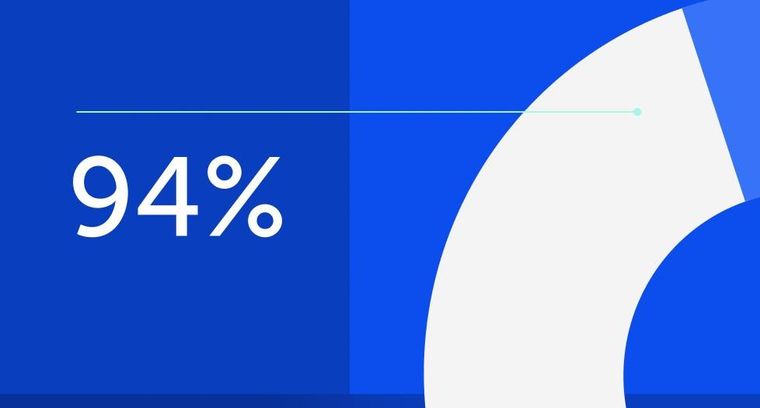
94% of researchers rate our articles as excellent or good
Learn more about the work of our research integrity team to safeguard the quality of each article we publish.
Find out more
ORIGINAL RESEARCH article
Front. Ecol. Evol., 19 May 2022
Sec. Behavioral and Evolutionary Ecology
Volume 10 - 2022 | https://doi.org/10.3389/fevo.2022.842264
This article is part of the Research TopicAvian Behavioral and Physiological Responses to Challenging Thermal Environments and Extreme Weather EventsView all 12 articles
Rapid anthropogenic climate change potentially severely reduces avian breeding success. While the consequences of high temperatures and drought are reasonably well-studied within single breeding seasons, their impacts over decadal time scales are less clear. We assessed the effects of air temperature (Tair) and drought on the breeding output of southern yellow-billed hornbills (Tockus leucomelas; hornbills) in the Kalahari Desert over a decade (2008–2019). We aimed to document trends in breeding performance in an arid-zone bird during a time of rapid global warming and identify potential drivers of variation in breeding performance. The breeding output of our study population collapsed during the monitoring period. Comparing the first three seasons (2008–2011) of monitoring to the last three seasons (2016–2019), the mean percentage of nest boxes that were occupied declined from 52% to 12%, nest success from 58% to 17%, and mean fledglings produced per breeding attempt from 1.1 to 0.4. Breeding output was negatively correlated with increasing days on which Tmax (mean maximum daily Tair) exceeded the threshold Tair at which male hornbills show a 50% likelihood of engaging in heat dissipation behavior [i.e., panting (Tthresh; Tair = 34.5°C)] and the occurrence of drought within the breeding season, as well as later dates for entry into the nest cavity (i.e., nest initiation) and fewer days post-hatch, spent incarcerated in the nest by the female parent. The apparent effects of high Tair were present even in non-drought years; of the 115 breeding attempts that were recorded, all 18 attempts that had ≥ 72% days during the attempt on which Tmax > Tthresh failed (equivalent to Tmax during the attempt ≥ 35.7°C). This suggests that global warming was likely the primary driver of the recent, rapid breeding success collapse. Based on current warming trends, the Tmax threshold of 35.7°C, above which no successful breeding attempts were recorded, will be exceeded during the entire hornbill breeding season by approximately 2027 at our study site. Therefore, our findings support the prediction that climate change may drive rapid declines and cause local extinctions despite the absence of direct lethal effects of extreme heat events.
Global heating is exacerbating the harsh conditions associated with arid environments by elevating average air temperatures (Tair) and increasing the frequency and intensity of heatwaves (Pachauri et al., 2014; Mbokodo et al., 2020) and drought (van Wilgen et al., 2016). Possible consequences for animals inhabiting arid regions include increased frequency of mass mortality events (Welbergen et al., 2008; Albright et al., 2017; McKechnie and Wolf, 2019; McKechnie et al., 2021) and disastrous reproductive failures (Bolger et al., 2005; Albright et al., 2010; Seabrook et al., 2014; McCowan and Griffith, 2021; Sharpe et al., 2021). However, heatwaves, especially in association with droughts, may also have insidious sublethal effects (Gardner et al., 2016; Conradie et al., 2019; Bourne et al., 2020b,2021; Cunningham et al., 2021; Moagi et al., 2021), including loss of body condition (du Plessis et al., 2012; Sharpe et al., 2019), reduced egg or clutch sizes in birds (Albright et al., 2010; Keynan and Yosef, 2010), reduced provisioning rates and compromised offspring quality (van de Ven et al., 2020a; Bourne et al., 2021; Oswald et al., 2021), and the foregoing of breeding altogether (Moreno and Møller, 2011; McCreedy and van Riper, 2015; Carstens et al., 2019).
The small size and high mass-specific metabolism of most birds, combined with a diurnal activity period and limited use of underground refugia, make this taxon among the most vulnerable to mismatches between energy and water balance over short time scales (Wolf, 2000; Albright et al., 2017; Conradie et al., 2019; Cooper et al., 2020; Kemp et al., 2020). Arid-zone birds are particularly vulnerable given that they are commonly constrained to breed in response to rainfall, which often occurs during the hottest time of the year (Bolger et al., 2005; Flesch, 2014; McCreedy and van Riper, 2015; Mares et al., 2017). Given that birds face the dual threats of dehydration and hyperthermia daily during this period, elucidating the limits of their capacity to cope with the increased resource (food, water, and time) demands associated with breeding is critical for understanding variation in reproductive success under current and future climates (Andreasson et al., 2020; Cunningham et al., 2021). There is a growing body of literature on the impacts of high temperatures and drought on nest success of arid-zone birds at the scale of nesting attempts and seasons (e.g., Catry et al., 2011, 2015; Salaberria et al., 2014; Cruz-McDonnell and Wolf, 2016; Bourne et al., 2020a,b; D’Amelio et al., 2021), but fewer studies investigate these impacts on population-level breeding outputs over longer timescales in relation to ongoing climate change trends (however, see e.g., Frederiksen et al., 2013; Hatch, 2013; Amélineau et al., 2019; Bourne et al., 2020a; Ridley et al., 2021).
The southern yellow-billed hornbill (Tockus leucomelas; hereafter hornbill) initiates breeding in response to rainfall in the arid western parts of its southern African range (Kemp and Woodcock, 1995; van de Ven et al., 2020a). Like most hornbills, these hornbills employ an unusual breeding strategy whereby the female seals herself into the nest cavity (Kemp and Woodcock, 1995). Nestling hornbills within the sealed nest cavity are therefore largely safe from predators, meaning that breeding success is primarily dependent on other factors (Moreau and Moreau, 1941). In our study population, which has been monitored since 2008, the mean maximum daily Tair (Tmax) during the nesting period negatively correlates with fledging probability and fledgling condition (van de Ven et al., 2020a). The reduction in breeding success during hot periods is likely caused by a combination of high nest temperatures (Tnest) and reduced provisioning rates by males, due to trade-offs between thermoregulation and parental effort (van de Ven et al., 2019, 2020a). Considering (a) the mechanistic links between weather conditions and breeding are well-studied in hornbills (van de Ven et al., 2019, 2020a; Van Jaarsveld et al., 2021), (b) a priori, the unusual breeding system of hornbills and the fact they are constrained to breed during the hottest time of year in an arid zone indicates this taxon could be especially vulnerable to global warming, and (c) a 10-year monitoring dataset exists for our study population, which resides near the hot, arid south-western edge of the species’ distribution, we suggest that this population of hornbills provides an excellent opportunity to test the prediction that lethal and sublethal fitness costs of high Tmax will lead to increasingly poor reproductive output over time, risking breeding collapse and population extirpation due to climate change (Conradie et al., 2019).
In this study, we examined climate trends (1960–2020) for the study region and the breeding success of hornbills at both broad (long-term trends 2008–2018) and fine (individual breeding attempts) scales. Our specific aims were to (1) quantify long-term climate trends in the study region, (2) quantify changes in the breeding success of this population of hornbills over the last decade, and (3) model the relationships between several extrinsic (metrics of rainfall and temperature) and intrinsic (female entry into the nest cavity date and days post-hatch the female spent incarcerated in the nest) variables on the breeding success of hornbills. Our overall aim was to describe the response of an arid-zone bird to a decade-long period of rapid warming, identify potential drivers of variability in breeding performance, and empirically demonstrate likely mechanistic links between climate change and collapse of breeding output.
The southern yellow-billed hornbill is listed as the least concern by the International Union for Conservation of Nature (IUCN) but are thought to be declining (BirdLife International, 2021). The hornbill’s distribution includes most of southern Africa, with a large portion falling within the Kalahari Desert. They are socially monogamous and live up to 23 years in captivity (Strehlow, 2001), although the oldest known marked bird at our study site was 8 years old. Hornbills are cavity nesters; once sealed into the nest, the females moult all their flight feathers simultaneously. At our study site, pairs usually attempt a single brood per season (though they may be multibrooded in more mesic areas, Stanback et al., 2021), and the mean duration from the first egg lay to the female completing sufficient feather regrowth and breaking out of the nest is 53 ± 6.3 SD days (n = 56, range: 36–73) in nests that successfully produce at least one fledgling (van de Ven et al., 2020a). After the female breaks out of the sealed cavity, she aids in the subsequent provisioning of nestlings.
Research on hornbills has been ongoing at our study site (Kuruman River Reserve in the southern Kalahari Desert, Northern Cape province, South Africa; 26°58′ S, 21°49′ E; hereafter, KRR) since 2008, and breeding data for ten breeding seasons (2008–2019, excluding the summer of 2011–2012 when no data were collected) were used for this study. Hornbills at KRR typically breed between October and the end of March (van de Ven et al., 2020a). Monitoring allowed us to estimate the number of pairs at the site and track if and where they bred. During the decade monitoring period, some pairs were recorded breeding in natural cavities. No breeding data were recorded from these attempts. Breeding primarily occurred in nest boxes, and the breeding data analyzed in this study were exclusively from nest boxes. Wooden nest boxes were available at KRR throughout the study period (43 boxes during 2008–2011, 44 boxes during 2012–2015, 38 boxes during 2015–2017, 33 boxes during 2017–2018, and 36 boxes during 2018–2019). In seasons with low nest box occupancy, pairs were observed to still be present at the site but to skip breeding. We defined the hornbill breeding season as 19 October (the earliest recorded date a female entered a box during the decade of the study) to 24 March (the latest recorded fledge/failure date).
The KRR is an arid savanna dominated by grasses interspersed with large trees (mainly camelthorn, Vachellia erioloba; gray camelthorn, Vachellia haematoxylon; shepherds’ tree, Boscia albitrunca; and buffalo thorn, Ziziphus mucronata). Rainfall occurs primarily in the Austral spring and summer between October and April [mean annual rainfall: 213.9 ± SD 102.0 mm; 1993–2020, Van Zylsrus (VZ) weather station, South African Weather Service (SAWS)]. The spring and summer are hot; Tmax [mean maximum daily air temperature (Tair)] between October and March is 34.9 ± SD 1.19°C (1991–2020, VZ), which is above the threshold Tair at which male hornbills showed a 50% likelihood of engaging in heat dissipation behavior, i.e., panting [Tthresh; Tair = 34.5°C](van de Ven et al., 2019).
In 2011, the study site was equipped with a weather station [Hot Birds Research Project (HBRP) Weather Station; Vantage Pro2, Davis Instruments, Hayward, CA, United States] that was set to record Tair (°C), wind speed (m.s–1), relative humidity (%), and solar radiation (W.m–2) at 5-min intervals. To create a continuous weather dataset appropriate to the study site spanning the entire hornbill monitoring period (2008–2019), we compared weather data from the HBRP station collected between 2011 and 2020 to those from SAWS stations at VZ, Northern Cape (∼30 km from the study site, data available 1992–2020), and Twee Rivieren (TR), Northern Cape (∼120 km from the study site, data available 1960–2020) to validate the use of VZ and TR weather data as proxies for the missing HBRP weather data prior to 2011.
Weather data from all three stations were highly correlated (see Supplementary Figures 1, 2), but the Tmax data from VZ and TR were consistently higher than those from the HBRP (VZ mean: 1.38°C ± 1.54 SD higher; TR, mean: 2.13°C ± 1.79 SD higher; Supplementary Figure 1). Therefore, these values were adjusted (refer to Supplementary Figure 2) before being used to supplement the HBRP weather data collected at the study site. Daily rainfall was not correlated between the three weather stations, but seasonal rainfall was observed as follows: HBRP∼VZ r2 = 0.97; HBRP∼TR r2 = 0.86 (Supplementary Figure 3), allowing us to establish the occurrence/absence of drought at the study site using VZ rainfall data. The mean breeding season rainfall recorded by the VZ weather station was 151.71 mm (average rainfall recorded during the hornbill breeding season was 19 October–24 March each year from 1993 to 2019). We defined “drought” seasons as those in which rainfall recorded at VZ was < 80% of the long-term seasonal average following Bourne et al. (2020a). Rainfall has a lagged effect on prey abundance at the study site (Doolan and Macdonald, 1997), therefore the cumulative rainfall within the 2 months prior to the start of the breeding season was included in analyses as a proxy for food availability during breeding (Ridley and Raihani, 2007). To analyze long-term weather trends in southern Kalahari, we used unadjusted weather data from all three weather stations.
Statistical analyses were conducted in the R statistical environment, version 3.5.1 (R Core Team, 2021), using the R Studio platform (R Studio Team, 2021). For each weather station (HBRP, TR, and VZ), we used generalized linear models (GLMs) to model yearly trends in the Tmax (TR: 1960–2020; VZ: 1990–2020; and HBRP: 2011–2020), cumulative days on which Tmax > Tthresh (DaysTthresh; TR: 1960–2020; VZ: 1990–2020; and HBRP: 2011–2020), and cumulative rainfall (TR: 2007–2020; VZ: 1995–2020; and HBRP: 2011–2020), for each hornbill breeding season (19 October–24 March annually). A visual inspection of the Tair data from TR indicated a non-linear relationship. Therefore, we used segmented analysis (package segmented, Muggeo, 2017) on the dataset with the longest record (TR, starting 1960) to investigate whether there was a statistically significant inflection in seasonal Tmax and cumulative DaysTthresh each season. We also modeled the yearly trends in the non-breeding season (25 March–18 October each year) mean minimum daily Tair (Tmin; TR: 1960–2020; VZ: 1990–2020; and HBRP: 2011–2020) and cumulative rainfall (TR: 2007–2020; VZ: 1995–2020; HBRP 2011–2020). Only breeding and non-breeding seasons with data covering > 90% of the days were used in analyses (for excluded seasons see Supplementary Table 1). All trends were plotted with the linear model predictions (package ggplot2, Wickham, 2016).
We tested for temporal autocorrelation in all response variables across seasons by assessing the degree of correlation between the model residuals associated with increasing lags within each model. As no temporal autocorrelation between seasons was found for any variables, and sample size in terms of number of seasons was small (n = 10), we used simple Pearson’s product-moment correlation coefficient tests to assess the statistical significance of the following long-term trends: (1) seasonal Tmax and cumulative rainfall for breeding seasons 2008–2019; (2) percentage of nest boxes used, percentage of nesting attempts that succeeded in fledging at least one chick (hereafter succeeded), and mean number of fledglings produced per breeding attempt per season 2008–2019; and (3) relationships between the following weather variables: breeding season (18 August–18 October annually) cumulative DaysTthresh (this metric used since panting is associated with heavy reductions in foraging success (van de Ven et al., 2019), making DaysTthresh a biologically instructive metric for analyses); breeding season drought occurrence; non-breeding season Tmin; and the cumulative rainfall within the 2 months prior to the start of the breeding season, and (a) percentage of boxes used per season (b) percentage of nesting attempts that succeeded, and (c) the mean number of fledglings produced per attempt per season. Simple linear trends were then visualized using ggplot2 (Wickham, 2016). We also performed Welch two-sample t-tests on each of these dependent variables as a function of drought occurrence.
A total of 115 breeding attempts were recorded in nest boxes over the study period, of which 91 succeeded. Complete or partial weather data were available for 109 breeding attempts, and female entry date was known for 105. Due to these data gaps, the final sample sizes for analyses of nest survival in relation to weather conditions were n = 88 for (a) female entry to fledge/fail (hereafter “entire attempt”) and (b) female entry to hatch/fail (hereafter “pre-hatch period”). Attempts that had the required combination of known hatch date (n = 91) and complete weather data allowed for a final sample size of n = 88 for analyses of (c) hatch to nestling fledge/fail (hereafter “nestling period”).
Bayesian generalized linear mixed models (BGLMer) with binomial distribution were used to model the success/failure of (a) each entire attempt, (b) the pre-hatch period, and (c) the nestling period, as a function of the female entry date (coded as the count of days since the start of the breeding season, with 19 October = day 0); the percentage DaysTthresh during the attempt; drought occurrence; and the interaction between the percentage DaysTthresh and drought occurrence. The number of days the female spent incarcerated in the nest after hatching was included as an additional explanatory variable for nestling period analyses. We used Bayesian linear mixed models (BLMer) to model the effect of drought occurrence on female entry date and on the number of days post-hatch spent in the nest by the female, with ANOVA (Type II Wald chi-square test) used to test for significant correlations with drought occurrence. Season identity was included in all models as a random effect.
We used variance inflation factors (VIF; package car, Fox and Weisberg, 2019) to test for collinearity between explanatory variables and did not fit correlated variables within the same model. We used percentage DaysTthresh of each nesting period as our “temperature” predictor variable rather than Tmax of each nesting period as our “temperature” predictor variable because these two variables showed notable collinearity in all models (VIF > 10), and models including only percentage DaysTthresh outperformed models including only Tmax according to Akaike information criterion (AICc) adjusted for small sizes. We used “drought occurrence” (number of breeding attempts in drought years = 28, mean rainfall = 75.0 ± 42.8 mm, number of breeding attempts in non-drought years = 86, mean rainfall = 257.0 ± 29.1 mm) as our “precipitation” predictor variable instead of cumulative rainfall during the attempt because daily rainfall data were available for only 36 of 115 individual breeding attempts.
For each analysis, candidate model sets included all possible nested models within a biologically sensible global model, including the null model. We used a Bayesian modeling approach since it allowed us to fit a global model with the a priori terms of interest while avoiding singularity errors (package blme, Chung et al., 2013). Candidate models were compared using AICc (package MuMIn, Barton, 2020). We reported on the top-performing model, and where more than one top model was identified (those with an Δ AICc < 2), results were reported for the model average of the top models (package MuMIn, Barton, 2020). The plots were based on the single model with the lowest AICc (package jtools, Long, 2020).
All our breeding success data were necessarily collected from birds breeding in nest boxes (as opposed to natural tree cavities, which were sealed and inaccessible). However, between 2012 and 2015, we collected nest temperature (Tnest) data from occupied natural cavities to compare thermal conditions in these two nest types. We analyzed Tnest data from 27 occupied nest boxes and 9 occupied natural cavities (cumulatively 58,632 h and 9,816 h recording at 5-min intervals, respectively) collected between 2012 and 2015 using Thermochron iButtons (DS1923, Maxim, Sunnyvale, CA, United States, resolution = 0.0625°C). For nest box measurements, iButtons were mounted within the nests on the underside of the nest box lids in plastic iButton wall holders to minimize bird contact and to avoid interference by the birds. For natural cavity measurements, iButtons were placed in an iButton reader holster, which was secured with threading wire at the top of the cavity via the nest opening. We used Bayesian linear mixed models (BLMers) to model Tnest as a function of Tair as measured by the HBRP weather station, nest type (box vs. cavity), and the interaction between those factors. Individual nest identity was included as a random factor. ANOVA (Type II Wald chi-square test) was used to identify factors with significant correlations with Tnest.
In the TR weather data, there was a significant inflection in the summer of 1996–1997, after which Tmax (t54 = 4.58, p < 0.001, ± 3.5 SE years) and the cumulative DaysTthresh (t54 = 3.9, p < 0.001, ± 4.1 SE years) began to increase (Figures 1A,B). After summer 1996–1997, TR mean seasonal Tmax increased by 1.3°C per decade and cumulative DaysTthresh by 19.7 days per decade. We did not test for an inflection point in the VZ Tmax data; rather, considering the strong correlation between TR and VZ Tmax data (rho.c = 0.94, r2 = 0.89; Supplementary Figure 1), we applied the same inflection point identified for the TR data to the VZ data. Above the same inflection point, the VZ mean seasonal Tmax increased 1.0°C per decade and the cumulative days on which Tmax > Tthresh by 19.8 days per decade. Too few years of data were available for the HBRP station to model a trend in Tmax; however, given the strong correlation between the VZ and HBRP data (rho.c = 0.94, r2 = 0.94, Supplementary Figure 1), a similar warming trend of 1.0°C per decade can be expected for HBRP. No clear long-term pattern in rainfall was identified for either station; however, rainfall records only date back to 1993 (Figures 1C,D). No trends or inflection points were apparent in Tmin or cumulative rainfall during the non-breeding season since 1960 and 1995 for TR, respectively (Supplementary Figure 4).
Figure 1. (A) Trends in seasonal Tmax [mean maximum daily air temperature (Tair)], (B) cumulative DaysTthresh [days on which Tmax > Tthresh (Tair = 34.5°C)], and (C) cumulative rainfall for each southern yellow-billed hornbill (Tockus leucomelas) breeding season (19 October–24 March each year) modeled from Hot Birds Research Project (HBRP; the on-site weather station, green crosses), Twee Rivieren (red circles, ∼120 km from the study site), and Van Zylsrus (blue triangles, ∼30 km from the study site) weather station data. A significant increase in Tmax (± 3.5 SE years) and cumulative DaysTthresh (± 4.1 SE years) starting in approximately 1996–1997 was identified using segmented analysis for the TR weather station. (D) Bar plot for cumulative rainfall from the VZ weather station (data from TR between 2009 and 2012 included where VZ rainfall data were unavailable) with a dotted line showing the threshold for drought (gray bars) vs. non-drought (clear bars) years.
The percentage of nest boxes used (r = −0.80, p = 0.005) and the percentage of nesting attempts that succeeded (r = −0.73, p = 0.017) per season declined over the course of the monitoring period (Table 1 and Figure 2). There was a marginally non-significant decline in the mean number of fledglings produced per breeding pair per season (r = −0.63, p = 0.051, Table 1). We found no evidence of temporal autocorrelation in the number or percentage of nest boxes used, the percentage of nesting attempts that succeeded, or the mean fledglings per attempt, modeled by season.
Table 1. Pearson’s correlation test results for the percentage of boxes used, percentage of attempts fledging at least one chick, and mean fledglings per attempt per season in the southern yellow-billed hornbills (Tockus leucomelas), correlated with the cumulative DaysTthresh {days on which Tmax [mean maximum daily air temperature (Tair)] > Tthresh (Tair = 34.5°C)} within the breeding season (19 October–24 March), Tmin (mean minimum daily Tair), and cumulative rainfall within the 2 months prior to the start of the breeding season (18 August–18 October each year).
Figure 2. (A) The percentage of available nest boxes occupied and (B) the percentage of breeding attempts that succeeded per season in our study population of the southern yellow-billed hornbills (Tockus leucomelas) between 2008 and 2019. Both trends are significant (p < 0.05); 95% confidence intervals are shown.
The percentage of boxes used (r = −0.88, p < 0.001), percentage of successful attempts (r = −0.90, p < 0.001), and mean fledglings per attempt (r = −0.78, p = 0.008) all declined significantly with increasing cumulative DaysTthresh and were all significantly higher in non-drought seasons compared to drought seasons (p = 0.014, p ≤ 0.001, and p = 0.005, respectively; Table 1 and Figure 3). However, neither percentage of boxes used (r = −0.43, p = 0.220; r = 0.27, p = 0.451), the percentage of successful attempts (r = −0.59, p = 0.075; r = 0.15, p = 0.670), nor the mean fledglings produced per attempt (r = −0.56, p = 0.092; r = 0.22, p = 0.537) were significantly related to the cumulative 2 months’ rainfall preceding the start of the breeding season or the Tmin of the preceding non-breeding season, respectively (Table 1).
Figure 3. (A,B) The percentage of boxes used, (C,D) the mean fledglings per attempt, and (E,F) the percentage of attempts that succeeded in the southern yellow-billed hornbills (Tockus leucomelas) as functions of the cumulative DaysTthresh {days on which Tmax [mean maximum daily air temperature (Tair)] > Tthresh (Tair = 34.5°C)}, and occurrence of drought across the breeding season. All trends are significant (p < 0.05), and 95% confidence intervals and means with upper and lower quartiles are shown.
One best-performing model was identified for variation in breeding success (model weight: 0.96, Supplementary Table 2). This model indicated that the probability of success was negatively correlated with later female entry dates (estimate: −1.10 ± 0.41; p = 0.007; Table 2 and Figure 4). Additionally, there was a significant interaction between the percentage DaysTthresh during the attempt and the drought occurrence on breeding success (estimate: −2.65 ± 1.04; p = 0.011, Table 2). Post hoc models of breeding success in drought and non-drought years revealed a significant negative correlation between the percentage DaysTthresh during the attempt and breeding success in non-drought seasons (estimate: −1.87 ± 0.65; p = 0.004), but not in drought seasons (estimate: −0.20 ± 0.67; p = 0.771, Table 2 and Figure 4). In drought seasons, breeding success was uniformly low; only four of 23 (17.4%) attempts succeeded in fledging at least one chick, compared to 40 of 65 (61.5%) in non-drought seasons. Of the 115 breeding attempts, we recorded all 18 attempts that experienced ≥ 72% of the days on which Tmax > Tthresh (corresponding to Tmax during the attempt ≥ 35.7°C) failed (Figure 4). Based on current warming trends of 1°C per decade (Figure 1), this Tmax threshold of 35.7°C will be exceeded throughout the entire breeding season by approximately 2027 at our study site.
Table 2. Bayesian generalized linear mixed model (BGLMer) of the outcomes of different stages [entire breeding attempt (n = 88), nest survival from female entry until hatch/fail (“pre-hatch period”; n = 88), and nest survival from hatch until fledge/fail (“nestling period”; n = 88)] of individual breeding attempts in the southern yellow-billed hornbills (Tockus leucomelas) as functions of female entry date, percentage DaysTthresh {days during the attempt on which Tmax [mean maximum daily air temperature (Tair)] > Tthresh (Tair = 34.5°C)}, drought occurrence over the breeding season (19 October–24 March), and the interaction between DaysTthresh and drought occurrence.
Figure 4. The binomial probability of breeding attempt success [0 = fail, 1 = successfully fledging at least one chick)] by the southern yellow-billed hornbills (Tockus leucomelas) as a function of (A) the female entry date (days since the start of the season, i.e., 19 October), and (B) the percentage DaysTthresh {days during the attempt on which Tmax [mean maximum daily air temperature (Tair)] > Tthresh (Tair = 34.5°C)} for drought seasons (red dashed line; not significant) and non-drought seasons (solid black line; significant). The 95% confidence intervals are shown for significant effects.
One top model was identified for the probability of a breeding attempt successfully progressing from female entry to hatch (model weight: 0.66, Supplementary Table 2), with nests significantly less likely to progress to hatching when females entered the nest later (estimate: −1.24 ± 0.44; p = 0.005, Table 2). The interaction between the percentage DaysTthresh during the attempt and drought occurrence was significant (estimate −1.55 ± 0.77; p = 0.005, Table 2); the probability of a breeding attempt successfully progressing from female entry to hatch declined significantly with an increasing percentage DaysTthresh during the pre-hatch period within non-drought seasons (estimate: −1.22 ± 0.00; p = 0.013), but not within drought seasons (estimate: −0.91 ± 0.97; p = 0.310; Table 2) where successful hatches were low; 15 out of 23 (65.2%) attempts hatched in drought seasons, compared to 56 out of 65 (86.2%) in non-drought seasons.
During the nestling period, four top models for breeding attempt success were identified (model weights: 0.30, 0.20, 0.13, and 0.11 respectively; Supplementary Table 2). The model average of these four top models revealed that survival during the nestling period was positively correlated with an increased number of days post-hatch spent in the nest by the female (estimate: 1.08 ± 0.38; p = 0.005) and negatively correlated with an increased percentage DaysTthresh during the nestling period (estimate: −0.86 ± 0.37; p = 0.021; Table 2 and Supplementary Figure 5). The effect of drought occurrence on survival during the nestling period was not significant in the averaged model (estimate: 0.71 ± 0.92; p = 0.444, Table 2), although 4 of 15 chicks (26.7%) that hatched in drought seasons fledged, compared to 40 of 56 chicks (71.4%) in non-drought seasons (Supplementary Figure 6).
There were two top models identified for the effect of drought occurrence on female entry date, including the null model (model weights: 0.56 and 0.44 respectively; Supplementary Table 2), and on the number of days post-hatch spent in the nest by the female, also including the null model (model weights: 0.55 and 0.45 respectively; Supplementary Table 2). Model averages indicated that drought occurrence did not significantly affect the female entry date (estimate: −0.07 ± 0.11; p = 0.552) or the number of days post-hatch spent in the nest by the female (estimate: −0.03 ± 0.05; p = 0.550) for breeding attempts that hatched at least one chick (Table 2).
A single top model was identified for Tnest, indicating that Tnest inside both nest boxes and natural cavities showed a significant positive relationship with outside Tair (estimate: 1.03 ± 0.00; p = 0.001), but that there was a significant interaction between Tair and the type of nest (estimate: −0.49 ± 0.00; p = 0.001; Supplementary Tables 3, 4). The slope of the relationship between Tnest and Tair was 0.55 Tnest °C.Tair–1 for natural cavities (estimate: 0.55 ± 0.00; p = 0.001) compared to 1.03 Tnest °C.Tair–1 for nest boxes (estimate: 1.03 ± 0.00; p = 0.001), suggesting that Tnest within natural cavities was buffered against Tair, while Tnest within nest boxes tracked Tair (Supplementary Table 4 and Supplementary Figure 7). The difference between Tnest within nest boxes and natural cavities was most pronounced at higher Tair; at Tair > Tthresh, the mean Tnest within nest boxes was 39.65°C ± 3.23 SD, while the mean Tnest within natural cavities was 34.91°C ± 3.68 SD (Supplementary Figure 7).
The negative impacts of extreme weather events and a rapidly warming climate on survival and reproduction are being recorded worldwide across multiple taxa (Moreno and Møller, 2011; Cunningham et al., 2021). Intensive monitoring allowed us to quantify the breeding performance of our study population of hornbills over a decade. Breeding effort and performance of hornbills collapsed throughout our monitoring period, correlating to rapid climate warming in the region. Comparing the first three seasons (2008–2011) of monitoring to the last three (2016–2019), the percentage of boxes occupied declined from an average of 52–12%, the percentage of breeding attempts that succeeded from 58% to 17%, and the mean fledglings produced per attempt from 1.1 to 0.4. Breeding effort and performance were negatively correlated with both extrinsic (higher Tair and the occurrence of drought within the breeding season) and intrinsic (later dates of entry into the nest cavity and shorter time periods spent in the nest post-hatch by the female parent) factors. Given the severe negative effects of high Tair on both adult conditions (van de Ven et al., 2019) and breeding success (van de Ven et al., 2020a, this study) in this population of hornbills, we suggest that rapid warming has likely been the primary driver of their recent collapse in breeding success.
The decline in the percentage of nest boxes used over the decade of monitoring could be a result of fewer potential breeding pairs in the study population as the monitoring period progressed (e.g., Rioux Paquette et al., 2014; Cruz-McDonnell and Wolf, 2016). However, the number of pairs present was continuously estimated to be approximately 20–25 each year, and in the majority of years since 2012, pairs were seen inspecting nest boxes between October and March but failed to ultimately breed (Pattinson and van de Ven, unpublished data). Moreover, there was a significant link between higher seasonal Tair and reduced nest box occupancy. Therefore, the declining breeding effort likely reflects an increasing number of resident pairs skipping breeding in response to increasingly challenging environmental conditions (i.e., higher Tair during the summer breeding season); skipping breeding is common in another southern African bucerotiforme, the southern ground-hornbill (Bucorvus leadbeateri; Carstens et al., 2019), and is suspected to occur in response to poor breeding conditions in various avian, mammalian, and amphibian taxa (e.g., Pietiainen, 1989; Pilastro et al., 2003; Kinkead and Otis, 2007; Keynan and Yosef, 2010; Cayuela et al., 2014; Griffen, 2018). Nesting hornbills experience low rates of predation because the birds seal up the entrance of the nest, leaving only a tiny slit through which food can be passed from the male to the female and chicks inside (Moreau and Moreau, 1941), so changes in predation rates with Tair or drought are unlikely responsible for variation in breeding performance.
Nest boxes were less buffered against changes in Tair compared to natural cavities. Therefore, our findings may represent a more severe response to high Tair than would be evident in birds breeding in natural cavities, especially given the strong effect of Tnest on fledging conditions (van de Ven et al., 2020a). However, the Tair recorded at our field site was consistently lower than that recorded by weather stations in surrounding areas, suggesting that the trends we measured are likely at least indicative of what is happening to populations of hornbills breeding in natural cavities in the hottest parts of their range. Moreover, variation in nest success is also driven by the effects of Tair on the provisioning behavior of the parents (van de Ven et al., 2019; van de Ven et al., 2020a) and drought, which are effects independent of nest type.
Inter-annual and within-season declines in breeding success were strongly associated with both high temperatures and drought. Of the 115 breeding attempts we recorded, none were successful when Tmax exceeded Tthresh ≥ 72% or more days during an attempt. The hottest period during which an attempt was successful involved 55 of 76 days (72%) with Tmax > Tthresh, but all 18 attempts during hotter conditions failed, indicating the potential for the precipitous decline in breeding success at high Tair. Additionally, significantly more breeding attempts succeeded (fledged at least one chick) in non-drought seasons (61.5%) compared to drought seasons (17.4%). The near-ubiquitous nest failure across a range of Tair in drought years presumably occurred because low rainfall leads to low food abundance in arid and semi-arid systems since rainfall drives primary productivity and the energy available through the entire trophic cascade (Mares et al., 2017; Carstens et al., 2019). These results suggest that breeding performance in this population of hornbill is susceptible to both low rainfall and high Tair independently, compared to studies suggesting vulnerability only when low rainfall and high Tair co-occur (Flesch, 2014; Cruz-McDonnell and Wolf, 2016; Iknayan and Beissinger, 2018; Bourne et al., 2020b). Although our models did not indicate a statistically significant effect of drought during the nestling period, the much lower percent of nestlings that fledged in drought (26.7%, n = 4 out of 15) compared to non-drought (71.4%, n = 40 out of 56) seasons suggested that drought was associated with reduced nest success during both pre-hatch and nestling periods. In fact, the low number of chicks that hatch or fledge during drought seasons creates a limited sample size for analyses, likely reducing our power to statistically demonstrate the effect of drought.
Variation in breeding success within breeding seasons was also correlated to intrinsic factors including female entry date (when the female sealed herself in the nest box) and the number of days post-hatch the female was incarcerated in the nest. Later female entry date and fewer days post-hatch spent incarcerated by the female both correlated with lower breeding success. Delayed nest initiation is associated with drought and low resource availability in some species (McCreedy and van Riper, 2015; Carstens et al., 2019). However, rainfall in the 2 months preceding the breeding season had no significant effect on seasonal breeding output, and neither female entry dates nor the number of days post-hatch spent in the nest by the female parents were significantly related to the occurrence or absence of drought, suggesting that their effects were likely not artifacts of rainfall and food availability (Harriman et al., 2017). Rather, we suspect that lower-quality parents delay the onset of breeding and/or that lower-quality females cannot stay incarcerated for extended periods. Fewer stored body reserves in either parent may preclude beginning breeding early or the ability to sustain continuous mass loss during the breeding attempt (van de Ven et al., 2020a). This pattern reflects widespread interactions between the timing of breeding and the quality of the parents (for review, see Verhulst and Nilsson, 2008) and supports the well-established concept of earlier breeding and higher parental quality positively affecting reproductive performance (Moreno et al., 2005; Verhulst and Nilsson, 2008; de Zwaan et al., 2019).
Our analyses of Tair and rainfall trends in the southern Kalahari revealed trends consistent with those reported in recent studies (Kruger and Sekele, 2013; van Wilgen et al., 2016; Mbokodo et al., 2020); Tmax and DaysTthresh during the breeding season of the hornbills have increased by more than 1.0°C and almost 20 days per decade, respectively, over the last ∼25 years, whereas no long-term trend in rainfall/drought recurrence was apparent. This trend indicates that hornbills face severe challenges to their persistence across the seasonally hot, arid parts of their range. The negative effects of high temperatures will increasingly cause reduced breeding success even in non-drought years as global warming advances. Therefore, while the magnitude of population decline is limited by the severity of droughts and heatwaves, population recovery and persistence will be limited by a decreasing capacity of the hornbills to breed successfully in non-drought seasons due to increased mean Tair (Williams et al., 2016; Albright et al., 2017; Palmer et al., 2017; Conradie et al., 2019). Based on (1) the rapid rate of warming, (2) the fact that no breeding attempts succeeded if ≥ 72% days during the attempt had Tmax > Tthresh (corresponding to a Tmax during the attempt ≥ 35.7°C, a threshold which will be exceeded across the entire breeding season by ∼2027 at our study site under current warming trends), and (3) 8 years is the longest a wild, color-ringed hornbill has survived in our study population, we arrive at the grim prediction that this population of hornbills could be extirpated by 2040. Moreover, model predictions suggest that the majority of the hornbill’s range will approach the Tair threshold, above which breeding success is < 50% by the turn of the century (Conradie et al., 2019).
Many birds in seasonally hot, summer rainfall, arid zones are constrained to breed in response to, or to coincide with, rainfall, making it difficult for them to shift breeding dates outside of the hottest periods of the year which correspond with the rainfall season (Wolf, 2000; McCreedy and van Riper, 2015; Mares et al., 2017; Iknayan and Beissinger, 2018; van de Ven et al., 2020a). Therefore, even small increases in summer maximum Tair could drive large consequences during breeding (Sinervo et al., 2010; Alagaili et al., 2017; Albright et al., 2017; Riddell et al., 2019; McKechnie et al., 2021). Moreover, an increase in the frequency and severity of sub-lethal, suboptimal conditions that reduce parental quality may also reduce fledgling conditions, generally resulting in lower survival probability, recruitment, and lifetime fecundity (Dawson et al., 2005; Gardner et al., 2016; Conradie et al., 2019; de Zwaan et al., 2019; Bourne et al., 2020a; Cunningham et al., 2021) (but see McLean et al., 2020). In the case of hornbills, there is evidence that the sub-lethal consequences of high Tair (regardless of high rainfall) and drought on the parents affect offspring quality (van de Ven et al., 2020a), and in this study, the probability of successfully fledging offspring or even attempting to breed at all. While the unusual breeding strategy of the hornbills could make them especially vulnerable, high temperatures and drought have negative impacts on breeding output in a variety of taxa across the globe (Welbergen et al., 2008; Sinervo et al., 2010; Gardner et al., 2014; Andreasson et al., 2020; van de Ven et al., 2020b; Cunningham et al., 2021). Therefore, we suggest that our findings are likely applicable to a range of species and support the proposition that even for species where catastrophic heat-related mortality events remain unlikely, climate change can drive rapid declines and potentially local extinctions (Conradie et al., 2019; McKechnie and Wolf, 2019; McKechnie et al., 2021). In fact, both Iknayan and Beissinger (2018) and Riddell et al. (2019) recently demonstrated that this extirpation process in arid zones is already taking place and is set to continue under future climate change.
The raw data supporting the conclusions of this article will be made available by the authors, without undue reservation.
The animal study was reviewed and approved by the Science Faculty Animal Ethics Committee, University of Cape Town, permit no. 2012/V44/PH, 2013/V24/PR, 2018/V15/SC, 2019/V6/SC; 2019/V16/SC, the Animal Ethics Committee of the University of Pretoria (protocol NAS 361/2019) and the Research Ethics and Scientific Committee of the South African National Biodiversity Institute (P19–23). The study was carried out on private land (Kuruman River Reserve, Leeupan Guest Farm and Rus en Vreede farm) with permission of the landowners and of the Northern Cape Department of Environment and Nature Conservation of South Africa (permit numbers 995/2012, 660/2013, 1166/2013; FAUNA 0172/2019).
AM, NP, SC, and TV developed the conceptual framework. LN, MF, NP, and TV collected data. NP analyzed the data and wrote the manuscript. All authors contributed to manuscript editing and revision, and read and approved the submitted version.
This research was funded by the DST-NRF Center of Excellence at the FitzPatrick Institute, funding from SC, Natural Environment Research Council, and the National Research Foundation of South Africa (grant 119754 to AM).
Any opinions, findings, conclusions, or recommendations expressed in this article are those of the authors and do not necessarily reflect the views of the National Research Foundation of South Africa.
The authors declare that the research was conducted in the absence of any commercial or financial relationships that could be construed as a potential conflict of interest.
The reviewer LK declared a past collaboration with one of the authors AM to the handling editor.
All claims expressed in this article are solely those of the authors and do not necessarily represent those of their affiliated organizations, or those of the publisher, the editors and the reviewers. Any product that may be evaluated in this article, or claim that may be made by its manufacturer, is not guaranteed or endorsed by the publisher.
We would like to thank the DSI/NRF Center of Excellence at the FitzPatrick Institute of African Ornithology for funding this research. We would also like to thank the De Bruin family of Leeupan farm, the Kotze family of Rus en Vreede farm, and the Kalahari Research Trust for the support and access provided to their land. We would also further like to thank fieldwork assistants, Alex Thompson, Amy Hunter, Amy Tipton, Angela Moreras, Dannielle Keys, Dean Portelli, Josephine Bruning, Justin Jacobs, Kirsty Scott, Krista Oswald, Mark Whiteside, Rowan Jordaan, Sue-Joy Schultz, and Tim Vink. VZ and TR data were provided with permission by the SAWS through M. Mkhwanazi (SAWS, Pretoria, South Africa).
The Supplementary Material for this article can be found online at: https://www.frontiersin.org/articles/10.3389/fevo.2022.842264/full#supplementary-material
Alagaili, A. N., Bennett, N. C., Mohammed, O. B. I, Zalmout, S., and Boyles, J. G. (2017). Body temperature patterns of a small endotherm in an extreme desert environment. J. Arid Environ. 137, 16–20. doi: 10.1016/j.jaridenv.2016.10.010
Albright, T. P., Mutiibwa, D., Gerson, A. R., Smith, E. K., Talbot, W. A., O’Neill, J. J., et al. (2017). Mapping evaporative water loss in desert passerines reveals an expanding threat of lethal dehydration. Proc. Natl. Acad. Sci. U.S.A. 114, 2283–2288. doi: 10.1073/pnas.1613625114
Albright, T. P., Pidgeon, A. M., Rittenhouse, C. D., Clayton, M. K., Flather, C. H., Culbert, P. D., et al. (2010). Effects of drought on avian community structure. Global Change Biol. 16, 2158–2170. doi: 10.1111/j.1365-2486.2009.02120.x
Amélineau, F., Grémillet, D., Harding, A., Walkusz, W., Choquet, R., and Fort, J. (2019). Arctic climate change and pollution impact little auk foraging and fitness across a decade. Sci. Rep. 9:1014. doi: 10.1038/s41598-018-38042-z
Andreasson, F., and Nilsson, J. -Å, and Nord, A. (2020). Avian reproduction in a warming world. Front. Ecol. Evol. 8:337.
BirdLife International (2021). Species Factsheet: Tockus Leucomelas. IUCN Red List for Birds. Cambridge: BirdLife International.
Bolger, D. T., Patten, M. A., and Bostock, D. C. (2005). Avian reproductive failure in response to an extreme climatic event. Oecologia 142, 398–406. doi: 10.1007/s00442-004-1734-9
Bourne, A. R., Cunningham, S. J., Spottiswoode, C. N., and Ridley, A. R. (2020a). High temperatures drive offspring mortality in a cooperatively breeding bird. Proc. R. Soc. B 287:20201140. doi: 10.1098/rspb.2020.1140
Bourne, A. R., Cunningham, S. J., Spottiswoode, C. N., and Ridley, A. R. (2020b). Hot droughts compromise interannual survival across all group sizes in a cooperatively breeding bird. Ecol. Lett. 23, 1776–1788. doi: 10.1111/ele.13604
Bourne, A. R., Ridley, A. R., McKechnie, A. E., Spottiswoode, C. N., and Cunningham, S. J. (2021). Dehydration risk is associated with reduced nest attendance and hatching success in a cooperatively breeding bird, the southern pied babbler Turdoides bicolor. Conserv. Physiol. 9:coab043. doi: 10.1093/conphys/coab043
Carstens, K. F., Kassanjee, R., Little, R. M., Ryan, P. G., and Hockey, P. A. (2019). Breeding success and population growth of southern ground hornbills Bucorvus leadbeateri in an area supplemented with nest-boxes. Bird Conserv. Int. 29, 627–643. doi: 10.1017/s0959270919000108
Catry, I., Catry, T., Patto, P., Franco, A. M. A., and Moreira, F. (2015). Differential heat tolerance in nestlings suggests sympatric species may face different climate change risks. Clim. Res. 66, 13–24. doi: 10.3354/cr01329
Catry, I., Franco, A. M. A., and Sutherland, W. J. (2011). Adapting conservation efforts to face climate change: modifying nest-site provisioning for lesser kestrels. Biol. Conserv. 144, 1111–1119. doi: 10.1016/j.biocon.2010.12.030
Cayuela, H., Besnard, A., Bonnaire, E., Perret, H., Rivoalen, J., Miaud, C., et al. (2014). To breed or not to breed: past reproductive status and environmental cues drive current breeding decisions in a long-lived amphibian. Oecologia 176, 107–116. doi: 10.1007/s00442-014-3003-x
Chung, Y., Rabe-Hesketh, S., Dorie, V., Gelman, A., and Liu, J. (2013). A nondegenerate penalized likelihood estimator for variance parameters in multilevel models. Psychometrika 78, 685–709. doi: 10.1007/s11336-013-9328-2
Conradie, S. R., Woodborne, S. M., Cunningham, S. J., and McKechnie, A. E. (2019). Chronic, sublethal effects of high temperatures will cause severe declines in southern African arid-zone birds during the 21st century. Proc. Natl. Acad. Sci. U.S.A. 116, 14065–14070. doi: 10.1073/pnas.1821312116
Cooper, C. E., Hurley, L. L., Deviche, P., and Griffith, S. C. (2020). Physiological responses of wild zebra finches (Taeniopygia guttata) to heatwaves. J. Exp. Biol. 2020:223. doi: 10.1242/jeb.225524
R Core Team (2021). R: A Language and Environment for Statistical Computing. Vienna, Austria: R Foundation for Statistical Computing.
Cruz-McDonnell, K. K., and Wolf, B. O. (2016). Rapid warming and drought negatively impact population size and reproductive dynamics of an avian predator in the arid southwest. Global Change Biol. 22, 237–253. doi: 10.1111/gcb.13092
Cunningham, S. J., Gardner, J. L., and Martin, R. O. (2021). Opportunity costs and the response of birds and mammals to climate warming. Front. Ecol. Environ. 19, 300–307. doi: 10.1002/fee.2324
D’Amelio, P. B., Ferreira, A. C., Fortuna, R., Paquet, M., Silva, L. R., Theron, F., et al. (2021). Disentangling climatic and nest predator impact on reproductive output reveals adverse high-temperature effects regardless of helper number in an arid-region cooperative bird. Ecology letters 25, 151–162. doi: 10.1111/ele.13913
Dawson, R. D., Lawrie, C. C., and O’Brien, E. L. (2005). The importance of microclimate variation in determining size, growth and survival of avian offspring: experimental evidence from a cavity nesting passerine. Oecologia 144, 499–507. doi: 10.1007/s00442-005-0075-7
de Zwaan, D. R., Camfield, A. F., MacDonald, E. C., and Martin, K. (2019). Variation in offspring development is driven more by weather and maternal condition than predation risk. Funct. Ecol. 33, 447–456. doi: 10.1111/1365-2435.13273
Doolan, S., and Macdonald, D. (1997). Breeding and juvenile survival among slender-tailed meerkats (Suricatu suricatta) in the south-western kalahari: ecological and social influences. J. Zool. 242, 309–327. doi: 10.1111/j.1469-7998.1997.tb05804.x
du Plessis, K. L., Martin, R. O., Hockey, P. A. R., Cunningham, S. J., and Ridley, A. R. (2012). The costs of keeping cool in a warming world: implications of high temperatures for foraging, thermoregulation and body condition of an arid-zone bird. Global Change Biol. 18, 3063–3070. doi: 10.1111/j.1365-2486.2012.02778.x
Flesch, A. D. (2014). Spatiotemporal trends and drivers of population dynamics in a declining sonoran desert predator. Biol. Conserv. 175, 110–118. doi: 10.1016/j.biocon.2014.04.021
Frederiksen, M., Anker-Nilssen, T., Beaugrand, G., and Wanless, S. (2013). Climate, copepods and seabirds in the boreal Northeast Atlantic–current state and future outlook. Global Change Biol. 19, 364–372. doi: 10.1111/gcb.12072
Gardner, J. L., Amano, T., Mackey, B. G., Sutherland, W. J., Clayton, M., and Peters, A. (2014). Dynamic size responses to climate change: prevailing effects of rising temperature drive long-term body size increases in a semi-arid passerine. Global Change Biol. 20, 2062–2075. doi: 10.1111/gcb.12507
Gardner, J. L., Amano, T., Sutherland, W., Clayton, M., and Peters, A. (2016). Individual and demographic consequences of reduced body condition following repeated exposure to high temperatures. Ecology 97:786. doi: 10.1890/15-0642.1
Griffen, B. D. (2018). Reproductive skipping as an optimal life history strategy in the southern elephant seal, Mirounga leonina. Ecol. Evol. 8, 9158–9170. doi: 10.1002/ece3.4408
Harriman, V. B., Dawson, R. D., Bortolotti, L. E., and Clark, R. G. (2017). Seasonal patterns in reproductive success of temperate-breeding birds: experimental tests of the date and quality hypotheses. Ecol. Evol. 7, 2122–2132. doi: 10.1002/ece3.2815
Hatch, S. A. (2013). Kittiwake diets and chick production signal a 2008 regime shift in the Northeast Pacific. Mari. Ecol. Prog. Ser. 477, 271–284. doi: 10.3354/meps10161
Iknayan, K. J., and Beissinger, S. R. (2018). Collapse of a desert bird community over the past century driven by climate change. Proc. Natl. Acad. Sci. U.S.A. 115, 8597–8602. doi: 10.1073/pnas.1805123115
Kemp, A. C., and Woodcock, M. (1995). The Hornbills: Bucerotiformes. Oxford: Oxford University Press.
Kemp, R., Freeman, M. T., van Jaarsveld, B., Czenze, Z. J., Conradie, S. R., and McKechnie, A. E. (2020). Sublethal fitness costs of chronic exposure to hot weather vary between sexes in a threatened desert lark. Emu Austr. Ornithol. 120, 216–229. doi: 10.1080/01584197.2020.1806082
Keynan, O., and Yosef, R. (2010). Annual precipitation affects reproduction of the southern grey shrike (Lanius meridionalis). Wilson J. Ornithol. 122, 334–339. doi: 10.1676/09-148.1
Kinkead, K. E., and Otis, D. L. (2007). Estimating superpopulation size and annual probability of breeding for pond-breeding salamanders. Herpetologica 63, 151–162. doi: 10.1655/0018-0831(2007)63[151:essaap]2.0.co;2
Kruger, A. C., and Sekele, S. S. (2013). Trends in extreme temperature indices in South Africa: 1962–2009. Int. J. Clim. 33, 661–676. doi: 10.1002/joc.3455
Long, J. A. (2020). Package Jtools: Analysis and Presentation of Social Scientific data. Rpackage Version 2.1.0.
Mares, R., Doutrelant, C., Paquet, M., Spottiswoode, C. N., and Covas, R. (2017). Breeding decisions and output are correlated with both temperature and rainfall in an arid-region passerine, the sociable weaver. R. Soc. Open Sci. 4:170835. doi: 10.1098/rsos.170835
Mbokodo, I., Bopape, M.-J., Chikoore, H., Engelbrecht, F., and Nethengwe, N. (2020). Heatwaves in the future warmer climate of south africa. Atmosphere 11:712. doi: 10.3390/atmos11070712
McCowan, L. S., and Griffith, S. C. (2021). Baked Eggs: Catastrophic Heatwave-Induced Reproductive Failure In The Desert-Adapted Zebra Finch (Taeniopygia Guttata).
McCreedy, C., and van Riper, C. III (2015). Drought-caused delay in nesting of sonoran desert birds and its facilitation of parasite-and predator-mediated variation in reproductive success. AUK Ornithol. Adv. 132, 235–247. doi: 10.1642/auk-13-253.1
McKechnie, A. E., Rushworth, I. A., Myburgh, F., and Cunningham, S. J. (2021). Mortality among birds and bats during an extreme heat event in eastern South Africa. Austr. Ecol. 46, 687–691.
McKechnie, A. E., and Wolf, B. O. (2019). The physiology of heat tolerance in small endotherms. Physiology 34, 302–313. doi: 10.1152/physiol.00011.2019
McLean, N. M., van der Jeugd, H. P., Van Turnhout, C. A., Lefcheck, J. S., and Van de Pol, M. (2020). Reduced avian body condition due to global warming has little reproductive or population consequences. Oikos 129, 714–730. doi: 10.1111/oik.06802
Moagi, L. L., Bourne, A. R., Cunningham, S. J., Jansen, R., Ngcamphalala, C. A., Ganswindt, A., et al. (2021). Hot days are associated with short-term adrenocortical responses in a southern African arid-zone passerine bird. J. Exp. Biol. 224:jeb242535. doi: 10.1242/jeb.242535
Moreno, J., and Møller, A. P. (2011). Extreme climatic events in relation to global change and their impact on life histories. Curr. Zool. 57, 375–389. doi: 10.1093/czoolo/57.3.375
Moreno, J., Merino, S., Sanz, J. J., Arriero, E., Morales, J., and Tomás, G. (2005). Nestling cell-mediated immune response, body mass and hatching date as predictors of local recruitment in the pied flycatcher Ficedula hypoleuca. J. Avian Biol. 36, 251–260. doi: 10.1111/j.0908-8857.2005.03413.x
Muggeo, V. M. R. (2017). Interval estimation for the breakpoint in segmented regression: a smoothed score-based approach. Aust. N. Z. J. Stat. 59, 311–322. doi: 10.1111/anzs.12200
Oswald, K. N., Smit, B., Lee, A. T., Peng, C. L., Brock, C., and Cunningham, S. J. (2021). Higher temperatures are associated with reduced nestling body condition in a range-restricted mountain bird. J. Avian Biol. 52.
Pachauri, R. K., Allen, M. R., Barros, V. R., Broome, J., Cramer, W., Christ, R., et al. (2014). Climate Change 2014: Synthesis Report. Contribution of Working Groups I, II and III to the fifth Assessment Report Of The Intergovernmental Panel On Climate Change. IPCC.
Palmer, G., Platts, P. J., Brereton, T., Chapman, J. W., Dytham, C., Fox, R., et al. (2017). Climate change, climatic variation and extreme biological responses. Philos. Trans. R. Soc. London B Biol. Sci. 372:20160144. doi: 10.1098/rstb.2016.0144
Pietiainen, H. (1989). Seasonal and individual variation in the production of offspring in the ural owl Strix uralensis. J. Animal Ecol. 1989, 905–920. doi: 10.2307/5132
Pilastro, A., Tavecchia, G., and Marin, G. (2003). Long living and reproduction skipping in the fat dormouse. Ecology 84, 1784–1792. doi: 10.1890/0012-9658(2003)084[1784:llarsi]2.0.co;2
Riddell, E. A., Iknayan, K. J., Wolf, B. O., Sinervo, B., and Beissinger, S. R. (2019). Cooling requirements fueled the collapse of a desert bird community from climate change. Proc. Natl. Acad. Sci. U.S.A. 116, 21609–21615. doi: 10.1073/pnas.1908791116
Ridley, A. R., and Raihani, N. J. (2007). Variable postfledging care in a cooperative bird: causes and consequences. Behav. Ecol. 18, 994–1000. doi: 10.1093/beheco/arm074
Ridley, A. R., Wiley, E. M., Bourne, A. R., Cunningham, S. J., and Nelson-Flower, M. J. (2021). Understanding the potential impact of climate change on the behavior and demography of social species: The pied babbler (Turdoides bicolor) as a case study. Adv. Study Behav. 53, 225–266.
Rioux Paquette, S., Pelletier, F., Garant, D., and Bélisle, M. (2014). Severe recent decrease of adult body mass in a declining insectivorous bird population. Proc. R. Soc. B Biol. Sci. 281:20140649. doi: 10.1098/rspb.2014.0649
Salaberria, C., Celis, P., López-Rull, I., and Gil, D. (2014). Effects of temperature and nest heat exposure on nestling growth, dehydration and survival in a mediterranean hole-nesting passerine. IBIS 156, 265–275.
Seabrook, L., McAlpine, C., Rhodes, J., Baxter, G., Bradley, A., and Lunney, D. (2014). Determining range edges: habitat quality, climate or climate extremes? Div. Distr. 20, 95–106. doi: 10.1111/ddi.12152
Sharpe, L. L., Bayter, C., and Gardner, J. L. (2021). Too hot to handle? Behavioural plasticity during incubation in a small, Australian passerine. J. Thermal Biol. 98:102921. doi: 10.1016/j.jtherbio.2021.102921
Sharpe, L., Cale, B., and Gardner, J. L. (2019). Weighing the cost: the impact of serial heatwaves on body mass in a small Australian passerine. J. Avian Biol. 2019:50.
Sinervo, B., Mendez-De-La-Cruz, F., Miles, D. B., Heulin, B., Bastiaans, E., Villagrán-Santa Cruz, M., et al. (2010). Erosion of lizard diversity by climate change and altered thermal niches. Science 328, 894–899. doi: 10.1126/science.1184695
Stanback, M., Millican, D., Versfeld, W., Nghikembua, M., Marker, L., and Mendelsohn, J. (2021). Double-brooding in southern yellow-billed hornbills tockus leucomelas. Ostrich 92, 105–112. doi: 10.2989/00306525.2021.1891479
van de Ven, T. M. F. N., McKechnie, A. E., and Er, S., and Cunningham S. J. (2020a). High temperatures are associated with substantial reductions in breeding success and offspring quality in an arid-zone bird. Oecologia 193, 225–235. doi: 10.1007/s00442-020-04644-6
van de Ven, T. M. F. N., Fuller, A., and Clutton-Brock, T. H. (2020b). Effects of climate change on pup growth and survival in a cooperative mammal, the meerkat. Funct. Ecol. 34, 194–202. doi: 10.1111/1365-2435.13468
van de Ven, T. M. F. N., McKechnie, A. E., and Cunningham, S. J. (2019). The costs of keeping cool: behavioural trade-offs between foraging and thermoregulation are associated with significant mass losses in an arid-zone bird. Oecologia 191, 205–215. doi: 10.1007/s00442-019-04486-x
Van Jaarsveld, B., Bennett, N. C., Czenze, Z. J., Kemp, R., van de Ven, T. M. F. N., Cunningham, S. J., et al. (2021). How hornbills handle heat: sex-specific thermoregulation in the southern yellow-billed hornbill. J. Exp. Biol. 224:jeb232777. doi: 10.1242/jeb.232777
van Wilgen, N. J., Goodall, V., Holness, S., Chown, S. L., and McGeoch, M. A. (2016). Rising temperatures and changing rainfall patterns in South Africa’s national parks. Int. J. Clim. 36, 706–721. doi: 10.1002/joc.4377
Verhulst, S., and Nilsson, J. -Å (2008). The timing of birds’ breeding seasons: a review of experiments that manipulated timing of breeding. Philos. Trans. R. Soc. B Biol. Sci. 363, 399–410. doi: 10.1098/rstb.2007.2146
Welbergen, J. A., Klose, S. M., Markus, N., and Eby, P. (2008). Climate change and the effects of temperature extremes on Australian flying-foxes. Proc. R. Soc. London B Biol. Sci. 275, 419–425. doi: 10.1098/rspb.2007.1385
Williams, C. M., Buckley, L. B., Sheldon, K. S., Vickers, M., Pörtner, H.-O., Dowd, W. W., et al. (2016). Biological impacts of thermal extremes: mechanisms and costs of functional responses matter. Int. Comparat. Biol. 56, 73–84.
Keywords: southern yellow-billed hornbill, high temperatures, drought, breeding, climate change, arid-zone bird
Citation: Pattinson NB, van de Ven TMFN, Finnie MJ, Nupen LJ, McKechnie AE and Cunningham SJ (2022) Collapse of Breeding Success in Desert-Dwelling Hornbills Evident Within a Single Decade. Front. Ecol. Evol. 10:842264. doi: 10.3389/fevo.2022.842264
Received: 23 December 2021; Accepted: 19 April 2022;
Published: 19 May 2022.
Edited by:
Rohit Naniwadekar, Nature Conservation Foundation, IndiaReviewed by:
Leigh Combrink, Oregon State University, United StatesCopyright © 2022 Pattinson, van de Ven, Finnie, Nupen, McKechnie and Cunningham. This is an open-access article distributed under the terms of the Creative Commons Attribution License (CC BY). The use, distribution or reproduction in other forums is permitted, provided the original author(s) and the copyright owner(s) are credited and that the original publication in this journal is cited, in accordance with accepted academic practice. No use, distribution or reproduction is permitted which does not comply with these terms.
*Correspondence: Nicholas B. Pattinson, bmlja3BhZGRpZUBnbWFpbC5jb20=
†These authors share senior authorship
Disclaimer: All claims expressed in this article are solely those of the authors and do not necessarily represent those of their affiliated organizations, or those of the publisher, the editors and the reviewers. Any product that may be evaluated in this article or claim that may be made by its manufacturer is not guaranteed or endorsed by the publisher.
Research integrity at Frontiers
Learn more about the work of our research integrity team to safeguard the quality of each article we publish.