- 1École Pratique des Hautes Études, PSL Research University, Paris, France
- 2Sorbonne University, Centre National de la Recherche Scientifique (CNRS), EPHE, UMR METIS, Paris, France
The frequency and strength of biotic interactions are thought to be shaped by environmental conditions. In this study, we reviewed and discussed the potential effects of toxic chemicals in driving shifts along the parasite-mutualist continuum. Some parasites have the astonishing capacity to accumulate trace metals and organic pollutants from various taxa within freshwater, marine, and terrestrial ecosystems. Recent studies have provided evidence of clear benefits for the host: when exposed to contaminants, infected organisms exhibited reduced contamination levels, less severe oxidative stress, and histological alterations, as well as higher body condition and survival rate compared with their uninfected conspecifics. Such effects might arise when the costs of parasitism are lower than their benefits in specific environmental conditions. Assessing the potential outcomes for parasites exploiting contaminated hosts is a crucial but neglected issue, since ecotoxicological effects on parasites may alter interspecific relationships. We identified possible avenues for future research using innovative tools and long-term experimental manipulations of both parasitism and pollution to better understand how toxic chemicals can modulate the strength and direction of host-parasite interactions.
Introduction
Ecological relationships refer to interactions between organisms within their environment and are classified according to the outcomes for each partner (positive, negative, or neutral effects). The Red Queen hypothesis (Van Valen, 1973) stated that biotic interactions are key drivers of evolution through adaptation and counteracting adaptations in interacting species. Selection favors hosts that are able to avoid or resist infection (resistance) or attenuate the parasite-induced damages (tolerance), whereas parasites evolve to impede the host’s strategies to detect, reject, or neutralize them. Such strategies are costly (Rigby et al., 2002) and are selected as long as the reduction in damages outweighs the cost of parasites’ resistance or tolerance (Sheldon and Verhulst, 1996).
Instead of a binary view of parasites and mutualists, interactions between hosts and symbionts can be addressed as a continuum from mutualism to parasitism according to the outcomes for each partner’s fitness (beneficial or harmful effects, Thomas et al., 2000; Leung and Poulin, 2008). In this context, parasites may evolve to be less deleterious to their host because of direct advantages for the transmission, reproduction, and survival of parasites. For instance, parasites may temporally improve antipredator behavior (“bodyguard manipulation,” Maure et al., 2013), especially in the intermediate host, to avoid non-host predators or postpone host mortality until parasites reach an infective stage and survive predation by a definitive host (Médoc et al., 2009). Some parasites species may also endorse a protective role for the host against lethal parasitoids (Sternberg et al., 2011) due to within-host competition. Feather mites can play a commensalistic–mutualistic role by feeding mainly on fungi including pathogenic ones and thus by cleaning host feathers (Dona et al., 2019). Finally, vertically transmitted parasites may enhance the short-term reproductive output of the host (Haine et al., 2004).
In addition, indirect consequences, which are unrelated to parasites’ fitness, may provide hosts with unexpected advantages in specific environments. Fellous and Salvaudon (2009) have proposed the term “conditionally helpful parasites” and have postulated that adverse environmental conditions seem to drive transitions along the parasite–mutualist continuum. For instance, shell-degrading endoliths attenuate the mortality of mussels during heat stress because of shell discoloration (Zardi et al., 2016). Trypanosoma otospermophili confers advantages to young of the year on Richardson’s ground squirrels (Spermophilus richardsonii) in a pyridoxine-deficient environment through the synthesis of vitamin B6 (i.e., pyridoxine, Munger and Holmes, 1988). This possible shift toward mutualism is more likely to occur for mild parasites with low-intensity infection, causing few damages (Fellous and Salvaudon, 2009).
Environmental pollution is a major and worldwide issue, which is likely to affect both hosts and parasites. During recent years, a couple of studies have yielded exciting results on the potential benefits of helminth parasites for hosts exposed to pollutants (Sánchez et al., 2016; Sures et al., 2017; Morrill et al., 2019; Molbert et al., 2020, 2021). This review aims to explore the potential effect of toxic chemicals in shifting interspecific relationships from parasitic to mutualistic. We review (i) the large body of studies showing that some parasites can accumulate pollutants and we discuss possible mechanisms. We then investigate the potential consequences of parasitism on (ii) the pollutant burden of the host and (iii) the costs and benefits for hosts in contaminated environments. In addition, we explore (iv) costs and benefits for parasites. We construct (v) a conceptual framework and we consider (vi) the consequences at the population and community levels. Finally, (vii) we propose future research directions.
Accumulation of Pollutants in Parasites
A Widespread Phenomenon
Some parasites have the remarkable ability to accumulate pollutants from their host. This has been extensively studied for lead, a non-essential trace metal. Lead can reach the levels of 2,700 times higher in acanthocephalans (Pomphorhynchus laevis) than in the muscle of its fish host, the European chub (Squalius cephalus, Sures et al., 1994; Sures and Siddall, 1999). This considerable bioaccumulation of lead in parasites is far from unique. Additionally, sequestration has been described for other trace metal elements (Schludermann et al., 2003; Thielen et al., 2004), in a wide range of parasite species (acanthocephalans, cestodes, nematodes, and digeneans, Sures et al., 2017), and in hosts from marine, freshwater, and terrestrial habitats: mollusks (Littorina littorea) (Evans et al., 2001), perches (Perca fluviatilis), and eels (Anguilla anguilla) (Sures, 2001), sharks (Carcharhinus dussumieri) (Malek et al., 2007), amphibians (African common toads, Amietophrynus regularis, Akinsanya et al., 2020), cormorants (Phalacrocorax carbo, Barus et al., 2001), harbor porpoises (Phocoena, Szefer et al., 1998), rats (Scheef et al., 2000), and red foxes (Jankovskaì et al., 2010; Borkovcova et al., 2020). In situ observations, as well as experimental exposure studies (Sures and Siddall, 2003; Sures et al., 2003) confirm this ability of parasites to concentrate lead to such an extent that these helminths have been proposed as indicators of environmental quality (Sures, 2001).
Similar findings have been reported for organic pollutants (mainly persistent ones), but studies remain scarce because of analytical challenges in providing accurate methods to quantify chemical analytes, such as their metabolites, from a limited amount of biological samples. For instance, polychlorobiphenyls (PCBs) levels were significantly higher in acanthocephalans than in perch (Brázová et al., 2012), in intestinal cestodes, Caryophyllaeus laticeps, than in their host (bream, Abramis brama, Brázová et al., 2021), and in the intestinal nematode, Anisakis sp., than in European hake (Mille et al., 2020). This bioaccumulation was observed for organochlorine pesticides (OCPs), polybrominated diphenyl ethers, phthalates, and polycyclic aromatic hydrocarbons (PAHs) in acanthocephalans compared with the muscle of their chub host but not for pyrethroid insecticides (Molbert et al., 2020) or musk fragrances (Mille et al., 2020). Hence, the levels of a wide variety of toxic chemicals, with some exceptions, are higher in intestinal parasites than in their host.
Possible Underlying Mechanisms
Understanding the mechanisms underlying the transfer of pollutants in host-parasite systems has been virtually neglected in ecotoxicological studies. Pollutant properties are known to play a key role in bioaccumulation and bioamplification (Goutte et al., 2020). A noteworthy point is that parasites, unlike predators, do not necessarily occupy higher trophic levels compared with their host (Lafferty et al., 2008; Robinson et al., 2010). Some parasites, such as acanthocephalans, are absorptive feeders and passively assimilate nutrients through the tegument, thus being on a lower trophic level than their host, as highlighted by the depletion of the heavier nitrogen isotope (Nachev et al., 2017). Because of these passive mechanisms, parasites without a gastrointestinal tract are supposed to absorb preferentially small and water-soluble molecules (Persson et al., 2007; Le et al., 2016). However, the few studies conducted on this issue did not support an effect of molecular size and hydrophobicity on the accumulation of pollutants in parasites relative to their host (Persson et al., 2007; Brázová et al., 2012; Molbert et al., 2020). Interestingly, metabolic transformation rate plays a key role, with easily degradable pollutants, such as PAHs and phthalates, being preferentially stored in parasites compared with persistent organic pollutants (POPs, Molbert et al., 2020). This finding could be explained by the lower ability of invertebrates to degrade and eliminate organic pollutants compared with vertebrates (Livingstone, 1998). Moreover, acanthocephalans and chub tissues had similar levels of PAH metabolites and of phthalate metabolites, despite the high accumulation of parent compounds in parasites (Molbert et al., 2020), corroborating the hypothesis of a poor metabolization of pollutants in parasites and an uptake of pollutant metabolites readily biotransformed by their host. Individual characteristics, such as age and sex, are also known to greatly influence bioaccumulation processes. POPs bioaccumulate during growth (Vives et al., 2005) and can reach very high levels in long-lived vertebrates (Rowe, 2008). With that in mind, the observed bioaccumulation of pollutants in parasites is considerable for these short-living organisms.
Concerning lead in the acanthocephalan-chub system, a possible underlying mechanism has been proposed (Sures and Siddall, 1999; Sures et al., 2003). This trace metal is mainly taken up by the gills, transported to the liver through the bloodstream and then excreted into the intestine via the bile. Lead ions form organometallic complexes with steroids in bile, which are either eliminated via the feces or reabsorbed in the small intestine. By assimilating nutrients from the host intestine, acanthocephalans are thought to take up these bile complexes, accumulate lead, and reduce its availability to the host (Sures and Siddall, 1999; Sures et al., 2003).
Consequences of Parasites as Contaminant Sinks on the Pollutant Burden of the Host
Differences in Pollutant Load Between Infected and Uninfected Hosts
This enormous bioaccumulation in parasites may result in reduced bioavailability of chemicals inside their host, as reviewed for lead and other metals (Sures et al., 2017). Field studies as well as experimental infection and chemical exposure evidenced a lower level of contamination of infected individuals compared with uninfected ones due to the uptake of element trace metals by parasites (Sures and Siddall, 1999; Sures et al., 2003). Concerning organic pollutants, PCB levels in the liver and muscle tissues of acanthocephalan-infected perch were about 20 times and 3 times lower compared with uninfected fish, respectively (Brázová et al., 2012). These lower PCB levels in infected fish were also recently observed in another host-parasite system (cestode-bream) but only for some PCB congeners (Brázová et al., 2021). Dichloro-dyphenyl-trichloroethane (DDT) concentrations were two times higher for non-parasitized Mayan catfish, Ariopsis assimilis, compared with congeners infected by the larval digenean Mesostephanus appendiculatoides (Vidal-Martínez et al., 2003). Dogs with heartworm disease (Dirofilaria immitis, a nematode feeding directly on the host’s blood) also exhibited lower serum levels of PCBs, OCPs, and PAH compared with non-infected dogs (Henríquez-Hernández et al., 2016). Similar findings were found for PCBs, but not for musk fragrances (Mille et al., 2020).
However, studies do not always converge toward a drastic reduction of pollutant burden in infected individuals. In European shags (Phalacrocorax aristotelis), parasite load (intestinal nematode Contracaecum rudolphii) was negatively linked with plasmatic concentrations of perfluorooctanesulfonic acid (PFOS) but only in females (Carravieri et al., 2020). Similarly, selenium-mercury molar ratios, which emphasize the protectiveness of selenium on mercury toxicity, also decreased with increasing parasite load in female shags (Carravieri et al., 2020), thus complicating our understanding of parasite infection on the host contamination. Moreover, methyl mercury sequestration in nematodes was estimated to be negligible compared with tissue burden in double-crested cormorants (Phalacrocorax auritus, Robinson et al., 2010). Finally, no differences in pollutant load were found between infected and uninfected chubs (Molbert et al., 2020), although their acanthocephalan parasites sequestered organic pollutants. Similarly, experimental exposure to PAH did not elicit higher PAH levels in uninfected chubs compared with infected ones (Molbert et al., 2021). This could be explained by the high metabolic transformation rate of phthalates and PAHs in the vertebrates and thus a rapid elimination of pollutants in fish. Moreover, the weak parasitic load encountered in chubs could result in limited efflux compared with pollutant uptake (but see Brázová et al., 2021).
Possible Confounding Factors
Parasitism induces a cascade of physiological and ecological changes (metabolism, foraging ecology, and social interaction), which may in turn influence pollutants’ exposure and accumulation (Molbert et al., 2020). Moreover, naturally infected individuals may share specific characteristics: larger fish feed more on intermediate hosts (e.g., gammarid species), thus being more prone to be infected by acanthocephalans (Molbert et al., 2020) and being less likely contaminated because of the growth and trophic dilution of several organic pollutants (Goutte et al., 2020). Hence, the relationship between parasitism and the pollutant burden of the host may be not causal. Similarly, physiological ability to cope with stressors, behavioral traits, or life-history traits could independently influence the probability of infection and of exposure to pollutants. Finally, most of these studies are transversal with individuals collected in situ at a single point in time. The “selection hypothesis” could thus not be excluded: phenotypes excessively sensitive to pollution or parasitism may have progressively disappeared, and the sampled individuals may be representative of the most robust fraction of the population. Specifically, it has been shown that parasites aggregate in a small proportion of individuals and that few organisms may bear a very high pollutant burden (Morrill et al., 2015). It is very likely that individuals experiencing both high contamination and high-intensity infections are in very poor health conditions and are not accessible during field sampling.
Costs and Benefits of Parasitism for the Host in the Polluted Environment
Several studies have reported additive and synergistic interactions between parasitism and pollution (Bustnes et al., 2006; Marcogliese and Pietrock, 2011), since some toxic chemicals may induce oxidative stress, depress the immune system, or disrupt endocrine functions, that may, in turn, favor parasitic infection and exacerbate their pathogenicity (Jobling and Tyler, 2003; Marcogliese, 2005; Morley et al., 2006; Sures, 2006). Joint effects of parasitism and pollution on host fitness are highly complex (Sures, 2008) and depend on the level of parasitism, as well as the level and mode of action of the environmental pollutant.
Recent findings suggest that some parasites, through their noteworthy ability to uptake pollutants, provide substantial benefits to their host. The first evidence came from an experimentally manipulated arsenic levels study under controlled conditions: arsenic-induced damages were attenuated in Artemia parthenogenetica infected by intestinal cestodes compared with uninfected ones (Sánchez et al., 2016). Acute mortality was reduced, the number of lipid droplets increased, and anti-oxidative defense mechanisms (catalase and glutathione reductase activity) were enhanced, without oxidative damage in parasitized compared with unparasitized Brine shrimps (Sánchez et al., 2016). Morrill et al. (2019) suggested that common eiders Somateria mollissima may benefit from intestinal helminths when exposed to lead: hens treated with an antihelminthic were more prone to being resighted the following year, with decreasing blood lead levels. Moreover, African common toads infected by the intestinal nematodes Amplicaecum africanum were not only less contaminated by trace metals (cadmium, lead, copper, nickel, and chromium) but also had less severe alterations of the intestinal mucosa and higher antioxidant activities (glutathione peroxidase, superoxide dismutase, and catalase) compared with uninfected conspecifics (Akinsanya et al., 2020). When infected by intestinal cestodes (C. laticeps), breams were in better body condition and less contaminated by PCBs (Brázová et al., 2021). Chubs infected by acanthocephalans exhibited lower oxidative damage than uninfected ones when naturally exposed to organic micropollutants (Molbert et al., 2020) and experimentally exposed to PAH (Molbert et al., 2021). Although these descriptive and experimental studies are still scarce, they support the hypothesis that some intestinal helminths, at low-intensity infections, could be less detrimental or even helpful for hosts exposed to trace metal elements or organic pollutants.
Costs and Benefits for Parasites in Polluted Environments
Pollutant exposure can directly or indirectly induce a wide array of costs and benefits for parasites (Marcogliese, 2005). First, toxic chemicals affect species differently and can be more deleterious for parasites compared with vertebrate hosts (Blanar et al., 2010) because of (i) a weaker tolerance to particular xenobiotics (e.g., fungicide, Hanlon and Parris, 2012) and (ii) the bioaccumulation rate and less efficient metabolization and excretion processes (Livingstone, 1998). Free-living stages and ectoparasites may also be particularly vulnerable to environmental pollution (Pietrock and Marcogliese, 2003; Blanar et al., 2009). As an example, abnormal attachment clamps were observed more frequently in parasites (diplozoons) from the gills of chubs and breams caught in the most polluted sites (Šebelová et al., 2002), suggesting pollution-induced morphological impairments. Harmful effects of the pollutant burden on parasites can lead to a lower infestation by parasites and/or higher mortality of adult forms in highly contaminated hosts, which could be indirectly beneficial for the host. In such a context, one may predict that parasites would adapt faster than their host under this toxic selection pressure because of the shorter generation time. However, such rapid microevolution was not demonstrated by a multigenerational experimental exposure to a globally marketed and used pesticide (Cuco et al., 2020).
On the other hand, pollution-tolerant parasites may benefit from environmental pollution since toxic compounds can impair the physiology and, particularly, the immune defenses of the host (Jokinen et al., 1995), thus weakening resistance to parasites (Valtonen et al., 1997). For instance, experimental exposure at sublethal concentrations to carbaryl, a neurotoxic pesticide, synergistically interacts with parasitism by enhancing parasite virulence (Coors et al., 2008). The impacts of pollutants’ bioaccumulation on parasites appear to be complex and context-dependent (Rohr et al., 2008). For instance, in a terrestrial host-parasite system, the establishment of the tapeworm Hymenolepis diminuta in beetles was either increased or reduced by the exposure to an insecticide depending on the timing of the contamination (Dhakal et al., 2020).
Finally, environmental pollution can positively or negatively impact the presence, density, or resistance of intermediate and final hosts (Valtonen et al., 1997), thus compromising parasite life cycles. Evaluating potential ecotoxicological consequences on parasites’ transmission, reproduction, or survival is thus a complex issue (Khan and Thulin, 1991; Sures et al., 2017). It is noteworthy that adverse impacts of pollutants on parasites could have cascading effects on ecological processes, ecosystem functioning, and services (Gómez and Nichols, 2013; Davis and Prouty, 2019).
Conceptual Framework
We propose a conceptual framework and we argue that parasites can be helpful (positive outcome for the host’s fitness) when the costs of parasitism (level of infestation and pathogenicity) are negligible compared with the benefits of pollutant sequestration (depending on the accumulation rate and toxicity for the host). The interaction may drastically change depending on levels of parasitism (or pathogenicity) and pollutant levels. When hosts experience high aggregation of parasites, their pathogenicity likely outweighs the potential benefits of pollutants sequestration (Figure 1). When environmental contamination exceeds the ability of parasites to sequester pollutants, ecotoxicological effects may be exacerbated by parasitism (Figure 1). This simplified model implies only one type of pollutant and one parasite species (Figure 1). We summarize the main environmental and ecological factors that can influence the host-parasite interaction in polluted environments (Figure 2), including (i) the type of pollutants’ mixture, their probabilities of occurrence, and their chemical properties (accumulation, metabolization, differential toxicity among species, etc.), (ii) the nature and intensity of parasites, tolerance to pollutants, the ability to sequester pollutants, life cycle (number of obligatory hosts), diversity of parasites’ species, and dynamics of co-infection, and (iii) host tolerance and resistance to parasites, tolerance to pollutants, individual traits (sex, age, body length, trophic ecology, etc.), and inter- and intra-specific relationships. All these biotic and abiotic factors can interact and it may be difficult to establish some predictions.
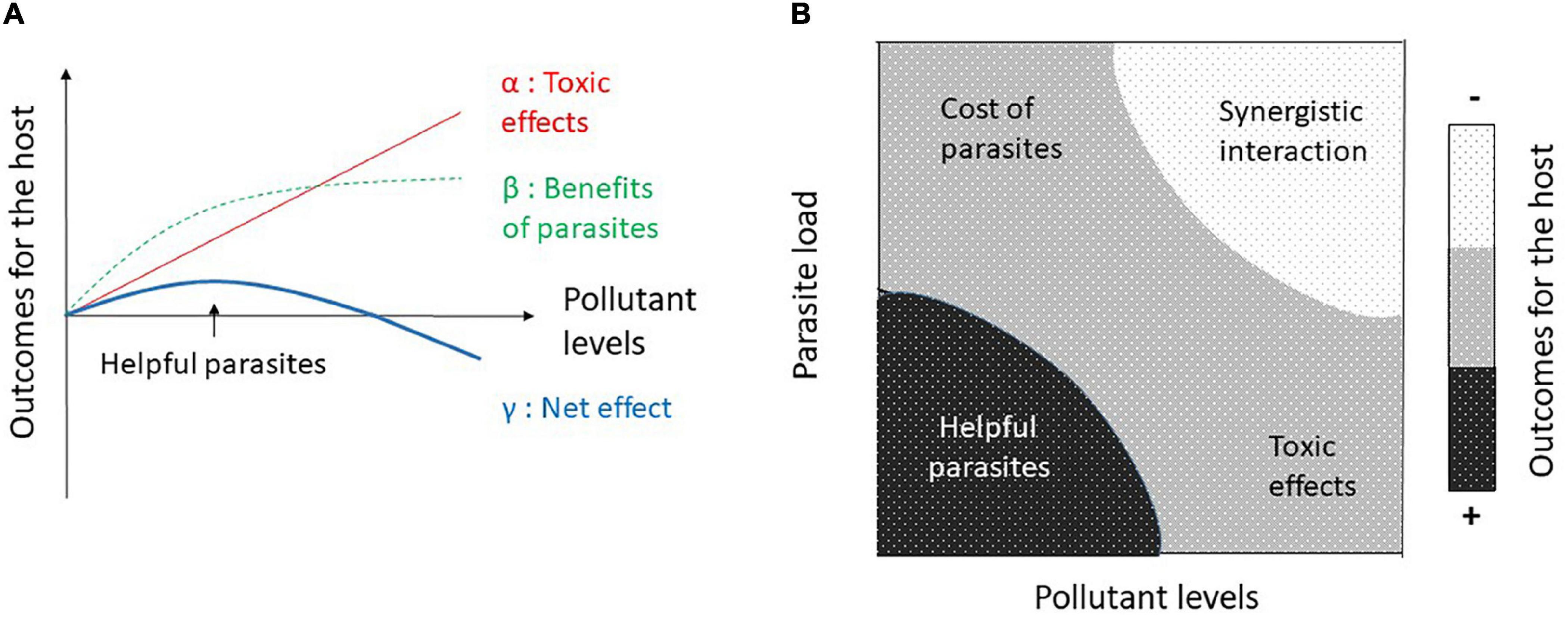
Figure 1. (A) Parasites can be helpful in polluted environments if toxic effects (α) are outweighed by the benefits of pollutant bioaccumulation by parasites (β). Net effects for the host (γ) vary according to pollutant levels. (B) Outcomes for the host also depend on parasite load since they induce costs and may synergistically interact with high environmental pollution. At low pollutant levels and parasite load, parasites may be helpful by buffering ecotoxicological effects. Slopes of all lines are purely arbitrary.
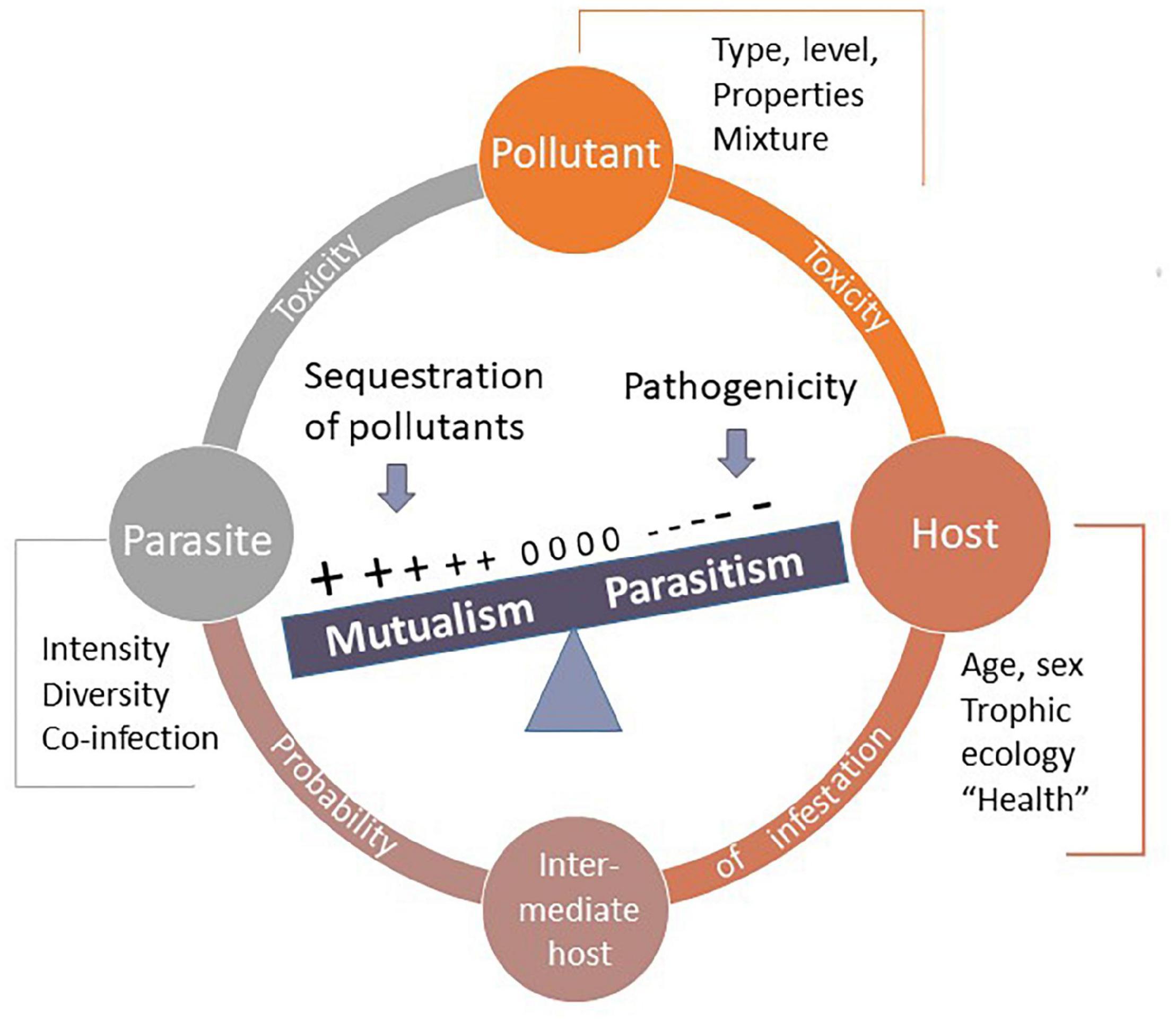
Figure 2. The strength and the direction of host-parasite interaction can be viewed as a balance between parasite-induced costs and benefits related to pollutant sequestration. The net benefit of parasites is influenced by three broad categories of factors (i) pollutant traits: type, mixture, levels, and chemical properties; (ii) parasite traits: nature, intensity, and diversity of parasites (such as, the risk of co-infection), sensitivity to pollutants, the ability to sequester pollutants, and life cycles; and (iii) host traits: tolerance and resistance to parasites, sensitivity to pollutants, individual traits, health, and ecology. These biotic and abiotic factors can interact, resulting in transitions along the parasite–mutualist continuum.
Population and Community and Level Consequences
Could context-dependent outcomes in host-parasite interactions have some impact on the dynamics and community structures of the host and parasite populations? This has been discussed for mutualistic interactions: costs, benefits, and thus net effects greatly vary in space and time, affecting geographical disparities in community structures as well as the rate of evolutionary change (Bronstein, 1994; Hay et al., 2004).
The consequences of context-dependent helpful parasites on host populations and communities have not been studied to the best of our knowledge. One may predict that, in a population facing environmental pollution, parasitism could be beneficial at a low-intensity infection but becomes detrimental as the parasite load increases.
In a quantitative meta-analysis, the effects of environmental pollution on parasite populations and communities have been explored (Blanar et al., 2009). Surprisingly, contaminants caused more damage to (i) monoxenous (single obligatory host in the life cycle) parasite taxa than to heteroxenous (at least two hosts) taxa and (ii) directly exposed (ectoparasites) than indirectly exposed (endo-) parasites (Blanar et al., 2009). These findings conflict with the assumptions of (i) stronger effects on parasites with complex life cycles due to potential altered transmission in intermediate hosts and (ii) an amplifying effect of pollutant bioaccumulation from the host gastrointestinal tract. While meta-analyses unveil the general pattern among functional groups, some specific responses have been evidenced depending on parasite ecology, pollutant levels, and direct and indirect mechanisms (Sures et al., 2017). For instance, parasite diversity and biomass of helminth species in fish, were much lower in a historically contaminated site (Oros and Hanzelová, 2009) not because of changes in fish communities or ecotoxicological effects of pollutant sequestration but probably as a consequence of pollution-related decline of intermediate hosts.
Future Directions
This review aimed to identify whether environmental contamination can modulate the direction and strength of host-parasite interactions. We argue that two conditions must be met to elicit a transition from parasitism to mutualism: (i) pollutant sequestration by parasites should provide greater advantages for the host than the cost of infection (Figure 1) and (ii) pollutant levels should not be deleterious for both partners (and intermediate host) to avoid profound changes in host–parasite interactions. Reciprocal and complex relationships are expected among pollutants, parasites, and hosts (Figure 2). As previously discussed, transversal studies could lead to biased assessments because of confounding factors. Hence, longitudinal studies must be favored to evaluate the long-term consequences of pollution and parasitism on host health as well as the effect of pollution on parasites’ establishment and development. In addition, appropriate experimental approaches are required to confirm the causal relationship: a simultaneous manipulation of parasitism and chemical exposure would be an elegant design. In addition, endoscopic procedures could be used to conduct the long-term monitoring of intestinal parasites in hosts experimentally exposed to contaminants. This method has been recently developed for seabirds to monitor intestinal parasitic infections without sacrificing hosts (Carravieri et al., 2020). This approach would thus allow assessing impacts of experimentally increased pollutant exposure on the infection rate and the developmental rate of parasites, as well as on the joint effect of parasitism (at different levels) and pollution (at different levels) on host health.
At the population level, it would be crucial to compare the host infection status according to environmental pollution without sacrificing individuals. A non-lethal and non-invasive method is the use of urine DNA (uDNA, Duval et al., 2021), but it requires methodological developments to be extended to several parasite species. This opens new avenues for research on parasitism in host populations exposed to environmental contamination.
Author Contributions
AG drafted the initial version of the manuscript. NM contributed to the revising of the manuscript. Both authors contributed to the article and approved the submitted version.
Funding
This work was financially supported by a grant from ITE-SU (Institut de la Transition Environnementale Sorbonne Université), the Agence de l’Eau Seine Normandie, and the Seine research program PIREN-Seine.
Conflict of Interest
The authors declare that the research was conducted in the absence of any commercial or financial relationships that could be construed as a potential conflict of interest.
Publisher’s Note
All claims expressed in this article are solely those of the authors and do not necessarily represent those of their affiliated organizations, or those of the publisher, the editors and the reviewers. Any product that may be evaluated in this article, or claim that may be made by its manufacturer, is not guaranteed or endorsed by the publisher.
References
Akinsanya, B., Isibor, P. O., Onadeko, B., and Tinuade, A. A. (2020). Impacts of trace metals on African common toad, Amietophrynus regularis (Reuss, 1833) and depuration effects of the toad’s enteric parasite, Amplicaecum africanum (Taylor, 1924) sampled within Lagos metropolis, Nigeria. Heliyon 6:e03570. doi: 10.1016/j.heliyon.2020.e03570
Barus, V., Tenora, F., Kracmar, S., and Prokes, M. (2001). Cadmium and lead concentrations in Contracaecum rudolphii (Nematoda) and its host, the cormorant Phalacrocorax carbo (Aves). Folia Parasitol. 48, 77–78. doi: 10.14411/fp.2001.012
Blanar, C. A., MacLatchy, D. L., Kieffer, J. D., and Munkittrick, K. R. (2010). Exposure to a mixture of zinc and copper decreases survival and fecundity of Discocotyle sagittata (Leuckart) parasitizing juvenile Atlantic salmon, Salmo salar L. Bull. Environ. Contam. Toxicol. 84, 692–697. doi: 10.1007/s00128-010-0024-y
Blanar, C. A., Munkittrick, K. R., Houlahan, J., MacLatchy, D. L., and Marcogliese, D. J. (2009). Pollution and parasitism in aquatic animals: a meta-analysis of effect size. Aquat. Toxicol. 93, 18–28. doi: 10.1016/j.aquatox.2009.03.002
Borkovcova, M., Fiser, V., Bednarova, M., Havlicek, Z., Adámková, A., Mlcek, J., et al. (2020). Effect of accumulation of heavy metals in the red fox intestine on the prevalence of its intestinal parasites. Animals 10:343. doi: 10.3390/ani10020343
Brázová, T., Hanzelová, V., and Miklisová, D. (2012). Bioaccumulation of six PCB indicator congeners in a heavily polluted water reservoir in Eastern Slovakia: tissue-specific distribution in fish and their parasites. Parasitol. Res. 111, 779–786. doi: 10.1007/s00436-012-2900-3
Brázová, T., Miklisová, D., Barčák, D., Uhrovič, D., Šalamún, P., Orosová, M., et al. (2021). Hazardous pollutants in the environment: fish host-parasite interactions and bioaccumulation of polychlorinated biphenyls. Environ. Pollut. 291:118175. doi: 10.1016/j.envpol.2021.118175
Bronstein, J. L. (1994). Conditional outcomes in mutualistic interactions. Trends Ecol. Evol. 9, 214–217. doi: 10.1016/0169-5347(94)90246-1
Bustnes, J. O., Erikstad, K. E., Hanssen, S. A., Tveraa, T., Folstad, I., and Skaare, J. U. (2006). Anti-parasite treatment removes negative effects of environmental pollutants on reproduction in an Arctic seabird. Proc. R. Soc. B: Biol. Sci. 273, 3117–3122. doi: 10.1098/rspb.2006.3687
Carravieri, A., Burthe, S. J., de la Vega, C., Yonehara, Y., Daunt, F., Newell, M. A., et al. (2020). Interactions between environmental contaminants and gastrointestinal parasites: novel insights from an integrative approach in a marine predator. Environ. Sci. Technol. 54, 8938–8948. doi: 10.1021/acs.est.0c03021
Coors, A., Decaestecker, E., Jansen, M., and De Meester, L. (2008). Pesticide exposure strongly enhances parasite virulence in an invertebrate host model. Oikos 117, 1840–1846. doi: 10.1111/j.1600-0706.2008.17028.x
Cuco, A. P., Wolinska, J., Santos, J. I., Abrantes, N., Gonçalves, F. J., and Castro, B. B. (2020). Can parasites adapt to pollutants? a multigenerational experiment with a Daphnia× Metschnikowia model system exposed to the fungicide tebuconazole. Aquat. Toxicol. 226:105584. doi: 10.1016/j.aquatox.2020.105584
Davis, A. K., and Prouty, C. (2019). The sicker the better: nematode-infected passalus beetles provide enhanced ecosystem services. Biol. Lett. 15:20180842. doi: 10.1098/rsbl.2018.0842
Dhakal, S., Meyling, N. V., Pedersen, K. E., Cedergreen, N., and Fredensborg, B. L. (2020). Timing of sub-lethal insecticide exposure determines parasite establishment success in an insect-helminth model. Parasitology 147, 120–125. doi: 10.1017/S0031182019001331
Dona, J., Proctor, H., Serrano, D., Johnson, K. P., Oploo, A. O. V., Huguet-Tapia, J. C., et al. (2019). Feather mites play a role in cleaning host feathers: new insights from DNA metabarcoding and microscopy. Mol. Ecol. 28, 203–218. doi: 10.1111/mec.14581
Duval, E., Blanchet, S., Quéméré, E., Jacquin, L., Veyssière, C., Lautraite, A., et al. (2021). Urine DNA (uDNA) as a non-lethal method for endoparasite biomonitoring: development and validation. Environmental DNA 3, 1035–1045. doi: 10.1002/edn3.228
Evans, D. W., Irwin, S. W. B., and Fitzpatrick, S. (2001). The effect of digenean (Platyhelminthes) infections on heavy metal concentrations in Littorina littorea. J. Mar. Biol. Assoc. UK 81, 349–350. doi: 10.1017/s0025315401003873
Fellous, S., and Salvaudon, L. (2009). How can your parasites become your allies? Trends Parasitol. 25, 62–66. doi: 10.1016/j.pt.2008.11.010
Gómez, A., and Nichols, E. (2013). Neglected wild life: parasitic biodiversity as a conservation target. Int. J. Parasitol. Parasites Wildlife 2, 222–227. doi: 10.1016/j.ijppaw.2013.07.002
Goutte, A., Alliot, F., Budzinski, H., Simonnet-Laprade, C., Santos, R., Lachaux, V., et al. (2020). Trophic transfer of micropollutants and their metabolites in an urban riverine food web. Environ. Sci. Technol. 54, 8043–8050. doi: 10.1021/acs.est.0c01411
Haine, E. R., Brondani, E., Hume, K. D., Perrot-Minnot, M. J., Gaillard, M., and Rigaud, T. (2004). Coexistence of three microsporidia parasites in populations of the freshwater amphipod Gammarus roeseli: evidence for vertical transmission and positive effect on reproduction. Int. J. Parasitol. 34, 1137–1146. doi: 10.1016/j.ijpara.2004.06.006
Hanlon, S. M., and Parris, M. J. (2012). The impact of pesticides on the pathogen Batrachochytrium dendrobatidis independent of potential hosts. Arch. Environ. Contam. Toxicol. 63, 137–143. doi: 10.1007/s00244-011-9744-1
Hay, M. E., Parker, J. D., Burkepile, D. E., Caudill, C. C., Wilson, A. E., Hallinan, Z. P., et al. (2004). Mutualisms and aquatic community structure: the enemy of my enemy is my friend. Annu. Rev. Ecol. Evol. Syst. 35, 175–197. doi: 10.1146/annurev.ecolsys.34.011802.132357
Henríquez-Hernández, L. A., Carretón, E., Camacho, M., Montoya-Alonso, J. A., Boada, L. D., Valerón, P. F., et al. (2016). Influence of parasitism in dogs on their serum levels of persistent organochlorine compounds and polycyclic aromatic hydrocarbons. Sci. Total Environ. 562, 128–135. doi: 10.1016/j.scitotenv.2016.03.204
Jankovskaì, I., Miholovaì, D., Bejček, V., Vadlejch, J., Šulc, M., Szaìkovaì, J., et al. (2010). Influence of parasitismon trace element contents in tissues of red fox (Vulpes vulpes) and its parasites Mesocestoides spp. (Cestoda) and Toxascaris leonina (Nematoda). Arch. Environ. Contam. Toxicol. 58, 469–477. doi: 10.1007/s00244-009-9355-2
Jobling, S., and Tyler, C. R. (2003). Endocrine disruption, parasites and pollutants in wild freshwater fish. Parasitology 126, S103–S107.
Jokinen, E. I., Aaltonen, T. M., and Valtonen, E. T. (1995). Subchronic effects of pulp and paper mill effluents on the immunoglobulin synthesis of roach, Rutilus rutilus. Ecotoxicol. Environ. Saf. 32, 219–225. doi: 10.1006/eesa.1995.1107
Khan, R. A., and Thulin, J. (1991). Influence of pollution on parasites of aquatic animals. Adv. Parasitol. 30, 201–238. doi: 10.1016/s0065-308x(08)60309-7
Lafferty, K. D., Allesina, S., Arim, M., Briggs, C. J., De Leo, G., Dobson, A. P., et al. (2008). Parasites in food webs: the ultimate missing links. Ecol. Lett. 11, 533–546. doi: 10.1111/j.1461-0248.2008.01174.x
Le, T. Y., Nachev, M., Grabner, D., Hendriks, A. J., and Sures, B. (2016). Development and validation of a biodynamic model for mechanistically predicting metal accumulation in fish-parasite systems. PLoS One 11:e0161091. doi: 10.1371/journal.pone.0161091
Leung, T. L. F., and Poulin, R. (2008). Parasitism, commensalism, and mutualism: exploring the many shades of symbioses. Vie et Milieu/Life Environ. 58, 107–115.
Livingstone, D. R. (1998). The fate of organic xenobiotics in aquatic ecosystems: quantitative and qualitative differences in biotransformation by invertebrates and fish. Comp. Biochem. Physiol. Part A: Mol. Int. Physiol. 120, 43–49. doi: 10.1016/s1095-6433(98)10008-9
Malek, M., Haseli, M., Mobedi, I., Gangali, M. R., and Mackenzie, K. (2007). Parasites as heavy metal bioindicator in the shark carcharhinusdussumieri from the Persian Gulf. Parasitology 2007, 1053–1059. doi: 10.1017/S0031182007002508
Marcogliese, D. J. (2005). Parasites of the superorganism: are they indicators of ecosystem health? Int. J. Parasitol. 35, 705–716. doi: 10.1016/j.ijpara.2005.01.015
Marcogliese, D. J., and Pietrock, M. (2011). Combined effects of parasites and contaminants on animal health: parasites do matter. Trends Parasitol. 27, 123–130. doi: 10.1016/j.pt.2010.11.002
Maure, F., Daoust, S. P., Brodeur, J., Mitta, G., and Thomas, F. (2013). Diversity and evolution of bodyguard manipulation. J. Exp. Biol. 216, 36–42. doi: 10.1242/jeb.073130
Médoc, V., Rigaud, T., Bollache, L., and Beisel, J. N. (2009). A manipulative parasite increasing an antipredator response decreases its vulnerability to a nonhost predator. Animal Behav. 77, 1235–1241. doi: 10.1016/j.anbehav.2009.01.029
Mille, T., Soulier, L., Caill-Milly, N., Cresson, P., Morandeau, G., and Monperrus, M. (2020). Differential micropollutants bioaccumulation in European hake and their parasites Anisakis sp. Environ. Poll. 2020:115021. doi: 10.1016/j.envpol.2020.115021
Molbert, N., Agostini, S., Alliot, F., Angelier, F., Biard, C., Decencière, B., et al. (2021). Parasitism reduces oxidative stress of fish host experimentally exposed to PAHs. Ecotoxicol. Environ. Saf. 219:112322. doi: 10.1016/j.ecoenv.2021.112322
Molbert, N., Alliot, F., Leroux-Coyau, M., Médoc, V., Biard, C., Meylan, S., et al. (2020). Potential benefits of acanthocephalan parasites for chub hosts in polluted environments. Environ. Sci. Technol. 54, 5540–5549. doi: 10.1021/acs.est.0c00177
Morley, N. J., Lewis, J. W., and Hoole, D. (2006). Pollutant-induced effects on immunological and physiological interactions in aquatic host–trematode systems: implications for parasite transmission. J. Helminthol. 80, 137–149. doi: 10.1079/joh2006345
Morrill, A., Provencher, J. F., and Forbes, M. R. (2015). Testing for dual impacts of contaminants and parasites on hosts: the importance of skew. Environ. Rev. 22, 445–456. doi: 10.1139/er-2014-0026
Morrill, A., Provencher, J. F., Gilchrist, H. G., Mallory, M. L., and Forbes, M. R. (2019). Anti-parasite treatment results in decreased estimated survival with increasing lead (Pb) levels in the common eider Somateria mollissima. Proc. R. Soc. B 286:20191356. doi: 10.1098/rspb.2019.1356
Munger, J. C., and Holmes, J. C. (1988). Benefits of parasitic infection: a test using a ground squirrel–trypanosome system. Canadian J. Zool. 66, 222–227. doi: 10.1186/s12936-018-2292-7
Nachev, M., Jochmann, M. A., Walter, F., Wolbert, J. B., Schulte, S. M., Schmidt, T. C., et al. (2017). Understanding trophic interactions in host-parasite associations using stable isotopes of carbon and nitrogen. Parasites Vectors 10:90. doi: 10.1186/s13071-017-2030-y
Oros, M., and Hanzelová, V. (2009). Re-establishment of the fish parasite fauna in the Tisa River system (Slovakia) after a catastrophic pollution event. Parasitol. Res. 104, 1497–1506. doi: 10.1007/s00436-009-1356-6
Persson, M. E., Larsson, P., and Stenroth, P. (2007). Biomagnification and polychlorinated biphenyl congener distribution in an aquatic predator-prey, host-parasite system. Environ. Toxicol. Chem. 26, 837–843. doi: 10.1897/06-305R.1
Pietrock, M., and Marcogliese, D. J. (2003). Free-living endohelminth stages: at the mercy of environmental conditions. Trends Parasitol. 19, 293–299. doi: 10.1016/s1471-4922(03)00117-x
Rigby, M. C., Hechinger, R. F., and Stevens, L. (2002). Why should parasite resistance be costly? Trends Parasitol. 18, 116–120. doi: 10.1016/s1471-4922(01)02203-6
Robinson, S. A., Forbes, M. R., and Hebert, C. E. (2010). Mercury in parasitic nematodes and trematodes and their double-crested cormorant hosts: bioaccumulation in the face of sequestration by nematodes. Sci. Total Environ. 408, 5439–5444. doi: 10.1016/j.scitotenv.2010.07.071
Rohr, J. R., Raffel, T. R., Sessions, S. K., and Hudson, P. J. (2008). Understanding the net effects of pesticides on amphibian trematode infections. Ecol. Appl. 18, 1743–1753. doi: 10.1890/07-1429.1
Rowe, C. L. (2008). “The calamity of so long life”: life histories, contaminants, and potential emerging threats to long-lived vertebrates. Bioscience 58, 623–631. doi: 10.1641/b580709
Sánchez, M. I., Pons, I., Martínez-Haro, M., Taggart, M. A., Lenormand, T., and Green, A. J. (2016). When parasites are good for health: cestode parasitism increases resistance to arsenic in brine shrimps. PLoS Pathogens 12:e1005459. doi: 10.1371/journal.ppat.1005459
Scheef, G., Sures, B., and Taraschewski, H. (2000). Cadmium accumulation in Moniliformis moniliformis (Acanthocephala) from experimentally infected rats. Parasitol. Res. 86, 688–691. doi: 10.1007/pl00008553
Schludermann, C., Konecny, R., Laimgruber, S., Lewis, J. W., Schiemer, F., Chovanec, A., et al. (2003). Fish macroparasites as indicators of heavy metal pollution in river sites in Austria. Parasitology 126, S61–S69. doi: 10.1017/s0031182003003743
Šebelová, Š, Kuperman, B., and Gelnar, M. (2002). Abnormalities of the attachment clamps of representatives of the family Diplozoidae. J. Helminthol. 76, 249–259. doi: 10.1079/JOH2002133
Sheldon, B. C., and Verhulst, S. (1996). Ecological immunology: costly parasite defences and trade-offs in evolutionary ecology. Trends Ecol. Evol. 11, 317–321. doi: 10.1016/0169-5347(96)10039-2
Sternberg, E. D., Lefèvre, T., Rawstern, A. H., and de Roode, J. C. (2011). A virulent parasite can provide protection against a lethal parasitoid. Infect. Genet. Evol. 11, 399–406. doi: 10.1016/j.meegid.2010.11.017
Sures, B. (2001). The use of fish parasites as bioindicators of heavy metals in aquatic ecosystems: a review. Aquatic Ecol. 35, 245–255. doi: 10.3390/ani10050811
Sures, B. (2006). How parasitism and pollution affect the physiological homeostasis of aquatic hosts. J. Helminthol. 80, 151–157. doi: 10.1079/joh2006346
Sures, B. (2008). Host–parasite interactions in polluted environments. J. Fish Biol. 73, 2133–2142. doi: 10.1111/j.1095-8649.2008.02057.x
Sures, B., and Siddall, R. (1999). Pomphorhynchus laevis: the intestinal acanthocephalan as a lead sink for its fish host, chub (Leuciscus cephalus). Exp. Parasitol. 93, 66–72. doi: 10.1006/expr.1999.4437
Sures, B., and Siddall, R. (2003). Pomphorhynchus laevis (Palaeacanthocephala) in the intestine of chub (Leuciscus cephalus) as an indicator of metal pollution. Int. J. Parasitol. 33, 65–70. doi: 10.1016/s0020-7519(02)00249-7
Sures, B., Dezfuli, B. S., and Krug, H. F. (2003). The intestinal parasite Pomphorhynchus laevis (Acanthocephala) interferes with the uptake and accumulation of lead (210Pb) in its fish host chub (Leuciscus cephalus). Int. J. Parasitol. 33, 1617–1622. doi: 10.1016/s0020-7519(03)00251-0
Sures, B., Nachev, M., Selbach, C., and Marcogliese, D. J. (2017). Parasite responses to pollution: what we know and where we go in ‘Environmental Parasitology’. Parasites Vectors 10:65. doi: 10.1186/s13071-017-2001-3
Sures, B., Taraschewski, H., and Jackwerth, E. (1994). Lead accumulation in Pomphorhynchus laevis and its host. J. Parasitol. 80, 355–357. doi: 10.2307/3283403
Szefer, P., Rokicki, G., and Frelek, K. (1998). Bioaccumulation of selected trace elements in lung nematodes,Pseudaliusin flexus,of harbor porpoise (phocoenaphocoena) in a polish zone of the Baltic Sea. Sci. Environ. 220, 19–24. doi: 10.1016/s0048-9697(98)00221-6
Thielen, F., Zimmermann, S., Baska, F., Taraschewski, H., and Sures, B. (2004). The intestinal parasite Pomphorhynchus laevis (Acanthocephala) from barbel as a bioindicator for metal pollution in the Danube River near Budapest. Hungary. Environ. Pollut. 129, 421–429. doi: 10.1016/j.envpol.2003.11.011
Thomas, F. P. J. F., Poulin, R., Guégan, J. F., Michalakis, Y., and Renaud, F. (2000). Are there pros as well as cons to being parasitized? Parasitol. Today 16, 533–536. doi: 10.1016/s0169-4758(00)01790-7
Valtonen, E. T., Holmes, J. C., and Koskivaara, M. (1997). Eutrophication, pollution and fragmentation: effects on parasite communities in roach (Rutilus rutilus) and perch (Perca fluviatilis) in four lakes in central Finland. Canadian J. Fisheries Aquatic Sci. 54, 572–585. doi: 10.1139/f96-306
Van Valen, L. (1973). A new evolutionary law. Evol. Theory 1, 1–30. doi: 10.1016/j.jhevol.2008.09.007
Vidal-Martínez, V. M., Aguirre-Macedo, M. L., Noreña-Barroso, E., Gold-Bouchot, G., and Caballero-Pinzoìn, P. I. (2003). Potential interactions between metazoan parasites of the Mayan catfish Ariopsis assimilis and chemical pollution in Chetumal Bay, Mexico. J. Helminthol. 77, 173–184. doi: 10.1079/JOH2002158
Vives, I., Grimalt, J. O., Ventura, M., Catalan, J., and Rosseland, B. O. (2005). Age dependence of the accumulation of organochlorine pollutants in brown trout (Salmo trutta) from a remote high mountain lake (Redo, Pyrenees). Environ. Pollut. 133, 343–350. doi: 10.1016/j.envpol.2004.05.027
Keywords: contaminant, biotic interactions, antagonistic interaction, stress, bioaccumulation
Citation: Goutte A and Molbert N (2022) Benefits of Parasitism in Polluted Environments: A Review and Perspectives. Front. Ecol. Evol. 10:847869. doi: 10.3389/fevo.2022.847869
Received: 03 January 2022; Accepted: 30 March 2022;
Published: 28 April 2022.
Edited by:
Lynne Beaty, Penn State Erie, The Behrend College, United StatesReviewed by:
Mark Forbes, Carleton University, CanadaCopyright © 2022 Goutte and Molbert. This is an open-access article distributed under the terms of the Creative Commons Attribution License (CC BY). The use, distribution or reproduction in other forums is permitted, provided the original author(s) and the copyright owner(s) are credited and that the original publication in this journal is cited, in accordance with accepted academic practice. No use, distribution or reproduction is permitted which does not comply with these terms.
*Correspondence: Aurelie Goutte, YXVyZWxpZS5nb3V0dGVAc29yYm9ubmUtdW5pdmVyc2l0ZS5mcg==