- 1School of Forestry, Northern Arizona University, Flagstaff, AZ, United States
- 2MPG Ranch, Missoula, MT, United States
The soil and its biota can shape the development of colonizing vascular plant communities. Because they occupy soil surfaces where most seeds disperse to, biological soil crusts (biocrusts) are uniquely positioned to influence vascular plant communities established by direct seeding, e.g., for restoration. We created mesocosms of soil overtopped by intact biocrust transplants from the field, varying in key community attributes: total cover, species richness, and proportional cover of mosses relative to lichens. We seeded the same diverse mixture of vascular plants into all mesocosms, including desired native species and problematic exotic invasive species. We tracked plant community development for two full growing seasons, both under ambient outdoor conditions and with supplemental irrigation to remove the influence of water limitation. Under ambient conditions, we found that total biocrust cover suppressed exotic plant emergence and biocrust richness slightly promoted native emergence (r = −0.23 to −0.39) but had weaker and less consistent effects on cover of either native or exotic plants (r ≤ |0.25|). Early emergence events were generally strong drivers of vascular plant recruitment (r = 0.17–0.78) and continued to influence community composition after 2 years, suggesting a priority effect. Biocrust cover also promoted final plant biomass under ambient conditions (r = 0.17–0.33) but did not influence the total cumulative number of native species (r ≤ |0.07|) nor the fecundity of exotics (r ≤ |0.08|). Biocrusts’ influence on total vascular plant biomass was minor. When water was added, biocrust effects sometimes switched from positive or negative to neutral, or vice-versa, indicating that our detection probability of biocrust effects on plants changes with moisture availability. Our results demonstrate that the condition of pre-existing biocrust communities can influence—but not strongly dictate—the outcome of multi-species restoration seedings, mostly positively or neutrally under normal conditions, but switching to potentially negatively under irrigated conditions. Our study also suggests that locations with more intact and richer biocrust communities might be slightly more conducive to successful seeding outcomes, while also providing additional contributions to ecosystem functions. As such, biocrusts, alongside vascular plants, have a role in restoring damaged or degraded ecosystems.
Introduction
Above-belowground interactions often shape the development of plant communities (Klironomos, 2001; Wubs et al., 2019). Experimental manipulations of soil community composition and diversity have induced responses in plant diversity (van der Heijden et al., 1998), plant-insect interactions (Heinen et al., 2018), productivity, function (Bender and van der Heijden, 2015), and invasibility (Liao et al., 2015). For example, increased mycorrhizal species richness positively affected plant community richness and productivity (van der Heijden et al., 1998). These above-belowground interactions occur because soil communities contain organisms that may affect plants directly in either negative (enemies, e.g., herbivores, pathogens, resource competitors) or positive ways (symbionts and facilitators, e.g., resource trading partners, nitrogen fixers), and indirectly through effects on the soil environment (decomposers and ecosystem engineers) (Wardle et al., 2004). Natural variability in the prevalence of these interactions lead to the general expectation that variation in a soil community should induce variation in a plant community. Biological soil crusts (biocrusts) are a soil community known to influence plant success at many life history stages including establishment, growth, and reproduction (Slate et al., 2018; Havrilla et al., 2019). Despite the body of literature that investigates how individuals or populations of a single species interact with one or more types of biocrust, there is a shortage of studies of how community characteristics—abundance, diversity, composition—of biocrusts influence the characteristics and trajectory of a developing plant community from emergence onward (but see Luzuriaga et al., 2012).
Biocrusts aggregate and occupy soil surfaces and are dominated by non-vascular primary producers such as cyanobacteria, algae, mosses, liverworts, and lichens, in various combinations (Belnap et al., 2003a). Biocrusts occur in habitats in which plant productivity is sufficiently low that light reaches the soil surface, including drylands (Rodriguez-Caballero et al., 2018), cold-limited environments (Briegel-Williams et al., 2017), and early successional environments (Grover et al., 2020), among others (Corbin and Thiet, 2020). Thus, in many different ecosystems around the globe, biocrusts determine the nature of the above-belowground boundary and modulate the flux of energy and materials into and out of the soil (Belnap et al., 2003b). Biocrusts are often but not always a germination barrier for plant seeds, though the strength of this effect is contingent both on biocrust characteristics and plant traits (Zhang and Belnap, 2015; Havrilla et al., 2019). Biocrusts function as ecosystem engineers because they influence other organisms’ access to resources through their physical modification of the environment (Jones et al., 1994). For example, they have a pervasive influence on soil water and hydrological processes (Eldridge et al., 2020), and thus could substantially influence water availability to plants. Further, biocrusts harbor N-fixers and accumulate fertility (Ferrenberg et al., 2018; Torres-Cruz et al., 2018) and have been shown to influence plant nutrition (Harper and Belnap, 2001). Therefore, there are numerous ways in which biocrust can directly interact with vascular plants or modify their habitats.
We chose semi-arid steppes as our focal ecosystem because they support diverse biocrust communities dominated by mosses and lichens, with the potential for high coverage (Ponzetti and McCune, 2001). In between vascular plant canopies, the soil surface is often very heterogeneous at small scales (sub-meter) and may range from 0 to nearly 100% in biocrust cover, from 0 to >20 in species richness, and may display strongly differing species compositions (Durham et al., 2018). Our specific study area, MPG Ranch, Missoula, MT, United States, is engaged in active vascular plant restoration activities including exotic plant suppression and seeding of desired grasses and forbs. Local management goals are oriented around (1) promoting a high degree of plant productivity and (2) promoting the success of native plants over exotic ones. Thus, we adopted these broad outcomes of plant community conditions and tracked their specific responses to biocrusts via emergence and cover.
We sought to broaden knowledge on the effect of biocrusts on plant community development, in the context of multi-species seeding for restoration purposes, by seeding a standard set of thirteen common species over soil surfaces spanning gradients of biocrust development and composition. Our soil surfaces intentionally varied in total cover and species richness of biocrusts, and in the dominance of mosses relative to lichens, to induce strong variation in the ways in which the biocrusts might influence the vascular plant community (e.g., modification of soil water and fertility, and presenting differently permeable germination barriers, among other differences). Our plant species included problematic exotic species, often present in restoration sites, and desired native species actively seeded by land managers. Further, we did so under both ambient and enhanced soil moisture conditions to determine the environmental contingency of our findings. We tested the following hypotheses:
1. Biocrust condition influences exotic and native plant emergence differently. Specifically, we expected a prevailing negative influence of total biocrust cover on exotic plant emergence and a lesser neutral to negative influence on native plant emergence, as observed in recent literature (Slate et al., 2018; Havrilla et al., 2019).
2. Biocrust condition influences exotic and native plant cover similarly. Specifically, we expected that biocrust conditions associated with greater soil resource availability, such as higher cover and species richness (Bowker et al., 2013), would promote cover of plants whether they are native or exotic.
3. Greater abundance of biocrusts will promote native plant biomass and cumulative richness of native species, and lower exotic plant fecundity in the final community. Native plants share an evolutionary history with biocrusts and may be more likely to have adaptive traits that allow them to succeed in biocrusted habitats.
4. Biocrust condition will not influence overall biomass production. Because we seeded several plant species simultaneously, we expected all possible biocrust conditions to be favorable to at least some of the plant species because of variability in niche requirements among the focal plants, dampening variability in final productivity. This is supported by a meta-analytical finding of no overall effect of biocrusts on many plant species across various life stages (Havrilla et al., 2019).
5. Biocrust effects on plants are contingent on water availability. We expected the overall magnitude of biocrusts effects to shift when water was abundant but considered either stronger or weaker effects plausible on a priori grounds.
In testing these hypotheses, we apply a novel community-scale view treating both biocrusts and vascular plant communities as complex and highly variable entities.
Materials and Methods
Study Site
Our research was conducted on the 3,800-ha property of MPG Ranch in the Bitterroot Valley of Montana. After a history of livestock grazing, logging, and farming of some portions, this property has been managed as a conservation ranch since 2009 and supports an active ecological restoration program. Ecosystems range from forests to open grasslands and shrub steppes and post-agricultural communities. The mean annual temperature across the ranch is 7.6°C, and annual precipitation is 327 mm (modeled 30-year normals; Hijmans et al., 2005) most of it falling as rain and snow during cold winters. Biocrusts are present throughout the ranch, in some areas covering the entirety of the interspaces among vascular plants (Durham et al., 2018). Biocrusts of the study area may be moss or lichen-dominated and collectively harbor over 90 moss and lichen species.
Mesocosm Construction and Experimental Design Overview
In May 2016, we installed 120 mesocosms in an experimental enclosure at MPG Ranch (46.667°N, −114.007°W, 998 m) that was fenced to exclude herbivores and regularly weeded. Each mesocosm consisted of a 2.31 L (10.2 cm × 10.2 cm × 30.5 cm) tree pot, backfilled with 1.8 L of native soil obtained from an on-site quarry in May 2016. The native soil was a sandy loam with pH = 5.59. We collected intact soil monoliths, sampling strata that captured a breadth of biocrust development and composition (see section “Biocrust Collection” below) and installed these on top of the backfilled soil in each pot (Figure 1). Minimizing soil disturbance, we weeded all vascular plants present in the monoliths. The soil surface under the mesocosm pots remained covered with synthetic weed cloth over the entire course of our experiment to minimize nearby seed sources. We installed drip irrigation to a random selection of 60 mesocosms, but we did not immediately begin irrigation because of persistent frost danger. Instead, we left the mesocosms to acclimate during the summer and early fall preceding seeding to allow some germination of the extant seed bank in the soil monoliths and to delay our seeding until a more favorable plant establishment period.
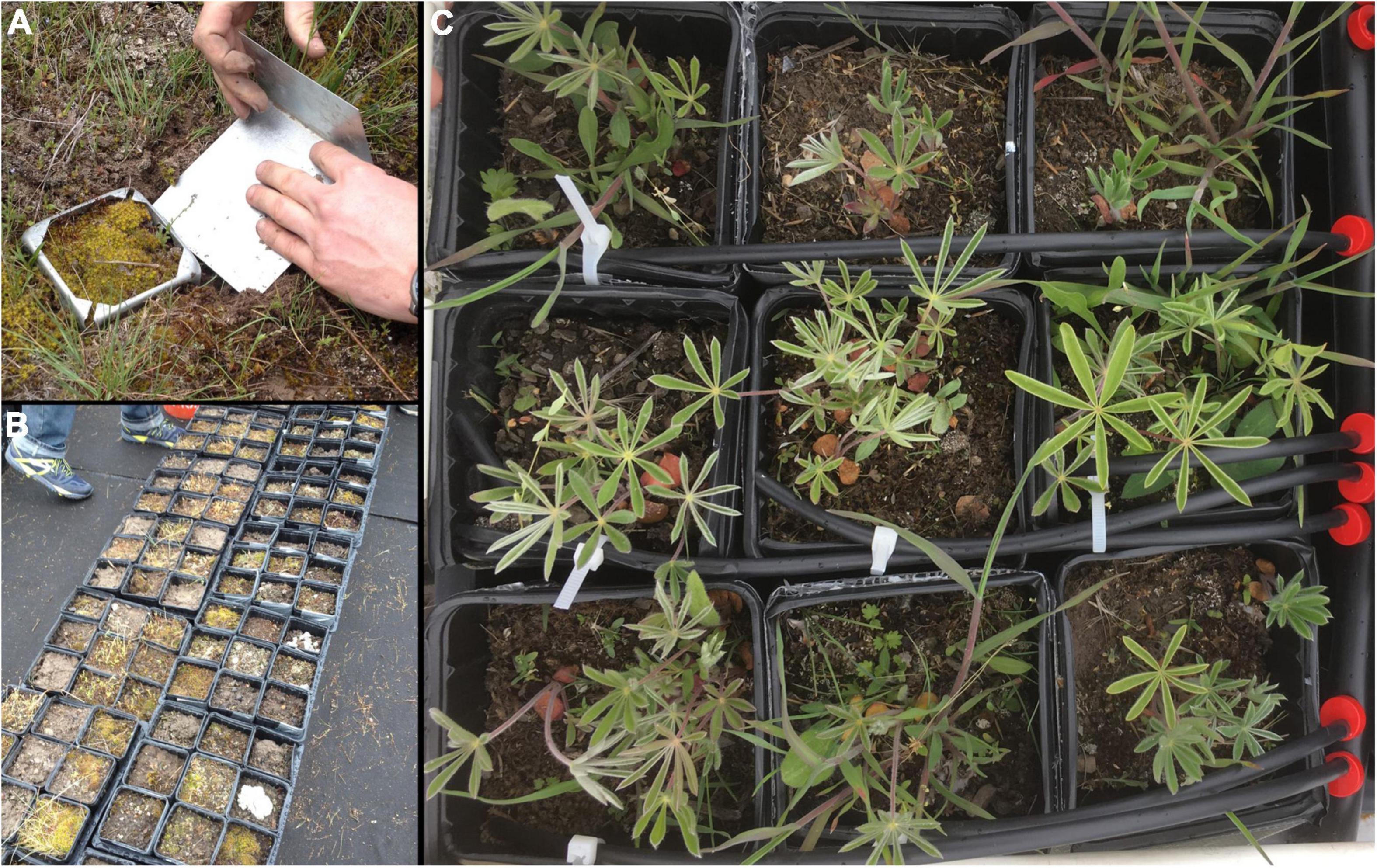
Figure 1. Experimental mesocosms. (A) Collection of intact biocrusts from the field. (B) Biocrusts were placed on top of backfilled tree pots. The variety of cover values and compositions is evident in this view, prior to both weeding and seeding. (C) Developing and variable vascular plant communities in a subset of pots.
In October of 2016, we again weeded all new germinants, either by clipping aboveground biomass or whole plant removal if it could be done without disturbing the surface. Then we seeded a common mixture of 13 native and exotic herbaceous vascular plants to each mesocosm (see section “Plant Materials and Seeding,” below). Seed was added by dispersal from a microcentrifuge tube about 5 cm above the center of each mesocosm, without burial. Before leaving the microcosms to overwinter, we measured the percentage cover of biocrusts to the highest taxonomic resolution possible without disturbing the mesocosms (species level for most taxa). Using this data, we quantified three key biocrust properties: total cover, species richness and proportional moss cover. Proportional moss cover measures moss dominance relative to lichens, is simply the cover of mosses divided by the total cover of biocrust; this was important to include because mosses and lichens have been shown to exert distinct effects on vascular plants (Sedia and Ehrenfeld, 2003; Havrilla et al., 2019).
In March – September of both 2017 and 2018, we monitored vascular plant emergence events, plants’ growth, and plant communities’ development monthly (see section “Plant Monitoring” below). We weeded non-target plants in March and April of 2017 but did not find it necessary afterward as target plants established well. Irrigation was initiated in May of both years and continued through September, delivering 250 mL of water to each irrigated mesocosm at 6:00 am, every other day. Irrigation was not meant to simulate a particular climate scenario but rather to diminish water limitation as an abiotic filter. Irrigation is not generally a realistic method that could be used to boost seeding success, instead it shows us whether our results are environmentally contingent.
During the two growing seasons, we collected seeds every 2 weeks once they began to cure. Seeds collected in the first growing season were returned to their mesocosm of origin after air-drying and weighing; those collected in the second growing season were compiled gradually for weighing and not returned to the mesocosms. We terminated the experiment in September 2018 with a final biomass harvest of vascular plants.
Biocrust Collection
To generate a wide array of surface conditions in our mesocosms we collected intact monoliths of surface soil varying in biocrust development and composition in May 2016. Collections were made from interspaces in an Artemisia tridentata and Poa secunda steppe (46.667°N, −114.009°W, 998 m, 1,220 m). Soil monoliths were collected by moistening soil, pressing a metal frame (a modified circuit box, 10.2 cm × 10.2 cm × 2.5 cm) into the soil, cutting around the edges with a serrated blade, and carefully lifting the box while supporting the soil underneath with a metal sheet. The soil was immediately placed on top of a mesocosm, previously backfilled with soil, keeping the monoliths as intact as possible.
We used a stratified approach to ensure a wide variety of different soil surfaces. We stratified by total biocrust cover in the following percentage ranges: (0 –<20), (20 –<40), (40 –<60), (60 –<80), and (80–100). Within each cover bracket, we selected four monoliths each with very high, high, medium, low, and very low proportional cover of mosses relative to lichens in the biocrust, resulting in 120 total collections. Although biocrust species richness was not formally part of our sampling design, we intentionally favored sampling of different levels of richness within each combination of sampling brackets, leading also to a strong richness gradient across our samples.
Plant Materials and Seeding
We seeded Achnatherum hymenoides (native grass), Festuca campestris (native grass), Hesperostipa comata (native grass), Koeleria macrantha (native grass), Antennaria parvifolia (native forb), Balsamorhiza sagittata (native forb), Gaillardia aristata (native forb), Lupinus sericeus (native forb), Phacelia hastata (native forb), Bromus tectorum (exotic grass), Centaurea stoebe (exotic forb), Euphorbia esula (exotic forb), and Potentilla recta (exotic forb). We selected this group of species to include grasses and forbs used in ecological restoration and diversification projects at MPG Ranch, and the most problematic exotic invasive species, including a grass and three forbs. Seeds were obtained from local seed collections with two exceptions: (1) local L. sericeus was supplemented with a regional commercial source of unknown provenance, and (2) we used an United States Natural Resources Conservation Service cultivar of Achnatherum hymenoides, because of insufficient local material at the time of collection.
Our seeding strategy was to include sufficient seed to result in an expected two individuals per mesocosm. This number of individuals was selected because such a high total number of individuals would ensure species interactions, and small populations would make competitive exclusion possible. The total density of plants is higher than would be observed naturally among established plants but might reflect a high rate of seed addition in a restoration project. To estimate the seed mass needed per species, we used a combination of published germination rates and germination trials (see Doherty, 2019).
Plant Monitoring
Monthly, we measured the number of individuals per pot (reflective of germination, emergence, and early survivorship; hereafter termed emergence) and obtained a visual estimate of canopy cover, all to the species level. Cover estimation continued through both growing seasons but counts of individuals were discontinued in 2018 as it became increasingly difficult due to dense growth. Both emergence and cover data were summed for two groups of plants, natives and exotics, to test the hypotheses advanced here. We used cumulative seed mass as a measure of fecundity per species, per pot. We note that seed predation was an unquantified influence on measured fecundity for some species, including C. stoebe which is subject to predation by insects introduced as biocontrol agents in the area. We summed the dry mass of all biweekly-collected seeds for each species in each mesocosm among those that produced seed.
Finally, we harvested final plant biomass. We harvested senescent aboveground biomass for Bromus tectorum after the first growing season in September 2017, because it is an annual and the biomass was not expected to persist until the end of the experiment. All other biomass harvests occurred the following year in September 2018. All aboveground biomass was clipped at the ground surface and sorted by species for each mesocosm. Because they were growing intermixed, native grasses could not practically be separated by species at this stage, thus their aboveground biomass was compiled for each pot. We transported mesocosms to the research greenhouse at Northern Arizona University for root washing to harvest belowground biomass. We removed roots and soil from pots while washing with water over soil sieves. Rocks and organic debris were removed by hand. Because roots of different species were highly entangled, we did not try to sort them by species. Instead, we complied all roots into a belowground biomass sample per pot. We dried all above and belowground biomass samples for 2 weeks at 60°C before weighing.
Quantifying Final Management Outcomes
First, we selected and calculated key variables representative of some of the goals of ecological restoration activity at our study site: nativeness of final biomass, the cumulative number of native species ever observed growing in our pots (termed cumulative native richness), an exotic fecundity index, and a final total plant biomass. Nativeness was calculated as the proportion of aboveground biomass belonging to native species; thus, the restoration goal of a fully native community would take a value of 1, and a value of 0 would normally signify complete exotic dominance. In two cases, our pots yielded no measurable biomass at all, in which case we ascribed a value of zero because there were no native species; therefore 0 had a second meaning, equally undesired as complete exotic dominance, in just these two samples. Because separation of belowground biomass by species was difficult and highly impractical, it was ignored for this calculation. The cumulative richness of natives was calculated by tallying the number of native species observed per pot across all time points in the cover data, regardless if they persisted over multiple time points. This value measured the variety of species that might plausibly have had an opportunity to establish had they been growing without competition. Exotic fecundity was based on final cumulative seed mass data. The reproductive value of a given mass of seed differed strongly by species due to traits like seed size and viability. For this reason, to calculate our index, we first relativized seed production within each exotic species by dividing the seed mass of a given species in each pot by the maximum mass observed across all pots. Then we averaged these values for all exotic species. Thus, a value of 1 would have meant that all the exotic species had achieved their maximum observed seed mass output and a value of 0 would have meant that no exotic plants produced any seed mass.
Statistical Analyses
We analyzed our data using structural equation modeling. This type of model was selected because it allows us not only detect effects of biocrusts on plant community properties and management outcomes, but also allows us to partition these effects along direct and indirect pathways, while illuminating the network of effects that our response variables have on each other (Grace, 2006). We describe the details of our modeling approach in the Supplementary Material. Briefly, we (1) developed an a priori model that we wished to test and fit to our data, enabling tests of our hypotheses, (2) checked for major departures from model assumptions, taking corrective steps where needed, (3) tested the overall fit of our models, and identified and implemented one post hoc change that improved model fits for irrigated samples, (4) fit the final models and interpreted their parameters in light of our hypotheses. Our models were used to estimate effects of individual biocrust properties – total cover, richness, proportional moss cover (calculated as moss cover divided by total biocrust cover) – on vascular plants, in addition to their composite effect, termed “biocrust condition” (Grace, 2006). To determine whether key effects were environmentally contingent, we used the degree of overlap of 95% bootstrap confidence intervals around the same parameters under ambient versus irrigated conditions, as well as indirect comparisons of effects to zero. Separate models were created for each combination of ambient and irrigated samples, each available time point, and each management outcome. Some information is redundant across multiple models, but all models contain unique information. We built our SEMs in AMOS 26.0 (IBM SPSS, Chicago, United States).
Results
Most of our structural equation models had an excellent fit to the data (χ2 ≤ 2.5, P = 0.09–0.85, GFI = 0.99, bootstrap P = 0.11–0.83). Fits to our models for September of 2017 were adequate (χ2 = 2.9–5.4, P = 0.07–0.09, GFI = 0.98–0.99, bootstrap P = 0.06–0.07).
Biocrust Effects on Emergence (H1)
Under ambient rainfall conditions, biocrust condition (the composite effect of biocrust total cover, richness, and proportional moss cover) explained 6–13% of the variation in exotic emergence, and 3–15% of the variation in native emergence (Figure 2 and Supplementary Table 1). The composite effect of biocrust condition on exotic emergence was strongest in the initial time point of our analysis (r = |0.36|; Figure 3) and weakened somewhat over time (r = |0.23–0.25|). In all time points, this effect was driven most strongly by a negative effect of total biocrust cover (r = −0.23 to −0.30; Figure 3). In contrast, comparable influences of biocrusts on native emergence were not observed in the initial time point but strengthened afterward (r ≥ |0.35–0.38|; Figure 3). These effects were driven by a positive influence of biocrust richness (r = 0.16–0.24) and a negative influence of proportional moss cover (r = −0.04 to −0.26) (Figure 3 and Table 1).
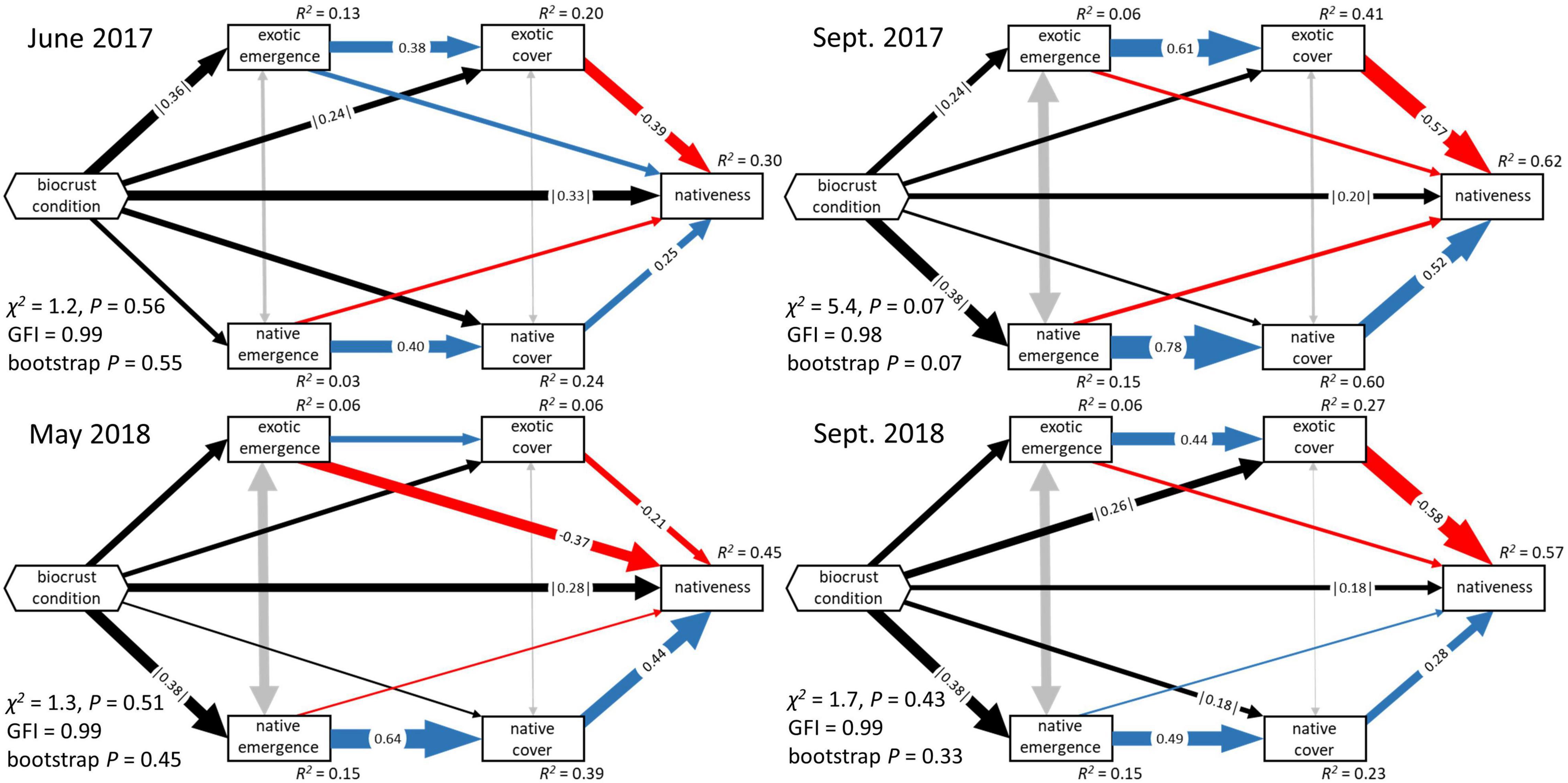
Figure 2. Fitted structural equation models of biocrust effects on plant community development and nativeness under ambient conditions, early and late in both years of the experiment. Additional SEM results are reported in full in Supplementary Material. Boxes represent measured variables, and hexagons represent the composite effect (biocrust condition) of initial total biocrust cover, biocrust richness, and proportional moss cover. Directed, single-headed arrows represent hypothesized causal influences, while gray-colored double headed arrows represent residual correlation caused by common influences external to the models. Width of all arrows is proportional to the path coefficient (shown when bootstrap confidence intervals do not overlap zero). Color of single-headed arrows signifies sign: blue represent positive effects, red represents negative effects, and black represent composite effects that cannot be given a meaningful sign.
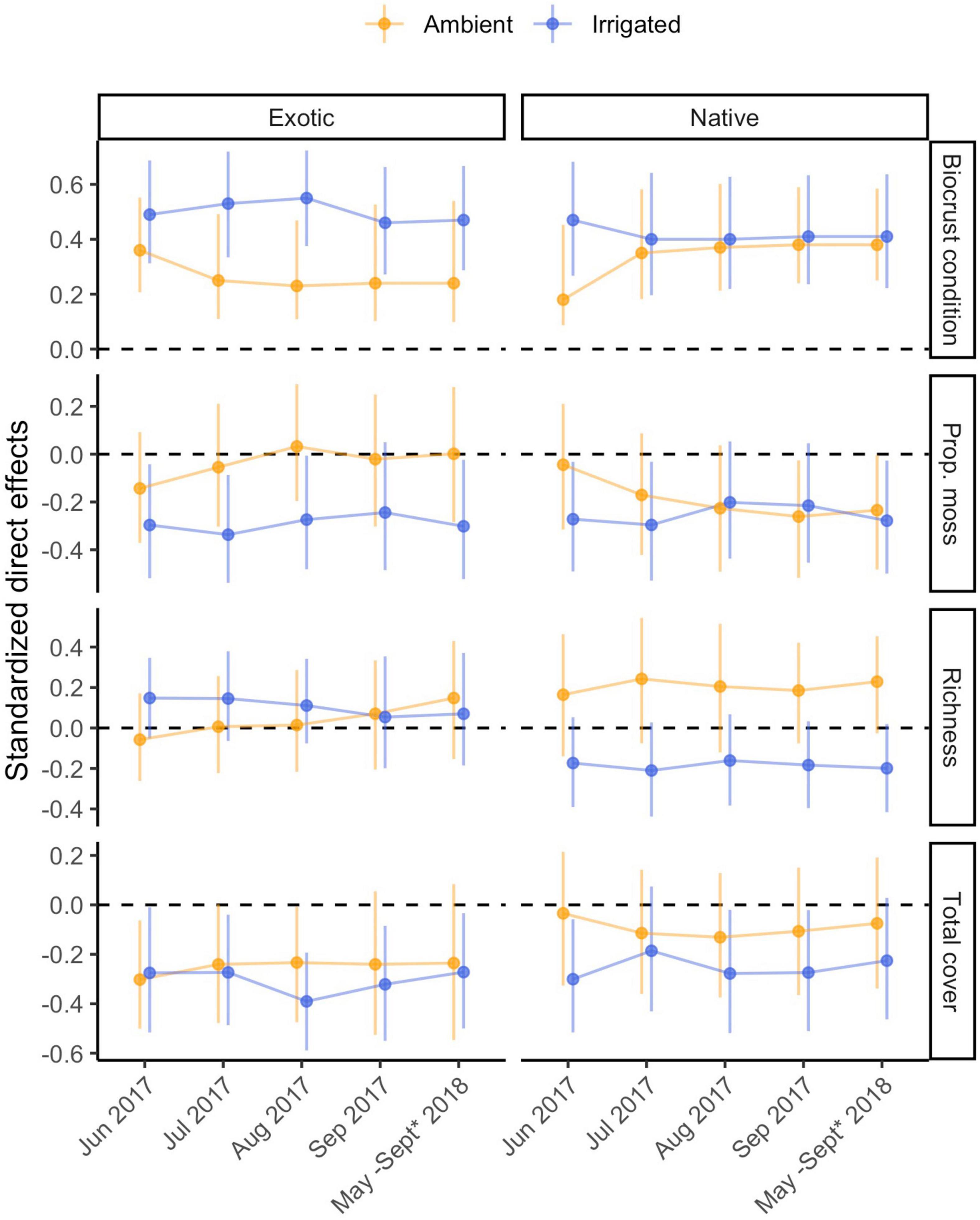
Figure 3. Standardized direct effects of biocrust condition and biocrust properties on exotic and native plant emergence through time, under ambient and irrigated conditions. Error bars represent 95% bootstrap confidence intervals based on 5,000 bootstrap samples. * Monitoring of these data was discontinued in 2018, therefore all models used emergence data from the final 2017 observation.
Under irrigation, biocrust condition explained more variation in both exotic and native emergence in every time point, compared to ambient rainfall, ranging from 21 to 30% in exotic emergence and ranging from 17 to 22% in native emergence (Supplementary Table 1). The composite effect of biocrusts on exotic emergence was strong (r = |0.47–0.55|), peaking mid-summer of 2017 and driven jointly by negative effects of total biocrust cover (r = −0.27 to −0.39) and proportional moss cover (r = −0.28 to −0.34) (Figure 3 and Table 1). The composite effect of biocrust condition on native emergence was also strong (r = |0.40–0.47|); this effect was driven jointly by negative effects of all three biocrust properties (Figure 3 and Table 1).
Biocrust Effects on Plant Cover (H2)
In our models, biocrust condition could have had either direct effects or indirect effects—through their influence on germination—on the cover of exotic and native plants. Under ambient rainfall conditions, our models were able to explain 6–41% of the variation in exotic cover (Figure 2 and Supplementary Table 1). Direct effects of biocrusts on exotic cover were minor to moderate, and were often distinguishable from no effect (r = |0.16–0.27|; Supplementary Table 1). These composite effects were difficult to attribute to particular biocrust properties, although it appeared that late in the experiment richness negatively influenced exotic cover. Exotic emergence had moderate to strong positive effects on exotic cover (r = 0.22–0.61; Supplementary Table 2). Encompassing both direct and indirect pathways as total effects, biocrust properties had no notable effects on exotic cover (Figure 4).
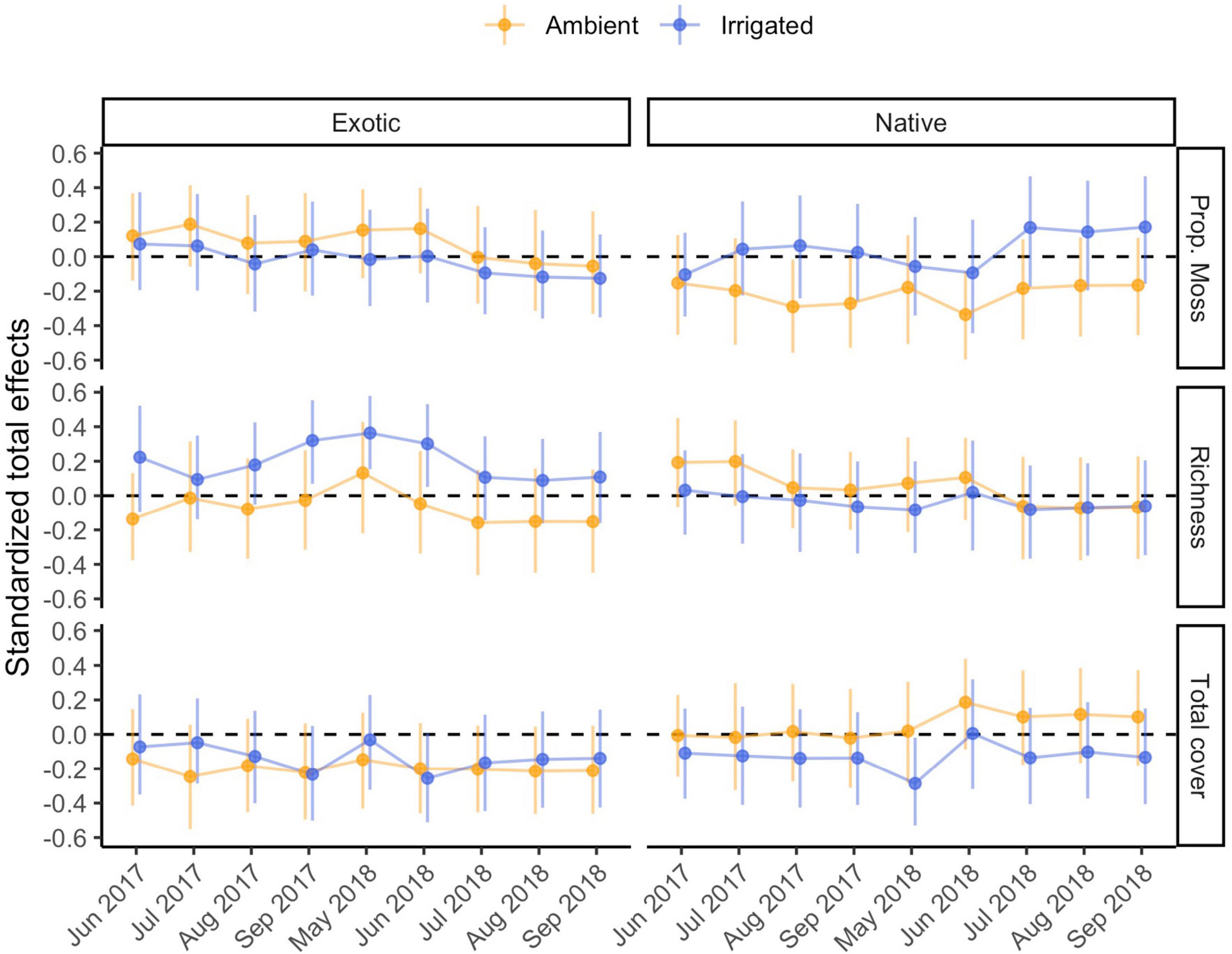
Figure 4. Standardized total effects of biocrust properties on exotic and native plant cover through time, under ambient and irrigated conditions. Error bars represent 95% bootstrap confidence intervals based on 5,000 bootstrap samples. Because total effects of composite variables cannot be computed, the effect of biocrust condition is omitted.
Under ambient rainfall our models explained 12–60% of the variation in native cover (Figure 2 and Supplementary Table 2). Direct effects of biocrusts on native cover were detected in the later portion of the experimental period, particularly in June 2018 (r = 0.28; Supplementary Table 2); this effect was co-driven by a positive influence of total biocrust cover, and a negative influence of proportional moss cover. Native emergence was generally a moderate to very strong positive driver of native cover (r = 0.18–0.78; Supplementary Table 2). Total effects of biocrust properties on native cover were generally weak to moderate, but proportional moss cover did exert a clear, moderate negative total effect (r = −0.34) in June 2018 (Figure 4), the same time point in which there was a stronger than typical direct biocrust effect (Supplementary Table 2).
Under irrigation, we were able to explain 15–46% of the variation in exotic cover (Supplementary Table 1). Most direct effects of biocrusts on exotic cover were weak (r = |0.06–0.11|), except for a period from September 2017 to July 2018 in which they were elevated (r = |0.21–0.31|; Supplementary Table 1). Throughout that period, the effect was driven by a positive effect of biocrust richness on exotic cover (r = 0.22–0.31), and in the final time point, a negative influence of biocrust cover was similar in magnitude (r = −0.22; Supplementary Table 1). Exotic emergence generally exerted much stronger influences on exotic cover than biocrusts did directly, always positive (r = 0.21–0.59) and tending to be higher later in each of the years (Supplementary Table 1). In irrigated pots, an additional negative influence of native emergence on exotic cover was detected. This influence was stronger in the first several observations (r = −0.19 to −0.32), but essentially disappeared in the final three (r = 0.03–0.05; Supplementary Table 1). There were clear total effects of biocrust richness on exotic cover only from September 2017 to July 2018 (r = 0.30–0.36), otherwise no detectable total effects of biocrust were found (Figure 4).
Under irrigation, we were able to explain 6–45% of the variation in native cover (Supplementary Table 2). Direct effects of biocrusts on native cover were weak to moderate throughout (r = |0.12–0.26|), with most of the stronger effects being mostly driven by a positive influence of moss cover (r < 0.01–0.26; Supplementary Table 2). In contrast, the effects of native emergence were strong and positive in the first year (r = 0.61–0.73) but diminished in the second year (r = 0.17–0.34; Supplementary Table 2); the degree of influence of native emergence largely determined the wide range in R2 of native cover. There were no total effects of biocrusts on native cover, except for a negative influence (−0.29) of total cover in May 2018 (Figure 4).
Biocrust Effects on Management Outcomes (H3, H4)
Nativeness
Under ambient rainfall, our models explained 30–62% of the variation in nativeness (Figure 2 and Supplementary Table 3). The strongest direct effect of biocrusts was observed in the first time point (r = |0.33|) and decreased afterward (r = |0.17–0.33|); these effects were driven by a positive influence of biocrust cover (Supplementary Table 3). Nativeness was usually strongly co-determined by negative effects of exotic cover (r = −0.18 to −0.66) and positive effects of native cover (r = 0.28–0.52; Supplementary Table 3). Occasionally, either exotic or native emergence also had direct effects on nativeness (Supplementary Table 3). Clear total effects of biocrusts were also detected. The strongest was always a positive effect of total biocrust cover (r = 0.34), whereas biocrust richness and proportional moss cover did not have detectable total effects (Figure 5).
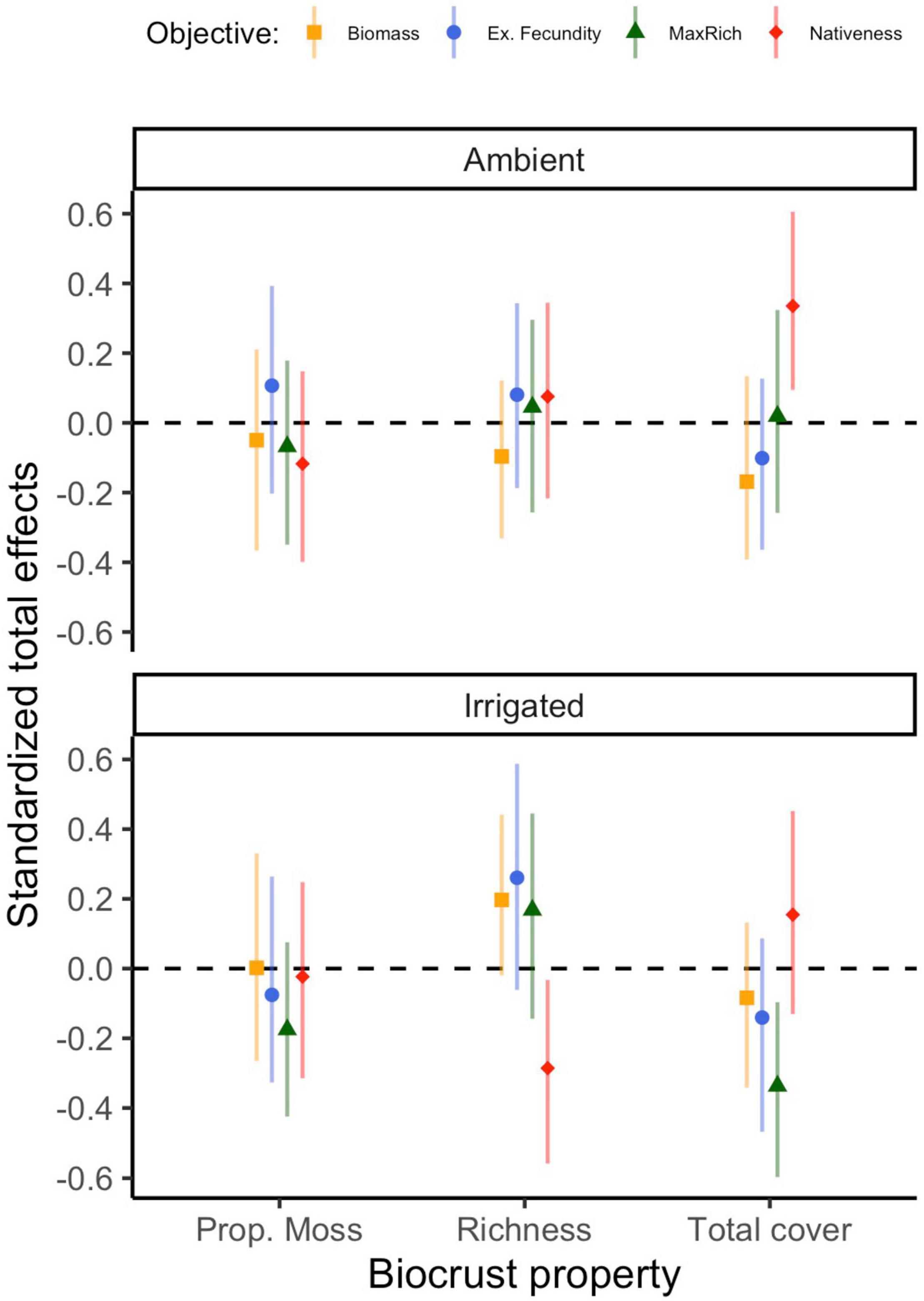
Figure 5. Standardized total effects of biocrust properties on management outcomes through time, under ambient and irrigated conditions. Estimates of these effects differ very slightly (≤0.009) across model runs in different time points, therefore the mean effect and its mean bootstrapped 95% confidence interval (based on 500 bootstrap samples) are plotted. Because total effects of composite variables cannot be computed, the effect of biocrust condition is omitted.
Under irrigation, our models explained 39–69% of the variation in nativeness (Supplementary Table 3). Direct effects of biocrusts on nativeness were moderate in July 2017 (r = |0.22|), but otherwise weaker (r = |0.12–0.18|; Supplementary Table 3). These were mostly driven by negative influences of biocrust richness and proportional moss cover. Exotic (r = −0.30 to −0.46) and native cover (r = 0.24–0.37) largely co-determined nativeness in the first year, but by the second year, negative effects of exotic cover were the prevalent factor (r = −0.63 to −0.67) and the effects of native cover were negligible (Supplementary Table 3). Negative influences of exotic emergence on nativeness were seen throughout (r = −0.18 to −0.44), at times comparable to the effects on exotic cover, and some positive influences of native emergence were seen in the final few time points (r = 0.21–0.25; Supplementary Table 3). The strongest total effect of the biocrust properties on nativeness under irrigation was a negative effect of biocrust richness (r = −0.29; Figure 5).
Cumulative Native Richness
Under ambient rainfall, our models explained 9–40% of the variation in cumulative native richness (Supplementary Table 4). Direct effects of biocrusts on cumulative native richness were not detected. Rather, the sole major influence on cumulative native richness was native emergence, especially in 2017 (r = 0.41–0.63), and less so in 2018 (r = 0.22–0.32; Supplementary Table 4). Total effects of biocrust properties were negligible (Figure 5).
Under irrigation, our models explained 33–40% of the variation in cumulative native richness (Supplementary Table 4). Biocrusts consistently influenced cumulative richness (r = |0.27–0.35|). These effects were mainly co-driven by a positive influence of total biocrust cover, and a negative influence of biocrust richness (Supplementary Table 4). Native emergence also consistently positively influenced cumulative native richness (r = 0.33–0.40; Supplementary Table 4), generally a clearer and somewhat stronger effect. Finally, we also detected a few effects of exotic germination or cover in the final few observations. The strongest total effect of the biocrust properties on cumulative native richness under irrigation was a negative effect of total biocrust cover (r = −0.34; Figure 5).
Exotic Fecundity
Under ambient rainfall, our models explained 11–46% of the variation in exotic fecundity (Supplementary Table 5). No clear direct effects of biocrust condition on exotic fecundity were detected (r = |0.09–0.18|). Exotic cover was the most frequent moderate to strong positive influence on exotic fecundity, especially before May of 2018 (r = 0.21–0.60) but was poorly predictive afterward (r = 0.07–0.10; Supplementary Table 5). There were some positive effects of either native emergence on exotic fecundity detected in some time points as well (r = −0.07 to 0.32; Supplementary Table 5). We did not detect any total effects of the individual biocrust properties on exotic fecundity (Figure 5).
Under irrigation, our models explained 19–37% of the variation in exotic fecundity (Supplementary Table 5). Direct effects of biocrusts on exotic fecundity under irrigation were sometimes detected, particularly later in 2018 (r = |0.20–0.28|); these were co-driven by positive effects of richness and negative effects of total cover. Exotic (r = 0.14–0.44) and native cover (r = −0.02 to −0.35) were frequently detected influences on exotic fecundity, though seldom very strong ones (Supplementary Table 5). There was a moderate positive influence of exotic emergence on exotic fecundity at one early time point (r = 0.29; Supplementary Table 5). None of the total effects of biocrust properties were clearly distinguishable from zero (Figure 5).
Total Biomass
Under ambient rainfall, our models explained 28–50% of the variation in total biomass (Supplementary Table 6). In only one time point (May 2018), biocrusts appeared to exert a direct influence (r = |0.24| on total biomass, mostly driven by a negative effect of biocrust richness), but otherwise effects were weaker (r ≤ |0.20|; Supplementary Table 6). By far the key effect, positive and at times very strong, was exerted by exotic cover (r = 0.39–0.80). At times, some positive effects of native cover or emergence were also observed (r = 0.20–0.35), and in one case we found a negative moderate effect of exotic emergence (Supplementary Table 6). We did not detect any notable total effects of biocrust properties on total biomass (Figure 5).
Under irrigation, our models explained 13–42% of the variation in total biomass (Supplementary Table 6). We failed to detect any direct effects of biocrust condition on total biomass. The key, positive, and consistent influence on total biomass was exotic cover (r = 0.23–0.71); in the final months of the experiment, we also observed some lesser negative effects of native emergence (r = −0.22 to −0.26; Supplementary Table 6). There were no notable total effects of biocrust properties on total biomass (Figure 5).
Environmental Contingency of Biocrust Effects on Vascular Plants (H5)
Confidence intervals of direct effects on emergence, and total effects on plant cover and management under ambient compared to irrigated conditions always overlapped indicating a lack of strong differences (Figures 3–5). In some instances, biocrust effects differed from zero in either the ambient or irrigated conditions, but not the other. Notable examples include: (1) the neutral direct effects of proportional moss cover on exotic emergence became mostly negative under irrigation (Figure 3), (2) the neutral total effects of richness on exotic cover became mostly positive under irrigation (Figure 4), (3) the sometimes negative total effect of proportional moss cover on native cover became neutral under irrigation (Figure 4), (4) the positive total effect of total biocrust cover on nativeness became neutral under irrigation (Figure 5), and (5) the neutral total effect of biocrust richness on nativeness became negative under irrigation (Figure 5).
Discussion
In this study we confronted a diverse vascular plant seed mix, with soil environments differing mainly due to highly variable biocrust properties, and environmental variation in the form of an irrigation treatment. Overall, we found that pre-existing biocrusts influenced the colonization of seeded vascular plant communities, primarily early in the emergence stage, and less so as plants grow and expand their cover. These influences upon the vascular plant community development sometimes reverberate to produce outcomes relevant to management and ecological restoration. Biocrust cover promoted nativeness under ambient rainfall conditions, but when water limitation was experimentally decreased through irrigation, biocrust cover may have suppressed cumulative native plant richness, and biocrust richness may have promoted exotic fecundity. Biocrusts had little impact on total plant biomass.
Biocrust Conditions Induce Priority Effects in Plant Community Development
It is challenging to make generalizations about how biocrusts influence vascular plants because effects vary with plant life history stage (Havrilla et al., 2019), even within one species (Ferrenberg et al., 2018). For example, biocrusts may delay germination of Bromus tectorum, but also promote growth of germinants due to greater resource availability (Ferrenberg et al., 2018). Studies which observe plant responses to biocrusts across multiple life history stages are promising developments (Langhans et al., 2009; Slate et al., 2018). In this study, we not only made observations across multiple life history stages and seasons but document the degree to which the earlier effects influence later ones.
Our most clearly detected biocrust effects on plants were at the emergence stage, where we found mixed support for the hypothesis that biocrusts would have different effects on native and exotic emergence (Hypothesis 1, Table 1). The overall magnitude of the effect of biocrust condition on exotic and native emergence was generally similar. Focusing on ambient conditions, the main way biocrust effects on exotic and native emergence differed was in the identity of individual biocrust effects. For example, biocrust cover generally negatively influenced exotic emergence compared to a more neutral effect on native emergence. More negative biocrust effects on exotic plant emergence compared to native plant emergence are common in the literature (Havrilla et al., 2019). On the other hand, biocrust richness generally positively influenced native emergence compared to a more neutral effect on exotic emergence. We are not aware of any other study that detected this pattern.
In our experiment, plant cover was meant to measure plant success after the initial colonization period represented by the emergence stage as plants grew and competed for space. In the cover data, we found little evidence that biocrusts affect both native and exotic cover in similar ways (Hypothesis 2; Table 1). Overall, we found only a few clear total effects of biocrusts on cover, in the middle period of the experiment and under irrigated conditions. These effects were always on exotic cover but not on native cover, providing some evidence against hypothesis 2 (Table 1). These total effects coincided with periods in which direct effects were also detected, and biocrust richness appeared to directly promote exotic cover but not native cover. Under irrigation, water became a less limiting factor and plant growth would be expected to be limited by soil nutrients. In some systems, biocrust richness is linked to greater nutrient and carbon stocks and cycling rates (Maestre et al., 2012; Bowker et al., 2013; Garibotti et al., 2018). Thus, we might propose that richer biocrusts present a favorable environment to already-established exotic plants, and this might be the basis for the observed direct effects; these effects could be magnified if some of the exotic species have a relatively large maximal canopy cover (e.g., C. stoebe).
There were additional time periods when total effects of biocrusts on cover were absent, but direct effects revealed that exotic cover was being affected by biocrusts in different ways than native cover, further casting doubt on Hypothesis 2 (Table 1). For example, under ambient conditions in early time points there was a clear direct effect of biocrust condition on exotic cover, but no clear effect on native cover. Presence of direct effects in the absence of total effects was not surprising because direct and indirect (via emergence) effects of biocrusts were often not driven in the same way by the same biocrust properties. For example, though native emergence appeared to be positively influenced by biocrust richness, the influence of biocrust richness on native cover was more likely to be slightly negative than positive. In general, opposing paths may lead to a total (net) effect that is difficult to distinguish from zero (Grace, 2006).
Perhaps one of our most interesting findings was that biocrusts appeared to exert their strongest effects on vascular plants at the emergence stage, yet these effects both persisted for the entire experiment and cascaded to influence other life history stages. Zhang and Belnap (2015) proposed that because biocrusts can favor emergence of some vascular plant species over others, they may shape the eventual plant community composition; we obtained evidence from developing plant communities that this is true. In this study, cover attained by exotic and native species was generally strongly linked to their rate of emergence in the previous time point; this was true in both ambient and irrigated conditions. In some time points and in irrigated conditions only, native emergence also influenced exotic cover, suggesting competitive species interactions. The persistent influence of emergence on later plant cover and eventual management outcomes may indicate strong priority effects, whereby the arrival and establishment order of plants creates large differences in the eventual composition and structure of the community (Fukami, 2015; Hess et al., 2019). Perhaps the induction of priority effects is one of the main ways that biocrusts can influence vascular plant community composition and is certainly worthy of further study; a direct test of this hypothesis could make use of different orders of seed delivery over contrasting biocrust surfaces.
Management Outcomes of Biocrust-Vascular Plant Interactions
In many places around the world, exotic plant invasions present a major challenge for land management and a barrier to ecological restoration. Therefore, many management and restoration activities aim to promote native plants (Kimball et al., 2015) and discourage the success of exotic ones (Weidlich et al., 2020). We studied three metrics aligned with this goal to test Hypothesis 3 (Greater abundance of biocrusts will promote nativeness of biomass and cumulative richness of native species, and lower exotic plant fecundity in the final community) and obtained context-dependent results (Table 1).
Under ambient rainfall, Hypothesis 3 was supported because nativeness of biomass was positively associated with biocrust cover (Table 1), mostly via the differential effects on native and exotic emergence, and to a lesser degree through direct effects of biocrusts on plant biomass. In contrast to nativeness, cumulative native richness and exotic fecundity were unresponsive to the properties of biocrusts.
Under irrigation, Hypothesis 3 had mixed support (Table 1). In support of the hypothesis, biocrust richness directly promoted cumulative native richness, and biocrust cover directly reduced exotic fecundity. Providing evidence against Hypothesis 3 there was a negative total effect of biocrust richness on nativeness, a negative effect of biocrust cover on cumulative native plant richness, and a positive effect of biocrust richness on exotic plant fecundity.
The varied effects of biocrust richness under irrigation could have arisen for different reasons. Biocrust richness might tend to promote greater heterogeneity in the properties of the soil surface, which could favor establishment of a wider array of native or exotic species, perhaps explaining its positive association with cumulative native richness (Baer et al., 2020). Nevertheless, exotic species appear to be more successful than native species in biomass production and reproductive output when biocrusts are more species rich, and the soil is presumably more heterogeneous. Exotic species often have higher relative growth rates than natives, and this trait is linked to greater resource foraging precision and performance in heterogeneous soils (James et al., 2010). On the other hand, heterogeneity in soil nutrients may increase exotic plant growth, but does not necessarily lead to invasion success of exotics growing in the presence of natives (Gao et al., 2021). Another plausible explanation for these influences of biocrust richness might be that biocrusts with higher species richness are more fertile due to richness-function relationships (Bowker et al., 2013) favoring some nitrophilic native species at the expense of others, and favoring exotic growth over native growth, and ultimately favoring exotic reproduction.
The negative effects of biocrust cover on both native and exotic plants under irrigation might be attributed to elevated resource competition for certain nutrients when biocrusts are abundant (Harper and Belnap, 2001). Alternatively, biocrust organisms might also produce allelopathic compounds (Sedia and Ehrenfeld, 2003; Escudero et al., 2007), thus a greater abundance of biocrust organisms might lead to a greater presence of these substances. However, it is unclear why these hypothetical negative interactions might occur under irrigation but not under ambient conditions.
Another driver of land management and restoration practices that is increasing in importance is promoting biomass production (Derner and Schuman, 2007). Increasing biomass production is an approach to carbon sequestration both in short-lived pools such as herbaceous biomass and in longer-term ones such as soil organic matter (Yang et al., 2019). We found that the experiment’s final cumulative biomass was very well-predicted by plant cover and that exotic cover was far more predictive than the native cover.
Exotic invasive species commonly grow faster or larger than native species in invaded sites (Weidlich et al., 2020). This might explain some of the success of exotics outside of their native range (van Kleunen et al., 2010). Exotic invasion may lead to a more productive ecosystem (Maron et al., 2014) as more plant niches are occupied. This may put the management goals of carbon storage and exotic plant reduction/native plant promotion at odds with one another to some degree. Given these observations, we might expect that biocrusts, by discouraging exotic plants, would also discourage biomass accumulation. On the contrary, direct effects of biocrusts on plant biomass were rare, temporary, and moderate in strength at most. The total effects of biocrusts suggest that early in the experiment, and only under irrigation, the richness of the biocrusts had some subtle positive effects on biomass, possibly though promotion of exotics. Overall, we cannot conclude that biocrusts were anything more than a minor influence on plant biomass, largely consistent with our Hypothesis 4 (Biocrust condition will not influence overall biomass production; Table 1). Although the biocrusts themselves are also an ecosystem C pool, this unquantified amount would be expected to be much smaller than plant biomass, thus we would posit that there were only minor effects of biocrusts on ecosystem C as well.
Overall, in our study system under a typical rainfall condition, more biocrust tended to promote either positive or neutral effects on key management outcomes of ecological restoration seedings. These are influences, not deterministic controls. For example, although more biocrust may lead to measurably more nativeness, exotic invasive plants can still establish and be successful in the presence of biocrusts. Much variance in plant community properties and management outcomes remained unexplained in our models and could be caused by influences as strong or stronger than biocrusts such as herbivory or random variation in seed viability. These mostly neutral to positive biocrust effects are in addition to well-documented benefits of biocrusts manifested in the soil environment, such as elevated nutrient cycling, soil carbon and fertility, and aggregate stability.
Environmental Contingency of Biocrust-Plant Interactions
We found mixed evidence for our hypothesis 5 (Table 1), that biocrust effects on plants were contingent on water availability. Direct comparisons of biocrust effects on plants under ambient and irrigated conditions did not reveal major differences, providing evidence against hypothesis 5. However, we detected several instances in which a neutral effect (indistinguishable from zero) under the ambient condition became a clearer positive or negative influence, or vice-versa, especially regarding management outcomes. Thus, our probability of detecting a particular biocrust effect on plants or management outcomes is environmentally contingent; this finding supports hypothesis 5 (Table 1). A major complication of interpreting our results and the results of any biocrust-plant interaction experiment is that omission of water limitation through record rainfall would tend to alter the nature of biocrust-plant interactions, for example by inducing more negative outcomes from a management perspective. Therefore, although our ambient rainfall results are probably the better guide to what might happen in most years, it becomes important to have a better grasp on how outcomes might shift due to interannual variability. For example, what would be the outcome of our experiment under drought or a slightly above-average rainfall year? Environmental contingency also implies that we cannot know based on a single study if our findings might transfer to other ecosystem types.
Bringing a Stronger Community Perspective to Biocrust-Plant Interaction Studies
In nature, and in ecological restoration, plant success or failure occurs in the context of a community (Noreika et al., 2020). Plants interact with other plants both negatively, e.g., allelopathy and resource competition, and positively, e.g., nurse plants and shared root symbionts (Tilman, 1988; Hacker and Gaines, 1997; Ridenour and Callaway, 2001; van der Heijden and Horton, 2009). Nevertheless, nearly all of the many studies which determine plant responses to biocrusts do so using only one plant species at a time. On one hand, this practice isolates the biocrust-plant interaction so that it can be seen more clearly. Simultaneously, the practice decreases our ability to translate results to the real world where the fates of plants are linked to other plants around them. Over the past few decades, the main thing that we have collectively learned about biocrust-plant species interactions is that they are variable and very difficult to generalize, because they are species-dependent and life-history stage-dependent (Havrilla et al., 2019). In this article we demonstrate and suggest wider adoption of studies testing the response of plant communities to biocrusts, not just plant individuals or populations of a single species. Our recommendation includes experimental approaches like ours, as well as observational studies (DeFalco et al., 2001; Sedia and Ehrenfeld, 2003; Luzuriaga et al., 2012). A single species’ response to biocrusts may be locally or regionally important, but the response of community properties (e.g., nativeness) could be relevant anywhere. Much vegetation management—including ecological restoration—is conducted at the community scale, and its outcomes are monitored by measuring community properties. Since we do not know the degree to which single-species focused experiments can translate to the community level, we need community-level experiments to meet the information needs of managers.
Our inferences can be richer still if we acknowledge that biocrusts are also communities with high spatial variability. Most commonly, biocrusts are treated as a binary (e.g., a biocrust compared to some type of reference lacking biocrust) or multi-level categorical factor (e.g., cyanobacterial, lichen, and moss-dominated biocrusts) in biocrust-plant interaction studies. While this may be adequate in some locations, in others it is an oversimplification because biocrusts may be highly variable at small scales, ranging from 0 to 100% cover, containing a few to many species, spatially homogenous to heterogeneous or clumped to dispersed, and containing many different mixtures of major organism types (e.g., mixed cyanobacterial, lichen, moss biocrusts) or different dominant species. Since we know that not all biocrusts are the same in different study sites, it may be beneficial to embrace the full variety of biocrusts, treating multiple key and universally important biocrust properties as continuous factors influencing a plant community response. A regression experimental or sampling design is a useful tool enabling this type of study (Cottingham et al., 2005). This practice would allow us to see differences between extremes and gain a better understanding of the functional relationships between biocrust community properties and plant community properties.
Because biocrust communities have existed during the entire history of vascular plant communities (Beraldi-Campesi and Retallack, 2016), the interaction of these two communities shows us how the properties of a pre-existing community can determine the properties of another developing one, both in the present and on ancient Earth.
Data Availability Statement
The raw data supporting the conclusions of this article will be made available by the authors, without undue reservation.
Author Contributions
MB: experimental design (co-lead), analysis (lead), and writing (lead). KD: experimental design (co-lead), data collection (lead), analysis (supporting), and writing (supporting). AA, PR, and RD: experimental design (supporting) and writing (supporting). MD: data collection (supporting) and writing (supporting). All authors edited and approved the final version.
Funding
This research was made possible by funding from the MPG Ranch Foundation.
Conflict of Interest
The authors declare that the research was conducted in the absence of any commercial or financial relationships that could be construed as a potential conflict of interest.
Publisher’s Note
All claims expressed in this article are solely those of the authors and do not necessarily represent those of their affiliated organizations, or those of the publisher, the editors and the reviewers. Any product that may be evaluated in this article, or claim that may be made by its manufacturer, is not guaranteed or endorsed by the publisher.
Acknowledgments
We thank the Gurinas family for supporting our work and applied ecology, generally. We are grateful to Prairie Wolfe for seed collections, Henry Grover and Chuck Casper for experimental setup, and Peter Chuckran, Beau Jennings, and Michael Sloan for data collection and technical assistance. We also thank the reviewers for improving the earlier versions of the manuscript. We also thank Phil Patterson and the Northern Arizona University Research Greenhouse for providing support and workspace for root washing.
Supplementary Material
The Supplementary Material for this article can be found online at: https://www.frontiersin.org/articles/10.3389/fevo.2022.840324/full#supplementary-material
References
Baer, S. G., Adams, T., Scott, D. A., Blair, J. M., and Collins, S. L. (2020). Soil heterogeneity increases plant diversity after 20 years of manipulations during grassland restoration. Ecol. Appl. 30:e02014. doi: 10.1002/eap.2014
Belnap, J., Büdel, B., and Lange, O. L. (2003a). “Biological soil crusts: characteristics and distribution,” in Biological Soil Crusts: Structure, Function, And Management, eds J. Belnap and O. L. Lange (Berlin: Springer-Verlag), 3–30.
Belnap, J., Hawkes, C. V., and Firestone, M. K. (2003b). Boundaries in miniature: two examples from soil. BioScience 53, 739–749.
Bender, S. F., and van der Heijden, M. G. A. (2015). Soil biota enhance agricultural sustainability by improving crop yield, nutrient uptake and reducing nitrogen leaching losses. J. Appl. Ecol. 52, 228–239.
Beraldi-Campesi, H., and Retallack, G. J. (2016). “Terrestrial ecosystems in the Precambrian,” in Biological Soil Crusts: An Organizing Principle In Drylands, eds B. Weber, B. Büdel, and J. Belnap (Switzerland: Springer), 37–54.
Bowker, M. A., Maestre, F. T., and Mau, R. L. (2013). Diversity and patch-size distributions of biological soil crusts regulate dryland ecosystem function. Ecosystems 16, 923–933.
Briegel-Williams, L., Borchhardt, N., Colesie, C., Baum, C., Komsic-Buchmann, K., Rippin, M., et al. (2017). Biological soil crusts of Arctic Svalbard and of Livingston Island Antarctica. Polar Biol. 40, 399–411.
Corbin, J. D., and Thiet, R. K. (2020). Temperate biocrusts: mesic counterparts to their better-known dryland cousins. Front. Ecol. Environ. 18:456–464. doi: 10.1002/fee.2234
Cottingham, K. L., Lennon, J. T., and Brown, B. (2005). Knowing when to draw the line: designing more informative ecological experiments. Front. Ecol. Environ. 3:145–152. doi: 10.1890/1540-9295
DeFalco, L. A., Detling, J. K., Tracy, C. R., and Warren, S. D. (2001). Physiological variation among native and exotic winter annual plants associated with microbiotic crusts in the Mojave Desert. Plant Soil. 234, 1–14.
Derner, J. D., and Schuman, G. E. (2007). Carbon sequestration and rangelands: A synthesis of land management and precipitation effects. J. Soil Water Conserv. 62, 77–85.
Doherty, K. D. (2019). Producing and Deploying Biocrust Moss Materials for Ecological Restoration. Flagstaff : AZ: Northern Arizona University. [PhD thesis].
Durham, R. A., Doherty, K. J., Antoninka, A. J., Ramsey, P., and Bowker, M. A. (2018). Insolation and disturbance history drive biocrust biodiversity in Western Montana rangelands. Plant Soil 430, 151–169.
Eldridge, D. J., Reed, S. C., Travers, S., Bowker, M. A., Maestre, F. T., Ding, J., et al. (2020). The pervasive and multifaceted influence of biocrusts on water in the world’s drylands. Glob. Chang. Biol. 26, 6003–6014. doi: 10.1111/gcb.15232
Escudero, A., Martínez, I., de la Cruz, A., Otálora, M. A. G., and Maestre, F. T. (2007). Soil lichens have species-specific effects on the seedling emergence of three gypsophile plant species. J. Arid Environ. 70, 18–28.
Ferrenberg, S., Faist, A. M., Howell, A., and Reed, S. C. (2018). Biocrusts enhance soil fertility and Bromus tectorum growth, and interact with warming to influence germination. Plant Soil 429, 77–90. doi: 10.1007/s11104-017-3525-1
Fukami, T. (2015). Historical contingency in community assembly: integrating niches, species pools, and priority effects. Ann. Rev. Ecol. Evol. Syst. 46, 1–23.
Gao, F. L., He, Q. S., Zhang, Y. D., Hou, J. H., and Yu, F. H. (2021). Effects of soil nutrient heterogeneity on the growth and invasion success of alien plants: a multi-species study. Front. Ecol. Evol. 8:619861. doi: 10.3389/fevo.2020.619861
Garibotti, I. A., Gonzales Polo, M., and Tabeni, S. (2018). Linking biological soil crust attributes to the multifunctionality of vegetated patches and interspaces in a semiarid shrubland. Funct. Ecol. 32, 1065–1078. doi: 10.1111/1365-2435.13044
Grace, J. B. (2006). Structural Equation Modeling and Natural Systems. Cambridge, UK: Cambridge University Press.
Grover, H. S., Bowker, M. A., Fulé, P. Z., Doherty, K. D., Seig, C. H., and Antoninka, A. J. (2020). Post-wildfire moss colonization and soil functional enhancement in forests of southwestern USA. Int. J. Wildland Fire 29, 530–540. doi: 10.1071/wf19106
Hacker, S. D., and Gaines, S. D. (1997). Some implications of direct positive interactions for community species diversity. Ecology 78, 1990–2003. doi: 10.1890/0012-9658(1997)078[1990:siodpi]2.0.co;2
Harper, K. T., and Belnap, J. (2001). The influence of biological soil crusts on mineral uptake by associated vascular plants. J. Arid Environ. 47, 347–357. doi: 10.1006/jare.2000.0713
Havrilla, C., Chaudhary, V. B., Ferrenberg, S., Antoninka, A., Belnap, J., Bowker, M., et al. (2019). Towards a predictive framework for biocrust mediation of plant performance: a meta-analysis. J. Ecol. 107, 2789–2807.
Heinen, R., Biere, A., Harvey, J. A., and Bezemer, T. M. (2018). Effects of soil organisms on aboveground plant-insect interactions in the field: patterns, mechanisms and the role of methodology. Front. Ecol. Evol. 6:106. doi: 10.3389/fevo.2018.00106
Hess, M., Mesléard, F., and Buisson, E. (2019). Priority effects: Emerging principles for invasive plant species management. Ecol. Eng. 127, 48–57.
Hijmans, R. J., Cameron, S. E., Parra, J. L., Jones, P. G., and Jarvis, A. (2005). Very high resolution interpolated climate surfaces for global land areas. Int. J. Climatol. 25, 1965–1978.
James, J. J., Zigenhagen, L., and Aanderud, Z. T. (2010). Exploitation of nutrient-rich soil patches by invasive annual and native perennial grasses. Inv. Plant Sci. Manage. 3, 169–177.
Jones, C. G., Lawton, J. H., and Shachak, M. (1994). Organisms as ecosystem engineers. Oikos 69, 373–386. doi: 10.1353/pbm.2003.0003
Kimball, S., Lulow, M., Sorenson, Q., Balazs, K., Fang, Y. C., Davis, S. J., et al. (2015). Cost-effective ecological restoration. Rest. Ecol. 23, 800–810.
Klironomos, J. N. (2001). Feedback with soil biota contributes to plant rarity and invasiveness in communities. Nature 417, 67–70. doi: 10.1038/417067a
Langhans, T. M., Storm, C., and Schwabe, A. (2009). Biological soil crusts and their microenvironment: impact on emergence, survival and establishment of seedlings. Flora 204, 157–168. doi: 10.1016/j.flora.2008.01.001
Liao, H., Luo, W., Peng, S., and Callaway, R. M. (2015). Plant diversity, soil biota and resistance to exotic invasion. Divers. Distrib. 21, 826–835. doi: 10.1111/ddi.12319
Luzuriaga, A. L., Sánchez, A. M., Maestre, F. T., and Escudero, A. (2012). Assemblage of a semi-arid annual plant community: Abiotic and biotic filters act hierarchically. PLoS One 7:e41270. doi: 10.1371/journal.pone.0041270
Maestre, F. T., Castillo-Monroy, A. P., Bowker, M. A., and Ochoa-Hueso, R. (2012). Species richness effects on ecosystem multifunctionality depend on evenness, composition and spatial pattern. J. Ecol. 10, 317–330.
Maron, J. L., Auge, H., Pearson, D. E., Korell, L., Hensen, I., Suding, K. N., et al. (2014). Staged invasions across disparate grasslands: effects of seed provenance, consumers and disturbance on productivity and species richness. Ecol. Let. 17, 499–507. doi: 10.1111/ele.12250
Noreika, N., Pärtel, M., and Öckinger, E. (2020). Community completeness as a measure of restoration success: multiple-study comparisons across ecosystems and ecological groups. Biodiv. Conserv. 29, 1–21.
Ponzetti, J. M., and McCune, B. P. (2001). Biotic soil crusts of Oregon’s shrub steppe: community composition in relation to soil chemistry, climate, and livestock activity. Bryologist 104, 212–225. doi: 10.1639/0007-2745(2001)104[0212:bscoos]2.0.co;2
Ridenour, W. M., and Callaway, R. M. (2001). The relative importance of allelopathy in interference: the effects of an invasive weed on a native bunchgrass. Oecologia 126, 444–450. doi: 10.1007/s004420000533
Rodriguez-Caballero, E., Belnap, J., Büdel, B., Crutzen, P. J., Andreae, M. O., Pöschl, U., et al. (2018). Dryland photoautotrophic soil surface communities endangered by global change. Nat. Geosci. 11, 185–189. doi: 10.1038/s41561-018-0072-1
Sedia, E. G., and Ehrenfeld, J. G. (2003). Lichens and mosses promote alternate stable plant communities in the New Jersey Pinelands. Oikos 100, 447–458. doi: 10.1034/j.1600-0706.2003.12058.x
Slate, M. L., Callaway, R. M., and Pearson, D. E. (2018). Life in interstitial space: Biocrusts inhibit exotic but not native plant establishment in semi-arid grasslands. J. Ecol. 107, 1317–1327.
Tilman, D. (1988). Plant Strategies And The Dynamics And Structure Of Plant Communities. Princeton, NJ: Princeton University Press.
Torres-Cruz, T. J., Howell, A. J., Reibold, R. H., McHugh, T. A., Eickhoff, M. A., and Reed, S. C. (2018). Species-specific nitrogenase activity in lichen-dominated biological soil crusts from the Colorado Plateau. U.S.A. Plant Soil 429, 113–125. doi: 10.1007/s11104-018-3580-2
van der Heijden, M., Klironomos, J. N., Ursic, M., Moutoglis, P., Streitwolf-Engel, R., Boller, T., et al. (1998). Mycorrhizal fungal diversity determines plant biodiversity, ecosystem variability and productivity. Nature 396, 69–72. doi: 10.1038/23932
van der Heijden, M. G. A., and Horton, T. R. (2009). Socialism in soil? The importance of mycorrhizal fungal networks for facilitation in natural ecosystems. J. Ecol. 97, 1139–1150.
van Kleunen, M., Weber, E., and Fischer, M. (2010). A meta-analysis of trait differences between invasive and non-invasive plant species. Ecol. Let. 13, 235–245. doi: 10.1111/j.1461-0248.2009.01418.x
Wardle, D. A., Bardgett, R. D., Klironomos, J. N., Setälä, H., van der Putten, W. H., and Wall, D. H. (2004). Ecological linkages between aboveground and belowground biota. Science 304, 1629–1633. doi: 10.1126/science.1094875
Weidlich, E. W. A., Flórido, F. G., Sorrini, T. B., and Brancalion, P. H. S. (2020). Controlling invasive plant species in ecological restoration: a global review. J. Appl. Ecol. 57, 1086–1817.
Wubs, E. R. J., van der Putten, W., Mortimer, S. R., Korthals, G. W., Duyts, H., Wagenaar, R., et al. (2019). Single introductions of soil biota and plants generate long-term legacies in soil and plant community assembly. Ecol. Let. 22, 1145–1151. doi: 10.1111/ele.13271
Yang, Y., Tilman, D., Furey, G., and Lehman, C. (2019). Soil carbon sequestration accelerated by restoration of grassland biodiversity. Nat. Comm. 10, 718. doi: 10.1038/s41467-019-08636-w
Keywords: plant-soil interactions, ecological restoration, exotic plants, invasion, lichen, moss
Citation: Bowker MA, Doherty KD, Antoninka AJ, Ramsey PW, DuPre ME and Durham RA (2022) Biocrusts Influence Vascular Plant Community Development, Promoting Native Plant Dominance. Front. Ecol. Evol. 10:840324. doi: 10.3389/fevo.2022.840324
Received: 21 December 2021; Accepted: 07 February 2022;
Published: 01 March 2022.
Edited by:
David Jack Coates, Department of Biodiversity, Conservation and Attractions (DBCA), AustraliaReviewed by:
Dario Liberati, University of Tuscia, ItalyStefan Siebert, North-West University, South Africa
Copyright © 2022 Bowker, Doherty, Antoninka, Ramsey, DuPre and Durham. This is an open-access article distributed under the terms of the Creative Commons Attribution License (CC BY). The use, distribution or reproduction in other forums is permitted, provided the original author(s) and the copyright owner(s) are credited and that the original publication in this journal is cited, in accordance with accepted academic practice. No use, distribution or reproduction is permitted which does not comply with these terms.
*Correspondence: Matthew A. Bowker, bWF0dGhldy5ib3drZXJAbmF1LmVkdQ==