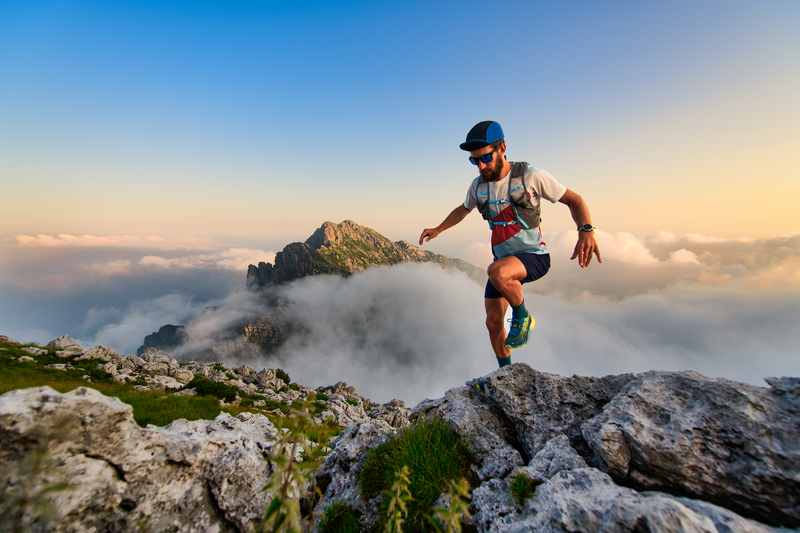
95% of researchers rate our articles as excellent or good
Learn more about the work of our research integrity team to safeguard the quality of each article we publish.
Find out more
ORIGINAL RESEARCH article
Front. Ecol. Evol. , 16 March 2022
Sec. Ecophysiology
Volume 10 - 2022 | https://doi.org/10.3389/fevo.2022.841318
This article is part of the Research Topic Ecophysiological Analysis of Vulnerability to Climate Warming in Ectotherms View all 15 articles
Investigating how aquatic animals respond to hypoxia brought about by changes in environmental temperature may be of great significance to avoid oxidative injury and maintain the quality of farmed fish in the background of global warming. Here, we investigated the effects of hypoxia on oxidative injury and environment-sensing pathway in blood cells of Micropterus salmoides. The total blood cell count (TBCC) and Giemsa staining showed that hypoxia could lead to damage of blood cells. Flow cytometry analysis confirmed that the apoptosis rate, Ca2+ level, NO production and ROS of blood cells were significantly increased under hypoxia stress. Environment-sensing pathways, such as Nrf2 pathway showed that hypoxia resulted in significant up-regulation of hiF-1 alpha subunit (Hif-1α), nuclear factor erythroid 2-related factor 2 (Nrf2) and kelch-1ike ECH- associated protein l (Keap1) expression. Meanwhile, the expression of Hippo pathway-related genes such as MOB kinase activator 1 (MOB1), large tumor suppressor homolog 1/2 (Lats1/2), yes-associated protein/transcriptional co-activator with PDZ-binding motif (YAP/TAZ), protein phosphatase 2A (PP2A) were significantly increased in blood cells after hypoxia exposure. In addition, hypoxia stress also increased the expression of catalase (CAT) and glutathione peroxidase (GPx), but decreased the expression of superoxide dismutase (SOD). Consequently, our results suggested that hypoxia could induce oxidative injury and apoptosis via mediating environment-sensing pathway such as Nrf2/Hippo pathway in blood cells of M. salmoides.
The effects of global warming have become increasingly prevalent in marine ecosystems around the world, where consistently increasing seawater temperatures have exposed marine species to environmental stress conditions. Climate warming has already led to many changes in key environmental factors such as water temperature, seawater pH and oxygen concentration in the oceans (Breitburg et al., 2018; Vagner et al., 2019). Numerous factitious and environmental factors such as high temperature, water pollution and high-density fish farming in the water body will inevitably lead to hypoxic conditions in fish (Wang et al., 2012; Levin and Breitburg, 2015). Hypoxia is one of the main reasons that threatens the survival and growth of fish, it causes massive fish deaths and significant economic losses in the aquaculture industry, and seriously hinder the sustainable and healthy development of the aquaculture industry (Zhao et al., 2020).
Hypoxia may induce oxidative stress by breaking the balance between the production of reactive oxygen species (ROS) and the removal of ROS. Excess ROS are generated, which can damage critical cellular components and activate the antioxidant signaling pathway in fish (Azimi et al., 2017). Further, large amounts of ROS could cause apoptosis, lipid oxidation, tissue damage, and DNA and protein degradation (Jls et al., 2020). Nitric oxide (NO), as a cellular signaling molecule, has also been found to play a primary role of vasorelaxation in hypoxic situations and can be synthesized by nitric oxide synthase (NOS; Cao et al., 1981). When activated by pathogens or cytokines, such as ROS (Kumar et al., 2018), NO could be produced by NOS (Dimmeler and Zeiher, 1997). Further, NO reacts with ROS and forms peroxynitrite (ONOO−), which is highly reactive toward a wide variety of biomolecules (Curtin et al., 2002). There is also growing evidence that NO damages mitochondria, leading to the opening of transition pores, followed by cytochrome c release and caspase activation leading to apoptosis (Brüne, 2003). Earlier studies indicated that when compared with the population at sea level, the contents of NO and its products in Tibetan circulation are significantly higher, indicating that NO had effects on vascular tension, blood flow and oxygen delivery (Erzurum et al., 2007). Meanwhile, Danio rerio data support this possibility, accompanied by significant NO production, vasodilation and lower blood pressure (Jensen, 2007). Apoptosis is a critical component in maintaining homeostasis and growth in all tissues and plays a significant role in immunity and cytotoxicity, and it can initiate and clear damaged cells to maintain homeostasis when exposed to hypoxia (Luo et al., 2017; D’Arcy, 2019). However, the challenge that hypoxia poses to oxidative stress and the mechanisms that hypoxia induce oxidative injury and apoptosis in fish are not as well understood despite intensive research effort (Borowiec and Scott, 2020).
The antioxidant system can alleviate the negative effects of hypoxia on the body by preventing the production of ROS or by eliminating ROS, and it is mainly mediated by the transcription factor nuclear factor erythroid 2 (NFE2)-related factor 2 (Nrf-2), which can induce the expression of genes related to exogenous detoxification and cell protection (Barrera et al., 2021). As an environment-sensing pathway, Nrf-2 and its repressor, Kelch-like ECH-associated protein 1 (Keap1) can help the body defend against oxidative stress, thereby maintaining the body’s homeostasis balance (Bellezza et al., 2018). Some research indicated that the interruption of Nrf-2-Keap1 signaling may occur during human aging and cause sarcopenia (Mondal et al., 2018). When oxidative stress occurs, Keap1 is oxidized at the active cysteine residue, causing Keap1 to inactivate, Nrf-2 to stabilize and translocate into the nucleus (Motohashi et al., 2002; Tonelli et al., 2018). The Hippo pathway also is an environment-sensing pathway, which always be a master regulator of tissue homeostasis (Hong et al., 2020). It is an evolutionarily conserved signal cascade that regulates many biological processes, including cell growth and fate determination, organ size control and regeneration (Ma et al., 2019). In previous studies, sterile 20-like kinase 1/2 (Mst1/2) kinases in Hippo pathway are associated with Salvador family WW domain containing protein 1 (SAV1) and Mps one binder kinase activator-like 1A and 1B (MOB1A/B or collectively, MOB1) to phosphorylate large tumor suppressor 1/2 (Lats1/2). Lats1/2 subsequently phosphorylate Yes- associated protein (YAP) and its paralog, WW domain–containing transcription regulator protein 1 (TAZ), causing them to bind via 14-3-3 (Zhao et al., 2007; Hao et al., 2008; Lei et al., 2008; Oka et al., 2008). Hereafter, YAP and TAZ are prevented from entering the nucleus, interacting with transcription factors (i.e., TEAD family members and others) in the cytoplasm and regulating downstream gene targets to initiate a series of signal cascades (Zhao et al., 2008; Zhang et al., 2009; Lehmann et al., 2016; Meng et al., 2016). Studies have shown that Nrf-2 could uses the Hippo pathway effector TAZ to induce tumorigenesis (Barrera et al., 2021). Meanwhile, the excessive ROS generated by hypoxia, superoxide dismutase (SOD) can efficiently convert superoxide anion (O2–) into H2O2 and O2. After this process, H2O2 could be transformed into H2O and O2 under the combined action of catalase (CAT) and glutathione peroxidase (GPx), so that the body could be protected from hypoxic damage (Cao et al., 2012). However, there have been relatively few detailed examinations about how hypoxia induces oxidative damage and apoptosis through the Nrf-2/Hippo pathway in hypoxia-tolerant fish.
Largemouth bass (Micropterus salmoides), which is a world-famous economic fish, has been widely cultivated and become the major freshwater aquaculture species in China (Li et al., 2017; Yu et al., 2018). But the recent increase in global warming, high-density farming, and deterioration of water quality have increased the probability of hypoxia, which will inevitably lead to hypoxia stress (Sun et al., 2020c). Blood is not only an important part of the digestive and respiratory systems, but also plays important role in the immune system (Dichiera et al., 2020). Previous studies have also shown that acute hypoxic stress can lead to apoptosis and inflammatory responses in fish blood cells, and in severe cases can cause a dramatic reduction in the oxygen-carrying capacity of fish blood cells, resulting in mass mortality (Huang et al., 2019; Cai et al., 2020). Understanding the physiological changes of fish blood cells under hypoxic conditions is one of the keys to understanding how environmental stress causes oxidative damage and cell apoptosis in fish. However, in recent years, research on largemouth bass under hypoxic stress has mainly focused on some other tissue such as liver and gill (Sun et al., 2020b). For example, hypoxia could induce an increase in anaerobic glycolysis in the liver and caused histological lesions in the gill tissue of the fish (Gaulke et al., 2014; Crans et al., 2015; Yang et al., 2017). It is little known about the mechanism of how hypoxia induces oxidative damage and apoptosis in blood cells of largemouth bass.
In this study, we simulated an acute hypoxia environment in the laboratory and analyzed the physiological parameters, environment-sensing pathways such as Nrf-2/Hippo pathway and antioxidant ability of largemouth bass exposed to acute hypoxic environment for various durations. The dynamic pattern of physiological parameters and the pattern of Nrf-2/Hippo pathway during hypoxic exposure would provide a theoretical basis for future studies on healthy aquaculture of largemouth bass and facilitate our understanding of the mechanisms in hypoxia stress and global warming adaptation.
Largemouth bass were obtained from the Panyu aquaculture base of South China Normal University. Fish with no disease, no injury, and strong vigor were selected. The average weight of experimental fish was 108.4 ± 5.2 g and the average body length was 18.5 ± 3.1 cm. Before the trial, the water quality indicators were detected daily (pH 7.6 ± 0.2, temperature 25 ± 1°C, ammonia nitrogen ≤ 0.05 mg/L, and dissolved oxygen(DO) ≥ 6.5 mg/L). The fish were temporarily cultured with continuous ventilation for 2 weeks of domestication and were fed by Commercial feed (Guangdong Evergreen Feed industry Co., Ltd., Guangdong, China) during domestication. The fish were fasted for 24 h before the experiment.
After an 2-week acclimation period prior to the experiments, 160 largemouth bass which held in one big tank (1000 cm × 525 cm × 100 cm, water volume 520 L) were seperated into 16 plastic aquaria (50 cm × 35 cm × 30 cm, water volume 52 L). 10 largemouth bass were placed in each aquarium and each group contains four aquaria. The initial dissolved oxygen concentration of the culture water was around 6.50 mg/L. Before hypoxia treatments, three largemouth bass were randomly selected from four aquaria (Control group, DO = 6.50 mg/L) for sample collection. In order to start the hypoxia state, the aquaria were not subjected to oxygenation and circulation, and the mouth of aquaria were sealed with a thin film, and the dissolved oxygen in the tanks was measured once per 5 min. The oxygen in the aquaria gradually decreased with the consumption of largemouth bass. Three samples were collected from each experimental group after around 20 min (H1 group, DO = 4.33 mg/L), around 40 min (H2 group, DO = 3.25 mg/L) of hypoxia exposure. After acute hypoxic stress, the remaining largemouth bass were re-oxygenated for 50 min and the dissolved oxygen reached 7.09 mg/L, which was recorded as the R group and then three fish were sampled. Blood cells were withdrawn from the tail vein and immediately transferred into individual plastic tubes. All samples were kept on ice to prevent blood cells clumping.
A glass microscope slide was placed on a horizontal surface, and 10 μL blood was added onto one end of the slide. A coverslip was used, lying across the glass slide and keeping the coverslip in contact with the blood. The coverslip was moved down the glass slide to the opposite end. The blood smear is made after air-dried. Blood smears were stained with Reisser-Giemsa dye (Biosharp Biotechnology Co., Ltd., Anhui, China). Garris-Giemsa A solution was dropped on the smear, and the whole smear was stained with the dye for 0.5 min. Then drop the Reisch-Jimsa B solution onto liquid A, blow the breeze with the mouth or ear ball to make the liquid surface ripple, make the two liquids fully mixed, dyeing for 1 min before being washed in distilled water. Finally, it was observed with polarized light microscope (Leica, Germany) and photographed after drying. At the same time, the blood cells after multiple dilutions were counted on the counting board under an ordinary microscope.
The blood was centrifuged at 500 × g, 4°C for 10 min, and the supernatant was discarded after centrifugation. Then red blood cell lysis buffer was added to the cell pellet to lyse mature erythrocytes for 5 min. After lysis, the supernatant lysate was removed by centrifugation, and proper amount of PBS was added to re-precipitate the suspension. Then the blood cells were analyzed with a BD FACS Aria III flow cytometer (BD, NJ, United States).
Annexin V-FITC/PI Fluorescence double staining apoptosis assay kit (Elabscience Biotechnology Co., Ltd., Hubei, China) was used to stain the cells. The cells were suspended with 100 μL diluted 1 × Annexin V Binding Buffer, then 2.5 μL Annexin V-FITC and 2.5 μL PI staining solution were added to cell suspension. The mixed buffer were incubated for 15 min at room temperature in the dark. 400 μL of diluted 1 × Annexin V Binding Buffer was added to make the cell concentration 1.0 × 105 cells/mL and mixed the samples. The samples were placed on ice in darkness and tested by flow cytometry within 1 h and data were analyzed by the Flow Jo 10 software (version 10.2).
Intracellular ROS was estimated by using a fluorescent probe, 2′,7′-dichlorodihydrofluorescein diacetate (DCFH-DA). The cells were stained by Meilun Reactive Oxygen Species Assay Kit (Dalian Meilun Biotechnology Co., Ltd., Liaoning, China). After the cells were collected, the cells were suspended and added with an appropriate volume of diluted DCFH-DA working solution. The cells were incubated in a cell culture box at 37°C for 30 min under dark conditions, and mixed upside down every 5 min to make the probe and cells fully contact. After incubation, the cells were washed with PBS 3 times to fully remove DCFH-DA that did not enter the cells. Collected to make single-cell suspensions. Then the fluorescence was determined by flow cytometry.
DAF-FM DA (NO fluorescent photoprobe) (Dalian Meilun Biotechnology Co., Ltd., Liaoning, China) was used to stain the cells, and the cells were suspended with diluted DAF-FM DA and incubated for 20 min in a cell culture box at 37°C. Mix upside down every 3–5 min so that the probe is in full contact with the cells. After incubation, the cells were washed with PBS 3 times to fully remove the DAF-FM DA that did not enter the cells. Then the cells were re-suspended by PBS to prepare single-cell suspension for flow cytometry.
Fluo-3 and AM ester (eBioscience Biotechnology, MA, United States) were used to stain the cells. The calcium ion (Ca2+) fluorescent probe Fluo-3 and AM ester were added to the cells and incubated at 37°C for 20 min in the dark. Cells were washed three times with PBS and collected to make single-cell suspensions. The intracellular fluorescence intensity measured by flow cytometry represented the Ca2+ level.
Total RNA was extracted from the blood cells of fish with Trizol (Takara Biotech, Kyoto, Japan) and their quality and quantity were determined by a Nanodrop2000 spectrophotometer (Thermo, MA, United States), and t the integrity was assessed by 1% agarose gel electrophoresis (Wang et al., 2015). The cDNA was synthesized by reverse transcription with PrimeScript reverse transcriptase (Takara Biotech, Kyoto, Japan) in a 20 μL reaction volume containing 1 μg total RNA. The reaction mixture was stored at −20°C for future use. Quantitative real-time RT-PCR (qRT-PCR) experiments were performed in a CFX96 Multicolor Real-Time PCR Detection System (Bio-Rad Laboratories, Inc., CA, United States) using SYBR Mixture (Takara Biotech, Kyoto, Japan). Use β-actin as an internal reference gene. Each sample was paralleled three times. PCRs were performed on a total reaction volume of 20 μL containing 4 μL of cDNA, 0.5 μL of primer, 10 μL of SYBR Mixture, and 5 μL of ddH2O. The thermal cycling program was as follows: activation at 95°C for 10 min, followed by 35 cycles of 95°C for 10 s, several annealing temperatures for 30 s, and 72°C for 32 s; melt curve detection of 60°C for 5 s to 95°C increments 0.5°C. Quantitative analyses were performed using the 2–ΔΔCt method. The PCR cycling protocol was 95°C for 60 s, 40 cycles of 94°C for 5 s and 60°C for 30 s, followed by 95.0°C for 5 sec, followed by melting curve analysis from 65.0 to 95.0°C (increment 0.5°C, 0:05). Quantitative analyses were performed using the 2–ΔΔCt method. The primer sequences are described in Table 1.
All analyses were carried out using the SPSS software (version 26.0). The data were analyzed by single-factor analysis of variance (ANOVA) and multiple comparisons by LSD method. The data were expressed as mean ± standard deviation (SD, n = 3). P < 0.05 indicated significant differences.
The results of the Giemsa staining (Figure 1A) of the blood cells showed a clearly significant decrease in the H1 and H2 group relative to control group. Interestingly, macrophage-like cells could be found in hypoxia groups, especially in H2 group.
Figure 1. Effects of hypoxia on (A) cell morphology and (B) total blood cell count in largemouth bass after hypoxia. Scale bar = 20 μm (top), 5 μm (bottom).
After oxidative stress, compared to the control group (1.70 × 109 ± 0.36 × 109 cell/ml), the TBCC of treatment group decreased at H1 (1.26 × 109 ± 0.1 × 109 cell/ml) (P < 0.05) and H2 (1.01 × 109 ± 0.21 × 109 cell/ml) (P < 0.05), and remain unchanged in the R group (0.94 × 109 ± 0.04 × 109 cell/ml) (P > 0.05), while the TBCC of H1 group decreased more than H2 group (Figure 1B). And this result showed a similar trend which was consistent with Giemsa staining.
After exposure to acute hypoxic conditions, the early (Q3 region) and late apoptosis (Q2 region) were increased in a dose dependent manner (Figure 2A). Further quantified these apoptosis cells by software, the number of apoptotic cells In Q2 and Q3 region were 22.72, 42.9 and 35.77% after treated with H1, H2, and R (P < 0.05, Figure 2B). Compared to the control group (10.52%), the proportion of apoptotic cells was demonstrated to be significantly increased from H1 to H2, peaking at H2, and subsequently declining (R). These results revealed that acute hypoxic conditions could promote apoptosis.
Figure 2. Effect of apoptosis in the blood cell of largemouth bass under acute hypoxia and reoxygenation. (A) Cell apoptosis rate was determined by flow cytometry. (B) Bar graph of apoptosis due to normoxia and hypoxia. Data are expressed as the mean ± SD (n = 3; one-way analysis of variance followed by the T-test). All experiments were conducted in triplicate. Different letters denote significant differences (P < 0.05).
The level of Ca2+ fluorescence was low when the largemouth bass treated with control dissolved oxygen, while the level of Ca2+ fluorescence in H1 (52.83 ± 0.75) was two times higher than control group (20.53 ± 0.93). With the decrease of dissolved oxygen, the level of Ca2+ fluorescence was apparently increased at H1 and H2 group (P < 0.05, Figure 3), the level of Ca2+ fluorescence show the greatest peak in the H2 group, and the fluorescence intensity of the H2 group (61.63 ± 1.27) was about three times that of the control group. In addition, the level of Ca2+ fluorescence was decelerated after reoxygenation (58.63 ± 0.68).
Figure 3. The change of Ca2+ levels in the blood cell of largemouth bass under acute hypoxia and reoxygenation. (A) The Ca2+ levels of blood cell was determined by flow cytometry. (B) The Ca2+ levels for each cell treatment. Data are expressed as the mean ± SD (n = 3; one-way analysis of variance followed by the T-test). All experiments were conducted in triplicate. Different letters denote significant differences (P < 0.05).
After largemouth bass treated with different dissolved oxygen, the level of Nitric oxide (NO) fluorescence was significantly increased when compared to the control group (20.87 ± 0.29) (P < 0.05, Figure 4). With the decrease of dissolved oxygen, the level of NO fluorescence was increased in a dependent manner at H1 and H2 group (26.7 ± 0.10, 35.47 ± 0.21). And after exposure to reoxygenation, the level of NO fluorescence was shown to be significantly decreased at R group (33.43 ± 0.25) (P < 0.05).
Figure 4. The difference of NO production in the blood cell of largemouth bass under acute hypoxia and reoxygenation. (A) The NO production of blood cell was determined by flow cytometry. (B) The NO production for each cell treatment. Data are expressed as the mean ± SD (n = 3; one-way analysis of variance followed by the T-test). All experiments were conducted in triplicate. Different lowercase letters above each bar represent significant differences (P < 0.05).
The level of ROS production of H1, H2 and R group were determined to be 37.80 ± 0.26, 45.83 ± 0.38 and 43.90 ± 0.26. In comparison with the control (31.97 ± 0.50), ROS formation significantly increase in H1 and H2 (P < 0.05, Figure 5); which the ROS formation was downregulated at R group. Although there was a subsequent upward trend, he level of ROS fluorescence remained significantly higher than those of control (P < 0.05).
Figure 5. The change of respiratory burst in the blood cell of largemouth bass under acute hypoxia and reoxygenation. (A) The respiratory burst of blood cell was determined by flow cytometry. (B) The respiratory burst for each cell treatment. Data are expressed as the mean ± SD (n = 3; one-way analysis of variance followed by the T-test). All experiments were conducted in triplicate. Different lowercase letters above each bar represent significant differences (P < 0.05).
The expression of hypoxic sensor protein Hypoxia-inducible factor-1 alpha (Hif-1α) gene was shown to be continuously increasing in H1 and H2 group during the hypoxic exposure stage, whereas decreasing to its lowest value at R group, which was not significantly different from that in Control (P < 0.05). Subsequently, the Hif-1α level was shown to be significantly increased in H1 and H2 group (P < 0.05), reaching its highest value in H2, with significant differences observed between Control, H1 and R (P < 0.05, Figure 6A). The expression level of Nrf-2 gene decreased to the lowest value in H1 first, then significantly increased (P < 0.05, Figure 6B), peaking at H2 group. During the hypoxic exposure stage, the Nrf-2 gene expression decreased obviously in R, which still higher than that in H1 and control group. Simultaneously, it was significantly different from that in H1 and H2 group (P < 0.05, Figure 6B). Expression of Keap1 gene was demonstrated to be continuously increased during normoxia to hypoxia stress, with H2 exhibiting the highest value, which was distinctly different from that in Control and H1 group (P < 0.05, Figure 6C). Nevertheless, after reoxygenation, the expression of Keap1 in Group R was significantly lower than that of H2 (P < 0.05, Figure 6C), but still obviously higher than that in Control and H1 (P < 0.05, Figure 6C). Throughout the study, the levels of Hif-1α and Keap1 showed similar trends. More noteworthy phenomenon was that the expression level of Keap1 in H1 was five times higher than that of Nrf-2, and the highest expression level of Keap1 was about three times higher than the highest expression level of Nrf-2. In addition, the expression level of Keap1 and Nrf-2 genes downregulated during the reoxygenation stage, while the expression level of Keap1 was still about five times higher than that of Nrf-2.
Figure 6. Expression of oxygen sensor in the blood cell of largemouth bass after acute hypoxia and reoxygenation using qRT-PCR analysis. (A) HiF-1 alpha subunit (Hif-1α) expression. (B) Nuclear factor erythroid 2-related factor 2 (Nrf2) expression level. (C) The expression of Kelch-1ike ECH- associated protein l (Keap1). Different lowercase letters above each bar represent significant differences (P < 0.05).
Furthermore, compared with the control group, the expression of SOD gene decreased obviously in H1 and H2 group (P < 0.05, Figure 7A), and there was no significant difference between H1 and H2 group (P > 0.05, Figure 7A). Moreover, the SOD gene expression in R Group reached the lowest level after reoxygenation (P < 0.05, Figure 7A). Besides, the CAT expression increased slightly in H1 first in hypoxia environment, with no significant difference with the control group, however, the expression of CAT significantly upregulated in H2 group (P < 0.05, Figure 7), reaching its peak. In addition, the level of CAT gene subsequently declining in R during reoxygenation, meanwhile, its expression was significantly lower than H2 group (P < 0.05, Figure 7B), which was shown to be significantly higher than that in the control group and H1 (P < 0.05, Figure 7B). Ultimately, the expression of GPx gene increased rapidly at H1 and H2 group, which has no significant difference between them (P > 0.05, Figure 7C), and then downregulated in R group, with distinctly different from that in H1 and H2 (P < 0.05, Figure 7C). The level of GPx was significantly higher than that of control group throughout the experimental period (P < 0.05, Figure 7C). Most noteworthy, GPx expression increased significantly in H1 group (P < 0.05, Figure 7), about three times as much as that of CAT, while the expression of CAT upregulated distinctively in H2 group with its expression two times higher than that of GPx. Additionally, during hypoxia and reoxygenation stage, the expression of CAT and GPx was always higher than that of SOD, with the expression of CAT in H2 group was about 80 times higher than that of SOD as well as the expression level of GPx was about 30 times that of SOD in H2 group.
Figure 7. Effects of hypoxia and reoxygenation on antioxidant genes. (A) The expression level of Superoxide Dismutase (SOD) gene. (B) Catalase (CAT) expression level. (C) Glutathione peroxidase (GPx) expression. Different lowercase letters above each bar represent significant differences (P < 0.05).
The expression levels of Hippo pathway-related genes were examined in blood cells from each group (Figure 8). Compared with the control group, the H1 group showed almost no significant change, but the H2 group showed a clear upward trend, and then the expression of the MOB1 decreased significantly in the R group. Moreover, MOB1 peaked and remarkably increased 10 times in H2 group (P < 0.05, Figure 8B), but no significant changes in the H1/R group (P > 0.05). Similar trend to MOB1, Lats1/2 expression levels peaked in the H2 group, followed by a decline in the R group, and increased by around 3 and 2.5 times, respectively, when compared to the control group (P < 0.05, Figure 8A). Moreover, in comparison with the control group, the trend of the YAP/TAZ expression levels was also highly similar to that of the MOB1. However, it is worth noting that expression levels of the H1 group of the YAP/TAZ has increased significantly by nearly 2-fold relative to the control group, and it reached a maximum in the H2 group, where YAP/TAZ expression rose sixfold, and then dropped a bit in the R group but was still four times as high as in the control group (all P < 0.05, Figure 8C). The trend of 14-3-3 expression levels was opposite to that of YAP/TAZ, with its expression significantly decreased nearly 2-fold in H1/H2/R group and reached its lowest point in the H1 group, followed by a slight increase in the H2 group and then remained almost the same as in the R group (all P < 0.05, Figure 8D). As shown in Figure 8E, no significant difference was observed between the control group and H1 group regarding the expression of PP2A (P > 0.05), while appreciably up-regulated by around four times in H2 group and reached the top, and then had slightly down-regulated in R group (all P < 0.05). Furthermore, PP2A expression in the R group was still nearly twice that of the control group. In contrast to the control group, TEAD expression significantly decreased to the lowest point in H1/H2 group and went up in R group, but decreased by around 1 and 0.5 times, respectively, when compared to the control group (all P < 0.05, Figure 8F). The results indicated hypoxia could remarkably strengthen the expression of YAP/TAZ, a gene central to the Hippo pathway. Taken together, these data demonstrated hypoxia could effectually enhance the antioxidant response in blood cells of M. salmoides.
Figure 8. Effects of hypoxia and reoxygenation on Hippo pathway genes. (A) The expression level of large tumor suppressor homolog 1/2 (Lats1/2) gene. (B) MOB kinase activator 1 (MOB1) expression level. (C) Yes-associated protein/transcriptional co-activator with PDZ-binding motif (YAP/TAZ) expression. (D) The expression of 14-3-3. (E) The expression level of Protein Phosphatase 2A (PP2A). (F) The expression level of TEA domain (TEAD) gene. Different lowercase letters above each bar represent significant differences (P < 0.05).
A large number of studies have shown that hypoxia can induce apoptosis (Saikumar et al., 1998; Sollid et al., 2003; Sun et al., 2020d). Studies have shown that an apoptotic and cell cycle arrest cascade downstream of HIF-1α involve transcriptional initiation of several genes, such as p53, BNip3 and BAX, which ultimately drive the cells into apoptosis (Harris, 2002). In previous study, the expression of Cyt-C, Caspase-9 and Caspase-3 in the stomach and intestinal tissues of Chinese sea perch (Lateolabrax maculatus) was initially increased and then decreased after acute hypoxia, suggesting that hypoxia and reoxygenation induced apoptosis (Sun et al., 2020d). Our work reveals that hypoxic stress leads to an increase in the expression of relevant proapoptotic genes in largemouth bass, which in turn leads to apoptosis and activates inflammatory responses in blood cells, thus forcing largemouth bass to activate a series of resilience mechanisms to cope with and adapt to the adverse effects of hypoxia on its organism.
Studies have proved that hypoxia induced increase of Ca2+ in cardiac microvascular endothelial cells, which led to cell apoptosis (Zhang et al., 2016). Recent research found that elevation of intracellular Ca2+ level in human glioblastoma cells and mouse Schwann cells triggers ubiquitination of Merlin and activate Lats1,which is an important molecular in Hippo pathway (Wei et al., 2020). Our findings also indicate that hypoxia could induce Ca2+ over-produced in hypoxia-damaged blood cells, which show a consonant pattern with previous research. In other research, the mRNA expression levels of NOS did not change significantly in Carassius carassius L gills during hypoxia-induced transformation (Sollid et al., 2006). But our study showed that the level of NO fluorescence obviously affected by the amount of dissolved oxygen in the water. This suggests that there are differences in the production of NO induced by hypoxia in different tissues. Studies have shown that YAP/TAZ plays a certain role in ROS production by controlling mitochondrial respiration (White et al., 2019). Many results support the idea that hypoxia can induce ROS. Oxidative stress was caused by hypoxia stress in Przewalski’s naked carp (Gymnocypris przewalskii), and ROS production in telencephalon cells increased. It is consistent with our findings which indicate that hypoxia induces ROS over-produced in hypoxia-damaged blood cells that may induce cells apoptosis under hypoxia.
Previous studies have demonstrated that hypoxia can cause metabolic disorders, decreased immunity, and respiratory dysfunction in aquatic animals (Han et al., 2017; Peruzza et al., 2018; Sun et al., 2018). Similarly, the negative effects of hypoxia on fishes have long been studied, including metabolic disorders, decreased immunity, and apoptosis (Sun et al., 2020a; Zhao et al., 2020; Wang et al., 2022). In hypoxic responses, the Hif-1α is a master regulator of oxygen sensitivity (Wang J. et al., 2021). Our study demonstrated that the activity of Hif-1α was significantly increased in the blood cell of largemouth bass after exposure to acute hypoxic conditions, consistent with the research on Mandarinfish (Siniperca chuatsi) (He et al., 2019; Sun et al., 2020a). Our study found significant differences in the content of Hif-1α with different levels of dissolved oxygen, which suggested that the expression level of Hif-1α may be regulated by the content of dissolved oxygen. Consequently, this study expected to deliberate the relationship between Hif-1α and Nrf-2 pathway, and further revealed the relationship between Hif-1α and antioxidant mechanism. The Nrf-2 is a key component of antioxidant system that its steady state levels are very low under un-stressed conditions, as Keap1 interacts with Nrf-2 primarily via its Neh2 domain and targets it for ubiquitin mediated degradation (Yamamoto et al., 2018). However, a variety of stressors such as excessive ROS acting on Keap1’s redox-sensitive cysteine residues interfere with its inhibition of Nrf-2, allowing it to assemble, move to the nucleus, and drive ARE-mediated gene expression, which include antioxidant, detoxification and proteostasis genes (Al-Mubarak et al., 2021). Keap1 and Nrf-2 genes expression was upregulated in blood cell under hypoxia condition, showing that the expression level of antioxidant enzyme genes was apparently consistent with that of Nrf-2. Faced with the excessive production of ROS, and the possible oxidative stress caused by the reoxygenation, the body will increase some antioxidant enzymes under hypoxic conditions to prepare in advance to reduce oxidative damage (Wang M. et al., 2021). In this study, the levels of GPx and CAT increased significantly in hypoxic condition, which was consistent with the previous study in the muscle tissue of largemouth bass (Yang et al., 2017). It is worth noting that the activity of CAT in blood cells did not increase significantly when the dissolved oxygen value was 4.29 mg⋅L–1, while the expression of GPx upregulated obviously in 4.29 mg⋅L–1 of dissolved oxygen, suggesting that the mechanism of response to hypoxia injury in blood cells may be different from that in other tissues. Most noteworthy, the expression of CAT and GPx gene was higher than that of SOD gene during hypoxia stage, which suggested that SOD and CAT, GPx complement each other in hypoxia stress.
As an important pathway prevalent in living organisms, the Hippo pathway is not only a key regulator in controlling organ size and maintaining homeostasis within tissues, but recent studies have also shown that the Hippo pathway controls metabolic processes at the cellular and organismal levels (Ardestani et al., 2018). In the current study, we focused on the role of hypoxia in the regulation of Hippo pathway as well as its negative effect on blood cell of largemouth bass. Previous studies in mammals and Drosophila have established the centrality of YAP/TAZ in the Hippo pathway and found that hypoxia leads to upregulation of YAP/TAZ, which is in good agreement with our findings in blood cells of largemouth bass (Yan et al., 2014). According to the results, hypoxia significantly promoted the expression of YAP/TAZ and remained at a high level after reoxygenation, while MOB1 and Last1/2, upstream regulators of the Hippo pathway, which are highly associated with the regulation of YAP/TAZ expression, also showed a high level of expression in the H2 group. Subsequently, we also found that the expression of 14-3-3, which acts as a repressor of YAP/TAZ entry into the nucleus, was decreased in the hypoxic group, whereas the expression of PP2A, which dephosphorylates YAP/TAZ and thus enters the nucleus to bind to downstream target genes, was a greater increase. These results suggest that hypoxia is able to induce upregulation of YAP/TAZ, the core gene of the Hippo pathway, and further entry into the nucleus (Zhao et al., 2007; Mui et al., 2015). However, the expression of TEAD, which eventually binds to YAP/TAZ after nucleation, showed a dramatic decrease in the H1/H2 group. From our results, we conclude that hypoxia can cause dysregulation of the Hippo pathway and ultimately induce oxidative damage and apoptosis in largemouth bass blood cells. Similarly, results from the study of Dey et al. (2020) also suggested that dysregulated Hippo pathway and YAP/TAZ–TEAD activity is associated with various diseases, most notably cancer in human (Dey et al., 2020).
In conclusion, our findings have demonstrated that hypoxia could induce oxidative injury and apoptosis via mediating the Nrf-2/Hippo pathway in blood cells of Largemouth bass (M. salmoides) (Figure 9). These findings might provide a novel and potential therapeutic approach to understand the mechanisms in hypoxia stress and global warming adaptation.
Figure 9. Schematic model of hypoxia -induced injury via mediating the Nrf-2/Hippo pathway in blood cells of Largemouth bass (Micropterus salmoides).
The original contributions presented in the study are included in the article/supplementary material, further inquiries can be directed to the corresponding author/s.
Animal experimental procedures were conducted under protocols approved by the Regulations for Animal Experimentation of South China Normal University.
YX and LW: conceptualization. YX, ZY, YZ, and YL: methodology. YX and ZY: formal analysis and writing – original draft. JY, WZ, and YC: data curation. XL, JH, JL, YM, and LW: writing – review and editing. YZ and WZ: visualization. LW: supervision. All authors read and approved the submitted version.
This research was supported by Guangzhou Science and Technology Program projects (202102020234), Guangdong Provincial Natural Science Foundation (2017A030313194), and the Fund of Uyu (Guangzhou) Technology Company.
JL is employed by Uyu (Guangzhou) Technology Company Ltd.
The remaining authors declare that the research was conducted in the absence of any commercial or financial relationships that could be construed as a potential conflict of interest.
The authors declare that this study received funding from Uyu (Guangzhou) Technology Company Ltd. The funder was not involved in the study design, collection, analysis, interpretation of data, the writing of this article or the decision to submit it for publication.
All claims expressed in this article are solely those of the authors and do not necessarily represent those of their affiliated organizations, or those of the publisher, the editors and the reviewers. Any product that may be evaluated in this article, or claim that may be made by its manufacturer, is not guaranteed or endorsed by the publisher.
We thank Prof. Weina Wang and Prof. Anli Wang for their support in the lab space sharing.
Al-Mubarak, B. R., Bell, K. F. S., Chowdhry, S., Meakin, P. J., Baxter, P. S., McKay, S., et al. (2021). Non-canonical Keap1-independent activation of Nrf2 in astrocytes by mild oxidative stress. Redox Biol. 47:102158. doi: 10.1016/j.redox.2021.102158
Ardestani, A., Lupse, B., and Maedler, K. (2018). Hippo Signaling: key Emerging Pathway in Cellular and Whole-Body Metabolism. Trends Endocrinol. Metab. 29, 492–509. doi: 10.1016/j.tem.2018.04.006
Azimi, I., Petersen, R. M., Thompson, E. W., Roberts-Thomson, S. J., and Monteith, G. R. (2017). Hypoxia-induced reactive oxygen species mediate N-cadherin and SERPINE1 expression, EGFR signalling and motility in MDA-MB-468 breast cancer cells. Sci. Rep. 7:15140. doi: 10.1038/s41598-017-15474-7
Barrera, G., Cucci, M. A., Grattarola, M., and Pizzimenti, S. (2021). “Nrf2, YAP, antioxidant potential, and cancer,” in Cancer(Second Edition), eds V. R. Preedy and V. B. Patel (Cambridge, Massachusetts: Academic Press), 159–170. doi: 10.1016/B978-0-12-819547-5.00015-8
Bellezza, I., Giambanco, I., Minelli, A., and Donato, R. (2018). Nrf2-Keap1 signaling in oxidative and reductive stress. Biochim. Biophys. Acta Mol. Cell Res. 1865, 721–733. doi: 10.1016/j.bbamcr.2018.02.010
Borowiec, B. G., and Scott, G. R. (2020). Hypoxia acclimation alters reactive oxygen species homeostasis and oxidative status in estuarine killifish (Fundulus heteroclitus). J. Exp. Biol. 223:jeb222877. doi: 10.1242/jeb.222877
Breitburg, D., Levin, L. A., Oschlies, A., Gregoire, M., Chavez, F. P., Conley, D. J., et al. (2018). Declining oxygen in the global ocean and coastal waters. Science 359:eaam7240. doi: 10.1126/science.aam7240
Brüne, B. (2003). Nitric oxide: NO apoptosis or turning it ON? Cell Death Differ. 10, 864–869. doi: 10.1038/sj.cdd.4401261
Cai, X., Zhou, Z., Zhu, J., Liao, Q., Zhang, D., Liu, X., et al. (2020). Zebrafish Hif3α modulates erythropoiesis via regulation of gata1 to facilitate hypoxia tolerance. Development 147:dev185116. doi: 10.1242/dev.185116
Cao, L., Huang, W., Shan, X., Ye, Z., and Dou, S. (2012). Tissue-specific accumulation of cadmium and its effects on antioxidative responses in Japanese flounder juveniles. Environ. Toxicol. Pharmacol. 33, 16–25. doi: 10.1016/j.etap.2011.10.003
Cao, W., Chen, Y., Wu, Y., and Zhu, S. (1981). “Origin and evolution of schizothoracine fishes in relation to the upheaval of the Xizang Plateau,” in Studies on the Period, Amplitude and Type of the Uplift of the Qinghai-Xizang Plateau, ed. Tibetan Expedition Team of the Chinese Academy of Science (Beijing: Science Press), 118–130.
Crans, K. D., Pranckevicius, N. A., and Scott, G. R. (2015). Physiological tradeoffs may underlie the evolution of hypoxia tolerance and exercise performance in sunfish (Centrarchidae). J. Exp. Biol. 218, 3264–3275. doi: 10.1242/jeb.124602
Curtin, J. F., Donovan, M., and Cotter, T. G. (2002). Regulation and measurement of oxidative stress in apoptosis. J. Immunol. Methods 265, 49–72. doi: 10.1016/s0022-1759(02)00070-4
D’Arcy, M. S. (2019). Cell death: a review of the major forms of apoptosis, necrosis and autophagy. Cell Biol. Int. 43, 582–592. doi: 10.1002/cbin.11137
Dey, A., Varelas, X., and Guan, K. L. (2020). Targeting the Hippo pathway in cancer, fibrosis, wound healing and regenerative medicine. Nat. Rev. Drug Discov. 19, 480–494. doi: 10.1038/s41573-020-0070-z
Dichiera, A. M., McMillan, O. J. L., Clifford, A. M., Goss, G. G., Brauner, C. J., and Esbaugh, A. J. (2020). The importance of a single amino acid substitution in reduced red blood cell carbonic anhydrase function of early-diverging fish. J. Comp. Physiol. B 190, 287–296. doi: 10.1007/s00360-020-01270-9
Dimmeler, S., and Zeiher, A. M. (1997). Nitric oxide and apoptosis: another paradigm for the double-edged role of nitric oxide. Nitric Oxide 1, 275–281. doi: 10.1006/niox.1997.0133
Erzurum, S. C., Ghosh, S., Janocha, A. J., Xu, W., Bauer, S., Bryan, N. S., et al. (2007). Higher blood flow and circulating NO products offset high-altitude hypoxia among Tibetans. Proc. Natl. Acad. Sci. U. S. A. 104, 17593–17598. doi: 10.1073/pnas.0707462104
Gaulke, G. L., Dennis, C. E. III, Wahl, D. H., and Suski, C. D. (2014). Acclimation to a low oxygen environment alters the hematology of largemouth bass (Micropterus salmoides). Fish Physiol. Biochem. 40, 129–140. doi: 10.1007/s10695-013-9830-6
Han, S. Y., Wang, B. J., Liu, M., Wang, M. Q., Jiang, K. Y., Qi, C. C., et al. (2017). Effect of cyclic serious/medium hypoxia stress on the survival, growth performance and resistance against Vibrio parahemolyticus of white shrimp Litopenaeus vannamei. ISJ-Invert. Surviv. J. 14, 259–270. doi: 10.25431/1824-307X/isj.v14i1.259-270
Hao, Y., Chun, A., Cheung, K., Rashidi, B., and Yang, X. (2008). Tumor suppressor LATS1 is a negative regulator of oncogene YAP. J. Biol. Chem. 283, 5496–5509. doi: 10.1074/jbc.M709037200
Harris, A. L. (2002). Hypoxia–a key regulatory factor in tumour growth. Nat. Rev. Cancer 2, 38–47. doi: 10.1038/nrc704
He, J., Yu, Y., Qin, X. W., Zeng, R. Y., Wang, Y. Y., Li, Z. M., et al. (2019). Identification and functional analysis of the Mandarin fish (Siniperca chuatsi) hypoxia-inducible factor-1alpha involved in the immune response. Fish Shellfish Immunol. 92, 141–150. doi: 10.1016/j.fsi.2019.04.298
Hong, A. W., Meng, Z., Plouffe, S. W., Lin, Z., Zhang, M., and Guan, K. L. (2020). Critical roles of phosphoinositides and NF2 in Hippo pathway regulation. Genes Dev. 34, 511–525. doi: 10.1101/gad.333435.119
Huang, Y., He, N., Kang, Q., Shen, D., Wang, X., Wang, Y., et al. (2019). A carbon dot-based fluorescent nanoprobe for the associated detection of iron ions and the determination of the fluctuation of ascorbic acid induced by hypoxia in cells and in vivo. Analyst 144, 6609–6616. doi: 10.1039/c9an01694e
Jensen, F. B. (2007). Nitric oxide formation from nitrite in zebrafish. J. Exp. Biol. 210, 3387–3394. doi: 10.1242/jeb.008748
Jls, A., Llz, A., Lei, L. A., Xht, A., Cc, A., Qiao, L. A., et al. (2020). Interactive effect of thermal and hypoxia on largemouth bass (Micropterus salmoides) gill and liver: aggravation of oxidative stress, inhibition of immunity and promotion of cell apoptosis. Fish Shellfish Immunol. 98, 923–936. doi: 10.1016/j.fsi.2019.11.056
Kumar, A., Singh, K. P., Bali, P., Anwar, S., Kaul, A., Singh, O. P., et al. (2018). iNOS polymorphism modulates iNOS/NO expression via impaired antioxidant and ROS content in P. vivax and P. falciparum infection. Redox Biol. 15, 192–206. doi: 10.1016/j.redox.2017.12.005
Lehmann, W., Mossmann, D., Kleemann, J., Mock, K., Meisinger, C., Brummer, T., et al. (2016). ZEB1 turns into a transcriptional activator by interacting with YAP1 in aggressive cancer types. Nat. Commun. 7:10498. doi: 10.1038/ncomms10498
Lei, Q. Y., Zhang, H., Zhao, B., Zha, Z. Y., Bai, F., Pei, X. H., et al. (2008). TAZ promotes cell proliferation and epithelial-mesenchymal transition and is inhibited by the hippo pathway. Mol. Cell. Biol. 28, 2426–2436. doi: 10.1128/MCB.01874-07
Levin, L. A., and Breitburg, D. L. (2015). Linking coasts and seas to address ocean deoxygenation. Nat. Clim. Chang. 5, 401–403. doi: 10.1038/nclimate2595
Li, R., Wen, Z. Y., Zou, Y. C., Qin, C. J., and Yuan, D. Y. (2017). Largemouth Bass Pond Culture in China: a Review. Int. J. Vet. Sci. Res. 3, 014–017. doi: 10.17352/ijvsr.000016
Luo, S. W., Kang, H., Kong, J. R., Xie, R. C., Liu, Y., Wang, W. N., et al. (2017). Molecular cloning, characterization and expression analysis of (B-cell lymphoma-2) Bcl-2 in the orange-spotted grouper (Epinephelus coioides) after the Vibrio alginolyticus challenge. Dev. Comp. Immunol. 76, 150–162. doi: 10.1016/j.dci.2017.06.003
Ma, S., Meng, Z., Chen, R., and Guan, K. L. (2019). The Hippo Pathway: biology and Pathophysiology. Annu. Rev. Biochem. 88, 577–604. doi: 10.1146/annurev-biochem-013118-111829
Meng, Z., Moroishi, T., and Guan, K. L. (2016). Mechanisms of Hippo pathway regulation. Genes Dev. 30, 1–17. doi: 10.1101/gad.274027.115
Mondal, N. K., Saha, H., Mukherjee, B., Tyagi, N., and Ray, M. R. (2018). Inflammation, oxidative stress, and higher expression levels of Nrf2 and NQO1 proteins in the airways of women chronically exposed to biomass fuel smoke. Mol. Cell. Biochem. 447, 63–76. doi: 10.1007/s11010-018-3293-0
Motohashi, H., O”Connor, T., Katsuoka, F., Engel, J. D., and Yamamoto, M. (2002). Integration and diversity of the regulatory network composed of Maf and CNC families of transcription factors. Gene 294, 1–12. doi: 10.1016/S0378-1119(02)00788-6
Mui, M. Z., Zhou, Y., Blanchette, P., Chughtai, N., Knight, J. F., Gruosso, T., et al. (2015). The Human Adenovirus Type 5 E4orf4 Protein Targets Two Phosphatase Regulators of the Hippo Signaling Pathway. J. Virol. 89, 8855–8870. doi: 10.1128/JVI.03710-14
Oka, T., Mazack, V., and Sudol, M. (2008). Mst2 and Lats kinases regulate apoptotic function of Yes kinase-associated protein (YAP). J. Biol. Chem. 283, 27534–27546. doi: 10.1074/jbc.M804380200
Peruzza, L., Gerdol, M., Oliphant, A., Wilcockson, D., Pallavicini, A., Hawkins, L., et al. (2018). The consequences of daily cyclic hypoxia on a European grass shrimp: from short-term responses to long-term effects. Funct. Ecol. 32, 2333–2344. doi: 10.1111/1365-2435.13150
Saikumar, P., Dong, Z., Weinberg, J. M., and Venkatachalam, M. A. (1998). Mechanisms of cell death in hypoxia/reoxygenation injury. Oncogene 17, 3341–3349. doi: 10.1038/sj.onc.1202579
Sollid, J., De Angelis, P., Gundersen, K., and Nilsson, G. E. (2003). Hypoxia induces adaptive and reversible gross morphological changes in crucian carp gills. J. Exp. Biol. 206, 3667–3673. doi: 10.1242/jeb.00594
Sollid, J., Rissanen, E., Tranberg, H. K., Thorstensen, T., Vuori, K. A. M., Nikinmaa, M., et al. (2006). HIF-1α and iNOS levels in crucian carp gills during hypoxia-induced transformation. J. Comp. Physiol. B 176, 359–369. doi: 10.1007/s00360-005-0059-2
Sun, J. L., Zhao, L. L., Wu, H., Liu, Q., Liao, L., Luo, J., et al. (2020c). Acute hypoxia changes the mode of glucose and lipid utilization in the liver of the largemouth bass (Micropterus salmoides). Sci. Total Environ. 713:135157. doi: 10.1016/j.scitotenv.2019.135157
Sun, J. L., Zhao, L. L., He, K., Liu, Q., Luo, J., Zhang, D. M., et al. (2020b). MicroRNA regulation in hypoxic environments: differential expression of microRNAs in the liver of largemouth bass (Micropterus salmoides). Fish Physiol. Biochem. 46, 2227–2242. doi: 10.1007/s10695-020-00877-7
Sun, Y., Dong, H., Zhan, A., Wang, W., Duan, Y., Xie, M., et al. (2020d). Protection of teprenone against hypoxia and reoxygenation stress in stomach and intestine of Lateolabrax maculatus. Fish Physiol. Biochem. 46, 575–584. doi: 10.1007/s10695-019-00732-4
Sun, J. L., He, K., Liu, Q., Luo, J., Wang, Y., Zhang, D. M., et al. (2020a). Inhibition of fatty acid oxidation induced by up-regulation of miR-124 and miR-205 during exposure of largemouth bass (Micropterus salmoides) to acute hypoxia. Aquaculture 529:10. doi: 10.1016/j.aquaculture.2020.735679
Sun, S. M., Guo, Z. B., Fu, H. T., Ge, X. P., Zhu, J., and Gu, Z. M. (2018). Based on the Metabolomic Approach the Energy Metabolism Responses of Oriental River Prawn Macrobrachium nipponense Hepatopancreas to Acute Hypoxia and Reoxygenation. Front. Physiol. 9:76. doi: 10.3389/fphys.2018.00076
Tonelli, C., Chio, I. I. C., and Tuveson, D. A. (2018). Transcriptional Regulation by Nrf2. Antioxid Redox Signal. 29, 1727–1745. doi: 10.1089/ars.2017.7342
Vagner, M., Zambonino-Infante, J. L., and Mazurais, D. (2019). Fish facing global change: are early stages the lifeline? Mar. Environ. Res. 147, 159–178. doi: 10.1016/j.marenvres.2019.04.005
Wang, J., Xu, Z., and He, J. (2021). The role of HIF-1α in the energy metabolism and immune responses of hypoxic Scylla paramamosain. Aquac. Rep. 20:100740. doi: 10.1016/j.aqrep.2021.100740
Wang, M., Wu, F., Xie, S., and Zhang, L. (2021). Acute hypoxia and reoxygenation: effect on oxidative stress and hypoxia signal transduction in the juvenile yellow catfish (Pelteobagrus fulvidraco). Aquaculture 531:735903. doi: 10.1016/j.aquaculture.2020.735903
Wang, L., Wang, X. R., Liu, J., Chen, C. X., Liu, Y., and Wang, W. N. (2015). Rab from the white shrimp Litopenaeus vannamei: characterization and its regulation upon environmental stress. Ecotoxicology 24, 1765–1774. doi: 10.1007/s10646-015-1481-1
Wang, L., Wu, J., Wang, W. N., Cai, D. X., Liu, Y., and Wang, A. L. (2012). Glutathione peroxidase from the white shrimp Litopenaeus vannamei: characterization and its regulation upon pH and Cd exposure. Ecotoxicology 21, 1585–1592. doi: 10.1007/s10646-012-0942-z
Wang, M., Li, B., Wang, J., Xie, S., and Zhang, L. (2022). Skin transcriptome and physiological analyses reveal the metabolic and immune responses of yellow catfish (Pelteobagrus fulvidraco) to acute hypoxia. Aquaculture 546:737277. doi: 10.1016/j.aquaculture.2021.737277
Wei, Y., Yee, P. P., Liu, Z., Zhang, L., Guo, H., Zheng, H., et al. (2020). NEDD4L-mediated Merlin ubiquitination facilitates Hippo pathway activation. EMBO Rep. 21:e50642. doi: 10.15252/embr.202050642
White, S. M., Avantaggiati, M. L., Nemazanyy, I., Di Poto, C., Yang, Y., Pende, M., et al. (2019). YAP/TAZ Inhibition Induces Metabolic and Signaling Rewiring Resulting in Targetable Vulnerabilities in NF2-Deficient Tumor Cells. Dev. Cell 49, 425–443.e9. doi: 10.1016/j.devcel.2019.04.014
Yamamoto, M., Kensler, T. W., and Motohashi, H. (2018). The KEAP1-NRF2 System: a Thiol-Based Sensor-Effector Apparatus for Maintaining Redox Homeostasis. Physiol. Rev. 98, 1169–1203. doi: 10.1152/physrev.00023.2017
Yan, L., Cai, Q., and Xu, Y. (2014). Hypoxic conditions differentially regulate TAZ and YAP in cancer cells. Arch. Biochem. Biophys. 562, 31–36. doi: 10.1016/j.abb.2014.07.024
Yang, S., Yan, T., Wu, H., Xiao, Q., Fu, H. M., Luo, J., et al. (2017). Acute hypoxic stress: effect on blood parameters, antioxidant enzymes, and expression of HIF-1alpha and GLUT-1 genes in largemouth bass (Micropterus salmoides). Fish Shellfish Immunol. 67, 449–458. doi: 10.1016/j.fsi.2017.06.035
Yu, L. L., Yu, H. H., Liang, X. F., Li, N., Wang, X., Li, F. H., et al. (2018). Dietary butylated hydroxytoluene improves lipid metabolism, antioxidant and anti-apoptotic response of largemouth bass (Micropterus salmoides). Fish Shellfish Immunol. 72, 220–229. doi: 10.1016/j.fsi.2017.10.054
Zhang, H., Liu, C. Y., Zha, Z. Y., Zhao, B., Yao, J., Zhao, S., et al. (2009). TEAD transcription factors mediate the function of TAZ in cell growth and epithelial-mesenchymal transition. J. Biol. Chem. 284, 13355–13362. doi: 10.1074/jbc.M900843200
Zhang, Y., Zhou, H., Wu, W., Shi, C., Hu, S., Yin, T., et al. (2016). Liraglutide protects cardiac microvascular endothelial cells against hypoxia/reoxygenation injury through the suppression of the SR-Ca(2+)-XO-ROS axis via activation of the GLP-1R/PI3K/Akt/survivin pathways. Free Radic. Biol. Med. 95, 278–292. doi: 10.1016/j.freeradbiomed.2016.03.035
Zhao, B., Wei, X., Li, W., Udan, R. S., Yang, Q., Kim, J., et al. (2007). Inactivation of YAP oncoprotein by the Hippo pathway is involved in cell contact inhibition and tissue growth control. Genes Dev. 21, 2747–2761. doi: 10.1101/gad.1602907
Zhao, B., Ye, X., Yu, J., Li, L., Li, W., Li, S., et al. (2008). TEAD mediates YAP-dependent gene induction and growth control. Genes Dev. 22, 1962–1971. doi: 10.1101/gad.1664408
Zhao, L., Cui, C., Liu, Q., Sun, J., He, K., Adam, A. A., et al. (2020). Combined exposure to hypoxia and ammonia aggravated biological effects on glucose metabolism, oxidative stress, inflammation and apoptosis in largemouth bass (Micropterus salmoides). Aquat. Toxicol. 224:105514. doi: 10.1016/j.aquatox.2020.105514
Keywords: climate warming, hypoxia, Micropterus salmoides, blood cells, Nrf2/Hippo pathway
Citation: Xin Y, Yang Z, Zhu Y, Li Y, Yu J, Zhong W, Chen Y, Lv X, Hu J, Lin J, Miao Y and Wang L (2022) Hypoxia Induces Oxidative Injury and Apoptosis via Mediating the Nrf-2/Hippo Pathway in Blood Cells of Largemouth Bass (Micropterus salmoides). Front. Ecol. Evol. 10:841318. doi: 10.3389/fevo.2022.841318
Received: 22 December 2021; Accepted: 21 January 2022;
Published: 16 March 2022.
Edited by:
Lin Zhang, Hubei University of Chinese Medicine, ChinaReviewed by:
Zhou Meng, Zhongkai University of Agriculture and Engineering, ChinaCopyright © 2022 Xin, Yang, Zhu, Li, Yu, Zhong, Chen, Lv, Hu, Lin, Miao and Wang. This is an open-access article distributed under the terms of the Creative Commons Attribution License (CC BY). The use, distribution or reproduction in other forums is permitted, provided the original author(s) and the copyright owner(s) are credited and that the original publication in this journal is cited, in accordance with accepted academic practice. No use, distribution or reproduction is permitted which does not comply with these terms.
*Correspondence: Lei Wang, d2FuZ2xlaUBzY251LmVkdS5jbg==
†These authors have contributed equally to this work
Disclaimer: All claims expressed in this article are solely those of the authors and do not necessarily represent those of their affiliated organizations, or those of the publisher, the editors and the reviewers. Any product that may be evaluated in this article or claim that may be made by its manufacturer is not guaranteed or endorsed by the publisher.
Research integrity at Frontiers
Learn more about the work of our research integrity team to safeguard the quality of each article we publish.