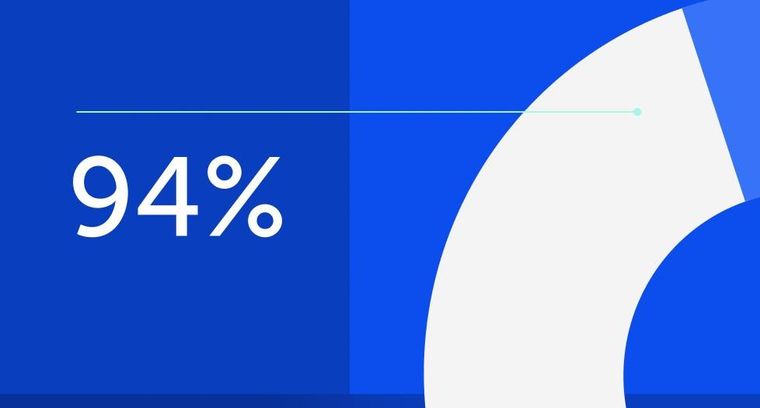
94% of researchers rate our articles as excellent or good
Learn more about the work of our research integrity team to safeguard the quality of each article we publish.
Find out more
REVIEW article
Front. Ecol. Evol., 19 May 2022
Sec. Evolutionary Ecology of Social Behaviour
Volume 10 - 2022 | https://doi.org/10.3389/fevo.2022.846586
This article is part of the Research TopicSocial Evolution and the What, When, Why and How of the Major Evolutionary Transitions in the History of LifeView all 11 articles
In endosymbiosis, two independently existing entities are inextricably intertwined such that they behave as a single unit. For multicellular hosts, the endosymbiont must be integrated within the host developmental genetic network to maintain the relationship. Developmental integration requires innovations in cell type, gene function, gene regulation, and metabolism. These innovations are contingent upon the existing ecological interactions and may evolve mutual interdependence. Recent studies have taken significant steps toward characterizing the proximate mechanisms underlying interdependence. However, the study of developmental integration is only in its early stages of investigation. Here, we review the literature on mutualistic endosymbiosis to explore how unicellular endosymbionts developmentally integrate into their multicellular hosts with emphasis on insects as a model. Exploration of this process will help gain a more complete understanding of endosymbiosis. This will pave the way for a better understanding of the endosymbiotic theory of evolution in the future.
Endosymbiosis is an association between different species where one lives inside the body of another, often involving a mutual benefit (Buchner, 1965). There are a multitude of forms of endosymbiotic relationships, in the case of both unicellular and multicellular organisms. The endosymbionts can be intracellular or extracellular, they can be obligate or facultative, they can be mutualistic, commensalistic, or parasitic (Gupta and Nair, 2020). Here we focus on the mutualistic endosymbioses, although some of the concepts are applicable to commensalism and parasitism, a discussion on those is beyond the scope of this review.
The processes involved in maintaining an endosymbiotic association must conform with the native molecular genetic processes of the host. In multicellular organisms, the genotype and environmental inputs are brought together by the process of development to give rise to a phenotype, which is subject to evolution (Abouheif et al., 2014; Gilbert et al., 2015). Development, in its broadest definition, is the process of progressive and continuous change that generates a complex multicellular organism from embryogenesis, maturation to senescence (Gilbert and Barresi, 2017). Therefore, understanding how a unicellular endosymbiont integrates with the biology of its multicellular host requires the study of development of the multicellular host in the context of endosymbiosis (Gilbert et al., 2015).
Whether the endosymbionts are maternally transmitted or acquired later in life, development guides interactions between the partners in ways that intertwine the endosymbiotic association with the developmental process (Figure 1). The infection of endosymbionts into the host is highly developmental stage-specific while the phase of the life cycle during which endosymbionts remain associated with the host is tightly regulated (Koga et al., 2012; Catta-Preta et al., 2015; Russel et al., 2017). The stage at which endosymbionts multiply within the host tissue is also under strict control and the population size and diversity of the endosymbionts are regulated by the developmental process (Wolschin et al., 2004). The movement and packaging of endosymbionts within the host body are characteristic of any given host-endosymbiont pair and specific cell types to harbor endosymbionts are uniquely present in particular hosts (Braendle et al., 2003; Stoll et al., 2010; Ratzka et al., 2012). Endosymbionts may influence and be affected by the process of metamorphosis, which also causes the removal of the gut microbiota between specific stages, while the cellular endosymbionts are retained (Hammer and Moran, 2019). Remarkably, loss of endosymbionts leads in some cases to developmental alterations that are lethal or costly in terms of reproductive success (Schwemmler, 1974; Rafiqi et al., 2020). The exact manner in which development coordinates between endosymbiont-host interactions are in the early stages of exploration at the experimental level and the theoretical framework for the involvement of development is lacking.
Figure 1. Illustration showing developmental integration in the process of maintenance of endosymbiotic association between a bacterial endosymbiont and an ant host as an example. Bacteria are shown in red and bacterial cell walls in black. Different developmental stages are shown representing the lifecycle of the ant that undergoes multiple steps of metamorphosis from egg to larva to pupa to adult. Red dotted arrows indicate transmission, which can be horizontal or vertical (maternal). Gene regulatory network is represented as colored circles connected with lines. Small arrows indicate influence, large arrows indicate metamorphic stage transitions.
In the sections that follow we first summarize the current understanding of the nature of inter-relations between organisms that engage with each other. We then discuss the genomic changes underlying these inter-relations. Taking into account the biological development of multicellular hosts, we explore the phenomenon of developmental integration of endosymbionts with their hosts. We suggest developmental integration as the driving process that coordinates genotype and inter-organismal relations to potentiate, originate, and maintain endosymbiosis, by means of which free-living bacteria become endosymbionts of multicellular hosts.
When free-living bacteria transition to being mutualistic endosymbionts, they start to influence the host in multiple aspects, which make either the endosymbiont or the host-dependent on the other in nutrition, metabolism, immunity, genetics, ecology, or a combination of these (Baumann, 2005; Moran et al., 2008; Flórez et al., 2015; Masson et al., 2016; Rafiqi et al., 2020). This dependence can be unidirectional or bidirectional, and potentially evolve from one to the other. Moreover, the changes leading to interdependence may occur prior to-, along with-, or after- the initial establishment of the endosymbiotic association. If an interdependence arises after the initial establishment of endosymbiosis, rewiring or interruption of non-essential pathways or processes in either of the organisms may occur by evolutionary mechanisms (Wernegreen and Moran, 1999; Wernegreen, 2002; Zientz et al., 2004; Baumann, 2005; McCutcheon and Moran, 2007, 2012). It can be speculated that under the scenario of interruptions or rewiring, notwithstanding the specific genes affected, conservation of resources such as substrates of molecular reactions and energy for the partners as a whole is likely to be favored by natural selection. However, it has been shown that in the absence of selective pressure, genes involved in these pathways or processes are often lost by genetic drift (Wu et al., 2006; McCutcheon and Moran, 2012; Sloan and Moran, 2012; Wernegreen, 2015). Conversely, a progressive co-adaptation leading to interdependence arises over evolutionary time with features in the partners being retained due to the advantages for both the endosymbionts and hosts (Wu et al., 2006; Douglas, 2014; Wernegreen, 2015). Given the changes discussed above, the degree of interdependence between the host and the endosymbiont appears to be constantly evolving throughout their association.
Nutritional, metabolic, immune-related, genetic, or ecological changes are accompanied by changes at the genomic level. Interdependence between host and endosymbiont ultimately comes from genetic input, which is further aided and potentiated in two ways (Wilson and Duncan, 2015). (i) Horizontal gene transfer between genomes of endosymbiont and host leads to the insertion of new genes in the host genome (Nakabachi, 2015). Surprisingly, horizontal gene transfer is more common from facultative endosymbionts than from obligate endosymbionts (Nikoh and Nakabachi, 2009; Husnik et al., 2013; Husnik and McCutcheon, 2018). The acquisition of new genes, by horizontal gene transfer, provides an important source of innovations facilitating further evolution of the relationship between endosymbiont and host (Keeling and Palmer, 2008; Sloan et al., 2014; Blondel et al., 2020). One way in which this can occur is by substitution of obligate endosymbiont genes by horizontally transferred genes from facultative endosymbionts provided the two endosymbionts have shared homology in genes and pathways. The gain of endosymbiont genes by hosts, from a facultative endosymbiont, would make the homologs of these genes redundant in their obligate endosymbionts and facilitate the loss of these genes in the obligate endosymbiont (Wilson and Duncan, 2015). (ii) The genomes of endosymbiotic bacteria are generally much smaller when compared to their free-living relatives implying that after the establishment of endosymbiosis, the endosymbiont genome undergoes size reduction (Douglas, 1998; Moran, 2002; Latorre et al., 2005; Toft and Andersson, 2010; McCutcheon and Moran, 2012; Russell et al., 2013). Genomic changes previously discussed as accompanying endosymbiosis in many cases further embed the process of interdependence between the endosymbiont and host. Yet, these genomic changes do not necessarily co-occur with the initial establishment of endosymbiotic association but may secondarily enhance the association.
The endosymbiont and host together behave as a single ecological unit, wherein being together increases the number of ways in which they can adapt to the environment or allows them to occupy specialized niches such as nutrient-poor diets (Buchner, 1965). The endosymbiont genome provides an additional source of heritable information that has the potential to impact the endosymbiotic association. As a whole, host-endosymbiont interdependence can be perceived as an intricate interplay of biological entities that impact all the levels of biological organization (Janson et al., 2008; Feldhaar, 2011). Interdependence between the endosymbiont and host—due to nutritional, metabolic, immune-related, genetic, or ecological changes—potentiated by horizontal gene transfer, and genome reduction—results in the maintenance of endosymbiosis that ultimately may lead to an irreversible dependence on each other. The origin of interdependencies is phylogenetically contingent in that it arose from ancestors with particular physiology, that lived under particular ecological conditions, and are shaped by evolutionary forces acting on interacting partners (Rafiqi et al., 2020). Even after the symbiont becomes established as a permanent resident over time, countless other adjustments in different biological levels are triggered in both partners to accommodate each other more efficiently (Moya et al., 2008; Toft and Andersson, 2010; Perreau and Moran, 2021). Recent research has pointed out the fact that both partners in endosymbiosis “converse” at the molecular level from the early stages of host development (Banfill et al., 2020; Rafiqi et al., 2020).
Understanding the mechanisms that govern the initial establishment of endosymbiotic interdependence will require the following two approaches: One, a thorough sampling of endosymbiosis-related phenotypes in a vast number of related organisms. Two, a detailed comparison of host molecular genetic processes between organisms that contain endosymbionts and their closest relatives that lack them. The latter approach could immensely benefit from vigorous research in the field of developmental biology in the recent decades to further understand the establishment as well as maintenance of endosymbiotic associations. This approach would involve studying host-centric mechanisms of endosymbiont transmission and bacteriocyte specification on the one hand and endosymbiont-centric mechanisms of integration into host development as well as the dynamic role of endosymbiont during host development on the other hand.
In an endosymbiotic relationship, the host acquires, maintains, and transmits their endosymbiont from generation to generation or acquires the endosymbiont in a stage-specific manner during development (Figure 1). It is during development that the genotype and environmental factors are integrated into the phenotype through multiple interacting mechanisms. Endosymbiosis must therefore have a dynamic interaction with the developmental process. Developing organisms are under the control of multiple essential processes including the migration, proliferation, specialization, and death of cells, for producing new cells with different characteristics at different locations in the organism. These cellular processes are achieved through differential gene expression, intra and inter-cellular transport of mRNAs and proteins, and cell-to-cell communications that lead to the organization of cells into multicellular arrangements such as tissues and organs (Gilbert and Barresi, 2017). Developmental events are controlled by gene regulatory networks that compose signaling cascades and pathways (Lewis, 1978; McGinnis et al., 1984; Lehmann and Nüsslein-Volhard, 1986; Schupbach and Wieschaus, 1986; Padgett et al., 1987; Ingham, 1988; Tautz, 1988; Akam, 1989; Mann and Hogness, 1990; Shimell et al., 1991; St Johnston and Nüsslein-Volhard, 1992; Arora et al., 1994; Mason et al., 1994; Biehs et al., 1996; Tomoyasu et al., 2005; Campos-Ortega and Hartenstein, 2013). In multicellular organisms, signaling pathways require an array of chemical substances such as hormones, growth factors, neurotransmitters, and extracellular matrix components, acting locally or traveling long distances within the organism (Gilbert and Barresi, 2017). This complex and well-orchestrated interplay of events inside the developing organisms often proceeds in the presence of endosymbionts. Additionally, endosymbionts that are vertically transmitted accompany the organisms from its single-cell stage to reproduction and death. Therefore, their continued presence must increase the likelihood of evolving interactions and influences on the developmental processes.
Several questions therefore arise from this intersection of endosymbiosis with development. How do the endosymbionts interact with the host to result in stable integration of one cell into another, given the tightly regulated developmental gene networks that define the cell identities, body axes, segments, and the germline? Conversely, how does the host regulate endosymbiotic populations without affecting their function during development? Additionally, evolutionary conflicts of interest between endosymbiotic partners complicate this process because organisms most often tend to evolve in such a way that promotes their own fitness at the expense of their partner’s fitness (Garcia and Gerardo, 2014; Bennett and Moran, 2015; Lowe et al., 2016; Keeling and McCutcheon, 2017). Therefore, the connection between the host and the endosymbiont is complicated and dynamic, including highly precise adaptations and counter-adaptations.
In the last three decades, there has been a lot of interest in the mechanisms of endosymbiosis (Moran and Baumann, 2000; Russell and Moran, 2006; Hansen and Moran, 2011; Login et al., 2011; Landmann et al., 2014; Weinert et al., 2015; Bennett et al., 2016; Kupper et al., 2016; Gray, 2017; Mergaert et al., 2017; Skidmore and Hansen, 2017). However, due to technological limitations, as noted by Wilson and Duncan (2015), most of the studies in the field have treated the interacting host and endosymbiont as distinct organisms. From this perspective, the two distinct genomes may appear to be thought of as interacting partners that merely communicate via signals. This view is incomplete because endosymbionts appear more embedded within the molecular genetic processes of the host. The endosymbionts directly influence the development of their hosts in numerous and innovative ways. For example, the endosymbiont Vibrio fischeri constitutes the essential light emitting cells of its host squid Euprymna scolopes where during its development bacterial cells are induced to become non-motile and trigger the host epithelial cells to swell for endosymbiont acquisition (Nyholm and McFall-Ngai, 2004). The bacteria release tracheal cytotoxin that acts as a morphogen influencing the development of the crypts in the epithelium that makes the light organ of its host squid E. scolopes (Koropatnick et al., 2004). The bacteria also release a small non-coding RNA that not only influences morphological changes in the host epithelium but also modulates the expression of host immune response genes such that when a mutant bacterium that lacks the gene for this small non-coding RNA is provided to the squid, it fails to cause the proper formation of the light organ (Moriano-Gutierrez et al., 2020).
In the aphids, Buchnera varies throughout clonal aphid lineages being influenced by environmental and host genetic variables. Gene expression in the bacteriocytes differs between clonal populations of aphids while Buchnera gene expression adjusts accordingly to the genotype of the host (Smith and Moran, 2020). Comparing hosts with low and high Buchnera titer shows that aphids and Buchnera oppositely regulate genes underlying cell growth and amino acid biosynthesis (Chong and Moran, 2016). Bacteriocytes and endosymbionts show a high level of expression of genes underlying energy metabolism in the case of high-titer aphids (Chong and Moran, 2016). Also, several cell signaling pathways of high-titer hosts such as cytokine pathways, lysosomal processes, membrane trafficking, and mechanistic target of rapamycin (mTOR) are up-regulated (Smith and Moran, 2020). Additionally, genes related to flagellar body secretion are overexpressed in low-titer hosts and those of flagellar assembly are overexpressed in high-titer hosts (Chong and Moran, 2016). Altering the diet of the aphid host also elicits changes in the expression of small non-coding RNAs of the endosymbiont target genes related to pathways involved in essential amino acid biosynthesis (Thairu et al., 2019).
In Drosophila, Wolbachia can influence the expression of germline genes (Fast et al., 2011; Ote et al., 2016). Wolbachia is found in germline tissues as well as detected in somatic tissues (Dobson et al., 1999; Cheng et al., 2000; Clark et al., 2002; Ijichi et al., 2002). Maternally transmitted Wolbachia persist throughout embryogenesis and are incorporated into the pole cells, which make the gonads (Kose and Karr, 1995). It has been shown that Wolbachia exhibits striking subcellular localization using microtubules and Dynein at the anterior pole during oogenesis (Ferree et al., 2005). This is the mechanism by which they transmit to the next generation exploiting the host’s microtubule cytoskeleton and transport system (Ferree et al., 2005). In most cases, only a smaller sub-population of endosymbionts is developmentally destined to be vertically transmitted, creating a bottleneck effect for the evolving endosymbiont population. For example in the Camponotus floridanus, the majority of endosymbionts are steered by the developmental process to become housed in the midgut epithelium for producing nutritional benefits to the host, while as an order of magnitude smaller populations reside in tissues closely proximal to the gonads (Rafiqi et al., 2020).
The role of endosymbionts in the development of the host is highlighted by studies where experimental elimination of endosymbionts leads to significant alteration of host development. For example, in the Euscelis bugs, the elimination of endosymbionts leads to truncation of the abdomen indicating that the endosymbiont influences posterior pattern formation in this organism (Schwemmler, 1974). In the filarial nematode Brugia malayi, loss of Wolbachia alters the anterior-posterior pattern formation hinting at an interaction with the segmentation cascade that defines this axis (Landmann et al., 2014). In the cereal weevil, Sitophilus oryzae, the endosymbiont Sodalis pierantonius affects transcripts involved in cell apoptosis, autophagy, and gut epithelial cell swelling and delamination (Masson et al., 2015). In the Cnidarian host Hydra, the genome of Curvibacter endosymbionts produces signaling molecules N-acyl homoserine lactones that are subsequently modified by host-encoded enzymes, resulting in dramatic shifts in endosymbiont gene expression and phenotype (Pietschke et al., 2017). In the C. floridanus ants, the elimination of Blochmannia leads to loss of germline and Hox gene expression domains in the embryo that in turn causes changes in the position of the functional germline formation and influences gonad formation leading to complete loss of gonad in a proportion of the embryos (Rafiqi et al., 2020). In some cases, there is an influence on growth, development, or reproduction but the pathways through which this is achieved remain elusive (Koga et al., 2003; Hosokawa et al., 2008; Kuriwada et al., 2010; Xue et al., 2012; Hickin et al., 2022). Endosymbionts and hosts therefore affect gene regulation of each other and exhibit diverse adaptations toward survival and transmission. But how the host gene regulatory networks adapt immediately after the initial encounter with endosymbionts has scarcely been explored. A couple of studies have so far uncovered possible key players in the process, and it appears that members of highly conserved gene families are involved in the developmental integration of endosymbionts.
Hox genes usually define segment identity along the anterior-posterior axis (Lewis, 1978; McGinnis et al., 1984; Lehmann and Nüsslein-Volhard, 1986; Schupbach and Wieschaus, 1986; Ingham, 1988; Tautz, 1988; Akam, 1989; Mann and Hogness, 1990; St Johnston and Nüsslein-Volhard, 1992; Tomoyasu et al., 2005). However, Hox genes of the Bithorax complex have been shown to play a role in patterning the bacteriocytes in a hemipteran bug Nysius plebeius, and the ant C. floridanus (Matsuura et al., 2015; Rafiqi et al., 2020). In the case of N. plebeius, a loss of function of the Hox gene Abdominal A (AbdA) leads to mis-regulation of bacteriocyte formation and that of the Hox gene Ultrabithorax (Ubx) leads to a complete loss of bacteriocytes (Matsuura et al., 2015). In C. floridanus, not only are the homologs of these Hox genes involved in bacteriocyte differentiation but also at the same time the expression of these Hox genes is dependent on the endosymbiont Blochmannia such that their expression patterns alter after removal of endosymbiont through antibiotic treatment (Rafiqi et al., 2020). Therefore, there appears to be a regulatory loop between Hox gene regulation and signals from the endosymbionts, raising the possibility that patterning components interact with the endosymbiont in these ants and perhaps in other organisms that carry and maintain such endosymbionts. Moreover, phylogenetic analysis has shown that in the Camponotus ants and their closest relatives within the ant tribe Camponotini, early development has been drastically changed following the acquisition of a bacterial endosymbiont in multiple ways (Rafiqi et al., 2020). Most strikingly, these ants form two germlines during embryogenesis: one germline through the accumulation of maternal mRNAs and a secondary germline through endosymbiont driven zygotic induction mechanisms via the activity of Hox genes (Rafiqi et al., 2020). In these ants, endosymbionts regulate the localization of mRNAs of genes that define the germline such as oskar, vasa, nanos, tudor, aubergine, and staufen via Hox genes Ubx and abdA such that these germline genes become localized in more than one subcellular locations in contrast to all other known insects where they localize to a single location of the embryo (Rafiqi et al., 2020). These results indicate that the endosymbiont brings about significant changes that affect the development of the host.
Intriguingly, the ant endosymbiont Blochmannia is closely related to endosymbionts present in hemipteran mealybugs (Wernegreen et al., 2009), and homologs of the Hox genes abdA and Ubx pattern bacteriocytes in both of these diverse lineages (Matsuura et al., 2015; Rafiqi et al., 2020). Is it possible that because the two hosts have very similar gene regulatory networks, an endosymbiont may benefit from host interactions acquired in an ancestral relationship? A kind of interaction that allows the endosymbiont to recognize a highly conserved gene regulatory pathway in novel hosts would provide an advantage for integration into the host. This suggests that Hox genes may have played a role in the horizontal transfer of Blochmannia from mealybugs to Camponotus. Other highly conserved genetic pathways in diverse organisms may influence the horizontal transfer of endosymbionts. Consequently, the prospect of endosymbionts transferring to more distantly related hosts, would increase or decrease based on the properties acquired within a primary host. We speculate that highly conserved gene networks such as that of Hox genes may therefore have contributed to the establishment of endosymbiosis among a large number of multicellular organisms.
Evolutionary novelties are unique phenotypes with novel functions found in a specific taxon (Pigliucci, 2008). Bacteriocytes are an example of evolutionary novelty that appear to have evolved multiple times independently in different taxa and differentiate during development to accommodate the endosymbiont (Moya et al., 2008). Bacteriocytes and their resident endosymbionts may also aggregate to form an organ-like structure called the “bacteriome” (Buchner, 1965). Organs or tissues housing intracellular endosymbionts are not only restricted to insects but also found in their closest relatives: trophosome of deep-sea tubeworms (Annelidae) or the gill filaments of lucinid bivalves (Mollusca) (Cavanaugh et al., 1981; Frenkiel and Mouëza, 1995). These organs develop either from mesodermal tissue or from undifferentiated cells in the lateral zone of the gill filaments (Gros et al., 1997; Bright and Sorgo, 2003). From an evolutionary and developmental point of view, these data provide insights into the cell type or germ layer the bacteriocytes are derived from in those early lineages. However, in insects even with one of the best-researched models—the aphid and its associated endosymbiont Buchnera—the mechanisms, cell types, or germ layer that the bacteriocytes arise from remain unknown (Braendle et al., 2003; Simonet et al., 2018; Banfill et al., 2020; Davis et al., 2021). At the genetic level, it is suspected that the transcription factors Ubx, and abdA are participating in the formation of bacteriocytes in the aphid—Buchnera endosymbiosis system (Braendle et al., 2003). A closer examination of the hemipteran insect N. plebeius shows that Ubx and abdA genes normally involved in defining abdominal segments, seem to have been additionally co-opted to induce novel cells to form the bacteriocytes (Matsuura et al., 2015). Interestingly, in a different lineage, the Camponotus and closely related ants, the endosymbiont Blochmannia, homologs of the same genes appear to be involved in bacteriocyte development (Rafiqi et al., 2020). The bacterial endosymbiont Blochmannia is found in the ovaries of adult ants and the posterior pole of mature oocytes. When the oocytes transition to freshly laid eggs, Blochmannia is found at the posterior pole (Blochmann, 1882; Buchner, 1965; Sauer et al., 2000; Kupper et al., 2016; Ramalho et al., 2018). When the syncytial embryos form cellular boundaries, the bacteria get enveloped in bacteriocytes, which migrate to the middle of the yolk and become part of the midgut epithelium (Rafiqi et al., 2020). In some species of genus Camponotus an intriguing novel cell type called the “germline capsule,” evolved to house a seed population of Blochmannia. This sub-population migrates to the germ cells and is transmitted to the next generation through the gonads (Rafiqi et al., 2020). The transition to obligate endosymbiosis therefore involves multiple changes in the host organisms that involve genetic and anatomical novelties that enhance the efficiency of the endosymbiotic association. Further exploration of the genetic signatures of these novel cells or structures in comparison to other neighboring or ontogenetically similar structures in these organisms will shed light on whether these evolved from pre-existing structures and genes or arose independently.
This review is an attempt to draw the framework of the study of developmental integration in endosymbiosis taking insects as a model. Because of their widespread occurrence, the integration of endosymbionts into a novel association is crucial for comprehending the evolution of life on Earth. The mechanism of establishment of endosymbiosis and its continuation through generations is in the early stages of exploration. Even though endosymbiotic associations have been known for a long time, it is important to point out that many of them were not understood at the molecular level. Moreover, there has been exceeding emphasis on the proximal interdependence between the endosymbiont and the host without regard to the developmental perspective. Recently, one of the biggest surprises in the studies of endosymbiosis has been that development not only affects the establishment, maintenance, and transmission of the endosymbiont but the endosymbiont also affects the developmental pattern formation of the host. Here, we propose that the developmental process plays an active role in coordinating and establishing endosymbiotic associations, incorporating inputs from the genome, and ecological interactions to efficiently engage the endosymbiont within the host. Subsequently, the organisms undergo changes that make them irreversibly interdependent. An interactive scheme encompassing and understanding the intersection of development with nutritional, metabolic, immune-related, genetic, or ecological aspects of these associations will reveal a broader and potentially more accurate picture. Future research on the key role of development integration in endosymbiosis will shed light upon the way endosymbiosis is established and maintained. More individual examples from the living world would be required to substantiate these ideas about the effect of development on endosymbiosis.
AMR conceived the idea and wrote the manuscript. PGP, NSM, ZÖD, BÇ-A, MEA, FZÇ, and AR wrote the manuscript. All authors contributed to the article and approved the submitted version.
This work was supported by TÜBİTAK Grant No. 120C157 awarded to AMR and Bezmialem Vakif University Project No. BAP 9.2019/5 awarded to PGP.
The authors declare that the research was conducted in the absence of any commercial or financial relationships that could be construed as a potential conflict of interest.
All claims expressed in this article are solely those of the authors and do not necessarily represent those of their affiliated organizations, or those of the publisher, the editors and the reviewers. Any product that may be evaluated in this article, or claim that may be made by its manufacturer, is not guaranteed or endorsed by the publisher.
The authors would like to thank the reviewers for their valuable comments.
Abouheif, E., Fave, M. J., Ibarraran-Viniegra, A. S., Lesoway, M. P., Rafiqi, A. M., and Rajakumar, R. (2014). Eco-evo-devo: the time has come. Adv. Exp. Med. Biol. 781, 107–125. doi: 10.1007/978-94-007-7347-9_6
Akam, M. (1989). Hox and HOM: homologous gene clusters in insects and vertebrates. Cell 57, 347–349. doi: 10.1016/0092-8674(89)90909-4
Arora, K., Levine, M. S., and O’Connor, M. B. (1994). The screw gene encodes a ubiquitously expressed member of the TGF-beta family required for specification of dorsal cell fates in the Drosophila embryo. Genes Dev. 8, 2588–2601. doi: 10.1101/gad.8.21.2588
Banfill, C. R., Wilson, A. C., and Lu, H.-L. (2020). Further evidence that mechanisms of host/symbiont integration are dissimilar in the maternal versus embryonic Acyrthosiphon pisum bacteriome. EvoDevo 11:23. doi: 10.1186/s13227-020-00168-5
Baumann, P. (2005). Biology of bacteriocyte-associated endosymbionts of plant sap-sucking insects. Annu. Rev. Microbiol. 59, 155–189. doi: 10.1146/annurev.micro.59.030804.121041
Bennett, G. M., McCutcheon, J. P., McDonald, B. R., and Moran, N. A. (2016). Lineage-Specific Patterns of Genome Deterioration in Obligate Symbionts of Sharpshooter Leafhoppers. Genome Biol. Evol. 8, 296–301. doi: 10.1093/gbe/evv159
Bennett, G. M., and Moran, N. A. (2015). Heritable symbiosis: the advantages and perils of an evolutionary rabbit hole. Proc. Natl. Acad. Sci. U. S. A. 112, 10169–10176. doi: 10.1073/pnas.1421388112
Biehs, B., Francois, V., and Bier, E. (1996). The Drosophila short gastrulation gene prevents Dpp from autoactivating and suppressing neurogenesis in the neuroectoderm. Genes Dev. 10, 2922–2934. doi: 10.1101/gad.10.22.2922
Blochmann, F. (1882). Über das Vorkommen bakterienähnlicher Gebilde in den Geweben und Eiern verschiedener Insekten. Zbl. Bakt. 11, 234–240.
Blondel, L., Jones, T. E., and Extavour, C. G. (2020). Bacterial contribution to genesis of the novel germ line determinant oskar. eLife 9:e45539. doi: 10.7554/eLife.45539
Braendle, C., Miura, T., Bickel, R., Shingleton, A. W., Kambhampati, S., and Stern, D. L. (2003). Developmental origin and evolution of bacteriocytes in the aphid-Buchnera symbiosis. PLoS Biol. 1:E21. doi: 10.1371/journal.pbio.0000021
Bright, M., and Sorgo, A. (2003). Ultrastructural reinvestigation of the trophosome in adults of Riftia pachyptila (Annelida. Siboglinidae). Invertebr. Biol. 122, 347–368.
Buchner, P. (1965). Endosymbiosis of animals with plant microorganisms. New York, NY: Interscience Publishers.
Campos-Ortega, J. A., and Hartenstein, V. (2013). The embryonic development of Drosophila melanogaster. Heidelberg: Springer Science & Business Media.
Catta-Preta, C. M. C., Brum, F. L., da Silva, C. C., Zuma, A. A., Elias, M. C., de Souza, W., et al. (2015). Endosymbiosis in trypanosomatid protozoa: the bacterium division is controlled during the host cell cycle. Front. Microbiol. 6:520. doi: 10.3389/fmicb.2015.00520
Cavanaugh, C. M., Gardiner, S. L., Jones, M. L., Jannasch, H. W., and Waterbury, J. B. (1981). Prokaryotic Cells in the Hydrothermal Vent Tube Worm Riftia pachyptila Jones: possible Chemoautotrophic Symbionts. Science 213, 340–342. doi: 10.1126/science.213.4505.340
Cheng, Q., Ruel, T., Zhou, W., Moloo, S., Majiwa, P., O’neill, S. L., et al. (2000). Tissue distribution and prevalence of Wolbachia infections in tsetse flies, Glossina spp. Med. Vet. Entomol. 14, 44–50. doi: 10.1046/j.1365-2915.2000.00202.x
Chong, R. A., and Moran, N. A. (2016). Intraspecific genetic variation in hosts affects regulation of obligate heritable symbionts. Proc. Natl. Acad. Sci. U. S. A. 113, 13114–13119. doi: 10.1073/pnas.1610749113
Clark, M. E., Veneti, Z., Bourtzis, K., and Karr, T. L. (2002). The distribution and proliferation of the intracellular bacteria Wolbachia during spermatogenesis in Drosophila. Mech. Dev. 111, 3–15. doi: 10.1016/s0925-4773(01)00594-9
Davis, G. K., Brisson, J. A., and Bickel, R. D. (2021). “Evo-Devo Lessons Learned from Aphids,” in Evolutionary Developmental Biology, eds L. Nuno de la Rosa and G. Müller (Cham: Springer), 817–829. doi: 10.1007/978-3-319-32979-6_182
Dobson, S. L., Bourtzis, K., Braig, H. R., Jones, B. F., Zhou, W., Rousset, F., et al. (1999). Wolbachia infections are distributed throughout insect somatic and germ line tissues. Insect Biochem. Mol. Biol. 29, 153–160. doi: 10.1016/s0965-1748(98)00119-2
Douglas, A. E. (1998). Nutritional interactions in insect-microbial symbioses: aphids and their symbiotic bacteria Buchnera. Annu. Rev. Entomol. 43, 17–37. doi: 10.1146/annurev.ento.43.1.17
Douglas, A. E. (2014). Symbiosis as a general principle in eukaryotic evolution. Cold Spring Harb. Perspect. Biol. 6:a016113. doi: 10.1101/cshperspect.a016113
Fast, E. M., Toomey, M. E., Panaram, K., Desjardins, D., Kolaczyk, E. D., and Frydman, H. M. (2011). Wolbachia Enhance Drosophila Stem Cell Proliferation and Target the Germline Stem Cell Niche. Science 334:990. doi: 10.1126/science.1209609
Feldhaar, H. (2011). Bacterial symbionts as mediators of ecologically important traits of insect hosts. Ecol. Entomol. 36, 533–543. doi: 10.1111/j.1365-2311.2011.01318.x
Ferree, P. M., Frydman, H. M., Li, J. M., Cao, J., Wieschaus, E., and Sullivan, W. (2005). Wolbachia utilizes host microtubules and Dynein for anterior localization in the Drosophila oocyte. PLoS Pathog. 1:e14. doi: 10.1371/journal.ppat.0010014
Flórez, L. V., Biedermann, P. H., Engl, T., and Kaltenpoth, M. (2015). Defensive symbioses of animals with prokaryotic and eukaryotic microorganisms. Nat. Prod. Rep. 32, 904–936. doi: 10.1039/c5np00010f
Frenkiel, L., and Mouëza, M. (1995). Gill ultrastructure and symbiotic bacteria in Codakia orbicularis (Bivalvia. Lucinidae). Zoomorphology 115, 51–61. doi: 10.1007/bf00397934
Garcia, J. R., and Gerardo, N. M. (2014). The symbiont side of symbiosis: do microbes really benefit? Front. Microbiol. 5:510. doi: 10.3389/fmicb.2014.00510
Gilbert, S. F., Bosch, T. C., and Ledon-Rettig, C. (2015). Eco-Evo-Devo: developmental symbiosis and developmental plasticity as evolutionary agents. Nat. Rev. Genet. 16, 611–622. doi: 10.1038/nrg3982
Gray, M. W. (2017). Lynn Margulis and the endosymbiont hypothesis: 50 years later. Mol. Biol. Cell 28, 1285–1287. doi: 10.1091/mbc.E16-07-0509
Gros, O., Frenkiel, L., and Moueza, M. (1997). Embryonic, larval, and post-larval development in the symbiotic clam Codakia orbicularis (Bivalvia: Lucinidae). Invertebr. Biol. 116, 86–101. doi: 10.2307/3226973
Gupta, A., and Nair, S. (2020). Dynamics of Insect–Microbiome Interaction Influence Host and Microbial Symbiont. Front. Microbiol. 11:1357. doi: 10.3389/fmicb.2020.01357
Hammer, T. J., and Moran, N. A. (2019). Links between metamorphosis and symbiosis in holometabolous insects. Philos. Trans. R. Soc. B 374:20190068. doi: 10.1098/rstb.2019.0068
Hansen, A. K., and Moran, N. A. (2011). Aphid genome expression reveals host-symbiont cooperation in the production of amino acids. Proc. Natl. Acad. Sci. U. S. A. 108, 2849–2854. doi: 10.1073/pnas.1013465108
Hickin, M. L., Kakumanu, M. L., and Schal, C. (2022). Effects of Wolbachia elimination and B-vitamin supplementation on bed bug development and reproduction. Res. Square [Preprint] doi: 10.21203/rs.3.rs-1274245/v1
Hosokawa, T., Kikuchi, Y., Shimada, M., and Fukatsu, T. (2008). Symbiont acquisition alters behaviour of stinkbug nymphs. Biol. Lett. 4, 45–48. doi: 10.1098/rsbl.2007.0510
Husnik, F., and McCutcheon, J. P. (2018). Functional horizontal gene transfer from bacteria to eukaryotes. Nat. Rev. Microbiol. 16, 67–79. doi: 10.1038/nrmicro.2017.137
Husnik, F., Nikoh, N., Koga, R., Ross, L., Duncan Rebecca, P., Fujie, M., et al. (2013). Horizontal Gene Transfer from Diverse Bacteria to an Insect Genome Enables a Tripartite Nested Mealybug Symbiosis. Cell 153, 1567–1578. doi: 10.1016/j.cell.2013.05.040
Ijichi, N., Kondo, N., Matsumoto, R., Shimada, M., Ishikawa, H., and Fukatsu, T. (2002). Internal spatiotemporal population dynamics of infection with three Wolbachia strains in the adzuki bean beetle, Callosobruchus chinensis (Coleoptera: Bruchidae). Appl. Environ. Microbiol. 68, 4074–4080. doi: 10.1128/AEM.68.8.4074-4080.2002
Ingham, P. W. (1988). The molecular genetics of embryonic pattern formation in Drosophila. Nature 335, 25–34. doi: 10.1038/335025a0
Janson, E. M., Stireman, J. O. III, Singer, M. S., and Abbot, P. (2008). Phytophagous insect–microbe mutualisms and adaptive evolutionary diversification. Evol. Int. J. Org. Evol. 62, 997–1012. doi: 10.1111/j.1558-5646.2008.00348.x
Keeling, P. J., and McCutcheon, J. P. (2017). Endosymbiosis: the feeling is not mutual. J. Theor. Biol. 434, 75–79. doi: 10.1016/j.jtbi.2017.06.008
Keeling, P. J., and Palmer, J. D. (2008). Horizontal gene transfer in eukaryotic evolution. Nat. Rev. Genet. 9, 605–618. doi: 10.1038/nrg2386
Koga, R., Meng, X.-Y., Tsuchida, T., and Fukatsu, T. (2012). Cellular mechanism for selective vertical transmission of an obligate insect symbiont at the bacteriocyte–embryo interface. Proc. Natl. Acad. Sci. U. S. A. 109, E1230–E1237. doi: 10.1073/pnas.1119212109
Koga, R., Tsuchida, T., and Fukatsu, T. (2003). Changing partners in an obligate symbiosis: a facultative endosymbiont can compensate for loss of the essential endosymbiont Buchnera in an aphid. Proc. Biol. Sci. 270, 2543–2550. doi: 10.1098/rspb.2003.2537
Koropatnick, T. A., Engle, J. T., Apicella, M. A., Stabb, E. V., Goldman, W. E., and McFall-Ngai, M. J. (2004). Microbial factor-mediated development in a host-bacterial mutualism. Science 306, 1186–1188. doi: 10.1126/science.1102218
Kose, H., and Karr, T. L. (1995). Organization of Wolbachia pipientis in the Drosophila fertilized egg and embryo revealed by an anti-Wolbachia monoclonal antibody. Mech. Dev. 51, 275–288. doi: 10.1016/0925-4773(95)00372-x
Kupper, M., Stigloher, C., Feldhaar, H., and Gross, R. (2016). Distribution of the obligate endosymbiont Blochmannia floridanus and expression analysis of putative immune genes in ovaries of the carpenter ant Camponotus floridanus. Arthropod Struct. Dev. 45, 475–487. doi: 10.1016/j.asd.2016.09.004
Kuriwada, T., Hosokawa, T., Kumano, N., Shiromoto, K., Haraguchi, D., and Fukatsu, T. (2010). Biological Role of Nardonella Endosymbiont in Its Weevil Host. PLoS One 5:e13101. doi: 10.1371/journal.pone.0013101
Landmann, F., Foster, J. M., Michalski, M. L., Slatko, B. E., and Sullivan, W. (2014). Co-evolution between an endosymbiont and its nematode host: Wolbachia asymmetric posterior localization and AP polarity establishment. PLoS Negl. Trop. Dis. 8:e3096. doi: 10.1371/journal.pntd.0003096
Latorre, A., Gil, R., Silva, F., and Moya, A. (2005). Chromosomal stasis versus plasmid plasticity in aphid endosymbiont Buchnera aphidicola. Heredity 95, 339–347. doi: 10.1038/sj.hdy.6800716
Lehmann, R., and Nüsslein-Volhard, C. (1986). Abdominal Segmentation, Pole Cell Formation, and Embryonic Polarity Require the Localized Activity of oskar, a Maternal Gene in Drosophila. Cell 47, 141–152. doi: 10.1016/0092-8674(86)90375-2
Lewis, E. B. (1978). A gene complex controlling segmentation in Drosophila. Nature 276, 565–570. doi: 10.1038/276565a0
Login, F. H., Balmand, S., Vallier, A., Vincent-Monegat, C., Vigneron, A., Weiss-Gayet, M., et al. (2011). Antimicrobial peptides keep insect endosymbionts under control. Science 334, 362–365. doi: 10.1126/science.1209728
Lowe, C. D., Minter, E. J., Cameron, D. D., and Brockhurst, M. A. (2016). Shining a light on exploitative host control in a photosynthetic endosymbiosis. Curr. Biol. 26, 207–211. doi: 10.1016/j.cub.2015.11.052
Mann, R. S., and Hogness, D. S. (1990). Functional dissection of Ultrabithorax proteins in D. melanogaster. Cell 60, 597–610. doi: 10.1016/0092-8674(90)90663-y
Mason, E. D., Konrad, K. D., Webb, C. D., and Marsh, J. L. (1994). Dorsal midline fate in Drosophila embryos requires twisted gastrulation, a gene encoding a secreted protein related to human connective tissue growth factor. Genes Dev. 8, 1489–1501. doi: 10.1101/gad.8.13.1489
Masson, F., Moné, Y., Vigneron, A., Vallier, A., Parisot, N., Vincent-Monégat, C., et al. (2015). Weevil endosymbiont dynamics is associated with a clamping of immunity. BMC Genomics 16:819. doi: 10.1186/s12864-015-2048-5
Masson, F., Zaidman-Rémy, A., and Heddi, A. (2016). Antimicrobial peptides and cell processes tracking endosymbiont dynamics. Philos. Trans. R. Soc. B Biol. Sci. 371:20150298. doi: 10.1098/rstb.2015.0298
Matsuura, Y., Kikuchi, Y., Miura, T., and Fukatsu, T. (2015). Ultrabithorax is essential for bacteriocyte development. Proc. Natl. Acad. Sci. U. S. A. 112, 9376–9381. doi: 10.1073/pnas.1503371112
McCutcheon, J. P., and Moran, N. A. (2007). Parallel genomic evolution and metabolic interdependence in an ancient symbiosis. Proc. Nat. Acad. Sci. U. S. A. 104, 19392–19397. doi: 10.1073/pnas.0708855104
McCutcheon, J. P., and Moran, N. A. (2012). Extreme genome reduction in symbiotic bacteria. Nat. Rev. Microbiol. 10, 13–26. doi: 10.1038/nrmicro2670
McGinnis, W., Garber, R. L., Wirz, J., Kuroiwa, A., and Gehring, W. J. (1984). A homologous protein-coding sequence in Drosophila homeotic genes and its conservation in other metazoans. Cell 37, 403–408. doi: 10.1016/0092-8674(84)90370-2
Mergaert, P., Kikuchi, Y., Shigenobu, S., and Nowack, E. C. (2017). Metabolic Integration of Bacterial Endosymbionts through Antimicrobial Peptides. Trends Microbiol. 25, 703–712. doi: 10.1016/j.tim.2017.04.007
Moran, N. A. (2002). Microbial Minimalism: genome Reduction in Bacterial Pathogens. Cell 108, 583–586. doi: 10.1016/S0092-8674(02)00665-7
Moran, N. A., and Baumann, P. (2000). Bacterial endosymbionts in animals. Curr. Opin. Microbiol. 3, 270–275. doi: 10.1016/S1369-5274(00)00088-6
Moran, N. A., McCutcheon, J. P., and Nakabachi, A. (2008). Genomics and evolution of heritable bacterial symbionts. Annu. Rev. Genet. 42, 165–190. doi: 10.1146/annurev.genet.41.110306.130119
Moriano-Gutierrez, S., Bongrand, C., Essock-Burns, T., Wu, L., McFall-Ngai, M. J., and Ruby, E. G. (2020). The noncoding small RNA SsrA is released by Vibrio fischeri and modulates critical host responses. PLoS Biol. 18:e3000934. doi: 10.1371/journal.pbio.3000934
Moya, A., Peretó, J., Gil, R., and Latorre, A. (2008). Learning how to live together: genomic insights into prokaryote–animal symbioses. Nat. Rev. Genet. 9, 218–229. doi: 10.1038/nrg2319
Nakabachi, A. (2015). Horizontal gene transfers in insects. Curr. Opin. Insect Sci. 7, 24–29. doi: 10.1016/j.cois.2015.03.006
Nikoh, N., and Nakabachi, A. (2009). Aphids acquired symbiotic genes via lateral gene transfer. BMC Biol. 7:12. doi: 10.1186/1741-7007-7-12
Nyholm, S. V., and McFall-Ngai, M. J. (2004). The winnowing: establishing the squid-vibrio symbiosis. Nat. Rev. Microbiol. 2, 632–642. doi: 10.1038/nrmicro957
Ote, M., Ueyama, M., and Yamamoto, D. (2016). Wolbachia Protein TomO Targets nanos mRNA and Restores Germ Stem Cells in Drosophila Sex-lethal Mutants. Curr. Biol. 26, 2223–2232. doi: 10.1016/j.cub.2016.06.054
Padgett, R. W., Johnston, R. D. S., and Gelbart, W. M. (1987). A transcript from a Drosophila pattern gene predicts a protein homologous to the transforming growth factor-β family. Nature 325, 81–84. doi: 10.1038/325081a0
Perreau, J., and Moran, N. A. (2021). Genetic innovations in animal–microbe symbioses. Nat. Rev. Genet. 23, 23–39. doi: 10.1038/s41576-021-00395-z
Pietschke, C., Treitz, C., Forêt, S., Schultze, A., Künzel, S., Tholey, A., et al. (2017). Host modification of a bacterial quorum-sensing signal induces a phenotypic switch in bacterial symbionts. Proc. Natl. Acad. Sci. U. S. A. 114, E8488–E8497. doi: 10.1073/pnas.1706879114
Pigliucci, M. (2008). What, if Anything. Is an Evolutionary Novelty? Philos. Sci. 75, 887–898. doi: 10.1086/594532
Rafiqi, A. M., Rajakumar, A., and Abouheif, E. (2020). Origin and elaboration of a major evolutionary transition in individuality. Nature 585, 239–244. doi: 10.1038/s41586-020-2653-6
Ramalho, M. O., Vieira, A. S., Pereira, M. C., Moreau, C. S., and Bueno, O. C. (2018). Transovarian Transmission of Blochmannia and Wolbachia Endosymbionts in the Neotropical Weaver Ant Camponotus textor (Hymenoptera. Formicidae). Curr. Microbiol. 75, 866–873. doi: 10.1007/s00284-018-1459-3
Ratzka, C., Gross, R., and Feldhaar, H. (2012). Endosymbiont Tolerance and Control within Insect Hosts. Insects 3, 553–572. doi: 10.3390/insects3020553
Russel, J., Røder, H. L., Madsen, J. S., Burmølle, M., and Sørensen, S. J. (2017). Antagonism correlates with metabolic similarity in diverse bacteria. Proc. Natl. Acad. Sci. U. S. A. 114, 10684–10688. doi: 10.1073/pnas.1706016114
Russell, C. W., Bouvaine, S., Newell, P. D., and Douglas, A. E. (2013). Shared metabolic pathways in a coevolved insect-bacterial symbiosis. Appl. Environ. Microbiol. 79, 6117–6123. doi: 10.1128/AEM.01543-13
Russell, J. A., and Moran, N. A. (2006). Costs and benefits of symbiont infection in aphids: variation among symbionts and across temperatures. Proc. R. Soc. B Biol. Sci. 273, 603–610. doi: 10.1098/rspb.2005.3348
Sauer, C., Stackebrandt, E., Gadau, J., Holldobler, B., and Gross, R. (2000). Systematic relationships and cospeciation of bacterial endosymbionts and their carpenter ant host species: proposal of the new taxon Candidatus Blochmannia gen. nov. Int. J. Syst. Evol. Microbiol. 50, 1877–1886. doi: 10.1099/00207713-50-5-1877
Schupbach, T., and Wieschaus, E. (1986). Germline autonomy of maternal-effect mutations altering the embryonic body pattern of Drosophila. Dev. Biol. 113, 443–448. doi: 10.1016/0012-1606(86)90179-x
Schwemmler, W. (1974). Endosymbionts - Factors of Egg Pattern Formation. J. Insect Physiol. 20, 1467–1474. doi: 10.1016/0022-1910(74)90078-X
Shimell, M. J., Ferguson, E. L., Childs, S. R., and O’Connor, M. B. (1991). The Drosophila dorsal-ventral patterning gene tolloid is related to human bone morphogenetic protein 1. Cell 67, 469–481. doi: 10.1016/0092-8674(91)90522-z
Simonet, P., Gaget, K., Balmand, S., Lopes, M. R., Parisot, N., Buhler, K., et al. (2018). Bacteriocyte cell death in the pea aphid/Buchnera symbiotic system. Proc. Natl. Acad. Sci. U. S. A. 115, E1819–E1828.
Skidmore, I. H., and Hansen, A. K. (2017). The evolutionary development of plant-feeding insects and their nutritional endosymbionts. Insect Sci. 24, 910–928. doi: 10.1111/1744-7917.12463
Sloan, D. B., and Moran, N. A. (2012). Genome Reduction and Co-evolution between the Primary and Secondary Bacterial Symbionts of Psyllids. Mol. Biol. Evol. 29, 3781–3792. doi: 10.1093/molbev/mss180
Sloan, D. B., Nakabachi, A., Richards, S., Qu, J., Murali, S. C., Gibbs, R. A., et al. (2014). Parallel histories of horizontal gene transfer facilitated extreme reduction of endosymbiont genomes in sap-feeding insects. Mol. Biol. Evol. 31, 857–871. doi: 10.1093/molbev/msu004
Smith, T. E., and Moran, N. A. (2020). Coordination of host and symbiont gene expression reveals a metabolic tug-of-war between aphids and Buchnera. Proc. Natl. Acad. Sci. U. S. A. 117, 2113–2121. doi: 10.1073/pnas.1916748117
St Johnston, D., and Nüsslein-Volhard, C. (1992). The origin of pattern and polarity in the Drosophila embryo. Cell 68, 201–219. doi: 10.1016/0092-8674(92)90466-p
Stoll, S., Feldhaar, H., Fraunholz, M. J., and Gross, R. (2010). Bacteriocyte dynamics during development of a holometabolous insect, the carpenter ant Camponotus floridanus. BMC Microbiol. 10:308. doi: 10.1186/1471-2180-10-308
Tautz, D. (1988). Regulation of the Drosophila segmentation gene hunchback by two maternal morphogenetic centres. Nature 332, 281–284. doi: 10.1038/332281a0
Thairu, M. W., Hansen, A. K., Hendry, T. A., and McFall-Ngai, M. J. (2019). Changes in Aphid Host Plant Diet Influence the Small-RNA Expression Profiles of Its Obligate Nutritional Symbiont, Buchnera. mBio 10, e01733–19. doi: 10.1128/mBio.01733-19
Toft, C., and Andersson, S. G. (2010). Evolutionary microbial genomics: insights into bacterial host adaptation. Nat. Rev. Genet. 11, 465–475. doi: 10.1038/nrg2798
Tomoyasu, Y., Wheeler, S. R., and Denell, R. E. (2005). Ultrabithorax is required for membranous wing identity in the beetle Tribolium castaneum. Nature 433:643. doi: 10.1038/nature03272
Weinert, L. A., Araujo-Jnr, E. V., Ahmed, M. Z., and Welch, J. J. (2015). The incidence of bacterial endosymbionts in terrestrial arthropods. Proc. R. Soc. B Biol. Sci. 282:20150249. doi: 10.1098/rspb.2015.0249
Wernegreen, J., and Moran, N. (1999). Evidence for genetic drift in endosymbionts (Buchnera): analyses of protein-coding genes. Mol. Biol. Evol. 16, 83–97. doi: 10.1093/oxfordjournals.molbev.a026040
Wernegreen, J. J. (2002). Genome evolution in bacterial endosymbionts of insects. Nat. Rev. Genet. 3, 850–861. doi: 10.1038/nrg931
Wernegreen, J. J. (2015). Endosymbiont evolution: predictions from theory and surprises from genomes. Ann. N. Y. Acad. Sci. 1360, 16–35. doi: 10.1111/nyas.12740
Wernegreen, J. J., Kauppinen, S. N., Brady, S. G., and Ward, P. S. (2009). One nutritional symbiosis begat another: phylogenetic evidence that the ant tribe Camponotini acquired Blochmannia by tending sap-feeding insects. BMC Evol. Biol. 9:292. doi: 10.1186/1471-2148-9-292
Wilson, A. C. C., and Duncan, R. P. (2015). Signatures of host/symbiont genome coevolution in insect nutritional endosymbioses. Proc. Natl. Acad. Sci. U. S. A. 112, 10255–10261. doi: 10.1073/pnas.1423305112
Wolschin, F., Hölldobler, B., Gross, R., and Zientz, E. (2004). Replication of the endosymbiotic bacterium Blochmannia floridanus is correlated with the developmental and reproductive stages of its ant host. Appl. Environ. Microbiol. 70, 4096–4102. doi: 10.1128/AEM.70.7.4096-4102.2004
Wu, D., Daugherty, S. C., Van Aken, S. E., Pai, G. H., Watkins, K. L., Khouri, H., et al. (2006). Metabolic Complementarity and Genomics of the Dual Bacterial Symbiosis of Sharpshooters. PLoS Biol. 4:e188. doi: 10.1371/journal.pbio.0040188
Xue, X., Li, S.-J., Ahmed, M. Z., De Barro, P. J., Ren, S.-X., and Qiu, B.-L. (2012). Inactivation of Wolbachia reveals its biological roles in whitefly host. PLoS One 7:e48148. doi: 10.1371/journal.pone.0048148
Keywords: bacteriocytes, developmental integration, Hox genes, interdependence, ecology, evolutionary novelty, endosymbiosis
Citation: Rafiqi AM, Polo PG, Milat NS, Durmuş ZÖ, Çolak-Al B, Alarcón ME, Çağıl FZ and Rajakumar A (2022) Developmental Integration of Endosymbionts in Insects. Front. Ecol. Evol. 10:846586. doi: 10.3389/fevo.2022.846586
Received: 31 December 2021; Accepted: 25 April 2022;
Published: 19 May 2022.
Edited by:
Karen Marie Kapheim, Utah State University, United StatesReviewed by:
Sandie M. Degnan, The University of Queensland, AustraliaCopyright © 2022 Rafiqi, Polo, Milat, Durmuş, Çolak-Al, Alarcón, Çağıl and Rajakumar. This is an open-access article distributed under the terms of the Creative Commons Attribution License (CC BY). The use, distribution or reproduction in other forums is permitted, provided the original author(s) and the copyright owner(s) are credited and that the original publication in this journal is cited, in accordance with accepted academic practice. No use, distribution or reproduction is permitted which does not comply with these terms.
*Correspondence: Ab. Matteen Rafiqi, bS5yYWZpcWlAYmV6bWlhbGVtLmVkdS50cg==
Disclaimer: All claims expressed in this article are solely those of the authors and do not necessarily represent those of their affiliated organizations, or those of the publisher, the editors and the reviewers. Any product that may be evaluated in this article or claim that may be made by its manufacturer is not guaranteed or endorsed by the publisher.
Research integrity at Frontiers
Learn more about the work of our research integrity team to safeguard the quality of each article we publish.