensitive and versatile solid-state pressure transducer. J. Exp. Biol. 134, 429–433. doi: 10.1242/jeb.134.1.429
Lighton, J. R. B. (2008). Measuring metabolic rates. A manual for scientists. New York: Oxford University Press, doi: 10.1093/acprof:oso/9780195310610.001.0001
Liknes, E. T., and Swanson, D. L. (2011). Phenotypic flexibility in passerine birds: seasonal variation of aerobic enzyme activities in skeletal muscle. J. Therm. Biol. 36, 430–436. doi: 10.1016/j.jtherbio.2011.07.011
Maina, J. N. (2015). The design of the avian respiratory system: development, morphology and function. J. Ornithol. 156, 41–63. doi: 10.1007/s10336-015-1263-9
McKechnie, A. E., Noakes, M. J., and Smit, B. (2015). Global patterns of seasonal acclimatization in avian resting metabolic rates. J. Ornithol. 156, 367–376. doi: 10.1111/j.1365-2435.2009.01646.x
Milsom, W. K. (1989). “Comparative aspects of vertebrate pulmonary mechanics,” in Comparative Pulmonary Physiology, ed. S. C. Wood (New York and Basel: Marcel Dekker), 587–619.
Morgan, K. R., Chappell, M. A., and Bucher, T. L. (1992). Ventilatory oxygen extraction in relation to ambient temperature in four Antarctic seabirds. Physiol. Zool. 65, 1092–1113. doi: 10.1086/physzool.65.6.30158270
Mortola, J. P., and Frappell, P. B. (2000). Ventilatort responses to changes in temperature in mammals and other vertebrates. Annu. Rev. Physiol. 62, 847–874. doi: 10.1146/annurev.physiol.62.1.847
Mortola, J. P., and Frappell, P. B. (2013). Measurements of air ventilation in small vertebrates. Respir. Physiol. Neurol. 186, 197–205. doi: 10.1016/j.resp.2013.02.001
Mortola, J. P., and Maskrey, M. (2011). Metabolism, temperature, and ventilation. Compr. Physiol. 1, 1679–1709. doi: 10.1002/cphy.c100008
Muff, S., Nilsen, E. B., O’Hare, R. B., and Nater, C. R. (2022). Rewriting results sections in the language of evidence. Trends Ecol. Evol. 37, 203–210. doi: 10.1016/j.tree.2021.10.009
Nord, A., Metcalfe, N. B., Page, J. L., Huxtable, A., McCafferty, D. J., and Dawson, N. J. (2021). Avian red blood cell mitochondria produce more heat in winter than in autumn. FASEB J. 35:e21490. doi: 10.1096/fj.202100107R
Norwegian Centre for Climate Services [NCCS] (2021). Observations and Weather Statistics. Available Online at: https://seklima.met.no/observations [accessed September 10, 2021].
Olson, J. R., Cooper, S. J., Swanson, D. L., Braun, M. J., and Williams, J. B. (2010). The relationship of metabolic performance and distribution in Black-capped and Carolina chickadees. Physiol. Biochem. Zool. 83, 263–275. doi: 10.1086/648395
Petit, M., Clavijo-Baquet, S., and Vezina, F. (2017). Increasing winter maximal metabolic rate improves intrawinter survival in small birds. Physiol. Biochem. Zool. 90, 166–177. doi: 10.1086/689274
Petit, M., Lewden, A., and Vezina, F. (2014). How does flexibility in body composition relate to seasonal changes in metabolic performance in a small passerine wintering at northern latitude? Physiol. Biochem. Zool. 87, 539–549. doi: 10.1086/676669
Pohl, H. (1989). “Survival strategies of high latitude resident redpolls,” in Thermal Physiology 1989, ed. J. B. Mercer (Amsterdam: Excerpta Medica), 713–718.
Powel, F. L. (2015). “Respiration,” in Sturkie’s Avian Physiology, ed. C. G. Scanes (Amsterdam: Academic Press), 301–336.
Reinertsen, R. E. (1982). Radio telemetry measurements of deep body temperature of small birds. Ornis Scand. 13, 11–16. doi: 10.2307/3675967
Reinertsen, R. E. (1996). “Physiological and ecological aspects of hypothermia,” in Avian Energetics and Nutritional Ecology, ed. C. Carey (London, UK: Chapman & Hall), 125–157. doi: 10.1007/978-1-4613-0425-8_5
Reinertsen, R. E., and Haftorn, S. (1986). Different metabolic strategies of northern birds for nocturnal survival. J. Comp. Physiol. B 156, 655–663. doi: 10.1007/BF00692743
Snow, D. W., and Perrins, C. M. (1998). The Birds of the Western Palearctic. Concise Edition. Oxford: Oxford University Press.
Stahel, C. D., and Nicol, S. C. (1988). Comparison of barometric and pneumotachographic measurements of resting ventilation in the Little penguin (Eudyptula minor). Comp. Biochem. Physiol. 89, 387–390. doi: 10.1016/0300-9629(88)91045-6
Swanson, D. L. (2010). Seasonal metabolic variation in birds: functional and mechanistic correlates. Curr. Ornithol. 17, 75–129. doi: 10.1007/978-1-4419-6421-2_3
Swanson, D. L., and Vézina, F. (2015). Environmental, ecological and mechanistic drivers of avian seasonal metabolic flexibility in response to cold winters. J. Ornithol. 156, 377–388. doi: 10.1007/s10336-015-1192-7
Velký, M., Kanuch, P., and Kristín, A. (2010). Selection of winter roosts in the great tit Parus major: influence of microclimate. J. Ornithol. 151, 147–153. doi: 10.1007/s10336-009-0436-9
Warnecke, L., Cooper, C. E., Geiser, F., and Withers, P. C. (2010). Environmental physiology of a small marsupial inhabiting arid floodplains. Comp. Biochem. Physiol. A 157, 73–78. doi: 10.1016/j.cbpa.2010.04.018
Keywords: great tit (Parus major), lung oxygen extraction, metabolic rate, breathing pattern, seasonal acclimatization, thermoregulation
Citation: Bech C and Mariussen C (2022) Breathing in the Cold: Seasonal Changes in the Ventilatory Pattern in a Small Boreal Passerine Bird. Front. Ecol. Evol. 10:866102. doi: 10.3389/fevo.2022.866102
Received: 30 January 2022; Accepted: 05 May 2022;
Published: 03 June 2022.
Edited by:
Andrew McKechnie, University of Pretoria, South AfricaReviewed by:
Sheldon Cooper, University of Wisconsin–Oshkosh, United StatesCopyright © 2022 Bech and Mariussen. This is an open-access article distributed under the terms of the Creative Commons Attribution License (CC BY). The use, distribution or reproduction in other forums is permitted, provided the original author(s) and the copyright owner(s) are credited and that the original publication in this journal is cited, in accordance with accepted academic practice. No use, distribution or reproduction is permitted which does not comply with these terms.
*Correspondence: Claus Bech, Y2xhdXMuYmVjaEBudG51Lm5v
Disclaimer: All claims expressed in this article are solely those of the authors and do not necessarily represent those of their affiliated organizations, or those of the publisher, the editors and the reviewers. Any product that may be evaluated in this article or claim that may be made by its manufacturer is not guaranteed or endorsed by the publisher.
Research integrity at Frontiers
Learn more about the work of our research integrity team to safeguard the quality of each article we publish.