- 1Department of Chemical Ecology, Bielefeld University, Bielefeld, Germany
- 2School of Applied Sciences, Edinburgh Napier University, Edinburgh, United Kingdom
Gregarines are ubiquitous endosymbionts in invertebrates, including terrestrial insects. However, the biodiversity of gregarines is probably vastly underestimated and the knowledge about their role in shaping fitness-related traits of their host in dependence of fluctuating environmental conditions is limited. Using morphological and molecular analyses, we identified a new gregarine species, Gregarina cochlearium sp. n., in the mustard leaf beetle, Phaedon cochleariae. Applying a full-factorial design, we investigated the effects of a gregarine infection in combination with fluctuating starvation conditions during the larval stage on the development time and fitness-related traits of adult beetles. Under benign environmental conditions, the relationship between gregarines and the host seemed neutral, as host development, body mass, reproduction and survival were not altered by a gregarine infection. However, when additionally exposed to starvation, the combination of gregarine infection and this stress resulted in the lowest reproduction and survival of the host, which points to a parasitic relationship. Furthermore, when the host experienced starvation, the development time was prolonged and the adult females were lighter compared to non-starved individuals, independent of the presence of gregarines. Counting of gregarines in the guts of larvae revealed a lower gregarine load with increasing host body mass under stable food conditions, which indicates a regulation of the gregarine burden in dependence of the host condition. Contrary, in starved individuals the number of gregarines was the highest, hence the already weakened host suffered additionally from a higher gregarine burden. This interactive effect between gregarine infection and fluctuating starvation conditions led to an overall reduced fitness of P. cochleariae. Our study emphasizes the need to study endosymbionts as important components of the natural environment and to investigate the role of host-symbiont relationships under fluctuating environmental conditions in an evolutionary and ecological context.
Introduction
Probably all plant and animal species are parasitized by at least one, often several protist or metazoan species (Roberts and Janovy, 2008). Gregarines belong to the Apicomplexa and occur in many marine, freshwater and terrestrial invertebrate taxa (Logan et al., 2012). They predominantly live as extracellular endosymbionts, often acting as parasites, in the digestive tract or body cavities of their hosts (Desportes and Schrével, 2013). Most gregarines develop and reproduce within their host and spread by releasing gametocysts, filled with oocysts that contain infectious sporozoites, into the environment (Clopton, 2002). Gregarines are likely highly host-specific in terrestrial invertebrates (Clopton and Gold, 1996). Although an increasing number of studies have examined the relationships between gregarines and their hosts, our knowledge about the gregarine identity and the effects of gregarines on their hosts under fluctuating environmental conditions is still limited.
The effects of gregarines on their host’s performance are various, as they can act as mutualists, commensals or parasites (Rueckert et al., 2019). Mutualistic interactions between gregarines and their hosts are suggested for cat fleas (Ctenocephalides felis; Siphonaptera: Pulicidae), because individuals infected with Steinina ctenocephali develop faster than non-infected ones (Alarcón et al., 2017). Besides such effects on developmental performance, beneficial behavioral changes leading to reduced predation rates can result from gregarine infection, as, for example, in larvae of Aedes triseriatus (Diptera: Culicidae) infected with Ascogregarina barretti (Soghigian et al., 2017). In other insect species, gregarines were found to have no or only subtle effects on the hosts and therefore sometimes remain undetected (Klingenberg et al., 1997; Tsubaki and Hooper, 2004). However, some studies provide evidence for harmful effects of gregarines, such as damaging the host gut epithelium (Åbro, 1971), occluding the midgut (Gigliolli et al., 2016) and affecting the host metabolism (Schilder and Marden, 2007), resulting in significantly increased host mortality. As the literature provides evidence for contrasting relationships between gregarines and their hosts, the question arises what determines these relationships from mutualistic to parasitic.
Many experiments testing the interactions of gregarines with their hosts were performed under constant, optimal environmental conditions. However, in nature, environments may fluctuate and hosts may repeatedly face suboptimal conditions such as nutrient-poor diet or limitation of food leading to partial food deprivation or starvation. Insects facing such harsh conditions often show a suppressed performance, which appears particularly drastic in early life stages (Boggs and Freeman, 2005; Dmitriew and Rowe, 2011). In addition to direct negative impacts exerted by harsh conditions on fitness parameters of the exposed insects, such conditions also affect the host-endosymbiont assemblage, impacting their ecology and evolution (Bénard et al., 2020). In fact, in several insect species negative influences of gregarines were only detected when hosts were kept under suboptimal conditions (Harry, 1967; Zuk, 1987; Tsubaki and Hooper, 2004). For example, larvae of Tenebrio molitor (Coleoptera: Tenebrionidae) infected with Gregarina polymorpha showed a lower pupal biomass and a prolonged development when kept on a suboptimal diet (Harry, 1967). Likewise, the longevity was reduced in gregarine-infected Gryllus pennsylvanicus (Orthoptera: Gryllidae) and Mnais costalis (Zygoptera: Calopterygoidea) only under limited food supply but not under ad libitum food conditions (Zuk, 1987; Tsubaki and Hooper, 2004). Under food scarcity the parasites may perceive the decline in the nutritional status of the host and promote their own development and reproduction to spread successfully (Jokela et al., 2005), leading to a more pronounced exploitation of the already weakened host and ultimately a reduction in its fitness.
In beetles, one or two gregarine species are usually present per host species (Logan et al., 2012). In field populations of Phaedon brassicae (Coleoptera: Chrysomelidae), almost all collected larvae were infected with Gregarina sp. (Kim et al., 2014). Likewise, gregarines have been regularly found within individuals of the mustard leaf beetle, Phaedon cochleariae, collected in The Netherlands or Germany. However, the gregarine species has not yet been described and systematic data are lacking. The effect of gregarines on fitness-related traits of P. cochleariae can be considered as moderate, as the development time of larvae is prolonged by 1–2 days when individuals are infected under optimal laboratory conditions (Wolz et al., 2022). However, when larvae of P. cochleariae were exposed to sublethal insecticide exposure, gregarine-infected individuals suffered more than uninfected ones under such an environmental challenge (Wolz et al., 2022). Larvae of P. cochleariae kept for many generations on either one of three distinct host plant species showed comparable intermediate infection with gregarines in terms of expression levels of gregarine ribosomal protein genes (Müller et al., 2017). When larvae were switched to another host plant species, levels either decreased or increased, depending on the plant species (Müller et al., 2017). Thus, nutritional quality of the food may be important for both, the gregarine and the insect development (Tremmel and Müller, 2013; Müller et al., 2017). However, we lack insights into effects of food quantity, i.e., starvation, on this beetle-gregarine interaction.
In the present study we aimed to identify and clarify the phylogenetic position of the gregarine species infecting P. cochleariae. Furthermore, we investigated the effects of this gregarine species in combination with fluctuating starvation conditions of the host on life-history traits of the beetle. We predicted that under ad libitum food supply life-history traits are not impaired, because we considered the gregarines as endosymbionts with a moderate effect on the leaf beetle. However, we assumed that under larval starvation the relationship changes from a neutral to a parasitic one, leading to a prolonged development time, reduced adult body mass, altered reproduction and increased mortality of infected and starved beetles. Finally, we studied whether host starvation may affect the infection load with gregarines and assumed that gregarines develop more successfully when the host suffers from starvation.
Materials and Methods
Study Organism and Rearing
The leaf beetle P. cochleariae was reared for several generations at 20°C, 16 h: 8 h light:dark, 70% r.h. in a climate cabinet and once a year crossed with field-collected beetles (51°51′21″ N, 8°41′37″ E). Groups of about 150 individuals were kept together in plastic rearing boxes (20 × 20 × 6.5 cm) with gauze lids. As food and for oviposition, leaves of Chinese cabbage (Brassica rapa spp. pekinensis) were provided. The plants were grown in a greenhouse (20°C, 16 h: 8 h light:dark, 70% r.h.) and only 7–10 weeks old non-flowering plants were used.
Isolation of Gregarines for Species Identification
The intestines of adult P. cochleariae were dissected under a light microscope (LM) (Zeiss Stemi, 2000, Carl Zeiss Microscopy, Jena, Germany) in physiological saline and gregarine trophozoites (feeding stages) were isolated with a hand-drawn glass pipette using an inverted microscope (Zeiss Axiovert A1, Carl Zeiss Microscopy, Jena, Germany). They were washed three times in autoclaved physiological saline before further morphological and molecular characterization. In addition, beetle feces were studied for the presence of gametocysts, which were isolated by a fine needle on a microscope slide in sodium phosphate buffer (0.1 M, pH = 7.2).
Morphological Characterization of Gregarine Species: Light and Scanning Electron Microscopy
Differential interference contrast (DIC) and phase contrast (PC) light micrographs of the gregarines were taken with a 5-megapixel CMOS camera AxioCam ERc 5s (Carl Zeiss Microscopy, Jena, Germany), attached to the inverted microscope (Zeiss Axiovert 1) and with a 2.3-megapixel Basler acA1920-40ucMED camera attached to a light microscope (Zeiss Axiophot, Carl Zeiss Microscopy, Jena, Germany). Measurements of the trophozoite, protomerite and deutomerite (Figure 1) were taken from around 40 specimens. For the measurements of the oocysts (n = 19), the oocysts were released from a gametocyst collected from feces of adult beetles.
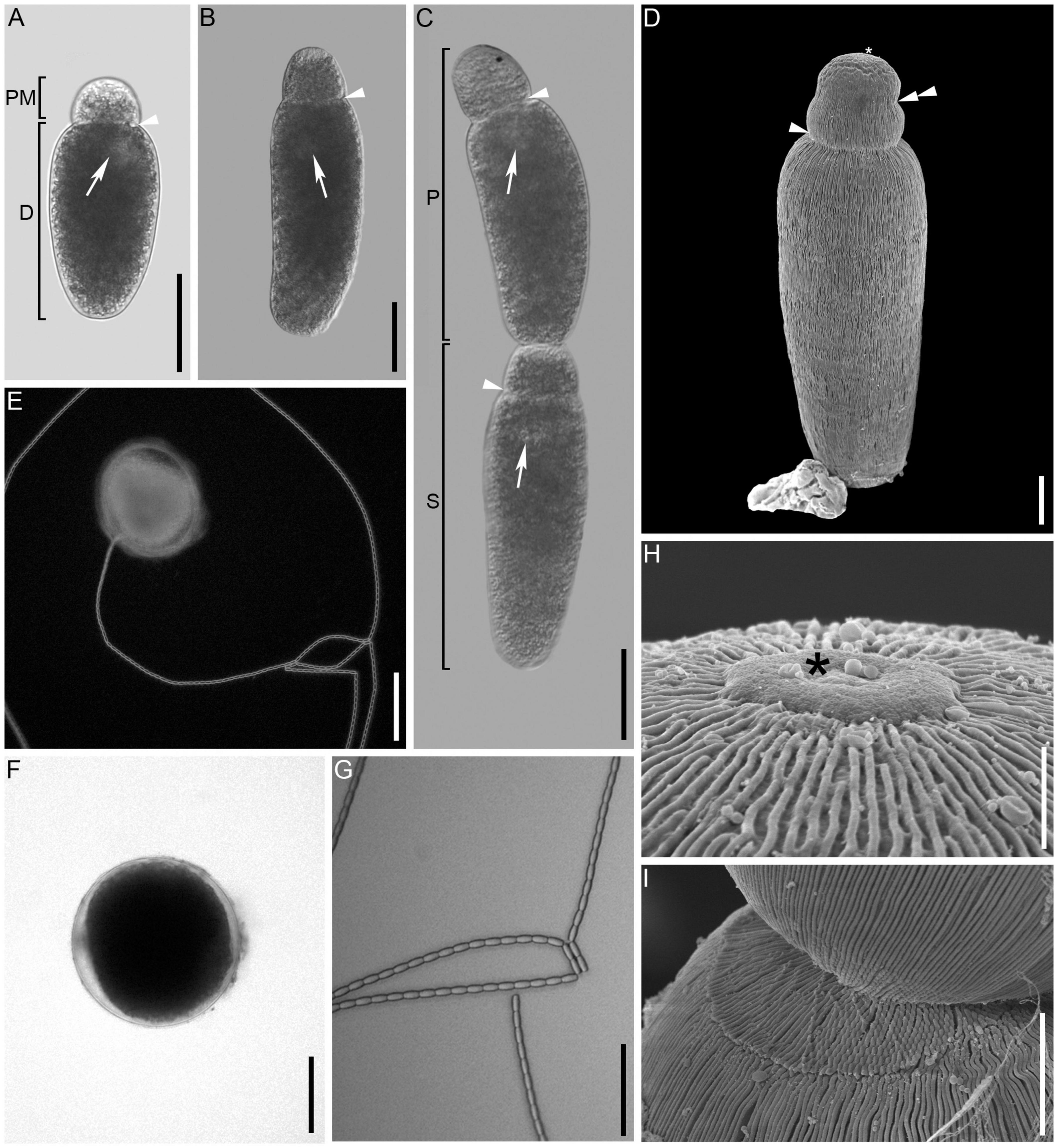
Figure 1. Light (differential interference contrast, bright, and darkfield) and scanning electron micrographs of Gregarina cochlearium sp. n. (A,B) General morphology of the solitary trophozoite with visible septum (arrowhead) separating the protomerite (PM) and deutomerite (D). Arrow points toward the nucleus. (C) Two gamonts in caudofrontal syzygy. The anterior gamont is the primite (P) and the posterior gamont is the satellite (S). (D) Scanning electron micrograph of trophozoite showing the epicytic folds running across the entire cell, the epimerite area (asterisk) and the indentation of the protomerite (double arrowhead). (E) Gametocyst extruding chain of oocysts. (F) Gametocysts in brightfield microscopy. (G) Chain of uniform, oblong oocysts. (H) High magnification view of the anterior end of the cell with button shape remnant of the epimerite (asterisk). (I) Attachment area of the two gamonts. Scale bars: (A–C,G) = 50 μm; (D) = 10 μm; (E,F) = 100 μm; (H) = 1.5 μm; (I) = 5 μm.
Around 40 specimens were prepared for scanning electron microscopy (SEM). Gregarines were fixed on 10 μm polycarbonate membrane filters (Millipore Corp., Billerica, MA, United States), following a standard protocol as described in Rueckert and Horák (2017). The filters were washed with water and dehydrated with a graded series of ethyl alcohol. Filters were critical point-dried with CO2, mounted on stubs, sputter coated with 5 nm of platinum, and viewed using a scanning electron microscope (Hitachi S-4300, Hitachi, Tokyo, Japan). Some SEM data were presented on a black background using Adobe Photoshop Version 22.5.1 (Adobe Systems Incorporated, San Jose, CA, United States).
Molecular Characterization of Gregarine Species: DNA Isolation, PCR, and Sequencing
The DNA of between 25 and 30 trophozoites was extracted using the DNeasy Blood and Tissue Kit (Qiagen, Hilden, Germany). The manufacturer’s protocol was changed slightly as only 40 μl of buffer AE were used for the DNA elution step to increase the DNA yield. Small subunit rDNA (SSU rDNA) sequences were PCR-amplified using a total volume of 25 μl containing 2 μl of primer, 2.5 μl of DNA template, 20.5 μl of dH2O and one PuReTaq Ready-to-go PCR Bead (GE Healthcare, Quebec, Canada). The SSU rDNA (∼1,800 bp) sequence of this species was amplified using universal eukaryotic PCR primers F1 5′-GCGCTACCTGGTTGATCCTGCC-3′ and R1 5′-GATCCTTCTGCAGGTTCACCTAC-3′ (Leander et al., 2003) and internal primers designed to match existing eukaryotic SSU sequences F2 5′-AAGTCTGGTGCCAGCAGCC-3′ and R2 5′-GCCTYGCGACCATACTCC-3′, F3 5′-TGCGCTACCTG GTTGATCC-3′ and R1. PCR was performed using PrimeG Gradient Thermal Cycler (Techne, Cambridge, United Kingdom) and the following protocol: 4 cycles of initial denaturation at 94°C for 4 min, 45°C for 1 min and 72°C for 1.45 min, 29 cycles of 94°C for 30 s (denaturation), 50°C for 1 min (annealing), 72°C for 1.45 min (extension), followed by a final extension period at 72°C for 10 min. PCR products corresponding to the expected size were gel-isolated using the GeneJET Gel Extraction Kit (Thermo Fisher Scientific, Waltham, United States). Cleaned PCR products were directly sequenced with ABI big dye reaction mix using vector primers and internal primers oriented in both directions. The new SSU rDNA sequence was initially identified by BLAST analysis and subsequently verified with molecular phylogenetic analyses (GenBank Accession number: OM286796).
Molecular Phylogenetic Analysis of Gregarine Species
The new SSU rDNA sequence was aligned with 59 other SSU rDNA sequences (1,460 aligned sites including gaps), which were the closest hits in the BLAST search of the NCBI database. They represented mainly the terrestrial gregarines, Cryptosporidium and the core Apicomplexa. Dinoflagellates were chosen as outgroup. The alignment was newly assembled using MUSCLE (Edgar, 2004) and visually checked in BioEdit (Hall, 1999). All analyses were performed using the CIPRES Science Gateway (Miller et al., 2010). The general time reversible (GTR) + G + I model was confirmed by the best fit model with jModeltest 2.1.10 under the Akaike Information Criterion (AIC) (Posada and Crandall, 1998; Darriba et al., 2012) and used with RAxML-HPC2 on XSEDE (Stamatakis, 2014) for Maximum-Likelihood (ML) analysis. ML bootstrap analyses were performed on 1,000 pseudo-replicates.
Bayesian analysis was performed using the program MrBayes 3.2.6 on XSEDE (Ronquist and Huelsenbeck, 2003). The program was set to operate with GTR + I + G, and four Monte Carlo Markov Chains (MCMC) starting from a random tree. A total of 2,000,000 runs were completed. Generations were calculated with trees sampled every 100 generations and the first 25% were discarded as burn-in. The parameters for the analyses were set to nst = 6, rates = invgamma, ngammacat = 4. The program was set to terminate at a standard deviation of split frequencies below 0.01. Posterior probabilities correspond to the frequency at which a given node was found in the post-burn-in trees. Pairwise distances for several clades were calculated based on uncut sequences using DNADIST version 3.5 in BioEdit (Hall, 1999).
Experimental Set-Up to Test Effects of Gregarine and Fluctuating Starvation on Life-History Traits of Beetles
To test consequences of a gregarine on its host under different environmental conditions, a full-factorial set-up was used with gregarines (G–, G+) and fluctuating starvation (S–, S+) as treatments, resulting in four treatment groups (G-S–, G-S+, G+ S–, G+ S+) with 67–70 replicates per group. For the experiment, cabbage leaves were offered for 24 h to the beetles in one rearing box. Then, eggs were randomly collected from these leaves. For gregarine infection we followed a protocol developed previously in our group (Wolz et al., 2022). Each egg was carefully cleaned from fecal residues and female secretions with a moist brush. Eggs were then randomly distributed to one of two gregarine treatment groups (uninfected and infected), kept each in a rearing box with fresh cabbage leaves. Shortly before larval hatching (after 6 days), individuals of the uninfected (G–) treatment group received cabbage leaves that had been mechanically damaged by knife cuttings (to provide leaves of comparable quality between treatment groups) and left for 48 h in a rearing box without any insects. Individuals of the gregarine-infected treatment (G+) group received cabbage leaves, which had served as food in rearing boxes with beetles for 48 h and were contaminated with feces from gregarine-infected conspecifics. Fecal residues contain oocysts with infectious sporozoites, which are ingested by the larvae and cause gregarine infection. Larvae of the two gregarine treatment groups received the respective food, which was replaced every 24 h, for 3 days.
On the fourth day after larval hatching, all individuals received untreated cabbage leaves, which were replaced every 48 h, until the end of the experiment (except during starvation periods). Within both gregarine treatment groups (G– and G+), larvae were subdivided into groups of five in Petri dishes (9 cm diameter) lined with filter paper. Half of the individuals of each of the gregarine treatment groups were fed ad libitum and assigned to the non-starvation treatment group (S–), the other half were starved three times (starvation group, S+), each time for a period of 24 h (day 4, day 7, and day 11 after larval hatching), as similarly performed in an earlier study with sawfly larvae (Paul et al., 2019). In the field, larvae may experience repeated bouts of starvation when their host plants are over-exploited by high population densities. To prevent any potential cannibalism and to keep up humidity, small moistened paper balls were added to the Petri dishes during the starvation periods, providing hiding places. However, cannibalism never occurred in this or former experiments with this species. To investigate the impact of this fluctuating starvation treatment and larval body mass on the number of gregarines, one larva was taken from each Petri dish on day 13 after larval hatching (n = 13 per group; for gregarine counting see below).
Remaining larvae that pupated were placed individually into Petri dishes (5 cm diameter) lined with filter paper. The day of adult eclosion was noted to determine the development time from larval hatch to reaching adulthood in dependence of the treatments. Two days after adult hatching, the beetles were sexed, weighed (micro balance, ME36S, Sartorius AG, Göttingen, Germany) and adult biomass used as further life-history parameter. Pairs of one male and one female were set up for mating within each treatment group (mating pairs: G-S–: n = 31, G-S+: n = 20, G+S–: n = 20, G+ S+: n = 10). The pairs remained together until the seventh day after adult hatching, after which the males were removed. The females were weighed again and the number of eggs laid was counted for each female for four consecutive days (from day 7 to 10 after adult hatching) as measure of fecundity. From larval hatching until the seventh day of adulthood, the number of individuals that had died were monitored daily to calculate the probability of survival. As the sex of larvae cannot be determined, these data were not separated by sex. The adults can usually live up to 3 months under laboratory conditions.
Counting Total Number of Gregarines in Hosts of the Different Treatments
Larvae taken for gregarine counting (see above) were weighed and frozen at –20°C (14 replicates per treatment group). To determine the total number of gregarines (only trophozoite stage) in the gut, the larvae were dissected, and their guts spread in sodium phosphate buffer (0.1 M, pH = 7.2) on microscope slides. The trophozoites were counted at 200–400 times magnification (ZEISS Axiophot).
Statistical Analyses
The statistical processing and visualization of the data were performed with R (version 3.6.3, R Core Team, 2020) in RStudio (version 1.2.5033, RStudio Team, 2019). Model residuals were tested for normality and variance homogeneity and stepwise backward deletion of non-significant interaction terms and predictors (F-test or Chisq test) was computed to obtain the minimum adequate models (package: MASS; Venables and Ripley, 2002). In the results section, only the statistical values of the predictors that remained in the final models are reported. The effects of gregarine treatment, starvation treatment and their interaction on development time and number of eggs laid by females were tested using generalized linear models [GLMs: Poisson distribution and identity link function (development time) or link log function (egg number)]. Treatment effects on the body mass of adult beetles were analyzed separately for males and females by linear models (LMs: Gaussian distribution, identity link function). The treatment effects on survival data were analyzed by a stratified cox model to control for proportional hazard assumption (package: survival, Therneau, 2020). The effects of starvation treatment, insect body mass and their interaction on the number of gregarines were tested using a GLM (Poisson distribution, identity link function).
Nomenclatural Acts
The electronic edition of this article conforms to the requirements of the amended International Code of Zoological Nomenclature, and hence the new name contained herein is available under that Code from the electronic edition of this article. This published work and the nomenclatural acts it contains have been registered in ZooBank, the online registration system for the ICZN. The ZooBank LSIDs (Life Science Identifiers) can be resolved, and the associated information viewed through any standard web browser by appending the LSID to the prefix ‘‘http://zoobank.org/.’’ The LSID for this publication is: urn:lsid:zoobank.org:pub:1DC2C7E0-9515-4ADC-B216-0EEA3111C745. The electronic edition of this work was published in a journal with an ISSN, and has been archived and is available from the following digital repositories: CLOCKSS, Edinburgh Napier Repository.1
Results
Morphological Characteristics of Gregarina cochlearium sp. n.
The main gregarine stages that were observed were solitary trophozoites (Figures 1A,B,D) or gamonts in syzygy (Figure 1C) in the gut of the beetles as well as gametocysts (Figures 1E,F) in the feces. The trophozoites were elongated with narrowly doliform to oblong deutomerites and broadly ovoid to oblong protomerites. Trophozoites were 109–334 μm long (194 ± 58.7 μm, mean ± SD, n = 38) and 38–118 μm wide (61 ± 15.6 μm). The protomerite was 22–56 μm in length (32 ± 7.3 μm) and 27–65 μm in width (41 ± 8.3 μm) with the deutomerite being 85–279 μm long (159 ± 51.3 μm) and 38–118 μm wide (see trophozoite width measurement above). The protomerite had sometimes a slight indentation, situated roughly in its middle (Figure 1D). The usually spherical nucleus (diameter: 18 ± 3.9 μm; range: 14–33 μm, n = 30) was mostly situated in the anterior or posterior third of the trophozoite (Figures 1A–C). Gamonts paired up in caudo-frontal syzygy (Figure 1C), with the satellite being attached with its anterior end to the posterior end of the so-called primate (Figure 1I). Scanning electron microscopy revealed epicytic folds of 3–4-folds/μm across the length of the cell (Figures 1D,H,I). There was a visible button-like structure, where the epimerite had been located (Figure 1H). Gametocysts (Figures 1E,F) isolated from the feces were whitish in color with a darker interior and measured 124–226 μm in diameter (176 ± 26.8 μm, n = 19; Figure 1F). Oocysts extruded in a single chain from the gametocyst (Figure 1E). The oocysts (Figure 1G) were very uniform, oblong, and measured 7.9–9.5 μm in length (8.8 ± 0.5 μm, n = 22) and 3.6–4.9 in width (4.1 ± 0.3 μm, n = 22). Trophozoites as well as gamonts in syzygy were capable of gliding.
Phylogenetic Analyses of Gregarine Species
Three terrestrial gregarine clades were recovered for Bayesian and ML analyses with high support (> 92/1.00): (1) terrestrial gregarine clade 1, consisting of mainly Gregarinoidea (except for Surculinium metchnikovi), (2) terrestrial gregarine clade 2, consisting of Stylocephaloidea, and (3) terrestrial gregarine clade 3, consisting of Actinocephaloidea (Figure 2). The sister clade to all terrestrial gregarines was a fully supported clade of Cryptosporidium species. The sequences of the core Apicomplexa Cytauxzoon felis and Theileria bicornis clustered basal to that. Terrestrial gregarine clades 1 and 2 formed together the sister clade to terrestrial gregarine clade 3. While there was mostly high statistical support for the main clades, some of the intermediate nodes had low statistical support and the branching order within especially terrestrial gregarine clade 1 could vary. This was also shown by the different branching pattern for the Bayesian analysis in this particular clade (some nodes were not supported). Our newly obtained sequence from the gregarine of P. cochleariae was most closely related to two sequences from an undescribed Gregarina species isolated from the closely related host Phaedon brassicae. This fully supported clade formed a sister clade to Gregarina coronata infecting Diabrotica undecimpunctata howardi and G. diabrotica infecting Acalymma vittatum (both Chrysomelidae). This clade of gregarines isolated from Chrysomelidae beetles clustered as sister group to a clade of gregarines infecting Tenebrionidae beetles, namely G. cloptoni, G. niphandrodes, and G. polymorpha. At the base of all Apicomplexa sequences clustered a clade of Chytridiomycota from environmental samples and freshwater algae, which is possibly an artifact.
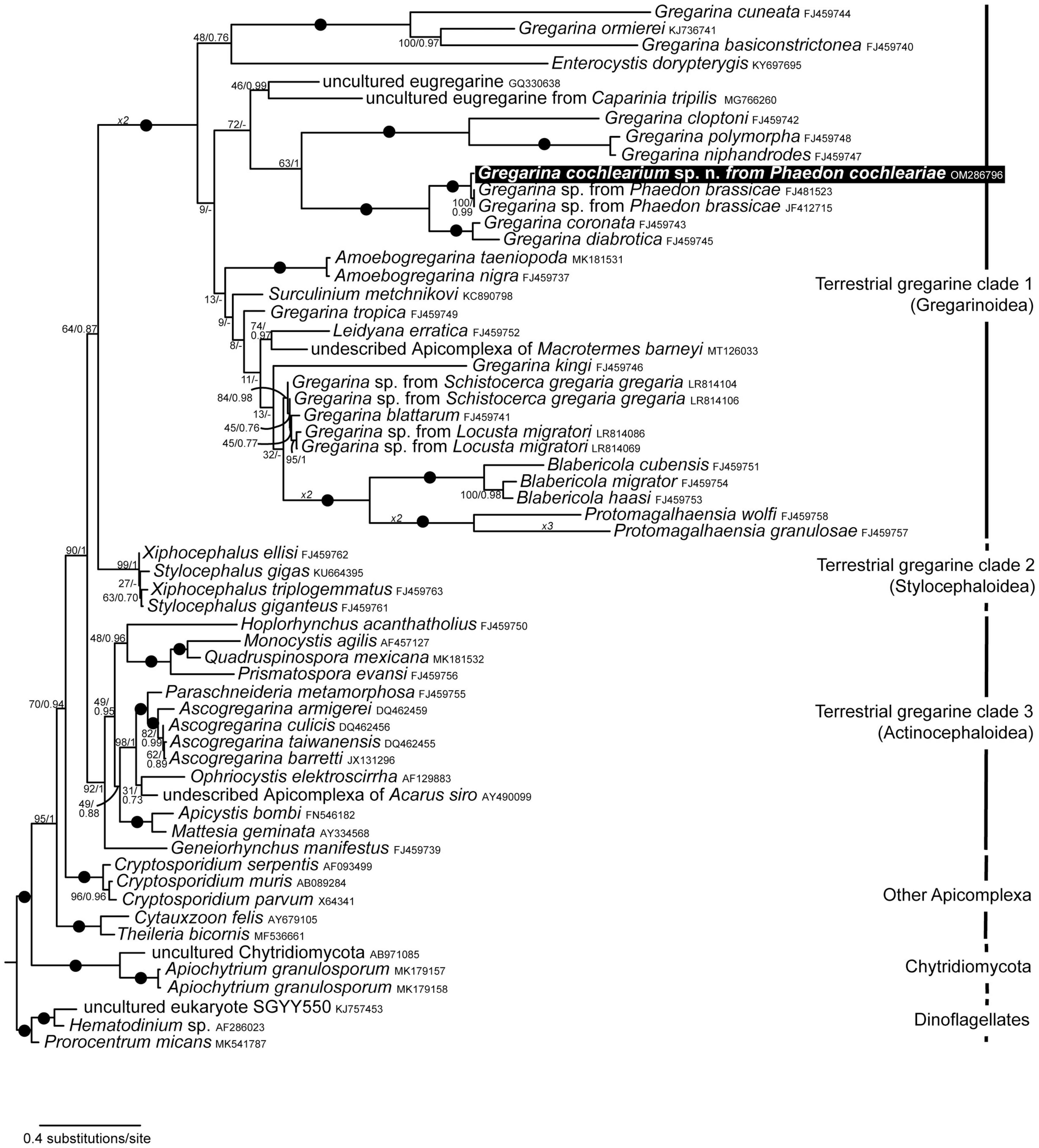
Figure 2. Maximum Likelihood (ML) tree derived from phylogenetic analyses of the 60-taxon dataset (1,460 aligned sites including gaps) of small subunit (SSU) rDNA sequences. This tree was inferred using the GTR + G + I substitution model. ML Bootstrap values and Bayesian posterior probabilities are provided. Black dots on branches denote values of 1. “–” denotes different branching topology for Bayesian analysis. The new sequence of Gregarina cochlearium sp. n. is highlighted in the black box.
Pairwise distance calculations revealed no divergence between the two sequences of Gregarina sp. from P. brassicae, but a 1.17% divergence to the new G. cochlearium sp. n. sequence from P. cochleariae. The sequence divergence of the new sequence in comparison to the two species from the sister clade was 9.98 and 11% for G. coronata and G. diabrotica, respectively.
Effects of Gregarine and Starvation Treatments on Life-History Traits
The development time from larval hatch to adult emergence was significantly influenced by the starvation treatment (GLM: X2 = 7.09, p < 0.01, 22–50 replicates per treatment group), but not by the gregarine infection. Starvation prolonged the development for several days in both uninfected and gregarine-infected larvae (Figure 3A).
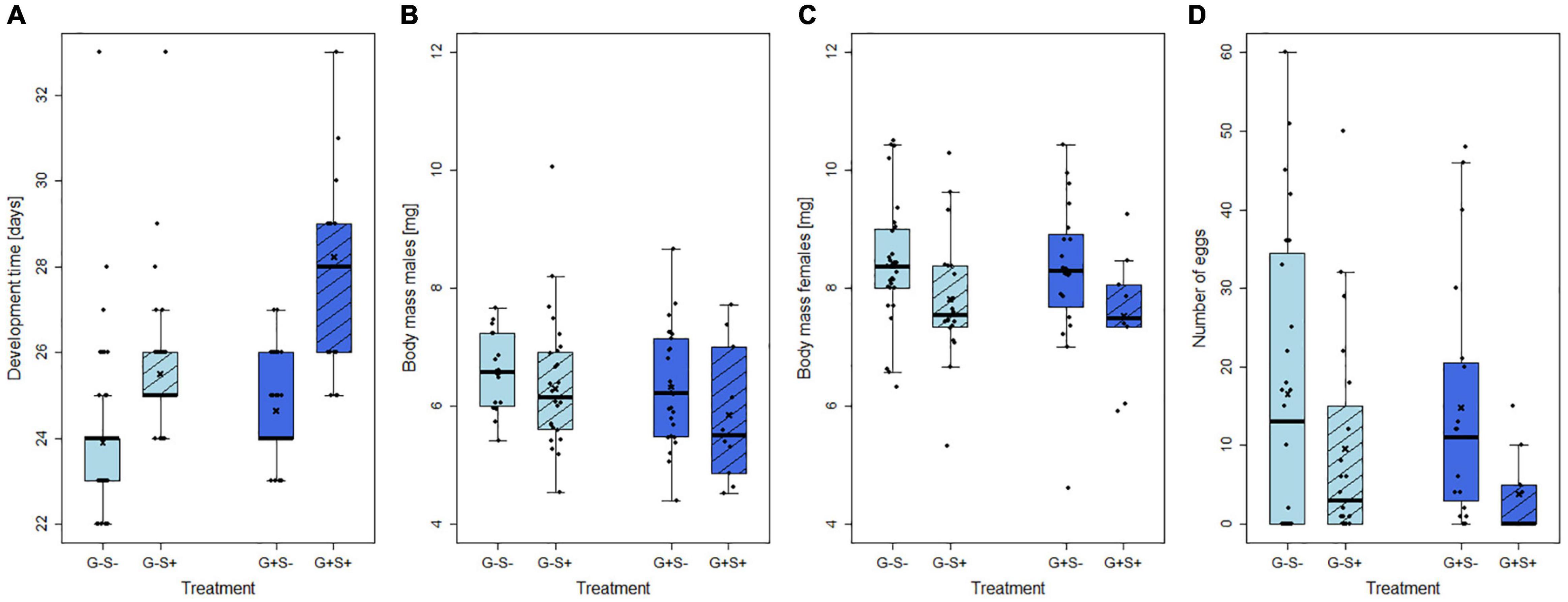
Figure 3. Effects of gregarine (G) treatment and starvation (S) treatment on (A) larval development time, (B) body mass of males and (C) females, and (D) number of eggs laid by females of Phaedon cochleariae. Data are presented as box–whisker plots with interquartile ranges (IQR) as boxes, including medians (horizontal lines), means (crosses), whiskers extending to the most extreme data points within 1.5 × IQR and raw data points.
The body mass of adult males was neither affected by the starvation treatment nor by the gregarine treatment (LM: F = 1.09, p > 0.05, 12–27 replicates per treatment group; Figure 3B). In contrast, the body mass of adult females was significantly reduced by on average almost 10% by starvation (LM: F = 21.83, p < 0.05, 9–31 replicates per treatment group; Figure 3C). The number of laid eggs was impaired by the interaction of gregarine infection and starvation treatment (GLM: X2 = 14.88, p < 0.001, 9–32 replicates per treatment group). Females that were gregarine-infected and starved laid the lowest average number of eggs. Compared to the infected females, the difference in egg number between starved and non-starved individuals was less pronounced (about 7 eggs) in uninfected females (Figure 3D).
Gregarine and starvation treatment had a significant interactive effect on the survival probability (cox model: X2 = 11.79, p < 0.001, 52–65 replicates per treatment group). Gregarine-infected and starved individuals showed the lowest survival probability, with half of them having died already at the beginning of the pupal stage (Figure 4). However, individuals from the gregarine-infected and non-starved treatment group (G+ S–) did not show an increased mortality.
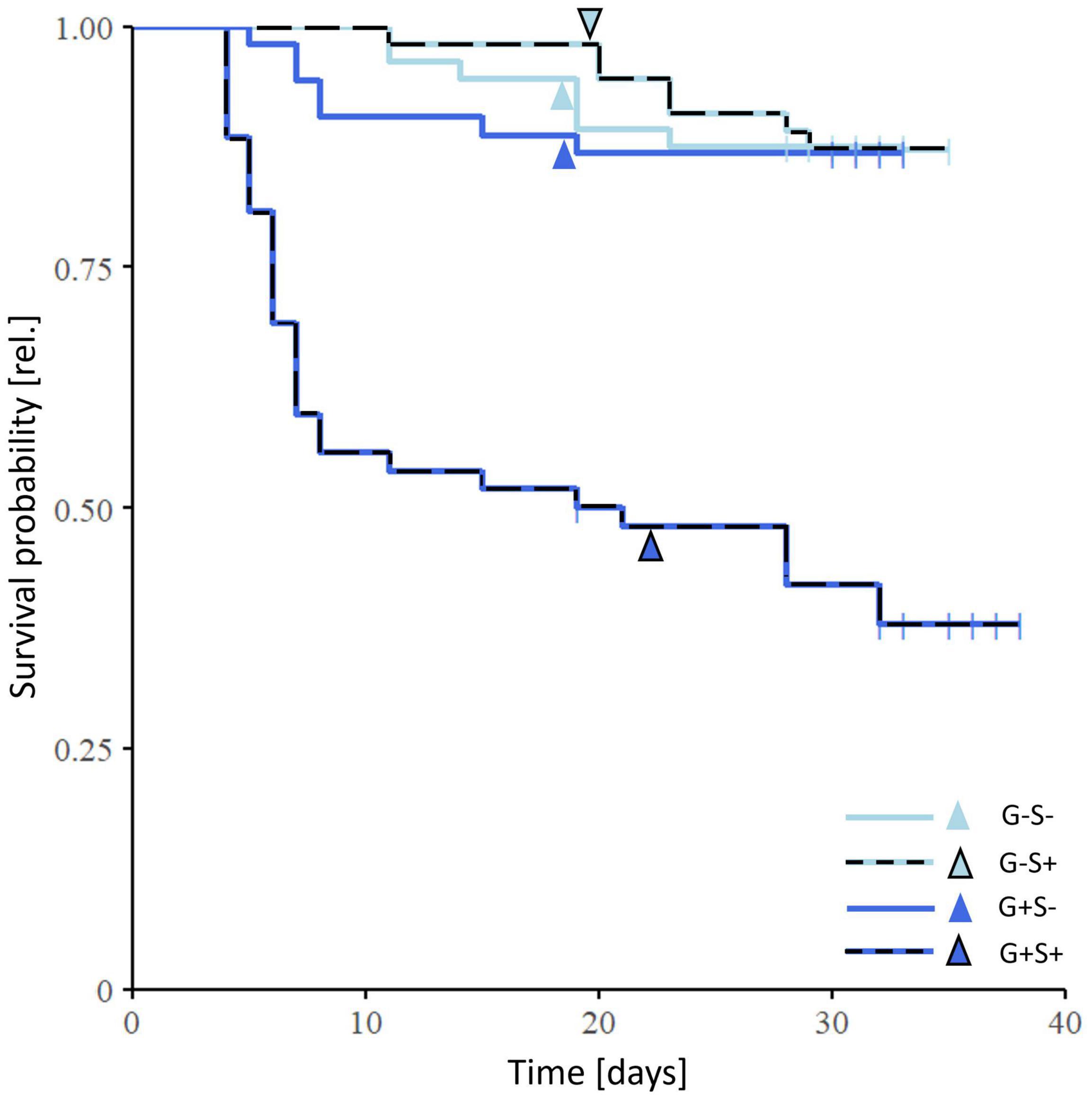
Figure 4. Effects of gregarine (G) treatment and starvation (S) treatment on survival of Phaedon cochleariae. The Kaplan-Meier curve shows the survival probability from larval hatching until 7 days after adult eclosion (52–56 replicates per treatment group). Vertical lines in the Kaplan–Meier survival curves indicate right censoring, arrows depict the mean timepoint of the beginning of the pupal stage.
Effects of Starvation Treatment and Larval Body Mass on Number of Gregarines
The number of gregarines in larvae was significantly influenced by the interaction of starvation treatment and larval body mass (GLM: X2 = 54.79, p < 0.001, 14 replicates per treatment group). In the non-starved larvae, the number of gregarines decreased with increasing body mass (Figure 5). In contrast, the number of gregarines in starved larvae was independent of their body mass and on average 33% higher than that in non-starved larvae. No gregarines were found in individuals of the uninfected (G–) treatment groups.
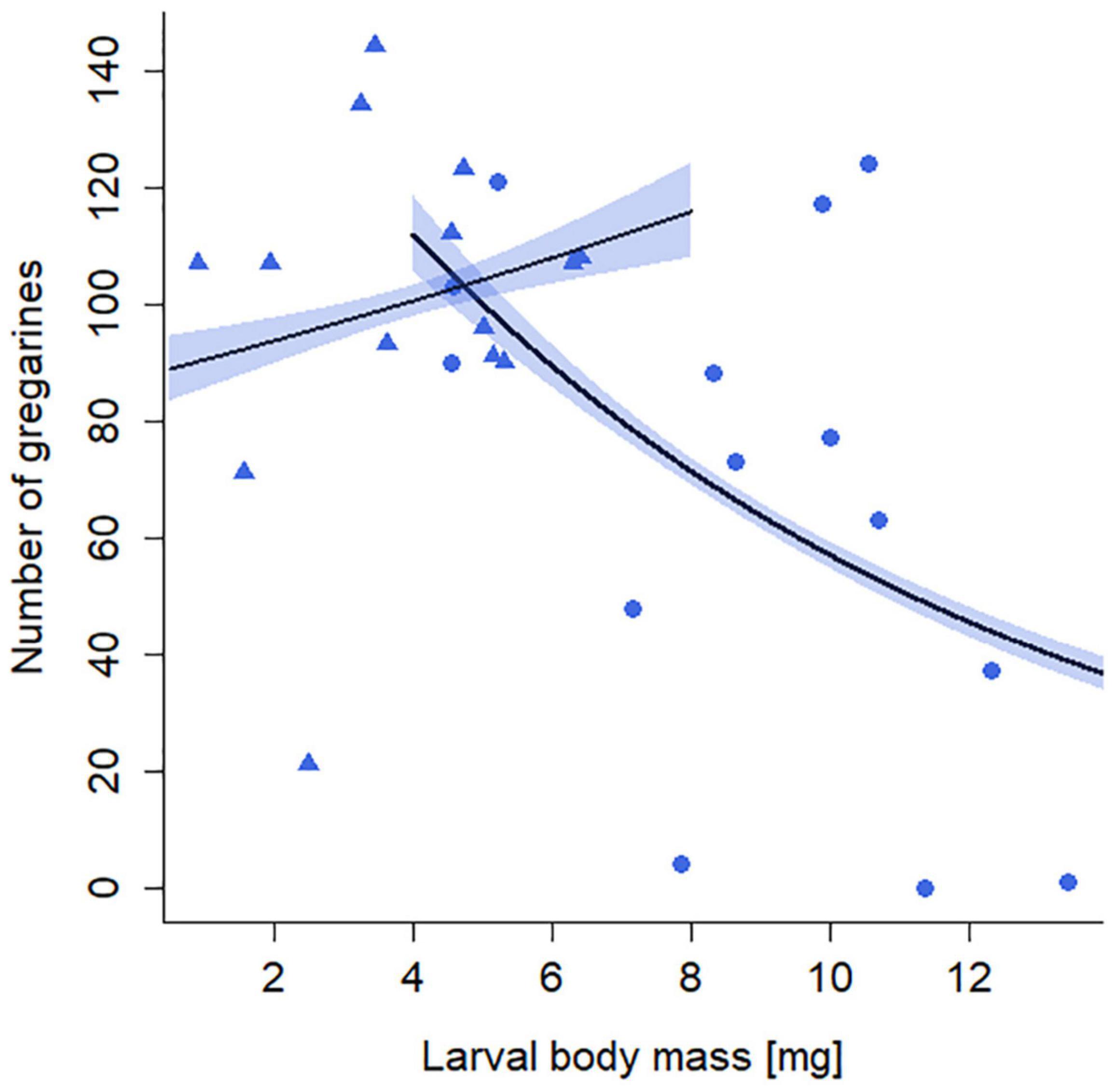
Figure 5. Effects of starvation (S) treatment and larval body mass on the number of gregarines in larval guts of Phaedon cochleariae. Larvae experienced either no starvation (blue circles) or starvation (blue rectangles). Lines represent model prediction of generalized linear model with associated SE depicted as colored polygons and overlaid with raw data points.
Discussion
Gregarines are naturally occurring endosymbionts in many terrestrial invertebrates (Schreurs and Janovy, 2008) and their prevalence is likely greatly underexplored (Levine, 1988). We identified a new gregarine species, Gregarina cochlearium sp. n., of the leaf beetle P. cochleariae and shed light on their interaction under ad libitum and fluctuating starvation conditions. Furthermore, we emphasize the role of host-endosymbiont relationships under fluctuating environmental conditions in an evolutionary and ecological context.
Morphological and Molecular Data Support New Gregarine Species
There are around 32 gregarine species that have been described for about 130 beetle species of the family Chrysomelidae (Desportes and Schrével, 2013). The most widespread by geographical means and host organisms is G. munieri, infecting roughly 90 Chrysomelidae species (Desportes and Schrével, 2013). Only two SSU rDNA sequences of gregarines infecting Chrysomelidae beetles are available on GenBank which belong to the species G. coronata and G. diabrotica both infecting D. undecimpunctata in the United States. In addition, there are two SSU rDNA sequences available from an undescribed gregarine species, Gregarina sp. isolated from P. brassicae in South Korea. An overview of morphological characteristics of these different gregarine species is provided in Table 1.
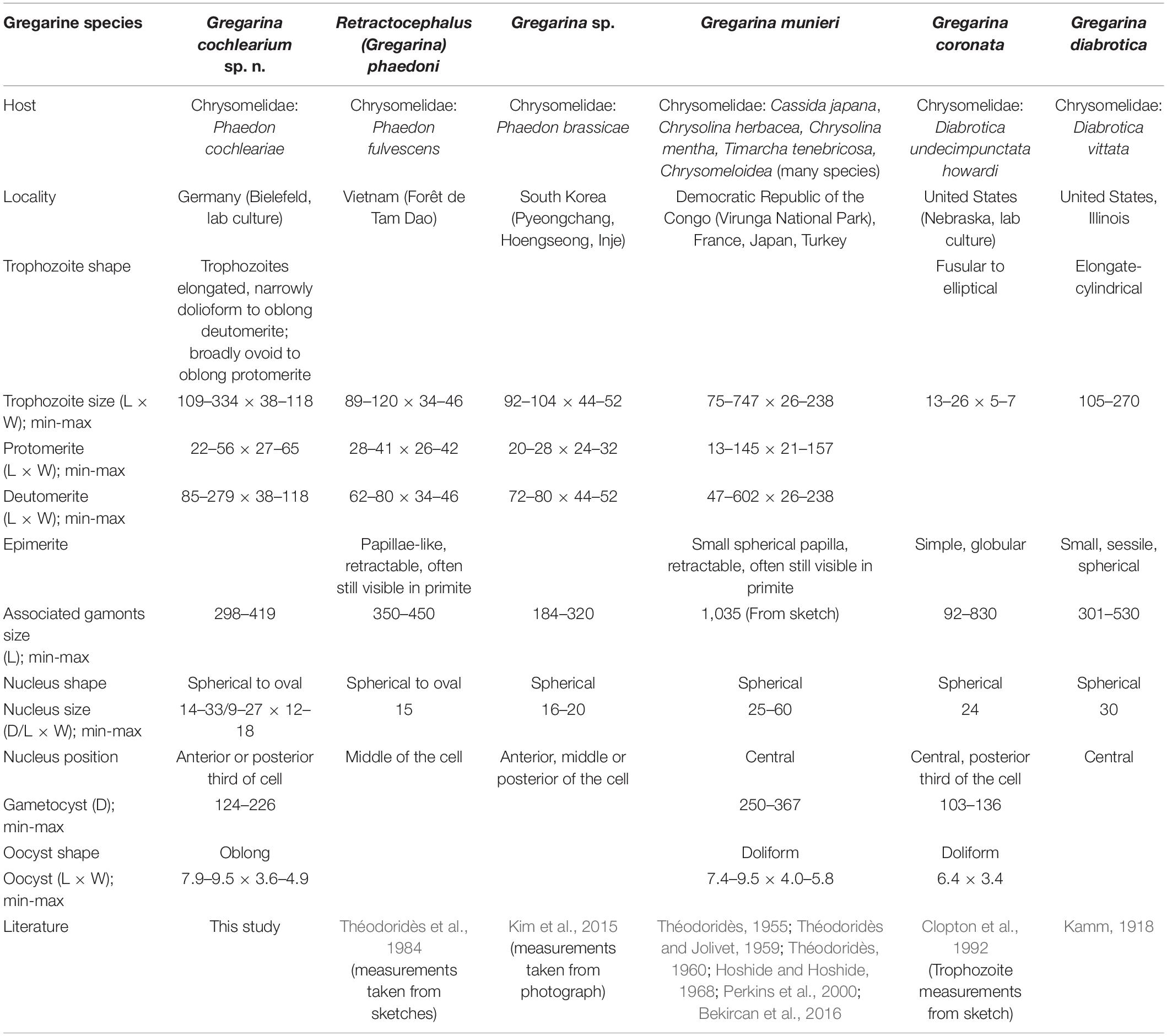
Table 1. Morphological comparisons of Gregarina cochlearium sp. n. and relevant, related species (D, Diameter; L, Length; W, Width, all measurements in μm).
In addition to Gregarina sp. from P. brassicae, there is only one further gregarine species known from another Phaedon species, Retractocephalus phaedoni previously known as Gregarina phaedoni from P. fulvescens in Vietnam (Théodoridès et al., 1984). While the overall cell shape of the new species G. cochlearium sp. n. is similar to Gregarina sp., morphological details and measurements are lacking as Gregarina sp. is only described as square-shaped and about 100 μm long (Kim et al., 2015). Measurements taken from the provided photograph in Kim et al. (2015) show that trophozoites as well as gamont associations are overall smaller than those of G. cochlearium sp. n. (Table 1). The trophozoite length and nucleus diameter of R. phaedoni are smaller compared to G. cochlearium sp. n., but the overall length of the syzygy stages is quite similar (Table 1). Other morphological differences to this species are based on the papillae-like epimerite that can be retracted, and is often still visible in the primite of the syzygy stage (Théodoridès et al., 1984), which could not be observed for G. cochlearium sp. n. The described constriction in the protomerite and deutomerite of R. phaedoni could only be detected in the protomerite of G. cochlearium sp. n. Depending on the literature, the measurements of the morphological characteristics for G. munieri differ considerably (e.g., Théodoridès and Jolivet, 1959; Perkins et al., 2000; Table 1), reaching overall larger sizes than measurements for the trophozoites of G. cochlearium sp. n. Cells of G. coronata have a slenderer appearance than G. cochlearium sp. n., trophozoites are a lot smaller, but associated gamonts are larger and have a larger nucleus (Clopton et al., 1992; Table 1). The gametocysts and oocysts in contrast are smaller. The name of G. coronata is based on the apical crown in the gamonts (Clopton et al., 1992), which has similarities to the indentation of the protomerite that can be found in several species infecting Chrysomelidae beetles, including G. cochlearium sp. n. Measurements for G. diabrotica are not clearly provided in the original description (Kamm, 1918), but trophozoites seem smaller compared to G. cochlearium sp. n., with similar sized associations and a larger nucleus. Clopton et al. (1992) did not provide additional measurements in their comparison between the three species G. coronata, G. munieri and G. diabrotica, but the phylogenetic analyses show a clear distinction of the latter species.
The new sequence from G. cochlearium sp. n. clustered with all four sequences from G. coronata, G. diabrotica and Gregarina sp. (2x) infecting Chrysomelidae beetles in a fully supported clade embedded in the “Terrestrial gregarine clade 1,” which consists of sequences from gregarines in the superfamily Gregarinoidea. The two sequences from Gregarina sp. from P. brassicae formed the closely related sister clade to G. cochlearium sp. n. from P. cochleariae. Together these three sequences clustered as sister group to G. coronata and G. diabrotica. This pattern had previously been shown for just one sequence of Gregarina sp. from P. brassicae (Kim et al., 2014). While there was no sequence divergence between the two Gregarina sp. sequences, the divergence of these two sequences to G. cochlearium sp. n. was 1.17%. The sequence divergence between these three Phaedon-infecting species and G. coronata and G. diabrotica was about 10 times higher (9.98–11.00%). Although the sequence divergence between G. cochlearium sp. n. and Gregarina sp. was quite a bit lower, it was similar to the sequence divergence of other distinct species and even genera in the phylogenetic tree, e.g., Amoebogregarina nigra and Amoebogregarina sp. (1.26%), Xiphocephalus ellisi and Stylocephalus giganteus (0.72%), Ascogregarina taiwanensis, A. culicis, and A. barrettii (between 0.64 and 1.11%).
Host specificity is still under debate and the majority of gregarines infecting Chrysomelidae beetles have been described from only one host species with a few exceptions (Desportes and Schrével, 2013). For the gregarine species that have been described from multiple host species no sequence data are available, thus these identifications rely on morphological similarities only. Based on the combined results from the morphological and phylogenetic analyses, as well as the sequence divergence calculations and the different host species, there is sufficient evidence that justifies the establishment of the new species Gregarina cochlearium sp. n.
Fluctuating Starvation Conditions Modulate Host-Endosymbiont Relationship
Our experimental set-up revealed that gregarine infections do not influence the development and adult body mass of P. cochleariae. This supports findings of previous studies showing that in many insect species a gregarine infection is benign or there are no apparent effects (Bollatti and Ceballos, 2014; Alarcón et al., 2017; Arcila and Meunier, 2020). Thus, the colonization of the intestine with gregarines is not necessarily associated with fitness costs, suggesting that the hosts may be sufficiently tolerant (Råberg et al., 2009) or that gregarines may affect their host’s fitness as little as possible to increase their own fitness (Jokela et al., 2005). However, impairments of the development of the host, such as a reduced larval growth or weight gain of the adults, have been found in several gregarine-infected insect species without further manipulated host conditions (Gigliolli et al., 2016), but also under suboptimal feeding conditions (Harry, 1967; Zuk, 1987). Thus, effects of gregarine infection on their hosts are obviously very species-specific, both with respect to the gregarines and their host.
In line with our expectation, starvation prolonged the development time and resulted in a lower adult body mass of P. cochleariae females. Similarly, only females of Melitaea cinxia (Lepidoptera: Nymphalidae) had a reduced body mass in response to larval food scarcity (Rosa and Saastamoinen, 2017), while in other insect species, such as Athalia rosae (Hymenoptera: Tenthredinidae), both sexes showed a significantly reduced adult body mass after food deprivation every fourth day during larval development, similar to the treatment applied in the present study (Paul et al., 2019). Differences in the responses of both sexes may be related to different life-histories of females and males (Boggs, 2009). However, several insects are obviously able to compensate for poor larval food conditions, for example by prolonging their developmental time, and thereby can reach the same pupal or adult mass as non-starved individuals (Saastamoinen et al., 2013; Rosa and Saastamoinen, 2017), as found here for the males of P. cochleariae. A compensatory ability when dealing with alternating or constant conditions of poor vs. high quality food has been demonstrated earlier for this species (Tremmel and Müller, 2013; Müller and Müller, 2016). The molecular regulation and physiological mechanisms of such compensation are only poorly understood (McCue et al., 2017). Potential costs for this compensation ability, as previously found in damselflies (De Block and Stoks, 2008), did not become evident under our laboratory conditions. However, under field conditions a prolonged development means that the larvae are potentially longer exposed to predation, imposing ecological costs (Häggström and Larsson, 1995; Dmitriew and Rowe, 2011).
In accordance with the lower body mass of adult P. cochleariae that had experienced starvation in early life, these females also laid less eggs, which may be related to fewer resources available for egg production for lighter females (Beukeboom, 2018). The fecundity of various insect species is reduced when experiencing limited resources during early ontogeny (Matos et al., 2017; Rosa and Saastamoinen, 2017; Xu et al., 2019). In our study, the number of eggs was lowest when females were both starved as larvae and gregarine-infected. A reduced reproductive success of the host may be the indirect consequence of a drain of host resources by the endoparasite (Bouwma et al., 2005; Krams et al., 2015). As a result, the energy demand of reproduction may be higher in gregarine-infected females compared to non-infected females.
In addition to drastic effects due to the combined gregarine exposure and larval starvation on female reproduction of P. cochleariae, individuals that experienced both challenges also suffered the highest mortality. In contrast, starved earwigs (Forficula auricularia; Dermaptera: Forficulidae) with gregarine infection had an advantage over uninfected individuals (Arcila and Meunier, 2020). However, in the latter study adults were exposed to starvation, a developmental stage that may be less sensitive to starvation periods in combination with gregarine infection than larvae. Larvae may have less energy and fat reserves to withstand starvation and prioritize primarily on development and growth. The finding that gregarine infection alone did not harm the leaf beetle survival, but that it led to a parasitic relationship in combination with harsh environmental conditions (i.e., starvation) is in line with our initial hypothesis. Likewise, a sublethal insecticide exposure lowered survival most in gregarine-infected P. cochleariae, indicating parasitism (Wolz et al., 2022). The idea of a symbiotic spectrum between host and gregarine (Rueckert et al., 2019) is thus supported for P. cochleariae and its gregarine endosymbiont G. cochlearium sp. n. under fluctuating environmental conditions.
An increased negative influence of gregarines on their hosts is often correlated with a higher infection rate (Siva-Jothy and Plaistow, 1999; Rodriguez et al., 2007). Indeed, the number of gregarines was significantly higher in starved compared to non-starved individuals of P. cochleariae. Starved larvae had a lower body mass shortly before pupation, but nevertheless had a higher gregarine load than the heavier, ad libitum fed larvae. Different fluctuating environmental conditions can affect the gregarine load of the hosts (Hupało et al., 2014; Kudô et al., 2019; Wolz et al., 2022). For example, gregarine loads change with season in dragonfly species (Locklin and Vodopich, 2010), or lead to a reduced gregarine infection with higher ambient temperatures in Phlebotomus sergenti (Diptera: Psychodidae) (Jancarova et al., 2016), potentially due to a disruption of the gregarines’ life cycle at high temperatures (Kolman et al., 2015). The immune system of insects protects against potentially harmful parasites and may play a key role in influencing the host burden of gregarines (Kaunisto and Suhonen, 2013; Arcila and Meunier, 2020). Assuming environmental fluctuation influences the host and reduces the ability of energy allocation into immune functions, a lower immune defense capacity of the host may benefit the endoparasites (Seppälä et al., 2008; Sadd and Schmid-Hempel, 2009). In turn, a decline in the resources available to the host could directly limit the amount of resources available to the gregarines. Whether this adversely affects the development of gregarines in the leaf beetle, for example through a lower production of infectious spores, is unclear and should be investigated in future studies. Moreover, P. cochleariae also contains various bacteria and yeasts, which have been shown to differ in abundance in dependence of the host plant quality of the insects (Müller et al., 2017). These and potential microbes of the gregarines themselves may have affected the development of P. cochleariae and G. cochlearium, as well as their interaction, which needs to be disentangled in future studies.
In summary, we describe here for the first time the gregarine species Gregarina cochlearium sp. n. that infects P. cochleariae. Further morphological and phylogenetic analyses and cross infection experiments are necessary to draw conclusions on host-specificity and occurrence of gregarines in different insect species. The results of this work indicate that P. cochleariae can deal well with a gregarine infection, but under fluctuating suboptimal conditions the fitness of the beetle is severely reduced, turning a seemingly “neutral” into a parasitic interaction. To better protect insects from environmental challenges, it is necessary to understand the impacts of endosymbionts, because they are crucial components of the natural environment, may modulate responses to other impacts and thus influence the ecology and evolution of the host-endosymbiont assemblage.
Taxonomic Summary
Superphylum: Alveolata Cavalier-Smith, 1991.
Phylum: Apicomplexa Levine, 1980, emend. Adl et al., 2005.
Class: Conoidasida Levine, 1988.
Subclass: Gregarinasina Dufour, 1828.
Order: Eugregarinorida Léger, 1900.
Family: Gregarinidae Ellis, 1912.
Gregarina cochlearium sp. n. Wolz, Müller and Rueckert 2022.
Description: Trophozoites elongate 109–334 μm long and 38–118 μm wide, with narrowly dolioform to oblong deutomerites 85–279 μm long and broadly ovoid to oblong protomerites 22–56 μm long and 27–65 μm wide. Protomerite sometimes with a slight indentation, situated roughly in its middle. Round nucleus (diameter = 14–33 μm), mostly situated in the anterior or posterior third of the trophozoite/gamonts. Gamonts form caudo-frontal syzygy. Cell surface with 3–4-folds/μm epicytic folds. Gametocysts whitish in color, and 124–226 μm in diameter. Oocysts released in a single chain from the gametocyst. Oocysts uniform, oblong, 7.9–9.5 μm long and 3.6–4.9 μm wide.
Type locality: Lab culture, Bielefeld, Germany.
Type host: Phaedon cochleariae (Fabricius, 1792) (Arthropoda, Insecta, Coleoptera, Chrysomelidae).
Holotype: The name-bearing type of this species is the specimen illustrated in Figure 1A (ICZN, 1999, Articles 73.1.4).
LSID: urn:lsid:zoobank.org:pub:1DC2C7E0-9515-4ADC-B216-0EEA3111C745.
Etymology: The species epithet cochlearium refers to the host species Phaedon cochleariae.
Data Availability Statement
The new SSU rDNA sequence and all analyzed sequences in this study are deposited in GenBank and publicly accessible at https://www.ncbi.nlm.nih.gov/genbank/ (accession number: OM286796). Data and code for the bioassays are available in Dryad and Zenodo: doi: 10.5061/dryad.fxpnvx0tk; doi: 10.5281/zenodo.6092758.
Author Contributions
MW and CM conceived the project and designed the experiment. SR performed the molecular laboratory work and generated phylogenetic analyses. SR and MW provided morphological material for analyses and description. MW conducted the bioassays, analyzed the data statistically, and wrote the first version of the manuscript. All authors contributed to the interpretation and refinement of the manuscript and gave final approval for publication.
Conflict of Interest
The authors declare that the research was conducted in the absence of any commercial or financial relationships that could be construed as a potential conflict of interest.
Publisher’s Note
All claims expressed in this article are solely those of the authors and do not necessarily represent those of their affiliated organizations, or those of the publisher, the editors and the reviewers. Any product that may be evaluated in this article, or claim that may be made by its manufacturer, is not guaranteed or endorsed by the publisher.
Acknowledgments
We thank Christian Kaltschmidt for granting access to the microscope and Johannes Greiner for an introduction into the microscope software. We are grateful to Sophie Henning and Karent Melissa Rodriguez Perez for their help during the experimental phase of the study. The gardeners of Bielefeld University are thanked for help in plant rearing. We acknowledge the financial support of the German Research Foundation (DFG) and the Open Access Publication Fund of Bielefeld University for the article processing charge.
Footnotes
References
Åbro, A. (1971). Gregarines: their effects on damselflies (Odonata: Zygoptera). Insect Syst. Evol. 2, 294–300. doi: 10.1163/187631271X00301
Alarcón, M. E., Jara, A., Briones, R. C., Dubey, A. K., and Slamovits, C. H. (2017). Gregarine infection accelerates larval development of the cat flea Ctenocephalides felis (Bouché). Parasitology 144, 419–425. doi: 10.1017/s0031182016002122
Arcila, F., and Meunier, J. (2020). Friend or foe? The apparent benefits of gregarine (Apicomplexa: Sporozoa) infection in the European earwig. Int. J. Parasit. 50, 461–469. doi: 10.1016/j.ijpara.2020.01.007
Bekircan, Ç, Tokarev, Y. S., Tosun, O., and Baki, H. (2016). Detection of Neogregarine and Eugregarine (Apicomplexa) infections from Chrysolina herbacea (Duftschmid 1825) (Coleoptera: Chrysomelidae) in Turkey. Turk. J. Life Sci. 2, 59–64.
Bénard, A., Vavre, F., and Kremer, N. (2020). Stress & symbiosis: heads or tails? Front. Ecol. Evol. 8:9. doi: 10.3389/fevo.2020.00167
Beukeboom, L. W. (2018). Size matters in insects - an introduction. Entomol. Exp. Appl. 166, 2–3. doi: 10.1111/eea.12646
Boggs, C. L. (2009). Understanding insect life histories and senescence through a resource allocation lens. Funct. Ecol. 23, 27–37. doi: 10.1111/j.1365-2435.2009.01527.x
Boggs, C. L., and Freeman, K. D. (2005). Larval food limitation in butterflies: effects on adult resource allocation and fitness. Oecologia 144, 353–361. doi: 10.1007/s00442-005-0076-6
Bollatti, F., and Ceballos, A. (2014). Effect of gregarines (Apicomplexa: Sporozoa) on survival and weight loss of Victorwithius similis Beier, 1959 (Arachnida: Pseudoscorpiones). J. Invertebr. Pathol. 117, 13–18.
Bouwma, A. M., Howard, K. J., and Jeanne, R. L. (2005). Parasitism in a social wasp: effect of gregarines on foraging behavior, colony productivity, and adult mortality. Behav. Ecol. Sociobiol. 59, 222–233. doi: 10.1007/s00265-005-0028-5
Clopton, R. E. (2002). “The Gregarines: a generic level review,” in Illustrated Guide To The Protozoa, eds J. Lee, G. Leedale, D. Patterson, and P. C. Bradbury (Lawrence, KS: Society of Protozoologists), 205–288.
Clopton, R. E., and Gold, R. E. (1996). Host specificity of Gregarina blattarum von Siebold, 1839 (Apicomplexa: Eugregarinida) among five species of domiciliary cockroaches. J. Invertebr. Pathol. 67, 219–223. doi: 10.1006/jipa.1996.0036
Clopton, R. E., Percival, T. J., and Janovy, J. (1992). Gregarina coronata n. pp. (Apicomplexa: Eugregarinida) described from adults of the Southern Corn Rootworm, Diabrotica undecimpunctata howardi (Coleoptera: Chrysomelidae). J. Eukaryot. Microbiol. 39, 417–420. doi: 10.1111/j.1550-7408.1992.tb01474.x
Darriba, D., Taboada, G. L., Doallo, R., and Posada, D. (2012). JModelTest 2: more models, new heuristics and parallel computing. Nat. Methods 9, 772–772. doi: 10.1038/nmeth.2109
De Block, M., and Stoks, R. (2008). Short-term larval food stress and associated compensatory growth reduce adult immune function in a damselfly. Ecol. Entomol. 33, 796–801. doi: 10.1111/j.1365-2311.2008.01024.x
Desportes, I., and Schrével, J. (2013). Treatise On Zoology-Anatomy, Taxonomy, Biology. The Gregarines (2 Vols): The Early Branching Apicomplexa. Leiden: Brill, 616–620.
Dmitriew, C., and Rowe, L. (2011). The effects of larval nutrition on reproductive performance in a food-limited adult environment. PLoS One 6:6. doi: 10.1371/journal.pone.0017399
Edgar, R. C. (2004). MUSCLE: multiple sequence alignment with high accuracy and high throughput. Nucleic Acids Res. 32, 1792–1797. doi: 10.1093/nar/gkh340
Gigliolli, A. A. S., Julio, A. H. F., and Conte, H. (2016). The life cycle of Gregarina cuneata in the midgut of Tribolium castaneum and the effects of parasitism on the development of insects. Bull. Entomol. Res. 106, 258–267. doi: 10.1017/s0007485315001121
Häggström, H., and Larsson, S. (1995). Slow larval growth on a suboptimal willow results in high predation mortality in the leaf beetle Galerucella lineola. Oecologia 104, 308–315. doi: 10.1007/bf00328366
Hall, T. A. (1999). BioEdit: a user-friendly biological sequence alignment editor and analysis program for Windows 95/98/NT. Nucl. Acid. Res. 41, 95–98.
Harry, G. O. (1967). The effect of a eugregarine Gregarina polymorpha (Hammerschmidt) on the mealworm larva of Tenebrio molitor (L.). J. Protozool. 14, 539–547. doi: 10.1111/j.1550-7408.1967.tb02039.x
Hoshide, H., and Hoshide, K. (1968). Notes on the gregarines in Japan, 1. Gregarina ampullaria n. sp. from Altica caerulescens Baly and two other already known gregarines from Chrysomelidae. Bull. Fac. Educat. 18, 35–42.
Hupało, K., Rachalewski, M., Rachalewska, D., and Tończyk, G. (2014). Gregarine parasitism in two damselfly hosts: comparison between species, sexes, and sites (Odonata: Calopterygidae). Odonatologica 43, 199–211.
ICZN (1999). International Code of Zoological Nomenclature, 4th Edn. London: International Trust for Zoological Nomenclature.
Jancarova, M., Hlavacova, J., Votypka, J., and Volf, P. (2016). An increase of larval rearing temperature does not affect the susceptibility of Phlebotomus sergenti to Leishmania tropica but effectively eliminates the gregarine Psychodiella sergenti. Parasit. Vectors 9:553. doi: 10.1186/s13071-016-1841-6
Jokela, J., Taskinen, J., Mutikainen, P., and Kopp, K. (2005). Virulence of parasites in hosts under environmental stress: experiments with anoxia and starvation. Oikos 108, 156–164. doi: 10.1111/j.0030-1299.2005.13185.x
Kamm, M. W. (1918). New gregarines from Coleoptera. Am. Soc. Parasitol. 4, 159–163. doi: 10.2307/3271242
Kaunisto, K. M., and Suhonen, J. (2013). Parasite burden and the insect immune response: interpopulation comparison. Parasitology 140, 87–94. doi: 10.1017/s0031182012001369
Kim, J. I., Kwon, M., and Maharjan, R. (2015). Morphological and molecular-biological characteristics of two gregarine species (Eugregarinida: Gregarinidae) associated to Phaedon brassicae and Phyllotreta striolata (Coleoptera: Chrysomelidae). J. Asia Pac. Entomol. 18, 651–655. doi: 10.1016/j.aspen.2015.07.014
Kim, J. I., Min, J. S., Kwon, M., Choi, J. Y., and Lee, S. H. (2014). Morphological and molecular characterizations of the Gregarina sp (Apicomplexa: Protozoa) parasitizing on Phaedon brassicae (Coleoptera: Chrysomelidae). J. Asia Pac. Entomol. 17, 1–5. doi: 10.1016/j.aspen.2013.08.008
Klingenberg, C. P., Leigh, R. H. B., Keddie, B. A., and Spence, J. R. (1997). Influence of gut parasites on growth performance in the water strider Gerris buenoi (Hemiptera: Gerridae). Ecography 20, 29–36. doi: 10.1111/j.1600-0587.1997.tb00344.x
Kolman, J. A., Clopton, R. E., and Clopton, D. T. (2015). Effects of developmental temperature on gametocysts and oocysts of two species of gregarines Blabericola migrator and Blabericola cubensis (Apicomplexa: Eugregarinida: Blabericolidae) parasitizing Blaberid cockroaches (Dictyoptera: Blaberidae). J. Parasitol. 101, 651–657. doi: 10.1645/14-673
Krams, I. A., Krama, T., Trakimas, G., Kaasik, A., Rantala, M. J., and Škute, A. (2015). Reproduction is costly in an infected aquatic insect. Ethol. Ecol. Evol. 29, 74–84. doi: 10.1080/03949370.2015.1089943
Kudô, K., Hasegawa, M., Mateus, S., Zucchi, R., and Nascimento, F. S. (2019). Effect of seasonality on rates of gregarine infection in workers of a social wasp Polybia paulista (Hymenoptera. Vespidae). Neotrop. Entomol. 48, 368–372. doi: 10.1007/s13744-018-0649-9
Leander, B. S., Clopton, R., and Keeling, P. (2003). Phylogeny of gregarines (Apicomplexa) as inferred from small-subunit rDNA and β-tubulin. Int. J. Syst. Evol. Microbiol. 53, 345–354. doi: 10.1099/ijs.0.02284-0
Levine, N. D. (1988). Progress in taxanomy of the apicomplexa protoza. J. Protzool. 35, 518–520. doi: 10.1111/j.1550-7408.1988.tb04141.x
Locklin, J. L., and Vodopich, D. S. (2010). Patterns of gregarine parasitism in dragonflies: host, habitat, and seasonality. Parasitol. Res. 107, 75–87. doi: 10.1007/s00436-010-1836-8
Logan, J. D., Janovy, J., and Bunker, B. E. (2012). The life cycle and fitness domain of gregarine (Apicomplexa) parasites. Ecol. Model. 233, 31–40. doi: 10.1016/j.ecolmodel.2012.03.018
Matos, Y. K., Osborne, J. A., and Schal, C. (2017). Effects of cyclic feeding and starvation, mating, and sperm condition on egg production and fertility in the common bed bug (Hemiptera: Cimicidae). J. Med. Entomol. 54, 1483–1490. doi: 10.1093/jme/tjx132
McCue, M. D., Terblanche, J. S., and Benoit, J. B. (2017). Learning to starve: impacts of food limitation beyond the stress period. J. Exp. Biol. 220, 4330–4338. doi: 10.1242/jeb.157867
Miller, M. A., Pfeiffer, W., and Schwartz, T. (2010). “Creating the CIPRES Science Gateway for inference of large phylogenetic trees,” in Proceedings of the Gateway Computing Environments Workshop (GCE), 14 Nov. 2010. New Orleans, LA: IEE, 1–8.
Müller, C., Vogel, H., and Heckel, D. G. (2017). Transcriptional responses to short-term and long-term host plant experience and parasite load in an oligophagous beetle. Mol. Ecol. 26, 6370–6383. doi: 10.1111/mec.14349
Müller, T., and Müller, C. (2016). Adult beetles compensate for poor larval food conditions. J. Insect Physiol. 88, 24–32. doi: 10.1016/j.jinsphys.2016.02.009
Paul, S. C., Putra, R., and Müller, C. (2019). Early life starvation has stronger intra-generational than transgenerational effects on key life-history traits and consumption measures in a sawfly. PLoS One 14:e0226519. doi: 10.1371/journal.pone.0226519
Perkins, F., Barta, J., Clopton, R., Peirce, M., and Upton, S. (2000). “Phylum Apicomplexa. The illustrated guide to the protozoa,” in An Illustrated Guide to the Protozoa, eds J.J. Lee, G.F. Leedale, and P. Bradbury (Lawrence, KS: Allen Press Inc), 190–304.
Posada, D., and Crandall, K. A. (1998). MODELTEST: testing the model of DNA substitution. Bioinformatics 14, 817–818. doi: 10.1093/bioinformatics/14.9.817
R Core Team (2020). R: A Language And Environment For Statistical Computing. R Foundation for Statistical Computing, Vienna, Austria: Available online at: https://www.R-project.org/ (accessed October 28, 2020).
Råberg, L., Graham, A. L., and Read, A. F. (2009). Decomposing health: tolerance and resistance to parasites in animals. Philos. Trans. R. Soc. B-Biol. Sci. 364, 37–49. doi: 10.1098/rstb.2008.0184
Roberts, L. S., and Janovy, J. (2008). Foundations Of Parasitology, 8th Edn. Dubuque, IA: McGraw-Hill.
Rodriguez, Y., Omoto, C. K., and Gomulkiewicz, R. (2007). Individual and population effects of Eugregarine, Gregatina niphandrodes (Eugregarinida: Gregarinidae), on Tenebrio molitor (Coleoptera : Tenebrionidae). Environ. Entomol 36, 689–693. doi: 10.1603/0046-225x(2007)36[689:iapeoe]2.0.co;2
Ronquist, F., and Huelsenbeck, J. P. (2003). MrBayes 3: bayesian phylogenetic inference under mixed models. Bioinformatics 19, 1572–1574. doi: 10.1093/bioinformatics/btg180
Rosa, E., and Saastamoinen, M. (2017). Sex-dependent effects of larval food stress on adult performance under semi-natural conditions: only a matter of size? Oecologia 184, 633–642. doi: 10.1007/s00442-017-3903-7
Rueckert, S., and Horák, A. (2017). Archigregarines of the english channel revisited: new molecular data on Selenidium species including early described and new species and the uncertainties of phylogenetic relationships. PLoS One 12:24. doi: 10.1371/journal.pone.0187430
Rueckert, S., Betts, E. L., and Tsaousis, A. D. (2019). The symbiotic spectrum: where do the gregarines fit? Trends Parasitol. 35, 687–694. doi: 10.1016/j.pt.2019.06.013
Saastamoinen, M., Hirai, N., and van Nouhuys, S. (2013). Direct and trans-generational responses to food deprivation during development in the Glanville fritillary butterfly. Oecologia 171, 93–104. doi: 10.1007/s00442-012-2412-y
Sadd, B. M., and Schmid-Hempel, P. (2009). Principles of ecological immunology. Evol. Appl. 2, 113–121. doi: 10.1111/j.1752-4571.2008.00057.x
Schilder, R. J., and Marden, J. H. (2007). Parasites, proteomics and performance: effects of gregarine gut parasites on dragonfly flight muscle composition and function. J. Exp. Biol. 210, 4298–4306. doi: 10.1242/jeb.011114
Schreurs, J., and Janovy, J. (2008). Gregarines on a diet: the effects of host starvation on Gregarina confusa Janovy et al., 2007 (Apicomplexa: Eugregarinida) in Tribolium destructor Uyttenboogaart, 1933 (Coleoptera: Tenebrionidae) larvae. J. Parasitol. 94, 567–570. doi: 10.1645/GE-1325.1
Seppälä, O., Liljeroos, K., Karvonen, A., and Jokela, J. (2008). Host condition as a constraint for parasite reproduction. Oikos 117, 749–753. doi: 10.1111/j.0030-1299.2008.16396.x
Siva-Jothy, M. T., and Plaistow, S. J. (1999). A fitness cost of eugregarine parasitism in a damselfly. Ecol. Entomol. 24, 465–470. doi: 10.1046/j.1365-2311.1999.00222.x
Soghigian, J., Valsdottir, L. R., and Livdahl, T. P. (2017). A parasite’s modification of host behavior reduces predation on its host. Ecol. Evol. 7, 1453–1461. doi: 10.1002/ece3.2748
Stamatakis, A. (2014). RAxML version 8: a tool for phylogenetic analysis and post-analysis of large phylogenies. Bioinformatics 30, 1312–1313. doi: 10.1093/bioinformatics/btu033
Théodoridès, J. (1955). Contribution À L’étude Des Parasites Et Phorétiques Des Coléoptères Terrestres. Paris: Hermann & Cie Éditeurs.
Théodoridès, J. (1960). Parasites et phorétiques de coléoptères et de myriapodes de Richelieu (Indre-et-Loire). Ann. Parasitol. Hum. Comp. 35, 488–503. doi: 10.1051/parasite/1960354488
Théodoridès, J., and Jolivet, P. (1959). Eugrégarines parasites de Coléopterères. Explor. Parc Nat. Albert 8:95.
Théodoridès, J., Jolivet, P., and Desportes, I. (1984). Grégarines d’arthropodes du Nord-Viêtnam. Ann. Sci. Nat. 6, 7–69.
Tremmel, M., and Müller, C. (2013). The consequences of alternating diet on performance and food preferences of a specialist leaf beetle. J. Insect Physiol. 59, 840–847. doi: 10.1016/j.jinsphys.2013.05.009
Tsubaki, Y., and Hooper, R. E. (2004). Effects of eugregarine parasites on adult longevity in the polymorphic damselfly Mnais costalis Selys. Ecol. Entomol. 29, 361–366. doi: 10.1111/j.0307-6946.2004.00613.x
Venables, W. N., and Ripley, B. D. (2002). Modern Applied Statistics With S, 4 Edn. New York, NY: Springer.
Wolz, M., Schrader, A., Whitelaw, E., and Müller, C. (2022). Gregarines modulate insect responses to sublethal insecticide residues. Oecologia 198, 255–265. doi: 10.1007/s00442-021-05086-4
Xu, X. L., Lv, N. N., Shi, Q., Hu, X. S., and Wu, J. X. (2019). Reproductive adaptation in alate adult morphs of the English grain aphid Sitobion avenae under starvation stress. Sci. Rep. 9:8. doi: 10.1038/s41598-019-38589-5
Keywords: eugregarine, Gregarina cochlearium, host-endosymbiont relationship, feeding conditions, Phaedon cochleariae, phylogeny, species description
Citation: Wolz M, Rueckert S and Müller C (2022) Fluctuating Starvation Conditions Modify Host-Symbiont Relationship Between a Leaf Beetle and Its Newly Identified Gregarine Species. Front. Ecol. Evol. 10:850161. doi: 10.3389/fevo.2022.850161
Received: 07 January 2022; Accepted: 02 March 2022;
Published: 13 April 2022.
Edited by:
Astrid T. Groot, University of Amsterdam, NetherlandsReviewed by:
Olivia Hannah Hewitt, The University of Queensland, AustraliaJoël Meunier, UMR7 261 Institut de Recherche Sur la Biologie de l’Insecte (IRBI), France
Copyright © 2022 Wolz, Rueckert and Müller. This is an open-access article distributed under the terms of the Creative Commons Attribution License (CC BY). The use, distribution or reproduction in other forums is permitted, provided the original author(s) and the copyright owner(s) are credited and that the original publication in this journal is cited, in accordance with accepted academic practice. No use, distribution or reproduction is permitted which does not comply with these terms.
*Correspondence: Caroline Müller, Y2Fyb2xpbmUubXVlbGxlckB1bmktYmllbGVmZWxkLmRl