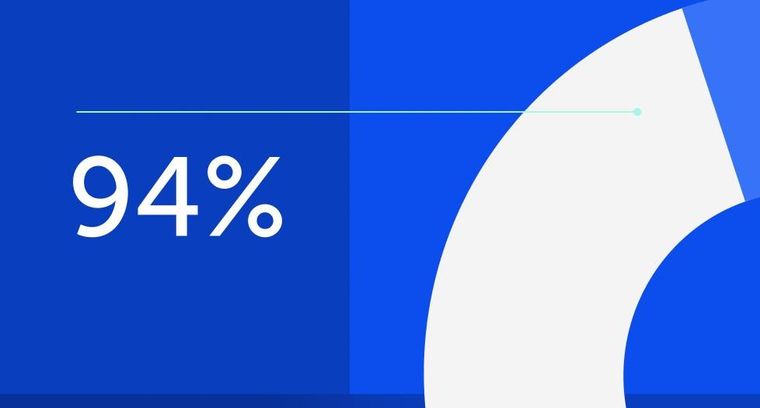
94% of researchers rate our articles as excellent or good
Learn more about the work of our research integrity team to safeguard the quality of each article we publish.
Find out more
ORIGINAL RESEARCH article
Front. Ecol. Evol., 13 June 2022
Sec. Behavioral and Evolutionary Ecology
Volume 10 - 2022 | https://doi.org/10.3389/fevo.2022.850612
This article is part of the Research TopicNesting in Reptiles: Natural and Anthropogenic Threats and Evolutionary ResponsesView all 18 articles
Sea turtle egg relocation and hatchery incubation (hereafter termed ex situ incubation) is an effective strategy to protect clutches when in situ egg incubation is not viable. Nevertheless, it negatively affects the ontogenesis of male gonads and brain areas homologous to the mammalian hippocampus, as well as body size and fitness. Thus, it is imperative to analyze the effects of ex situ incubation on other developmental aspects and extend these observations to females. This work evaluated the effect of ex situ management on neurogenesis (cell proliferation in the dorsal and medial ventricular zones, neuronal integration in the dorsomedial and medial cortices), ovary cell proliferation, body size (mass and length) and self-righting ability. Additionally, this study examined if the incubation microenvironment is different between in situ and ex situ nests and whether it could contribute to explain the biological traits. An analysis of principal components showed differences in biological variables of hatchlings between in situ and ex situ clutches, driven by contrasting temperatures and silt composition. Each biological variable was also analyzed with linear mixed models using in situ vs. ex situ clutches, abiotic variables and their interaction. Turtles from ex situ clutches showed: (1) fewer proliferating cells in the dorsal and medial ventricular zones; (2) less mature neurons in the dorsomedial and medial cortices; (3) ovaries with a lesser number of proliferating cells; (4) lower body mass and length at emergence; and (5) slower self-righting time. Together, the results suggest that ex situ incubation in hatcheries is related to a slowing down of neurogenesis, ovariogenesis, body size and self-righting ability in hatchlings. Future studies should evaluate the effect of ex situ incubation on cognitive and reproductive performance to understand the long-term consequences of altered organogenesis. These studies should also disentangle the differential contribution of egg movement, reburial, nesting environment and parental origin to development. This information would likely result in better conservation strategies for sea turtles.
Natural sea turtle populations are threatened globally (IUCN, 2020). Conservation efforts implemented in the last decades have gradually managed to mitigate the loss of some populations with scarce effect on others (Mazaris et al., 2017). One of the most broadly employed strategies is the relocation of endangered eggs to protected hatcheries. Eggs continue their development in ex situ nests constructed near the original site selected by the mother. This strategy has shown considerable success and may be the only chance for survival in some species of major concern (Blanck and Sawyer, 1981; Heppell et al., 2005, 2007; Mazaris et al., 2017).
However, several studies have reported negative effects associated to ex situ protection such as diminished hatching success, incomplete yolk resorption, sexual ratio bias, low body mass or length and reduced locomotor performance at emergence (Limpus et al., 1979; Eckert and Eckert, 1990; Pintus et al., 2009; Maulany et al., 2012; McElroy et al., 2015; Ahles and Milton, 2016; Tanabe et al., 2020). Recent studies evaluated the impact of the ex situ incubation strategy on organogenesis and proposed potential mechanisms of action for its negative effects (Sönmez et al., 2011; Herrera-Vargas et al., 2017).
Some studies explored the causal effect of abiotic variables on developmental traits (Sieg et al., 2011). A chief variable is nest temperature (Telemeco et al., 2013), which modifies incubation duration (Merchant-Larios et al., 1997; Booth and Astill, 2001a,b), sex determination (Reece et al., 2002), immune competence (Freedberg et al., 2008; Dang et al., 2015; Robledo-Avila et al., 2022) and brain development in reptiles (Amiel and Shine, 2012; Paredes et al., 2016; Amiel et al., 2017; Sanger et al., 2018). Another important factor is nest moisture, which alters sex ratio, incubation duration and embryo growth (Wallace et al., 2004; Patino-Martínez et al., 2012; Lolavar and Wyneken, 2020; Tezak et al., 2020). Successful sea turtle embryo development also depends on substrate composition, which could affect gas exchange and water retention, as well as the incubation period and sex-ratio (Ackerman, 1977; Lutcavage et al., 1997; Mitchell and Janzen, 2019).
The brain is a plastic organ; its early development is affected by external factors, such as temperature and moisture in ectotherms (Coomber et al., 1997; Beltrán et al., 2021; Sanger et al., 2021). This is especially true for the hippocampus, a structure that participates in cognitive tasks such as spatial learning and memory (Suh et al., 2011; Bannerman et al., 2014), as well as in the regulation of the hypothalamic-pituitary-adrenal axis (Sapolsky et al., 1983, 1984). Anatomical and genetic results suggest that the reptilian dorsomedial cortex is homologous to the mammalian CA1/CA3 hippocampal regions; whereas the medial cortex corresponds to the dentate gyrus (Medina et al., 2017; Tosches et al., 2018). Development of these cortices results from proliferation of neural precursors in the dorsal and medial walls of the lateral ventricles (Butler and Hodos, 2005; Medina et al., 2017; McDonald and Vickaryous, 2018; Tosches et al., 2018).
Proliferation is the earliest step in neurogenesis, which is followed by migration, differentiation, and integration of these new cells into the neural tissue (Altman and Das, 1965; Gage, 2002; Bayer and Altman, 2004; Kuhn et al., 2016). In mammals, early adverse environmental conditions can induce long-lasting brain alterations, including reduced hippocampal neurogenesis (Lajud and Torner, 2015; Vaiserman and Koliada, 2017). These alterations could result in lifelong cognitive and affective deficits, such as learning and memory disabilities, as well as anxiety-like or depressive-like behaviors (Heim and Nemeroff, 2002; McEwen, 2012; Lajud and Torner, 2015; Vaiserman and Koliada, 2017). Interestingly, species-specific non-optimal incubation temperatures decrease hippocampal neurogenesis in lizards, producing spatial learning disabilities (Amiel and Shine, 2012; Amiel et al., 2017; Dayananda and Webb, 2017). Likewise, early non-optimal conditions produce anxiety-like behaviors in lizards (Trnik et al., 2011), suggesting that reduced hippocampal neurogenesis during critical time windows may alter cognitive and behavioral traits in both mammals and reptiles.
Similarly, early environmental conditions also influence the development of the gonad. Its differentiation in reptiles displays the same progression as in other vertebrates and is highly sensitive to environmental variables such as temperature (Yntema and Mrosovsky, 1981; Ge et al., 2018; Weber et al., 2020). In humans and mice, altered seminiferous cord formation is associated with infertility, dysgenesis, and sexual development disorders (Chen and Liu, 2016). Likewise, disturbed oogenesis and folliculogenesis is related to reduced follicle reserves and infertility (Holm et al., 2016).
Motor abilities such as self-righting, crawling, and swimming speed constitute developmental traits associated with microenvironmental incubatory conditions like temperature and grain size. Better performance has been associated with cooler incubation temperatures and medium grain sand (Stewart et al., 2019; Reboul et al., 2021). Since reduced motor skills increase predation risk for newly emerged hatchlings during their displacement on land and sea, these traits constitute a good indicator of physical fitness and survival during this period. Indeed, these abilities may be good indicators of non-optimal incubation conditions in sea turtles (Fleming et al., 2020; Martins et al., 2020).
Ex situ incubation may alter neural and gonadal development in male Lepidochelys olivacea hatchlings by reducing the cell size and dendritic arborization of dorsomedial cortical neurons, as well as the number of epithelial cells per seminiferous cord (Herrera-Vargas et al., 2017). The functional relevance of these alterations is unknown, but results in mammals and lizards suggests that defective differentiation of hippocampal neurons and the seminiferous cord causes cognitive and reproductive problems (see above). Studies in rodents suggest that males are more vulnerable to developmental disruption (Dimatelis et al., 2015; Cowan and Richardson, 2018; de Melo et al., 2018), but in ectotherms some studies associate better phenotypes with male-producing environmental conditions (Freedberg et al., 2008).
Neurogenesis, ovariogenesis and motor performance are vital for the survival of sea turtle populations. These processes are highly sensitive to early adverse environmental stimuli. Ex situ incubation in hatcheries involves the early handling of eggs, as well their relocation to hatcheries. Moreover, nesting beaches include microenvironments that vary in moisture (depending on distance to the shoreline or vegetation), substrate composition, microbial load, and temperature (Stewart et al., 2019). All these variables could contribute to create non-optimal conditions for suitable development of turtle hatchlings. Thus, it is necessary to evaluate the effects of ex situ incubation in female turtles to determine if early adverse conditions have sex-specific effects on developmental traits. Moreover, it is imperative to identify the environmental variables likely modifying these developing traits in sea turtles. To test these hypotheses, this study evaluated the effects of ex situ incubation on hippocampal neurogenesis (cell proliferation in the dorsal and medial ventricular zones, as well as integration of newborn neurons into the dorsomedial and medial cortices), ovariogenesis (cell proliferation), body size (mass and length), and self-righting ability in L. olivacea females at emergence. Furthermore, this work analyzed the contribution of several nest-related abiotic variables (sand temperature, moisture, and grain size) to identify the most relevant variables for hatchling development. The results will contribute to determine the impact of ex situ incubation in hatcheries on female organogenesis and to identify some of the variables determining the altered phenotype observed in turtles incubated in hatcheries.
This study was conducted at the Centro de Protección de la Tortuga Marina in Boca Seca beach, located in Lázaro Cárdenas, Michoacán, México (18° 04′ N, 102° 58′ W; Figure 1). Egg manipulation was kept to the bare minimum and done by the hatchery staff according to protocols stated in Mexican regulation (NOM-162-SEMARNAT, 2014) and a previous report (Herrera-Vargas et al., 2017). Briefly, beach patrolling during three consecutive nights (September 13–15, 2017) allowed identification of nesting females. Ten nests located approximately in the same beach zone (30–60 m away from the shoreline) were chosen and sheltered immediately after the female turtle covered the eggs and left the site. Five randomly selected natural nests remained undisturbed in situ, only fenced with cyclone mesh until hatchling emergence. The complete clutch of the other five nests was carefully collected as soon as the female left the nest, placed in individual plastic bags and transported to the hatchery. There, the eggs were immediately buried in previously built nests and remained undisturbed until emergence. This ensured that conditions related to clutch size (e.g., oxygen availability, temperature, metabolic heat, etc.) remained unaltered. The total time between laying and re-burial lasted less than 2 h. Efforts were made to avoid egg rotation and excessive handling, as well as to emulate natural nest architecture in hatcheries. Ex situ nests were constructed by the hatchery staff according to international norms for L. olivacea, with a narrow neck (20–25 cm) and a wider flask-shaped bottom, at a depth of 40–50 cm and 1 m separation between them (Kutzari, 2006). Nests from both conditions were not shaded or watered. Forty days after incubation started, the hatchery clutches were fenced with cyclone mesh until turtle emergence. This experimental design ensured that in situ nests were not disturbed and that clutches relocated to hatcheries only went through the routine procedures done by the hatchery staff. Egg handling was performed before organogenesis started (Miller, 1985).
Figure 1. Location of the study site and nesting conditions. (A) Map showing Lázaro Cárdenas nesting beaches in Michoacán, México. (B) Aerial photograph of ex situ (dotted line) and in situ nests (continuous line). (C,D) Lepidochelys olivacea hatchlings crawling to sea and emerging from nest, respectively.
Animal sampling, handling and sacrifice protocols were approved by an Animal Rights Committee, under License Number SEMARNAT: SGPA/DGVS/10395/17; in accordance with Mexican regulation (NOM-033-SAG/ZOO, 2014). One hundred and fifty turtles were collected from five in situ and five ex situ nests (15 hatchlings/nest). Fifty hatchlings were used for histological and morphometric observations: for neurogenesis studies, 24 brains per nest type were evaluated, since one brain for each condition was damaged during dissection (48 brains in total); for ovarian cell proliferation quantification, at least two ovaries per nest were used (27 ovaries in total), while all fifty individuals were sexed. The other one hundred hatchlings were used to evaluate motor performance.
Hatchling collection was described by Herrera-Vargas et al. (2017) and Robledo-Avila et al. (2022). Briefly, fifteen emerging turtles from each nest were collected at 5-min intervals, as soon as they surfaced from each nest. Five randomly selected hatchlings per nest were weighted with a digital precision balance (OHAUS™ Scout Pro Sp 602, Max 600 g, d = 0.01 g). Their straight carapace length was measured using a digital Vernier caliper (Mitutoyo™). These same five turtles per nest were used to investigate neurogenesis and gonadal cell proliferation.
To evaluate the effect of ex situ incubation on brain and ovary cell proliferation, hatchlings received an intraperitoneal injection of the cell birth marker 5′-bromo-2′-deoxyuridine (BrdU, a thymidine analog incorporated in the S phase of the cell cycle. Sigma-Aldrich, 100 mg/Kg in 0.9% NaCl) immediately after morphometric data recording and a second injection 2 h after. Turtles were then placed in sand tubs and sacrificed 4 h after the last injection. This procedure (i.e., the timings) minimized the effect of factors other than the incubation condition on cell proliferation. The brain and gonad-mesonephros complex were dissected in situ, incubated in Bouin’s solution (Sigma-Aldrich, Saint Louis, MO, United States) for 24 h and incubated in buffered paraformaldehyde (Sigma-Aldrich, Saint Louis, MO, United States, 4% in 0.1 M phosphate buffer) for 3 days at room temperature.
In the laboratory, brains were rinsed with 70% ethanol and transferred to buffered sucrose (Sigma-Aldrich, Saint Louis, MO, United States, 30% in 0.1 M phosphate buffer) at 4°C until they sank. Then they were frozen in the Peltier module of a cryostat (Microm) and sectioned coronally at 30 μm. Free-floating sections were collected in Tris-buffered saline (Sigma-Aldrich, Saint Louis, MO, United States, 50 mM Tris–HCl, 150 mM NaCl, pH 7.6) and processed for immunohistochemistry. Cell proliferation in neurogenic niches was evaluated by immunoreactivity for BrdU and neuronal integration was evaluated by immunoreactivity for the anti-neuronal nuclear protein (NeuN) in separate brain sections. Briefly, the tissue was incubated in ImmunoDNA retriever 20× with citrate (Bio SB), then in 2 N HCl for 30 min at 65°C and finally in 0.1 M sodium borate buffer at room temperature. Blocking of non-specific binding was done by incubating the sections in 0.1% bovine serum albumin (Sigma-Aldrich, Saint Louis, MO, United States) for 30 min. Sections including the dorsal and medial ventricular zones were incubated with mouse anti-BrdU monoclonal antibody (1: 500, Roche) and independent cortical sections were incubated with mouse anti-NeuN monoclonal antibody (1: 1000, Millipore) for 16 h at 4°C. Then they were incubated with a donkey anti-mouse biotin-conjugated secondary antibody (1:500, Vector Laboratories) for 2 h. Sections were incubated in avidin/biotin horseradish peroxidase (Vectastain Elite, PK-6100) for 2 h at room temperature and then incubated in diaminobenzidine as a chromogen, with peroxide and buffer for 10 min (Vector Staining Kit, SK-4100). Finally, the tissue was mounted onto gelatinized slides, dehydrated and cover-slipped using Cytoseal 60 (Richard Alan Scientific).
To evaluate cell proliferation in neurogenic niches, three equivalent non-adjacent brain sections containing the dorsal and medial ventricular zones were selected per turtle according morphological criteria (appearance of lateral ventricles) along the antero-posterior axis. BrdU + immunoreactive cells were quantified in two microphotographs per section per neurogenic zone at 1000× magnification. To evaluate neuronal integration, three equivalent non-adjacent brain sections including the dorsomedial and medial cortices were selected per turtle according morphological criteria (opening of the lateral ventricles). NeuN + immunoreactive cells in the cellular layer were quantified in three microphotographs per section per cortex at 1000× magnification. Microphotographs were captured with a Zeiss microscope using the Axio Vision 4.6 software and analyzed using NIH ImageJ software.
For gonadal sex determination, one gonad-mesonephros complex was dehydrated using increasing ethanol concentrations, embedded in paraffin, sliced transversally (7 μm) in a microtome (Leica) and stained with hematoxylin-eosin (Merck), as previously described (Herrera-Vargas et al., 2017). Gonadal histology showed that all fifty specimens were females, thus all gonadal analysis were performed in ovaries. To evaluate ovarian cell proliferation, at least two gonad-mesonephros complexes per nest were frozen, cryosectioned at 30 μm and immuno-stained for BrdU as described for the brain (the other gonad-mesonephros complexes were frozen for RNA quantification). Five adjacent gonadal sections per hatchling were selected from the central ovary. BrdU + immunoreactive germ cells were quantified in the cortex of ovaries throughout the whole section at 400 x magnification, as described for brain sections. After quantification, selected ovarian sections were stained with hematoxylin-eosin to observe cell density and cytoarchitecture.
To evaluate the effect of ex situ incubation on motor skills, ten hatchlings per nest were randomly selected and separated in tubs with sand for 15 min, to prevent lethargy from interfering with performance (Booth et al., 2013). Turtles were placed upside-down on a tray full of dry sand and the time they took to self-right was recorded with a chronometer (Sper scientific 810015 5 channel timer). Hatchlings that exceeded 10 min to self-right were discarded from the analysis. Thus, 48 turtles from in situ nests and 31 ex situ hatchlings were analyzed.
To evaluate the contribution of abiotic variables to the developmental traits, nest temperature, moisture and sand grain size were determined. Nest temperature was recorded from developmental day 11 until emergence, since this period includes the bulk of hippocampal neurogenesis previously described for Emys orbicularis (Goffinet et al., 1986) and the critical time for gonadal development in L. olivacea (Merchant-Larios et al., 1997). Temperature was registered by data loggers (Onset HOBO® Bluetooth Pendant MX2202 series; accuracy ± 0.2°C) carefully located outside the nest to avoid disturbing the clutch. They were placed in sand 30 cm from the center of the nest and 50 cm deep, 11 days after the incubation period began. They were programmed to record the temperature every hour; results were averaged by nest.
Moisture and grain size were determined from 100 g of sand, sampled 10 cm deep inside the nests, immediately after hatchling emergence. The sand was placed in a sealed plastic bag, weighed, dried at 105–110°C in a standard oven and weighed again. Moisture content was calculated as the ratio of wet to dry sand mass (Head, 1992). Grain size analysis was performed by particle sieving, using international parameters (gravel: >2.0; coarse sand: 2.0 ± 0.2; fine sand: 0.2 ± 0.02; silt: 0.02–0.002 mm; Brady and Weil, 2008), and subsequent weighting with an analytical balance. Gravel, coarse- and fine-sand, as well as silt proportions were calculated dividing by the total dry mass (Gee and Or, 2002).
Preliminary analysis showed that only one nest had a different gravel composition from the rest, thus gravel was discarded from further examination. Similarly, fine sand was collinear with coarse sand, hence only the latter was used. This was done because multivariate analyses are sensitive to collinearity between variables, which causes interpretation problems (Harrison et al., 2018). Accordingly, the selected parameters for analysis were abiotic variables within nests (temperature and moisture, coarse sand and silt), as well as turtle biological variables (cell proliferation in the dorsal and medial ventricular zones, as well as the ovary; neuronal integration in the cellular layer of the dorsomedial and medial cortices; body mass and length, as well as self-righting time).
Multivariate principal components analysis (PCA) was performed to reduce data dimensionality and investigate the distribution of samples in two-dimensions. This allowed the assessment of possible differences between conditions, based on the abiotic variables within nests and turtle biological data.
The outcomes of in situ (n = 5) vs. ex situ clutches (n = 5), abiotic variables and their interaction (in situ vs. ex situ condition interacting with each abiotic variable) were studied with linear mixed models to avoid violation of independence assumptions (turtles within clutches). Biological results were used as the response variables in these models; which included the following effects: in situ vs. ex situ clutches (main); abiotic variables and their interaction (fixed) plus turtles within clutches (random). All abiotic variables (temperature, moisture, coarse sand and silt) were standardized by subtracting the mean from every value and dividing by the standard deviation (Harrison et al., 2018), due to wide differences in their ranges. Outliers were removed from most turtle biological data (all except body mass and length). Ovarian cell proliferation and self-righting were also transformed to meet normality and homocedasticity assumptions; with square-root and natural logarithm, respectively.
Stepwise backward elimination followed by selection with the conditional Akaike information criterion (cAIC) were performed to obtain the best linear mixed model (Supplementary Data 1). The marginal R2 for each model was determined as a measure of the proportion of the variance explained by the model. The effect size for in situ vs. ex situ clutches was evaluated by Glass’s Δ (Sink and Mvududu, 2010; Sullivan and Feinn, 2012). Graphs presented in the results for each of the eight biological variables were obtained back-transforming the predictions of the final models. Residuals for each model were plotted to assess the distribution of the model fit (Supplementary Data 1).
Data analyses were done using R (R Core Team, 2020; version 4.1.1) and RStudio (RStudio Team, 2020; version 1.3.1073). Linear mixed models were performed using: readxl (Wickham and Bryan, 2019), lme4 (Bates et al., 2015), lmerTest (Kuznetsova et al., 2017), and cAIC (Säefken et al., 2018). Model parameters, including residuals, were evaluated with the performance package (Lüdecke et al., 2021). Principal component analysis results were graphed with ggbiplot (Vu, 2011).
Data provided by the national meteorological service during the entire incubation period (September 13–October 31, 2017) showed an average environmental temperature of 26.41°C ± 0.98 SD (mean minimum temperature = 20.53°C and mean maximum temperature = 32.27°C; Supplementary Table 1). Data loggers in sand near the nests recorded average maximum temperatures of 35.68°C ± 0.83 SD for in situ nests and 37.03°C ± 1.29 SD for ex situ nests, as well mean minimum temperatures of 26.28 ± 2.91 SD for in situ nests and 25.01 ± 0.14 SD for ex situ nests (Table 1). The average incubation period ± SD for hatchlings from in situ clutches was 46.40 ± 0.55 and 45.60 ± 1.82 days for hatchlings from ex situ clutches (Table 1). The mean moisture, coarse and fine sand, as well as silt proportions were: in situ (0.1180 ± 0.0936 SD; 0.9238 ± 0.0363 SD; 0.0643 ± 0.0384 SD; and 0.0005 ± 0.0008 SD, respectively) and ex situ clutches (0.0980 ± 0.0487 SD; 0.9011 ± 0.0176 SD; 0.0880 ± 0.0156 SD; and 0.0062 ± 0.0041 SD, respectively; Supplementary Table 2).
Table 1. Sand temperatures (°C) monitored by data loggers located 30 cm from the center of the clutch and 50 cm deep, as well as incubation periods registered for L. olivacea hatchlings at Boca Seca beach, Lázaro Cárdenas, Michoacán, México.
The PCA suggested that the synergic effect of some or all abiotic variables may be important to determine turtle phenotypes (see below). The first two principal components of the PCA, based on the biological and abiotic variables, explained 71.7% of the total data variance (Figure 2 and Supplementary Table 3). Principal component 1 (52%) showed that coarse sand (r = -0.202) and moisture (r = -0.122) were higher for in situ nests, while temperature (r = 0.304) and silt (r = 0.295) were lower. In addition, all biological variables from hatchlings (except self-righting) were higher for in situ conditions (Figure 2 and Supplementary Table 3). Principal component 2 (20%) showed that moisture (r = -0.543) and coarse sand proportions (r = -0.300) most influenced environmental variation for in situ nests (Figure 2 and Supplementary Table 3).
Figure 2. Multivariate principal components analysis of developmental and abiotic variables from in situ (n = 5, dark gray, left) vs. ex situ clutches (n = 5, light gray, right). W, body weight/mass (gr); L, body length (mm); M, neuronal integration in the cellular layer of the medial cortex (number of NeuN + cells/mm); D, neuronal integration in the cellular layer of the dorsomedial cortex (number of NeuN + cells/mm); O, cell proliferation in the ovary (number of BrdU + cells/mm); Dv, cell proliferation in the dorsal ventricular zone (number of BrdU + cells/mm); Mv, cell proliferation in the medial ventricular zone (number of BrdU + cells/mm); Cs, coarse sand (proportion); H, humidty (proportion); T, temperature (°C); SR, self-righting time (s); S, silt (proportion); PC1, principal component 1 of PCA; PC2, principal component 2 of PCA.
Principal components analysis results showed a clear difference between nest conditions. Thus, linear mixed models were performed to confirm these differences and explore their relationship to abiotic variables. Linear mixed models showed that ex situ nests were negatively related to L. olivacea development at nest emergence with moderate (Glass’s Δ < 1) to very large effect sizes (Glass’s Δ > 1.3; Sullivan and Feinn, 2012; Table 2). The simplest linear mixed models (in situ vs. ex situ nests and intercept) were the best fit for all biological variables (Table 2). Additionally, models with each abiotic variable alone (i.e., without in situ-ex situ factor) were performed for each biological variable. In all cases, the cAIC was better with the in situ-ex situ factor than with only one abiotic variable (Supplementary Data 1). Altogether, linear mixed models indicated that abiotic variables (temperature, moisture, and the proportion of different sediment sizes) did not individually affect any response variables, at least when using backward elimination and the lowest conditional AIC to select the best model.
Table 2. Summary of the best linear mixed effect models for each variable, selected by cAIC, explaining biological variables of Lepidochelys olivacea in situ vs. ex situ hatchlings.
In situ hatchlings showed on average 253.72 and 502.09 more proliferating cells in the dorsal (in situ: 491.04 ± 186.63 vs. ex situ: 237.32 ± 158.5 BrdU + cells/mm2 mean ± SD; p = 0.014) and medial ventricular zones (in situ: 1005.45 ± 468.77 vs. ex situ: 503.36 ± 333.62 BrdU + cells/mm2 mean ± SD; p = 0.031), respectively (Figure 3 and Table 2) than ex situ incubated offspring. They also showed on average 148.26 and 664.82 more mature neurons in the dorsomedial (in situ: 2033.85 ± 151.42 vs. ex situ: 1885.59 ± 157.67 NeuN + cells/mm2 mean ± SD; p = 0.041) and medial cortices (in situ: 2061.57 ± 283.11 vs. ex situ: 1396.75 ± 541.07 NeuN + cells/mm2 mean ± SD; p = 0.001), respectively (Figure 4 and Table 2) than ex situ hatchlings. The best models for dorsal and medial ventricular zone cell proliferation explained 33 and 28% of the data variation, while those for the dorsomedial and medial cortices explained 18 and 37% of the data variation (Table 2).
Figure 3. Cell proliferation in neurogenic niches of L. olivacea hatchlings. (A) Schematic drawing of turtle brain hemi-section showing the dorsal (Dvz, dotted line) and medial ventricular zone (Mvz, continuous dark line), lining the lateral ventricle. (B) Timeline for 5′-bromo-2′-deoxyuridine (BrdU) pulse-chase protocol for cell proliferation analysis. (C,D) Representative brain sections showing BrdU + nuclei in the Dvz (open arrow) and Mvz (filled arrow) of one turtle from each condition (in situ and ex situ, respectively). (E,F) Representative high magnification photographs showing BrdU + nuclei in the Dvz of one turtle from each condition (in situ and ex situ, respectively). (G,H) Representative high magnification photographs showing BrdU + nuclei in the Mvz of one turtle from each condition (in situ and ex situ, respectively). (I,J) Graphs showing the density of BrdU + nuclei. In situ (n = 5), ex situ (n = 5). Mean ± SD, Type III Analysis of Variance *p ≤ 0.05, scale bar = 200 μm for panels (C,D) and 50 μm for panels (E–H).
Figure 4. Neuronal integration in brain areas homologous to the mammalian hippocampus in L. olivacea hatchlings. (A) Schematic drawing of turtle brain hemi-section showing the dorsomedial (Dmc, stripes) and medial (Mc, stippled) cortices. (B) Timeline for neuronal integration analysis. (C,D) Representative brain sections showing neuronal nuclear protein (NeuN) in the Dmc and Mc of one turtle from each condition (in situ and ex situ, respectively). (E,F) Representative high magnification photographs showing NeuN + nuclei in the Dmc of one turtle from each condition (in situ and ex situ, respectively). (G,H) Representative high magnification photographs showing NeuN + nuclei in the Mc of one turtle from each condition (in situ or ex situ, respectively). (I,J) Graphs showing the density of NeuN + nuclei. In situ (n = 5), ex situ (n = 5). Mean ± SD, Type III Analysis of Variance *p ≤ 0.05, ***p ≤ 0.001, scale bar = 200 μm for panels (C,D) and 50 μm for panels (E–H).
The ovaries from in situ incubated hatchlings showed on average 11.99 more proliferating cells (in situ: 13.28 ± 2.21 vs. ex situ: 1.29 ± 1.38 BrdU + cells/mm2 mean ± SD; p < 0.001; Figure 5 and Table 2) in comparison with ovaries from ex situ offspring. The best model for ovarian cell proliferation explained 89% of the data variation (Table 2). Cell density and architecture were evaluated in sections with hematoxylin/eosin staining after BrdU quantification, but it was not possible to quantify the number of ovarian cells due to section thickness (Supplementary Figure 1).
Figure 5. Ovarian cell proliferation in L. olivacea females. (A) Schematic drawing of the turtle gonad-mesonephros complex. (B) Timeline for ovarian cell proliferation analysis. (C,D) Representative ovarian sections showing 5′-bromo-2′-deoxyuridine (BrdU) + nuclei in one turtle from each condition (in situ and ex situ, respectively). (E,F) Representative high magnification photographs showing BrdU + nuclei in the ovarian cortex of one turtle from each condition (in situ and ex situ, respectively). (G) Graph showing the density of ovarian BrdU + nuclei. In situ (n = 5), ex situ (n = 5). Mean ± SD, Type III Analysis of Variance ***p ≤ 0.001, scale bar = 200 μm for panels (C,D) and 50 μm for panels (E,F).
Turtles from in situ clutches were on average 1.66 g heavier (in situ: 17.65 ± 1.35 g vs. ex situ: 15.99 ± 1.1 g mean ± SD; p = 0.041; Figure 6A and Table 2), and 3.34 mm larger than ex situ offspring (in situ: 67.22 ± 2.50 mm vs. ex situ: 63.88 ± 2.33 mm mean ± SD; p = 0.012; Figure 6B and Table 2). Additionally, in situ incubated hatchlings were on average 5.49 s faster for self-righting (in situ: 2.56 ± 8.45 s vs. ex situ: 8.05 ± 6.90 s mean ± SD; p = 0.007; Figure 6C and Table 2) than ex situ hatchlings. The best model for body mass explained 31% of the data variation, while the model for body length explained 32% of the data variation and that for self-righting explained 26% of data variation (Table 2).
Figure 6. Body size and self-righting time of L. olivacea hatchlings. (A) Graph showing body mass. (B) Graph of body length. (C) Graph of self-righting time. In situ (n = 5), ex situ (n = 5). Mean ± SD, Type III Analysis of Variance *p ≤ 0.05, **p ≤ 0.01.
This work shows that ex situ protection of eggs in hatcheries can result in developmental deficits (compared to in situ nests) in female hatchlings, expanding our prior report in males (Herrera-Vargas et al., 2017). Herein, the ex situ incubation strategy was related to negative effects on neurogenesis, ovariogenesis, body size, and motor performance. Turtles from ex situ clutches showed lower cell proliferation in the ovary and neurogenic niches, as well as fewer differentiated neurons in brain areas homologous to the mammalian CA1/CA3 hippocampal regions and dentate gyrus. Additionally, newly hatched turtles from ex situ clutches showed lower body size and lesser motor abilities. Many factors could potentially account for the negative effects of ex situ incubation on offspring development. This study focused on the nest microenvironment (sand temperature, moisture, and sediment particle size). The PCA showed that this microenvironment was related to turtle phenotypes. However, the isolated contribution of the different abiotic variables to development could not be disentangled. A main limitation of this study was an impossibility to differentiate the effects of hatchery incubation from the effects of egg movement and reburial as well as genetic factors (i.e., parental origin). Of note, this study used a relatively small number of nests over a relatively narrow, but important, temperature range.
To evaluate the effect of ex situ incubation while minimizing the effect of turtle retention, two important events for early neurogenesis were evaluated 6 h after nest emergence: cell proliferation in neurogenic niches and neuron integration in cortices homologous to the mammalian hippocampus. The results showed that the ex situ incubation strategy is associated with a lower number of proliferating cells and differentiated neurons in turtle hatchlings. In vertebrates, hippocampal neurogenesis is a highly regulated process that includes cell proliferation, migration, differentiation and integration (Altman and Das, 1965; Gage, 2002; Bayer and Altman, 2004; Kuhn et al., 2016; McDonald and Vickaryous, 2018). Proper development of the mammalian hippocampus is required to achieve ecologically relevant cognitive tasks such as spatial learning and memory, as well as to regulate emotional responses (Gould et al., 1999; Deng et al., 2010).
In reptiles, newly born cells from the dorsal and lateral ventricular zones migrate radially for several days and mature as neurons as they approach the cortices. Neurogenic niches in the postnatal gecko brain produce neurons exclusively (McDonald and Vickaryous, 2018). Thus, it is highly likely that the BrdU + cells we observed herein were neuronal progenitors. Nonetheless, future cell-fate mapping studies should confirm the lineage of BrdU + cells produced in newly emerged sea turtles. In this study, BrdU + cells were only present lining the ventricular walls in both conditions, confirming the idea that proliferating neurons migrate several days after birth.
Offspring from ex situ clutches showed fewer BrdU + proliferating cells early after nest emergence, suggesting that a lower number of neural precursors will migrate to hippocampal cortices postnatally. Thus, less neurons (or glial cells) differentiate and integrate into functional circuits. Similarly, the lower density of NeuN + cells observed in the medial and dorsomedial cortices of hatchlings from ex situ clutches could reflect deficient prenatal cell proliferation or precursor migration. The difference between in situ and ex situ conditions for NeuN + cells was higher in the medial cortex, suggesting differential cortical sensitivity to early non-optimal conditions, as observed in mammals (Alkadhi, 2019). These findings, together with our prior work in male hatchlings (Herrera-Vargas et al., 2017), suggest that ex situ incubation alters neurogenic events during critical prenatal and early postnatal windows. The functional relevance of our findings for sea turtle cognitive and behavioral performance in the short and long-term are still unknown. However, recent evidence in lizards supports prior studies in mammals and birds, showing that a disturbance in neurogenesis during development could impair spatial memory and migration either during early life or adulthood (Amiel et al., 2017; Dayananda and Webb, 2017). Interestingly, these studies have related non-optimal incubation temperatures with decreased hippocampal neurogenesis in lizards (Amiel et al., 2017; Dayananda and Webb, 2017). Herein, nest temperature, moisture, and substrate composition differentiate in situ vs. ex situ nests; however, neither isolated abiotic variable was directly associated to altered neurogenesis in hatchling turtles. Follow-up studies should experimentally assess the effect of either variable separately or as a whole on hippocampal cell proliferation and neuronal integration early during ontogenesis in sea turtles.
Brain development begins at stage III (incubation day 4) in Caretta caretta turtles (García-Cerdá and López-Jurado, 2009). Thus, early relocation to hatcheries is not likely to account for the observed developmental alterations. However, future studies should be done to rule-out this possibility.
The ex situ incubation strategy was associated with a dramatic reduction of ovarian cell proliferation in turtle hatchlings at nest emergence, evidenced by few BrdU + cells. In L. olivacea, gonadal development starts in the middle third of incubation (day 16 of development), when primordial germ cells accumulate at the base of gonadal ridge (Merchant-Larios et al., 1997). In this species, warm temperatures (32–33°C) promote ovary formation by stimulating the production of estradiol and aromatase from the undifferentiated gonad and adjacent tissues: the mesonephros and inter-renal glands (Wibbels et al., 1991, 1993; Ewert et al., 2004; Freedberg et al., 2006; Díaz-Hernández et al., 2015, 2017). The highest peak of ovarian cell proliferation is not known for L. olivacea hatchlings. However, folliculogenesis and oocyte entry into meiosis occur in the 3rd–5th post-hatching months (Merchant-Larios et al., 1989), suggesting that this peak occurs perinatally. The consequences of reduced germ cell proliferation in female juvenile or adult turtles have not been evaluated. In rodents, germ cell absence does not allow ovarian follicle development (McLaren et al., 1984) or results in follicle degeneration (Ray and Potu, 2010; Bishop et al., 2019). In mammals, reduced cell proliferation may result in delayed ovarian formation or even complete infertility (Monniaux, 2018).
In this study, ovarian weight was not formally evaluated, since each was dissected together with the mesonephros. Interestingly, qualitative histological observations of ovarian sections did not obviously evidence a reduction in cell density. Herein, the main variable explaining the diminished ovarian cell proliferation was the ex situ condition, which probably included the effect of incubation temperature plus moisture and substrate composition. Future studies are needed to identify the effects of ex situ incubation on ovarian cell density, evaluate the contribution of each abiotic variable on the ovarian phenotype and elucidate the long-term effects of poorly developed ovaries.
Hatchlings from ex situ clutches showed a lower body mass and straight carapace length than those from in situ nests. Linear mixed models for body mass and length suggested that the nest condition (in situ vs. ex situ) was an important factor influencing them. A direct contribution of isolated abiotic variables could not be identified. However, it is known that temperature plays a chief role determining reptile body size (Stewart et al., 2019). Although sand temperatures were above the threshold to promote female differentiation in both conditions, they likely differentially affected hatchling body size, as previously suggested (Robledo-Avila et al., 2022). Mean sand temperatures registered for in situ clutches were approximately 1°C below those in ex situ clutches, whereas mean maximum temperatures registered for both conditions showed broader ranges (35.68°C ± 0.83 SD for in situ nests and 37.03°C ± 1.29 SD for ex situ nests). Accordingly, the incubation duration was shorter by one day for ex situ clutches.
The mechanisms that may account for a differential temperature effect on the growth rate include a direct action on cell, tissue, or organ differentiation, as well as long-term neuroendocrine changes possibly via epigenetic alterations (Singh et al., 2020). Additionally, it is recognized that moisture also plays a role on body size, although the mechanisms are less well known. Modifications to gas exchange could explain the effects of moisture on development (Wallace et al., 2004). Other variables, such as nesting female size (Chatting et al., 2018), egg mass (Wallace et al., 2006), metabolic expenditure (Rusli et al., 2016; Gammon et al., 2020), or yolk absorption (Stand, 2002) also may contribute to determining body size in reptiles. A study showed an interaction between the maternal component, sand temperature, moisture, and body length in the loggerhead sea turtles. Moreover, it described differential effects of moisture on body length throughout development (i.e., a more prominent role of nest moisture on body mass during the last third of development; Tezak et al., 2020). Thus, the combined contribution of several abiotic and biotic variables could explain our results, as discussed below.
A larger body size has been consistently related with better motor performance and thus with better survival chances (Fleming et al., 2020; Martins et al., 2020). In this study, principal component 1 showed a negative relationship between body mass and length with self-righting ability, supporting prior observations. Moreover, self-righting has been associated with incubatory conditions like temperature and substrate composition, therefore constituting a good indicator of microenvironmental conditions (Stewart et al., 2019; Reboul et al., 2021). Herein, nest silt and temperature were related to increased turning time of hatchlings, in accordance with prior studies (Stewart et al., 2019; Reboul et al., 2021).
Several nest-related abiotic variables were measured to determine their potential contribution to the observed developmental effects in L. olivacea hatchlings. The results showed that in situ clutch conditions were related to a better turtle phenotype (i.e., greater body size, higher neurogenesis, increased ovariogenesis, and lesser time to self-righting). In situ nests showed lower temperatures, were located higher on the beach and in coarse sand with lower silt levels, while ex situ clutches showed the opposite. Accordingly, sand composition, temperature, and moisture were important for differences between in situ and ex situ clutches. However, a differential contribution of each abiotic variable, in isolation, to the developmental traits could not be identified. One possible explanation is that emergent properties of microenvironment-associated abiotic variables affect the turtle hatchling development. Recent reports support this idea (Tezak et al., 2020; Tanabe et al., 2021).
Interestingly, although in situ nests showed lower temperatures than ex situ nests, the average temperatures for the incubation period, as well the mean maximum temperatures for both conditions were above the thermal tolerance reported for L. olivacea embryos (Valverde et al., 2010; Maulany et al., 2012). Temperature is a chief parameter that determines brain, gonadal and motor system development (Reece et al., 2002; Amiel and Shine, 2012; Paredes et al., 2016; Amiel et al., 2017; Fleming et al., 2020), as well embryo survival inside the nest (Robledo-Avila et al., 2022). Thus, it likely also plays a major role in explaining the observed phenotypes. However, its direct contribution could not be determined.
Ex situ clutches showed a higher silt proportion, which was negatively associated with hatchling development. Previous studies have shown that successful embryo development occurs in sandy substrates (grain diameter: 0.063–2 mm), whereas substrates with a high silt content (grain size < 0.063) cause mortality and diminished egg weight, reduced hatchling mass and size, as well as lower fitness (Sarmiento-Ramírez et al., 2014; Marco et al., 2017). The proportion of silt observed in both incubation conditions was below the value described as detrimental for embryo development (0.02; Abella-Pérez, 2011). Thus, although its effects on neurogenesis, ovariogenesis, body size, and motor abilities should not be ruled out, silt is unlikely to completely explain the observed phenotypes. The proportion of silt may affect moisture, gas exchange and/or microbial load (Marco et al., 2017).
Alternatively, the scarce contribution of sediment size, temperature, and moisture to completely explain the observed phenotypes could result from procedural details. Sand temperature was recorded before the thermosensitive period began (developmental day 11), until turtle emergence to avoid disturbing egg development. This interval includes the peak of hippocampal neurogenesis and the critical window for sex determination. Surface sand (10 cm deep) was sampled for moisture and particle size measurements right after turtles emerged. Future studies should record the temperature inside the nest during the entire incubation period and collect sand surrounding the eggs. This should verify the microenvironmental contribution to the effects of clutch relocation on development of sea turtle hatchlings. Moreover, future experiments should measure temperature inside the nest to consider the effect of metabolic heating on the observed phenotypes.
It must be noted that the ex situ incubation strategy, per se likely did not directly affect hatchling development. However, the combined effect of the incubation microenvironment was probably directly to blame for the phenotypic effects. This idea is supported by our prior study on the action of ex situ nests on the configuration of the immune system (Robledo-Avila et al., 2022).
Besides the measured abiotic factors, other variables such as micro-biological parameters (Patino-Martínez et al., 2012) may explain the developmental changes. Recent split-clutch designs have highlighted the maternal contribution to hatchling body size and self-righting response (Kobayashi et al., 2020; Tezak et al., 2020). This study did not consider the maternal component to privilege normal conditions (i.e., leaving natural nests undisturbed). However, parental origin, egg movement, and reburial should be studied to identify the factors that determine the altered phenotypes. These studies will result in recommendations for hatchery management to maximize the developmental potential of sea turtles.
Ex situ protection in hatcheries is undoubtedly the only choice if in situ incubation is not viable. However, our results suggest that this strategy can be associated with negative effects on sea turtle phenotypes. This include reduced neurogenesis, ovariogenesis, and motor performance during critical windows of development. Our results suggest a synergic effect of environmental variables on the observed phenotypes, but it was not possible to identify a differential contribution of each factor alone. More research should be done on the impact of ex situ incubation on cognitive, behavioral, and reproductive performance of juvenile or adult sea turtles experimentally manipulating abiotic variables.
The original contributions presented in the study are included in the article/Supplementary Material, further inquiries can be directed to the corresponding authors.
The animal study was reviewed and approved by Secretaría de Medio Ambiente y Recursos Naturales SGPA/DGVS/10395/17.
NU-D and CA: methodology and investigation. BP-F: formal analysis, writing – original draft, review, and editing. HN: formal analysis and writing – review and editing. LL-T and CM: methodology and formal analysis. NL and LT: visualization and supervision. MH-V: methodology. AF-F: conceptualization and funding acquisition. EM-H: conceptualization, data curation, writing – original draft, review, and editing. All authors contributed to the article and approved the submitted version.
This work was supported by the Consejo Nacional de Ciencia y Tecnología (CONACyT no. 258747 to AF-F) and the Coordinación de la Investigación Científica-UMSNH to EM-H and AF-F. NU-D was Ph.D. fellow from CONACYT (grant number 256790).
The authors declare that the research was conducted in the absence of any commercial or financial relationships that could be construed as a potential conflict of interest.
All claims expressed in this article are solely those of the authors and do not necessarily represent those of their affiliated organizations, or those of the publisher, the editors and the reviewers. Any product that may be evaluated in this article, or claim that may be made by its manufacturer, is not guaranteed or endorsed by the publisher.
We thank M. C. José Alejandro Marmolejo Valencia, Biol. Edel Pineda López, and Biol. Fidel Anguiano Rodríguez for their valuable technical assistance.
The Supplementary Material for this article can be found online at: https://www.frontiersin.org/articles/10.3389/fevo.2022.850612/full#supplementary-material
Abella-Pérez, E. (2011). Environmental and management factors affecting embryonic development in the loggerhead turtle Caretta caretta (L., 1758): implications for controlled egg incubation programmes. Zool. Caboverdiana 2, 40–42.
Ackerman, R. A. (1977). The respiratory gas exchange of sea turtle nests (Chelonia, Caretta). Respir. Physiol. 31, 19–38. doi: 10.1016/0034-5687(77)90062-7
Ahles, N., and Milton, S. L. (2016). Mid-incubation relocation and embryonic survival in Loggerhead sea turtle eggs. J. Wildl. Manage. 80, 430–437. doi: 10.1002/jwmg.1023
Alkadhi, K. A. (2019). Cellular and Molecular Differences Between Area CA1 and the Dentate Gyrus of the Hippocampus. Mol. Neurobiol. 56, 6566–6580. doi: 10.1007/s12035-019-1541-2
Altman, J., and Das, G. D. (1965). Autoradiographic and histological evidence of postnatal hippocampal neurogenesis in rats. J. Comp. Neurol. 124, 319–335. doi: 10.1002/cne.901240303
Amiel, J. J., Bao, S., and Shine, R. (2017). The effects of incubation temperature on the development of the cortical forebrain in a lizard. Anim. Cogn. 20, 117–125. doi: 10.1007/s10071-016-0993-2
Amiel, J. J., and Shine, R. (2012). Hotter nests produce smarter young lizards. Biol. Lett. 8, 372–374. doi: 10.1098/rsbl.2011.1161
Bannerman, D. M., Sprengel, R., Sanderson, D. J., McHugh, S. B., Rawlins, J. N., Monyer, H., et al. (2014). Hippocampal synaptic plasticity, spatial memory and anxiety. Nat. Rev. Neurosci. 15, 181–192. doi: 10.1038/nrn3677
Bates, D., Maechler, M., Bolker, B., and Walker, S. (2015). Fitting linear mixed-effects models using lme4. J. Stat. Softw. 67, 1–48. doi: 10.18637/jss.v067.i01
Bayer, S., and Altman, J. (2004). “Development of the telencephalon: neural stem cells, neurogenesis and neuronal migration,” in The Rat Nervous System, ed. G. Paxinos (London: Academic Press), 27–73.
Beltrán, I. Herculano-Houzel, S., Sinervo, B., and Whiting, M. J. (2021). Are ectotherm brains vulnerable to global warming? Trends Ecol. Evol. 36, 691–699. doi: 10.1016/j.tree.2021.04.009
Bishop, C. V., Reiter, T. E., Erikson, D. W., Hanna, C. B., Daughtry, B. L., Chavez, S. L., et al. (2019). Chronically elevated androgen and/or consumption of a Western-style diet impairs oocyte quality and granulosa cell function in the nonhuman primate periovulatory follicle. J. Assist. Reprod. Genet. 36, 1497–1511. doi: 10.1007/s10815-019-01497-8
Blanck, C. E., and Sawyer, R. H. (1981). Hatchery practices in relation to early embryology of the loggerhead sea turtle, Caretta caretta (Linné). J. Exp. Mar. Bio. Eco. 49, 163–177. doi: 10.1016/0022-0981(81)90068-X
Booth, D., and Astill, K. (2001a). Incubation temperature, energy expenditure and hatchling size in the green turtle (Chelonia mydas), a species with temperature-sensitive sex determination. Aust. J. Zool. 49, 389–396. doi: 10.1071/ZO01006
Booth, D., and Astill, K. (2001b). Temperature variation within and between nests of the green sea turtle, Chelonia mydas (Chelonia: Cheloniidae) on Heron Island, Great Barrier Reef. Aust. J. Zool. 49, 71–84. doi: 10.1071/ZO00059
Booth, D. T., Feeney, R., and Shibata, Y. (2013). Nest and maternal origin can influence morphology and locomotor performance of hatchling green turtles (Chelonia mydas) incubated in field nests. Mar. Biol. 160, 127–137. doi: 10.1007/s00227-012-2070-y
Brady, N., and Weil, R. (2008). The Nature and Properties of Soils, 14th Edn. Hoboken, NJ: Pearson Prentice Hall.
Butler, A. B., and Hodos, W. (2005). “Overview of the Forebrain,” in Comparative Vertebrate Neuroanatomy, Second Edn, eds A. B. Butler and W. Hodos (Hoboken, NJ: Wiley), 341–372. doi: 10.1002/0471733849.ch19
Chatting, M., Smyth, D., Al-Maslamani, I., Obbard, J., Al-Ansi, M., Hamza, S., et al. (2018). Nesting ecology of hawksbill turtles, Eretmochelys imbricata, in an extreme environmental setting. PLoS One 13:e0203257. doi: 10.1371/journal.pone.0203257
Chen, S. R., and Liu, Y. X. (2016). Testis cord maintenance in mouse embryos: genes and signaling. Biol. Reprod. 94:42. doi: 10.1095/biolreprod.115.137117
Coomber, P., Crews, D., and Gonzalez-Lima, F. (1997). Independent effects of incubation temperature and gonadal sex on the volume and metabolic capacity of brain nuclei in the leopard gecko (Eublepharis macularius), a lizard with temperature-dependent sex determination. J. Comp. Neurol. 380, 409–421. doi: 10.1002/(sici)1096-9861(19970414)380:3<409:aid-cne9<3.0.co;2-6
Cowan, C. S. M., and Richardson, R. (2018). A brief guide to studying fear in developing rodents: important Considerations and Common Pitfalls. Curr. Protoc. Neurosci. 83:e44. doi: 10.1002/cpns.44
Dang, W., Zhang, W., and Du, W. G. (2015). Incubation temperature affects the immune function of hatchling soft-shelled turtles, Pelodiscus sinensis. Sci. Rep. 5:10594. doi: 10.1038/srep10594
Dayananda, B., and Webb, J. K. (2017). Incubation under climate warming affects learning ability and survival in hatchling lizards. Biol. Lett. 13:20170002. doi: 10.1098/rsbl.2017.0002
de Melo, S. R., de David, Antoniazzi, C. T., Hossain, S., and Kolb, B. (2018). Neonatal stress has a long-lasting sex-dependent effect on anxiety-like behavior and neuronal morphology in the prefrontal cortex and hippocampus. Dev. Neurosci. 40, 93–103. doi: 10.1159/000486619
Deng, W., Aimone, J. B., and Gage, F. H. (2010). New neurons and new memories: How does adult hippocampal neurogenesis affect learning and memory? Nat. Rev. Neurosci. 11, 339–350. doi: 10.1038/nrn2822
Díaz-Hernández, V., Marmolejo-Valencia, A., and Merchant-Larios, H. (2015). Exogenous estradiol alters gonadal growth and timing of temperature sex determination in gonads of sea turtle. Dev. Biol. 408, 79–89. doi: 10.1016/j.ydbio.2015.05.022
Díaz-Hernández, V., Vázquez-Gómez, A., Marmolejo-Valencia, A., Montaño, L. M., and Merchant-Larios, H. (2017). 17ß-Estradiol modulates cell proliferation of medullary cords during ovarian differentiation of the Lepidochelys olivacea sea turtle. Dev. Biol. 431, 263–271. doi: 10.1016/j.ydbio.2017.09.008
Dimatelis, J. J., Vermeulen, I. M., Bugarith, K., Stein, D. J., and Russell, V. A. (2015). Female rats are resistant to developing the depressive phenotype induced by maternal separation stress. Metab. Brain. Dis. 31, 109–119. doi: 10.1007/s11011-015-9723-8
Eckert, K. L., and Eckert, S. A. (1990). Embryo mortality and hatch success in “in situ” and translocated leatherback sea turtle (Dermochelys coriacea) eggs. Biol. Cons. 53, 37–46. doi: 10.1016/0006-3207(90)90061-S
Ewert, M. A., Etchberger, C., and Nelson, C. E. (2004). “Turtle Sex-Determining Modes and TSD Patterns, and Some TSD Pattern Correlates,” in Temperature-Dependent Sex Determination in Vertebrates, eds N. Valenzuela and V. Lance (Washington, D.C: Smithsonian Institution Press), 21–32.
Fleming, K. A., Perrault, J. R., Stacy, N. I., Coppenrath, C. M., and Gainsbury, A. M. (2020). Heat, health and hatchlings: associations of in situ nest temperatures with morphological and physiological characteristics of loggerhead sea turtle hatchlings from Florida. Conserv. Physiol. 8:coaa046. doi: 10.1093/conphys/coaa046
Freedberg, S., Greives, T. J., Ewert, M. A., Demas, G. E., Beecher, N., and Nelson, C. E. (2008). Incubation environment affects immune system development in a turtle with environmental sex determination. J. Herpetol. 42, 536–541. doi: 10.1670/07-133.1
Freedberg, S., Nelson, C. E., and Ewert, M. A. (2006). Estradiol-17ß induces lasting sex reversal at male-producing temperatures in kinosternid turtles. J. Herpetol. 40, 95–98.
Gage, F. H. (2002). Neurogenesis in the adult brain. J. Neurosci. 22, 612–613. doi: 10.1523/JNEUROSCI.22-03-00612.2002
Gammon, M., Fossette, S., McGrath, G., and Mitchell, N. (2020). A systematic review of metabolic heat in sea turtle nests and methods to model its impact on hatching success. Front. Ecol. Evol. 8:556379. doi: 10.3389/fevo.2020.556379
García-Cerdá, M. R., and López-Jurado, L. F. (2009). Desarrollo Embrionario de Caretta:Análisis Descriptivo de su Evolución Morfológica. Ph.D. thesis. Las Palmas: Departamento de Biología Universidad de Las Palmas de Gran Canaria.
Ge, C., Ye, J., Weber, C., Sun, W., Zhang, H., Zhou, Y., et al. (2018). The histone demethylase KDM6B regulates temperature-dependent sex determination in a turtle species. Science 360, 645–648. doi: 10.1126/science.aap8328
Gee, G. W., and Or, D. (2002). “Particle-size analysis,” in Methods of soil analysis, Soil Science Society of America, eds J. H. Dane and G. C. Topp (Madison, WI: Soils Science Society of America), 255–293. doi: 10.2136/sssabookser5.4.c12
Goffinet, A. M., Daumerie, C., Langerwerf, B., and Pieau, C. (1986). Neurogenesis in reptilian cortical structures: 3H-Thymidine autoradiographic analysis. J. Comp. Neurol. 243, 106–116. doi: 10.1002/cne.902430109
Gould, E., Beylin, A., Tanapat, P., Reeves, A., and Shors, T. J. (1999). Learning enhances adult neurogenesis in the hippocampal formation. Nat. Neurosci. 2, 260–265. doi: 10.1038/6365
Harrison, X. A., Donaldson, L., Correa-Cano, M. E., Evans, J., Fisher, D. N., Goodwin, C. E. D., et al. (2018). A brief introduction to mixed effects modelling and multi-model inference in ecology. PeerJ 6:e4794. doi: 10.7717/peerj.4794
Heim, C., and Nemeroff, C. B. (2002). Neurobiology of early life stress: clinical studies. Semin. Clin. Neuropsychiatry 7, 147–159. doi: 10.1053/scnp.2002.33127
Heppell, S. S., Burchfield, P. M., and Peña, L. J. (2007). “Kemp’s ridley recovery. How far have we come, and where are we headed?,” in Biology and Conservation of Ridley Sea Turtles, ed. P. T. Plotkin (Baltimore, MD: Johns Hopkins University Press), 325–335.
Heppell, S. S., Crouse, D. T., Crowder, L. B., Epperly, S. P., Gabriel, W., Henwood, T., et al. (2005). A population model to estimate recovery time, population size, and management impacts on Kemp’s ridley sea turtles. Chelonian Conserv. Biol. 4, 767–773.
Herrera-Vargas, A., Meléndez-Herrera, E., Gutiérrez-Ospina, G., Bucio-Piña, F. B., Báez-Saldaña, A., Siliceo-Cantero, H., et al. (2017). Hatchlings of the marine turtle Lepidochelys olivacea display signs of prenatal stress at emergence after being incubated in man-made nests: a preliminary report. Front. Mar. Sci. 4:400. doi: 10.3389/fmars.2017.00400
Holm, J. B., Mazaud-Guittot, S., Danneskiold-Samsoe, N. B., Chalmey, C., Jensen, B., Norregárd, M. M., et al. (2016). Intrauterine exposure to paracetamol and aniline impairs female reproductive development by reducing follicle reserves and fertility. Toxicol. Sci. 150, 178–189. doi: 10.1093/toxsci/kfv332
Kobayashi, S., Endo, D., Kondo, S., Kitayama, C., Ogawa, R., Arai, K., et al. (2020). Investigating the effects of nest shading on the green turtle (Chelonia mydas) hatchling phenotype in the Ogasawara islands using a field-based split clutch experiment. J. Exp. Zool. A Ecol. Integr. Physiol. 2020, 629–636. doi: 10.1002/jez.2411
Kuhn, H. G., Eisch, A. J., Spalding, K., and Peterson, D. A. (2016). Detection and phenotypic characterization of adult neurogenesis. Cold Spring Harb. Perspect. Biol. 8, a025981. doi: 10.1101/cshperspect.a025981
Kutzari (2006). Manual de Técnicas de Protección de Tortugas Marinas. Available online at: https://savepacificleatherbacks.org/wp-content/uploads/2017/08/KUTZARI-MANUAL_TORTUGAS.pdf (accessed Dec. 28, 2020).
Kuznetsova, A., Brockhoff, P. B., and Christensen, R. H. B. (2017). lmertest package: tests in linear mixed effects models. J. Stat. Softw. 82, 1–26. doi: 10.18637/jss.v082.i13
Lajud, N., and Torner, L. (2015). Early life stress and hippocampal neurogenesis in the neonate: sexual dimorphism, long term consequences and possible mediators. Front. Mol. Neurosci. 8, doi: 10.3389/fnmol.2015.00003
Limpus, C., Baker, V., and Miller, J. (1979). Movement induced mortality of loggerhead eggs. Herpetologica 35, 335–338.
Lolavar, A., and Wyneken, J. (2020). The impact of sand moisture on the temperature-sex ratio responses of developing loggerhead (Caretta caretta) sea turtles. Zoology 138:125739. doi: 10.1016/j.zool.2019.125739
Lüdecke, D. Ben-Shachar, M. S., Patil, I., Waggoner, P., and Makowski, D. (2021). performance: an R package for assessment, comparison and testing of statistical models. J. Open Sour. Softw. 6:3139. doi: 10.21105/joss.03139
Lutcavage, M. E., Plotkin, P., Witherington, B., and Lutz, P. (1997). “Human impacts on sea turtle survival,” in The biology of Sea Turtles, eds P. L. Lutz J. A. Musick (Boca Raton, FL: CRC Press), 387–409.
Marco, A., Abella-Perez, E., and Tiwari, M. (2017). Vulnerability of loggerhead turtle eggs to the presence of clay and silt on nesting beaches. J. Exp. Mar. Biol. Ecol. 486, 195–203. doi: 10.1016/j.jembe.2016.10.015
Martins, S., Silva, E., Abella, E., de Santos Loureiro, N., and Marco, A. (2020). Warmer incubation temperature influences sea turtle survival and nullifies the benefit of a female-biased sex ratio. Clim. change 163, 689–704.
Maulany, R. I., Booth, D. T., and Baxter, G. S. (2012). The effect of incubation temperature on hatchling quality in the olive ridley turtle, Lepidochelys olivacea, from Alas Purwo National Park, East Java, Indonesia: implications for hatchery management. Mar. Biol. 159, 2651–2661. doi: 10.1007/s00227-012-2022-6
Mazaris, A. D., Schofield, G., Gkazinou, C., Almpanidou, V., and Hays, G. C. (2017). Global sea turtle conservation successes. Sci. Adv. 3:e1600730. doi: 10.1126/sciadv.1600730
McDonald, R. P., and Vickaryous, M. K. (2018). Evidence for neurogenesis in the medial cortex of the leopard gecko, Eublepharis macularius. Sci. Rep. 8:9648. doi: 10.1038/s41598-018-27880-6
McElroy, M., Dodd, M. G., and Catleberry, S. B. (2015). Effects of common loggerhead sea turtle nest management methods on hatching and emergence success at Sapelo Island, Georgia, USA. Chelonian Conserv. Biol. 14, 49–55. doi: 10.2744/ccab-14-01-49-55.1
McEwen, B. S. (2012). Brain on stress: How the social environment gets under the skin. Proc. Natl. Acad. Sci. U.S.A. 109, 17180–17185. doi: 10.1073/pnas.1121254109
McLaren, A., Simpson, E., Tomonari, K., Chandler, P., and Hogg, H. (1984). Male sexual differentiation in mice lacking H-Y antigen. Nature 312, 552–555. doi: 10.1038/312552a0
Medina, L., Abellán, A., and Desfilis, E. (2017). Contribution of genoarchitecture to understanding hippocampal evolution and development. Brain Behav. Evol. 90, 25–40. doi: 10.1159/000477558
Merchant-Larios, H., Ruiz-Ramirez, S., Moreno-Mendoza, N., and Marmolejo-Valencia, A. (1997). Correlation among thermosensitive period, estradiol response, and gonad differentiation in the sea turtle Lepidochelys olivacea. Gen. Comp. Endocrinol. 107, 373–385. doi: 10.1006/gcen.1997.6946
Merchant-Larios, H., Villalpando-Fierro, I., and Centeno-Urruiza, B. (1989). Gonadal morphogenesis under controlled temperature in the sea turtle Lepidochelys olivacea. Herpetol. Monogr. 3, 43–61. doi: 10.2307/1466985
Miller, J. D. (1985). “Embryology of marine turtles,” in Biology of the Reptilia Vol. 14A Development, eds C. Gans, F. Billett, and P. F. A. Maderson (New York, NY: Wiley-Interscience), 269–328.
Mitchell, T. S., and Janzen, F. J. (2019). Substrate influences turtle nest temperature, incubation period, and offspring sex ratio in the field. Herpetologica 75, 57–62. doi: 10.1655/D-18-00001
Monniaux, D. (2018). Factors influencing establishment of the ovarian reserve and their effects on fertility. Anim. Reprod. 15, 635–647. doi: 10.21451/1984-3143-AR2018-0011
NOM-162-SEMARNAT (2012). Que establece las especificaciones para la protección, recuperación y manejo de las poblaciones de las tortugas marinas ensuhábitat de anidación. Available online at: http://dof.gob.mx/nota_detalle.php?codigo=5286506&fecha=01/02/2013 (accessed December 28, 2020).
NOM-033-SAG/ZOO (2014). Métodos para dar muerte a los animales domésticos y silvestres. Available online at: https://www.dof.gob.mx/nota_detalle.php?codigo=5405210&fecha=26/08/2015 (accessed December 28, 2020).
Paredes, U., Radersma, R., Cannell, N., While, G. M., and Uller, T. (2016). Low incubation temperature induces DNA hypomethylation in lizard brains. J. Exp. Zool. A Ecol. Genet. Physiol. 325, 390–395. doi: 10.1002/jez.2024
Patino-Martínez, J., Marco, A., Quiñones, L., Abella, E., Abad, R. M., and Diéguez-Uribeondo, J. (2012). How do hatcheries influence embryonic development of sea turtle eggs? Experimental analysis and isolation of microorganisms in leatherback turtle eggs. J. Exp. Zool. A Ecol. Genet. Physiol. 317, 47–54. doi: 10.1002/jez.719
Pintus, K. J., Godley, B. J., McGowan, A., and Broderick, A. C. (2009). Impact of clutch relocation on green turtle offspring. J. Wild. Manage. 73, 1151–1157. doi: 10.2193/2008-103
R Core Team (2020). R: A Language and Environment for Statistical Computing. R Foundation for Statistical Computing. Available online at: https://www.R-project.org/ (accessed Dec. 28, 2020).
Ray, B., and Potu, B. K. (2010). Ovarian folliculogenesis: detrimental effect of prenatal exposure to cyclophosphamide: a preliminary study. Bratisl. Lek. Listy 111, 369–372.
Reboul, I., Booth, D., and Rusli, U. (2021). Artificial and natural shade: implications for green turtle (Chelonia mydas) rookery management. Ocean Coast. Manag. 204:105521.
Reece, S. E., Broderick, A. C., Godley, B. J., and West, S. A. (2002). The effects of incubation environment, sex and pedigree on hatchling phenotype in a natural population of loggerhead sea turtles. Evol. Ecol. Res. 4, 737–748.
Robledo-Avila, L. A., Phillips-Farfán, B. V., Harfush, M., López, L., Tafolla, D., Herrera-Vargas, M. A., et al. (2022). Ex-situ conservation in hatcheries is associated with spleen development in Lepidochelys olivacea turtle hatchlings. Comp. Biochem. Physiol. Part A Mol. Integr. Physiol. 265:111130. doi: 10.1016/j.cbpa.2021.111130
Rusli, M. U., Stand, D. T., and Joseph, J. (2016). Synchronous activity lowers the energetic cost of nest escape for sea turtle hatchlings. J. Exp. Biol. 10, 1505–1513. doi: 10.1242/jeb.134742
Säefken, B., Rügamer, D., Kneib, T., and Greven, S. (2018). Conditional model selection in mixed-effects models with cAIC4. ArXiv [Preprint]. doi: 10.48550/arXiv.1803.05664
Sanger, T. J., Harding, L., Kyrkos, J., Turnquist, A. J., Epperlein, L., Nunez, S. A., et al. (2021). Environmental thermal stress induces neuronal cell death and developmental malformations in reptiles. Integr. Org. Biol. 3:obab033. doi: 10.1093/iob/obab033
Sanger, T. J., Kyrkos, J., Lachance, D. J., Czesny, B., and Stroud, J. T. (2018). The effects of thermal stress on the early development of the lizard Anolis sagrei. J Exp Zool A Ecol Integr Physiol 329, 244–251. doi: 10.1002/jez.2185
Sapolsky, R. M., Krey, L. C., and McEwen, B. S. (1984). Glucocorticoid-sensitive hippocampal neurons are involved in terminating the adrenocortical stress response. Proc. Natl. Acad. Sci. U.S.A. 81, 6174–6177. doi: 10.1073/pnas.81.19.6174
Sapolsky, R. M., McEwen, B. S., and Rainbow, T. C. (1983). Quantitative autoradiography of [3H] corticosterone receptors in rat brain. Brain Res. 271, 331–334. doi: 10.1016/0006-8993(83)90295-0
Sarmiento-Ramírez, J. M., Abella-Pérez, E., Phillott, A. D., Sim, J., van West, P., Martín, M. P., et al. (2014). Global distribution of two fungal pathogens threatening endangered sea turtles. PLoS One 9:e85853. doi: 10.1371/journal.pone.0085853
Sieg, A. E., Binckley, C. A., Wallace, B. P., Tomillo, P. S., Reina, R. D., Paladino, F. V., et al. (2011). Sex ratios of leatherback turtles: hatchery translocation decreases metabolic heating and female bias. Endanger. Species Res. 15, 195–204.
Singh, S. K., Das, D., and Rhen, T. (2020). Embryonic temperature programs phenotype in reptiles. Front. Physiol. 11:35. doi: 10.3389/fphys.2020.00035
Sink, C. A., and Mvududu, N. H. (2010). Statistical power, sampling, and effect sizes: three keys to research relevancy. Couns. Outcome Res. Evaluat. 1, 1–18. doi: 10.1177/2150137810373613
Sönmez, B., Turan, C., and Yalçin Özdilek, S. (2011). The effect of relocation on the morphology of green turtle, Chelonia mydas (Linnaeus, 1758), hatchlings on Samandag beach, Turkey. Zool. Middle East 52, 29–38.
Stand, D. T. (2002). Incubation of rigid-shelled turtle eggs: do hydric conditions matter? J. Comp. Physiol. B 172, 627–633. doi: 10.1007/s00360-002-0291-y
Stewart, T. A., Booth, D. T., and Rusli, M. U. (2019). Influence of sand grain size and nest microenvironment on incubation success, hatchling morphology and locomotion performance of green turtles (Chelonia mydas) at the Chagar Hutang Turtle Sanctuary, Redang Island, Malaysia. Aust. J. Zool. 66, 356–368. doi: 10.1071/ZO19025
Suh, J., Rivest, A. J., Nakashiba, T., Tominaga, T., and Tonegawa, S. (2011). Entorhinal cortex layer III input to the hippocampus is crucial for temporal association memory. Science 334, 1415–1420. doi: 10.1126/science.1210125
Sullivan, G. M., and Feinn, R. (2012). Using Effect Size-or Why the P Value Is Not Enough. J. Grad. Med. Educ. 4, 279–282. doi: 10.4300/JGME-D-12-00156.1
Tanabe, L. K., Ellis, J., Elsadek, I., and Berumen, M. L. (2020). Potential feminization of Red Sea turtle hatchlings as indicated by in situ sand temperature profiles. Conserv. Sci. Pract. 2:e266. doi: 10.1111/csp2.266
Tanabe, L. K., Steenacker, M., Rusli, M. U., and Berumen, M. L. (2021). Implications of nest relocation for morphology and locomotor performance of green turtle (Chelonia mydas) hatchlings. Ocean Coast. Manag. 207:105591. doi: 10.1016/j.ocecoaman.2021.105591
Telemeco, R. S., Warner, D. A., Reida, M. K., and Janzen, F. J. (2013). Extreme developmental temperatures result in morphological abnormalities in painted turtles (Chrysemys picta): a climate change perspective. Integr. Zool. 8, 197–208. doi: 10.1111/1749-4877.12019
Tezak, B., Bentley, B., Arena, M., Mueller, S., Snyder, T., and Sifuentes-Romero, I. (2020). Incubation environment and parental identity affect sea turtle development and hatchling phenotype. Oecologia 192, 939–951. doi: 10.1007/s00442-020-04643-7
Tosches, M. A., Yamawaki, T. M., Naumann, R. K., Jacobi, A. A., Tushev, G., and Laurent, G. (2018). Evolution of pallium, hippocampus, and cortical cell types revealed by single-cell transcriptomics in reptiles. Science 360, 881–888. doi: 10.1126/science.aar4237
Trnik, M., Albrechtová, J., and Kratochvíl, L. (2011). Persistent effect of incubation temperature on stress-induced behavior in the Yucatan banded gecko (Coleonyx elegans). J. Comp. Psychol. 125, 22–30. doi: 10.1037/a0021186
Vaiserman, A. M., and Koliada, A. K. (2017). Early-life adversity and long-term neurobehavioral outcomes: Epigenome as a bridge? Hum. Genomics 11:34. doi: 10.1186/s40246-017-0129-z
Valverde, R. A., Wingard, S., Gómez, F., Tordoir, M. T., and Orrego, C. M. (2010). Field lethal incubation temperature of olive ridley sea turtle Lepidochelys olivacea embryos at a mass nesting rookery. 12, 77–86. doi: 10.3354/esr00296
Vu, V. Q. (2011). ggbiplot: A ggplot2 based biplot. R package version 0.55. Available online at: http://github.com/vqv/ggbiplot (accessed Dec. 28, 2020).
Wallace, B. P., Sotherland, P. R., Spotila, J. R., Reina, R. D., Franks, B. F., and Paladino, F. V. (2004). Biotic and abiotic factors affect the nest environment of embryonic Leatherback Turtles, Dermochelys coriacea. Physiol. Biochem. Zool. 77, 423–432. doi: 10.1086/420951
Wallace, B. P., Sotherland, P. R., Tomillo, P. S., Bouchard, S. S., Reina, R. D., Spotila, J. R., et al. (2006). Egg components, egg size, and hatchling size in leatherback turtles. Comp. Biochem. Physiol. A Mol. Integr. Physiol. 145, 524–532. doi: 10.1016/j.cbpa.2006.08.040
Weber, C., Zhou, Y., Lee, J. G., Looger, L. L., Qian, G., Ge, C., et al. (2020). Temperature-dependent sex determination is mediated by pSTAT3 repression of Kdm6b. Science 368, 303–306. doi: 10.1126/science.aaz4165
Wibbels, T., Bull, J. J., and Crews, D. (1991). Synergism between temperature and estradiol: A common pathway in turtle sex determination? J. Exp. Zool. 260, 130–134. doi: 10.1002/jez.1402600117
Wibbels, T., Gideon, P., Bull, J. J., and Crews, D. (1993). Estrogen- and temperature-induced medullary cord regression during gonadal differentiation in a turtle. Differentiation 53, 149–154. doi: 10.1111/j.1432-0436.1993.tb00703.x
Wickham, H., and Bryan, J. (2019). readxl: Read Excel Files. R package version 1.3.1. Available online at: https://CRAN.R-project.org/package=readxl (accessed Dec. 28, 2020).
Keywords: Lepidochelys olivacea, brain development, ovarian development, neuronal integration, temperature, substrate composition, moisture
Citation: Unda-Díaz NM, Phillips-Farfán BV, Nava H, Lopez-Toledo L, Murata C, Lajud N, Herrera-Vargas MA, Arreola Camacho CA, Torner L, Fuentes-Farías AL and Meléndez-Herrera E (2022) Negative Effects on Neurogenesis, Ovariogenesis, and Fitness in Sea Turtle Hatchlings Associated to ex situ Incubation Management. Front. Ecol. Evol. 10:850612. doi: 10.3389/fevo.2022.850612
Received: 07 January 2022; Accepted: 12 May 2022;
Published: 13 June 2022.
Edited by:
Jeanine M. Refsnider, University of Toledo, United StatesReviewed by:
Nathan Freeman Putman, LGL, United StatesCopyright © 2022 Unda-Díaz, Phillips-Farfán, Nava, Lopez-Toledo, Murata, Lajud, Herrera-Vargas, Arreola Camacho, Torner, Fuentes-Farías and Meléndez-Herrera. This is an open-access article distributed under the terms of the Creative Commons Attribution License (CC BY). The use, distribution or reproduction in other forums is permitted, provided the original author(s) and the copyright owner(s) are credited and that the original publication in this journal is cited, in accordance with accepted academic practice. No use, distribution or reproduction is permitted which does not comply with these terms.
*Correspondence: Esperanza Meléndez-Herrera, ZW1lbGVuZGV6QHVtaWNoLm14; Alma L. Fuentes-Farías, YWxtYS5mdWVudGVzQHVtaWNoLm14
Disclaimer: All claims expressed in this article are solely those of the authors and do not necessarily represent those of their affiliated organizations, or those of the publisher, the editors and the reviewers. Any product that may be evaluated in this article or claim that may be made by its manufacturer is not guaranteed or endorsed by the publisher.
Research integrity at Frontiers
Learn more about the work of our research integrity team to safeguard the quality of each article we publish.