- Department of Entomology, Texas A&M University, College Station, TX, United States
Social insect colonies are characterized by an efficient division of labor, allowing high-value individuals (i.e., reproductives and brood) to be sheltered from tasks associated with increased risk of pathogen exposure, such as foraging or corpse disposal. This social organization helps limit the transmission of disease throughout the colony. Further, individuals can actively respond to imminent disease threats by altering their behaviors as a means of social immunity. In subterranean termites, although workers typically avoid detected pathogens, they can be attracted to pathogen cues when a nestmate is infected. Infected termites are usually groomed, but they may instead be cannibalized if the infection has already become lethal. The mechanisms governing these changes in behavior are unclear. We set out to examine immediate changes in individual behaviors, investigating the role that the infected individual plays in communicating its infection status to nestmates. We also assessed gradual changes in social organization after the re-introduction of an infected termite to the colony. Our results reveal that infected termites likely do not signal their infection status to nestmates through shaking behaviors and reduced movements, suggesting the occurrence of other mechanisms used in communicating infection. We also found that infected termites do not self-isolate and may travel to the densest part of the colony, where they can potentially benefit from grooming by large groups of nestmates. These results provide new insights into how individual changes in immune behaviors contribute to overall colony health, highlighting that, at early stages of infection, termites favor a rescuing strategy rather than isolation and/or cannibalization.
Introduction
The evolution of eusociality in animals has led to tremendous ecological success. This has reached a paradigm in social insects, which have rapidly diversified throughout different ecological niches (Wilson, 1990). However, living in large groups may entail several costs, especially in densely packed groups of closely related individuals. Disease can spread more easily as frequent interactions increase the chance of transmission (Hamilton, 1987; Schmid-Hempel, 1998; Godfrey et al., 2006; Brahma et al., 2021; Schmid-Hempel, 2021) and related individuals are more likely to suffer from the same disease agent (Anderson and May, 1985; Shykoff and Schmid-Hempel, 1991; Nunn et al., 2006). Yet, social insect colonies are well protected against epidemic outbreaks, due to a variety of individual- and group-level defenses, such as allogrooming (Peng et al., 1987; Drees et al., 1992; Oi and Pereira, 1993; Rosengaus et al., 1998b; Hughes et al., 2002; Wilson-Rich et al., 2007; Yanagawa and Shimizu, 2007; Chouvenc et al., 2009b; Liu et al., 2019a; Cini et al., 2020), the transfer of antimicrobial substances through trophallaxis (Hamilton et al., 2011b), and corpse disposal (Sun and Zhou, 2013). These defenses, collectively referred to as social immunity, take advantage of an organized workforce in order to mitigate the costs of social living (Cremer et al., 2007; Cremer et al., 2018; Liu et al., 2019b).
Division of labor is the hallmark of social insect colonies, whereby individuals are allocated to different colony tasks. Within a colony, reproduction is the responsibility of one, or a few, individuals, while a larger workforce tends to all other needs. This social organization allows the reproductive caste to be protected from external threats, as some tasks, such as foraging, defense or corpse disposal, increase the risk of pathogen exposure (Durrer and Schmid-Hempel, 1994; Sun and Zhou, 2013; Stroeymeyt et al., 2014; Sah et al., 2018). Typically, colony members responsible for these risky tasks have reduced contact with high-value members of the colony (i.e., reproductives and brood), thus decreasing the chance that these valuable individuals will become infected (Wang and Mofller, 1970; Naug and Camazine, 2002; Naug and Smith, 2007; Stroeymeyt et al., 2018; Guo et al., 2020). Even within the worker caste, particularly dangerous tasks are handled by more expendable individuals. Many social insects exhibit age polyethism, such that younger individuals work inside the nest while older workers are responsible for more hazardous tasks (Seeley, 1982; Schmid-Hempel and Schmid-Hempel, 1984; Sun and Zhou, 2013; Natsopoulou et al., 2016). This serves to prolong the life of workers, and thus maximizes their contributions to the colony. Division of labor improves social immunity by constraining disease transmission throughout the colony.
While the organization of the colony workforce provides a measure of passive immune defense, individuals still actively respond to imminent disease threats. Selective pressures associated with disease are thought to have played a large role in the evolution of eusociality (Gadagkar, 1992). Consequently, many social insects are acutely sensitive to pathogen cues (Schmid-Hempel, 1998; Hussain et al., 2010; Tranter et al., 2014; Yanagawa et al., 2015; Cappa et al., 2019; Almeida et al., 2022) and, in some cases, can even discern the degree of pathogen virulence (Yanagawa et al., 2012). This strong detection ability allows social insects to adjust their behavior to reduce disease transmission risk. In several species of ants and bees, infected and contagious individuals self-isolate themselves, either by reducing their contact with nestmates or by leaving the nest entirely (Walker and Hughes, 2009; Chapuisat, 2010; Heinze and Walter, 2010; Bos et al., 2012; Stroeymeyt et al., 2018; Geffre et al., 2020; Alciatore et al., 2021).
In termites, the typical response to pathogen cues is avoidance or shaking alarm displays to warn nestmates (Rosengaus et al., 1999; Yanagawa et al., 2015; Bulmer et al., 2019). Shaking behaviors comprise an assortment of vibratory signals transmitted through the substrate and perceived by nestmates. They represent an effective form of communication in termites, especially in subterranean environments where visual and odorant signals can be less effective (Hill, 2009). Termites are therefore able to transmit different signals using shaking behavior, such as alarm signaling, caste identification and reproductive regulation (Eyer et al., 2021; Pailler et al., 2021). In addition, termites can exhibit different behaviors toward infected nestmates. Termites rely heavily on allogrooming to remove pathogenic spores from other workers (Rosengaus et al., 1998b; Chouvenc et al., 2009b; Davis et al., 2018; Liu et al., 2019a; Aguero et al., 2020). Although workers are typically repelled by olfactory pathogen cues, they may be attracted if those odors are presented alongside nestmate cues (Yanagawa et al., 2015). In some cases, workers will prevent the infected individuals from returning to the colony by sealing them inside a chamber (Epsky and Capinera, 1988). In the subterranean termite, Reticulitermes flavipes, when workers have incubated a lethal infection, the typical grooming response is replaced by cannibalization (Davis et al., 2018). It is currently unclear how termites determine when nestmates have developed lethal infections and can no longer be saved by grooming. If the infected individual is responsible for communicating its infection status, it may do so by either increased shaking alarms or reduced movement. A lack of movement, or moribundity, has frequently been observed in diseased termites, but it is unknown if this is a signal meant to communicate with nestmates or just a symptom of disease (Chouvenc et al., 2009b; Davis et al., 2018).
We set out to determine if pathogen-exposed workers of R. flavipes alter their behavior in the presence of nestmates. We examined immediate behavioral changes by measuring locomotion and shaking displays before and after reintroduction to a small group of nestmates. Then, we investigated gradual changes in behavior when infected workers return to a larger group within a nest. We used a fungal entomopathogen to infect termites and test if different incubation times affect these behavioral changes. Overall, our results assessed how individual immune behaviors relate to social immunity.
Methodology
Termite and Pathogen Preparation
In November 2019, groups of termites were collected from eight R. flavipes colonies in College Station, TX, United States. Collection points were located at least 15 m apart from each other to ensure that each group of termites came from a different colony (Vargo, 2003; DeHeer and Vargo, 2004; DeHeer et al., 2005). For each colony, some termites were dyed blue, so that they could be identified among undyed nestmates. Those termites were fed for 1 week on cellulose material containing Nile blue dye (Sigma, St. Louis, MO, United States), which is a fat-soluble stain commonly used to mark termites (Su, 1991; Davis et al., 2018; Aguero et al., 2020). Once the termites were dyed, pathogenic treatments were prepared from the conidia of the fungal pathogen, Metarhizium robertsii, suspended in a 0.1% TWEEN® 80 solution (Sigma-Aldrich Chemie N.V, Netherlands) at a concentration of 1 × 107 conidia/mL (Aguero et al., 2021a). A similar preparation of this fungal strain at the same concentration resulted in successful infection and reduced survival compared to controls in R. flavipes (Aguero et al., 2021a). The spores of Metarhizium usually germinate and the hyphae that emerge penetrate the cuticle in the first 12–24 h. The fungus further develops inside the host body in the next couple of days, and usually kills the insect after 3–6 days (Schrank and Vainstein, 2010). The 0.1% TWEEN® 80 solution by itself was used as a control treatment. Individual termites were treated by 30 s of immersion in 0.5 mL in either a pathogen or a control solution. Treated individuals were individually moved to 60 mm diameter petri dishes lined with moistened filter paper and allowed to incubate for 15 min, 24 h, or 48 h. Experiments were started after the appropriate incubation time.
Immediate Changes in Behaviors
After incubation, a five-minute video of the Petri dish was recorded. Six total treatments were run (15-min incubation control, 24-h incubation control, 48-h incubation control, 15-min pathogen incubation, 24-h pathogen incubation, 48-h pathogen incubation). One worker was observed per treatment and two replicates were conducted for each of the four colonies (LB3, LC1, LC2, and LC5). Locomotion (i.e., time spent moving) and the number of shaking events of each individual termite were counted through blind observation by the same individual researcher. After 15 min, four undyed workers from the same colony were added to each Petri dish. In order to reduce the effects of this disturbance, an additional 15 min were allowed to pass before recording another five-minute video. Locomotion and number of shaking events of the focal termites were counted.
A single zero-altered negative binomial model (ZANB) (Zeileis et al., 2008) was used to determine the effects of both treatment and the presence of nestmate workers on immediate changes in locomotive behavior. The effect of the presence of nestmate workers on locomotion was also tested separately using a paired Wilcoxon test. A different zero-altered negative binomial model was used to test whether individual termites changed their shaking behavior once nestmate termites were added. This change in shaking behavior was also tested using a paired Wilcoxon test. However, the overall low number of shaking events was not sufficient to test for a potential effect of treatment.
Gradual Changes to Behaviors
Groups of 500 workers and five soldiers from each of four colonies (LB02, LB04, LB07, and LB08) were introduced into 25 cm × 25 cm planar arenas filled with moistened sand (Figure 1, similar to Chouvenc et al., 2011). The planar arenas contain a single entrance container connected to the arena through a small plastic tube. The side of the arena opposite to the entrance was lined with discs of filter paper, serving as food material on which the colony can establish its main chamber. Each plate was divided into four levels (A-D), so that observers could identify individuals in different areas of the arena (Figure 1). Termites were introduced through the entrance and allowed to tunnel through the plate for 1 month, which was enough time for the colony to settle and form stable tunnels and chambers. Unfortunately, the colonoids used in this study did not produce secondary reproductives after this one-month period, so there were no reproductives present in the planar arenas during the experiment. The entrance container was continuously supplied with filter paper (i.e., food source), so that foraging workers could be repeatedly collected and returned (which is impossible in the different levels of the plate arena).
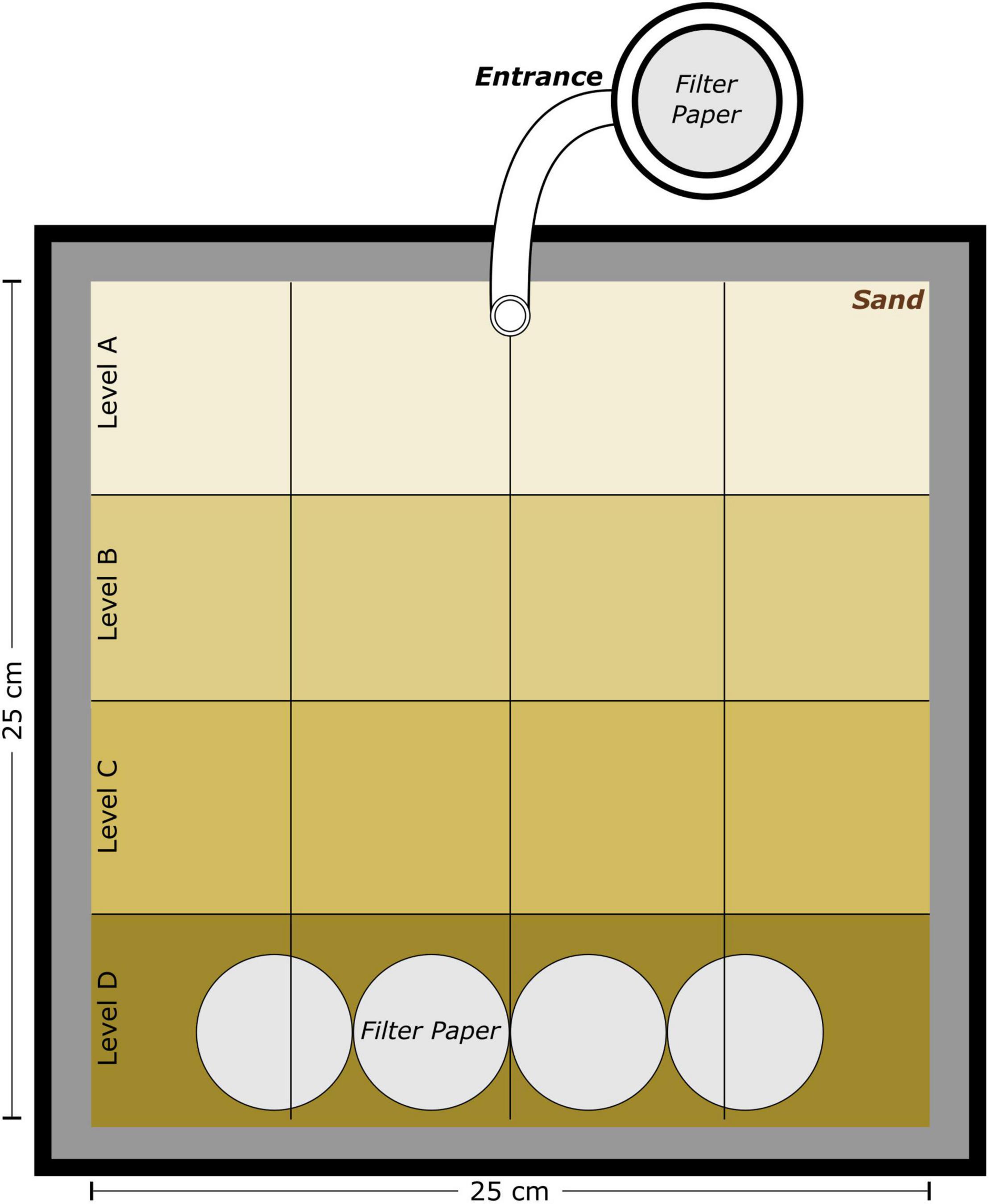
Figure 1. Diagram of planar arenas. 500 workers and five soldiers from each colony were placed into the arena entrance, which leads into a 25 cm × 25 cm planar arena filled with moistened sand. The entrance was continuously supplied with filter paper, so that foraging workers could be repeatedly collected and returned. Each arena was divided into four levels (A-D). Level D contained filter paper discs that served as a food material on which the colony could establish its main chamber.
Four total treatments were run (24-h incubation control, 48-h incubation control, 24-h pathogen incubation, 48-h pathogen incubation). Each treatment was replicated by using 2–3 different colonies, resulting in a total of nine experimental replicates. All treatments were run simultaneously. After the colonies had settled for the one-month period, five workers were removed from the entrance of each plate. These workers were fed cellulose material containing Nile blue for 1 week then treated with either a pathogen or control solution. Treated workers were left to incubate for either 24 or 48 h before being reintroduced to the entrance container of the arenas. In order to assess changes in social organization, the number of nestmates in each level was counted through blind observation by the same individual researcher at 15 min before the reintroduction of treated individuals, as well as at 15 min, 1 day, and 7 days after their reintroduction. In order to assess potential self-isolation, the number of dyed termites (i.e., infected or control treated) was also counted in each level at 15 min, 1 day, and 7 days after their reintroduction. To ease counting, each level was further split into 4 grid squares, which were later summed.
To analyze changes in social organization of the colonies, the distribution of nestmate workers among different levels was investigated using Fisher’s exact tests for each treatment. We compared the distribution of nestmate workers found in different levels at each observation time to levels before the reintroduction of dyed termites (i.e., infected or control treated). To assess potential self-isolation, U-statistics permutation (USP) tests of independence (Berrett and Samworth, 2021) were used to determine if the number of dyed individuals differed between levels of the colonies. Different USP tests were performed separately for each treatment. Finally, two global Fisher tests (one for control data and one for pathogen data) were applied after combining data for the different observation times and incubation times. These tests were used to determine whether the dyed individuals (either control-treated or pathogen treated) were similarly distributed among levels compared to their nestmate workers. All analyses were performed in the statistical software R Studio Version 1.4.1717 (R Core Team, 2022).
Results
When examining immediate changes to behavior, neither treatment (ZANB, p = 1.0 and p = 0.529–0.936 for zero-value and count data respectively) nor the presence of nestmate termites (ZANB, p = 0.70 and p = 0.056 for zero-value and count data respectively) were found to have a significant effect on immediate changes in locomotion when both of them were analyzed together (ZANB; Figure 2A). Consistently, a similar result was found when the presence of nestmate termites was investigated separately across all treatments, with no significant difference in locomotion before or after nestmate termites were added (Wilcoxon test, p = 0.065). However, there was a significant difference in locomotion before and after nestmate termites were added in the 15-min pathogen incubation treatment (Wilcoxon test, p = 0.040). Interestingly, a similar reduction in locomotion before and after nestmate termites were added was also observed in the 15-min incubation control treatment, despite not being significant (Wilcoxon test, p = 0.092). Additionally, the presence of nestmate termites did not significantly influence the number of shaking events observed using both the ZANB (p = 0.591 and p = 0.411 for both zero-value and count data respectively) and Wilcoxon test (p = 0.670; Figure 2B).
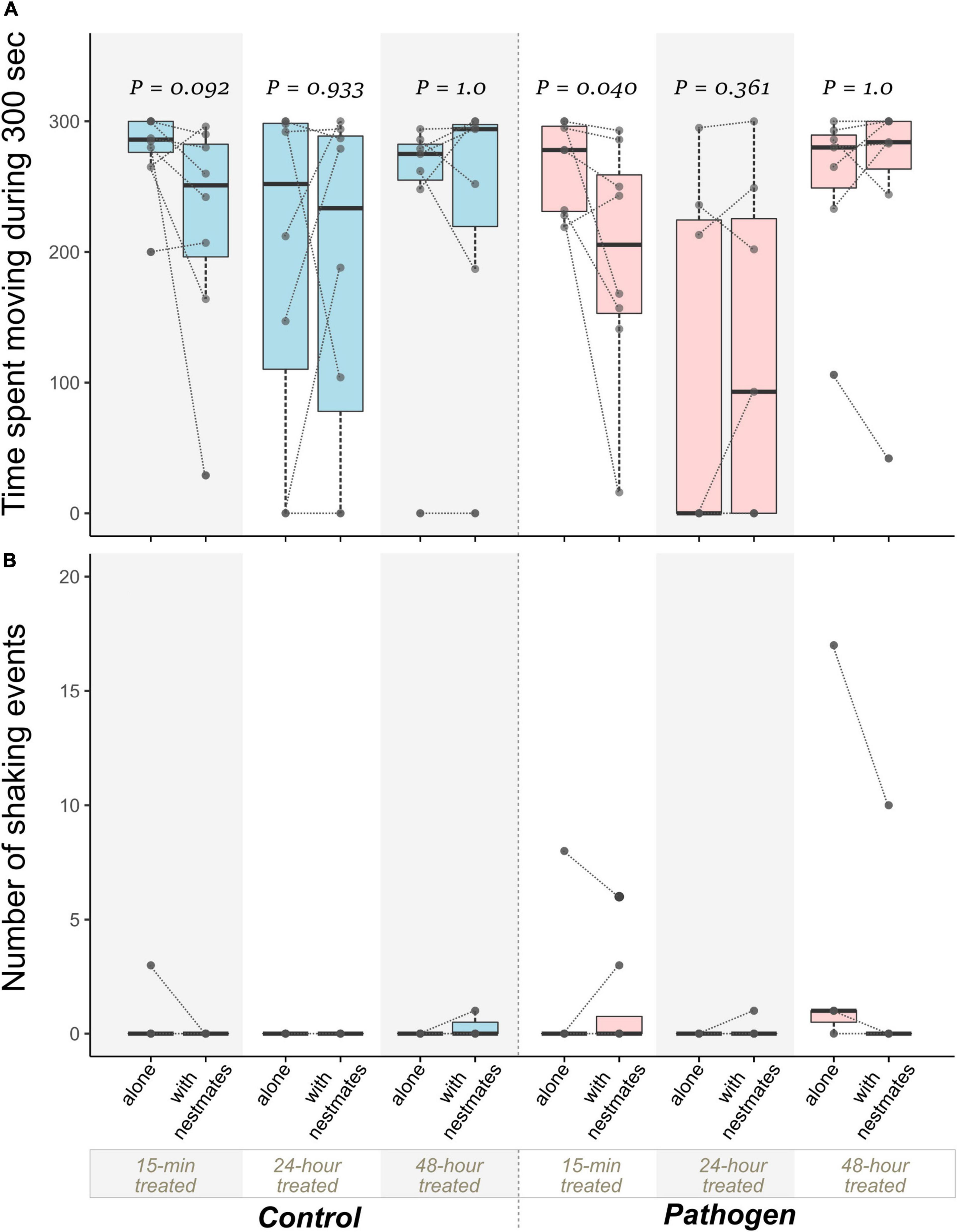
Figure 2. Immediate changes in immune behaviors. Individual data points were connected with a line to illustrate the behavioral change of each individual. (A) Overall, treatment did not have a significant effect on immediate changes in locomotion (Wilcoxon test, p = 0.065). Reduced locomotion was observed shortly after nestmate workers were added in the 15-min incubation periods. For all other incubation periods, pathogen-exposed workers generally did not alter their locomotion in the presence of nestmate workers (n = 4 colonies). (B) The presence of nestmate workers did not significantly influence the number of shaking events (n = 4 colonies).
First, gradual changes within colonies after reintroduction of dyed termites were investigated through changes in social organization (i.e., abundance and distribution of nestmates among the different levels). Regardless of treatment, colony density concentrated away from the entrance of the plates, as workers were significantly more abundant in level D (60–80% of nestmate workers), suggesting this level represented the center of the colony (Figure 3). Similar patterns of worker abundance among levels were observed before and after reintroduction of control-treated or pathogen-treated individuals for the different observation times. No significant change in the distribution of nestmate termites was observed at any time after reintroduction of dyed termites compared to nestmate distribution before reintroduction (All Fisher’s exact tests, p = 1; Figure 4). These results were observed for both incubation times of control treatment and both incubation times of pathogen treatment.
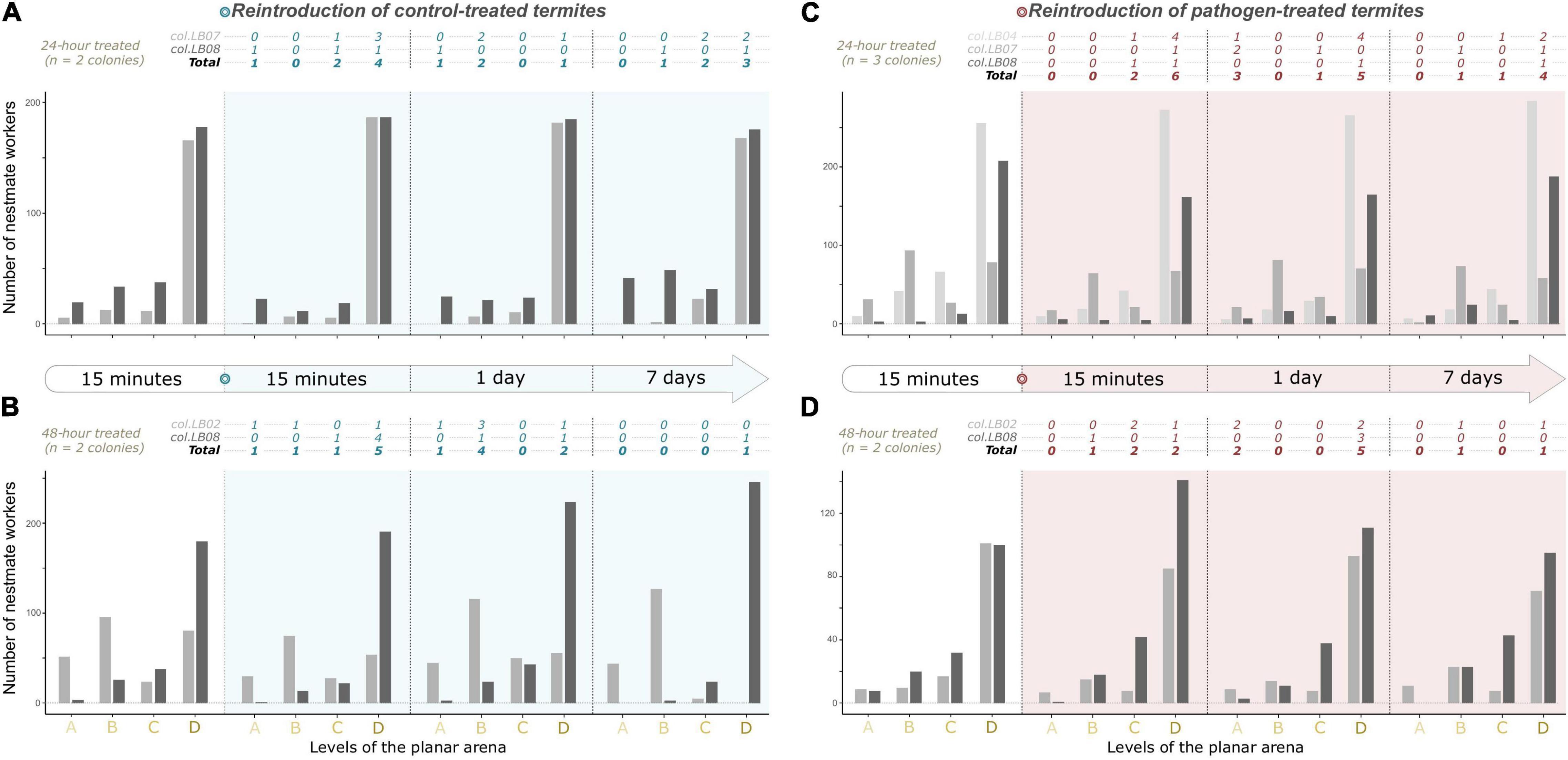
Figure 3. Gradual changes in immune behaviors. Barplots depict the number of nestmate workers found in each level over time for the (A) 24-h incubation control, (B) 48-h incubation control, (C) 24-h pathogen incubation, and (D) 48-h pathogen incubation. N in each plot indicates the number of colonies tested for each treatment. The single dot on the observation time scale represents when the focal individuals were added (i.e., control-treated or pathogen treated). Regardless of treatment, colony density concentrated away from the entrance of the plates, as workers were significantly more abundant in level D (60–80% of nestmate workers). The rows of numbers on top of each barplot represent the number of dyed individuals (i.e., control-treated or pathogen treated) found in each level per colony. The bottom row is the summed total.
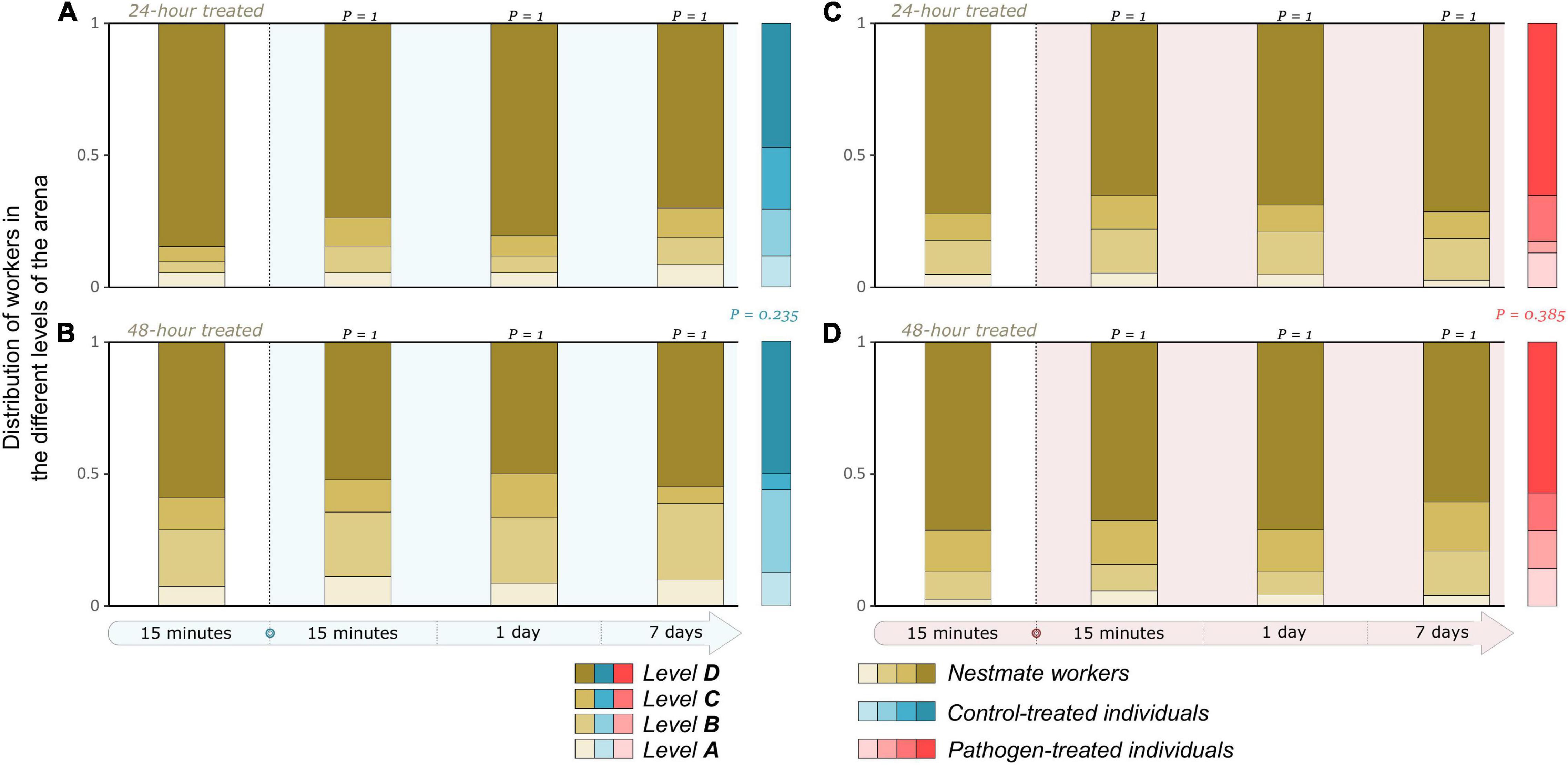
Figure 4. Distribution of nestmate workers and focal individuals per level. Barplots represent the distribution of nestmate workers in different levels of the arena over time for the (A) 24-h incubation control, (B) 48-h incubation control, (C) 24-h pathogen incubation, and (D) 48-h pathogen incubation. The single dot on the observation time scale represents when the focal individuals were added (i.e., control-treated or pathogen treated). No significant difference was observed when the distribution of nestmate workers per observation time was compared separately to the distribution of nestmate works before focal individuals were added (Fisher tests, all p = 1). For each incubation time, colored barplots represent the overall distribution of focal individuals (i.e., combining observation times) among the different levels of the colonies from both the control and pathogen treatments. The overall distribution of dyed individuals closely mirrored the distribution of nestmate workers for both control (p = 0.235) and pathogen treatments (p = 0.385).
Second, gradual changes within colonies were investigated through potential self-isolation of infected termites. These results should be taken with caution due to the small sample size and associated observational difficulty. The count of dyed termites did not always match the number of reintroduced individuals. These discrepancies may have resulted from the death of infected workers or some being cannibalized or buried by nestmates. However, these discrepancies also occurred midway through the experiment and even for the control treatment. This suggests that missing termites may also be the result of the dye fading over time or termites hiding within tunnels or underneath the filter paper. After their reintroduction, the number of dyed individuals did not significantly differ between levels when examining each treatment separately (All USP tests, p > 0.05). This result was observed for both incubation times of control treatment (p = 0.508 and p = 0.158 for 24 h-treated and 48 h-treated individuals, respectively) and both incubation times of pathogen treatment (p = 0.326 and p = 0.177 for 24 h-treated and 48 h-treated individuals, respectively) (Figure 3). When incubation times and observation times were combined, the overall distribution of dyed individuals closely mirrored the distribution of nestmate workers for both control (p = 0.235) and pathogen treatments (p = 0.385; Figure 4).
Discussion
We found that pathogen-exposed workers generally did not alter their locomotion or shaking behaviors in the presence of nestmates, regardless of the incubation time up to 48 h. We found reduced locomotion of isolated termites (control-treated and pathogen-treated individuals) shortly after nestmate workers were added in the 15-min incubation treatments. This behavioral change is probably used as a preventive inspection and grooming of reintegrated foragers.
Throughout the experiment, we found that the majority of the nestmate workers congregated within level D, which therefore represented the center of the colony. Interestingly, after reintroduction, the distribution of both control-treated and pathogen-treated individuals closely mirrored the distribution of their nestmates, indicating that they do not self-isolate after isolation or infection. In addition, the distribution of nestmate workers did not differ before and after the reintegration of infected workers. Overall, these results suggest that termites readily accept and care for infected individuals, generally adopting a rescuing strategy toward infected nestmates at the risk of an increased chance of spreading disease.
Although moving into dense areas of the colony may promote the spread of disease, it also increases the chance of being groomed by nestmates. Termites can self-groom, but it is much more efficient to be groomed by others (Rosengaus et al., 1998b; Yanagawa and Shimizu, 2007; Chouvenc et al., 2009b; Liu et al., 2019a). Thus, the cost for a colony to risk spreading disease by grooming, appears to be outweighed by the benefit of rescuing workers before they develop a lethal infection (Davis et al., 2018). Interestingly, similar results have been found in the clonal raider ant, where infected individuals are reintegrated within colonies and occupied a central place in the network of interactions among nestmates, leading to a general increase in physical contact toward infected individuals (Alciatore et al., 2021). Our findings also suggest that, similarly to this ant species, R. flavipes termites favor a general rescuing strategy toward infected nestmates, rather than avoidance. Additionally, termite nest material exhibits strong antimicrobial activity from feces (Rosengaus et al., 1998a), defensive salivary secretions (Bulmer et al., 2010; Hamilton et al., 2011a), and beneficial bacteria (Chouvenc et al., 2018; Aguero et al., 2021b). By traveling to more active parts of the nest, infected individuals may also be seeking areas with the strongest antimicrobial activity and may sanitize themselves in the process.
Even when a termite has developed a lethal infection and can no longer be saved, they do not become infective until the fungus has killed them and sporulates from their body. By seeking out nestmates, workers could be inviting cannibalistic responses for the safe disposal of their bodies before they can become infective. The termite gut serves an important role in termite immunity, as harmful spores are inhibited in the alimentary tract (Chouvenc et al., 2009b). Indeed, when an infected worker may no longer be saved from infection, the response of nestmates switches from grooming to cannibalization (Davis et al., 2018). Reduced movement has been suggested as the cue for nestmates to begin cannibalizing them (Chouvenc et al., 2009a; Davis et al., 2018). Additionally, shaking displays are used to signal pathogen presence and may also play a role in communicating infection status (Rosengaus et al., 1999; Yanagawa et al., 2015; Bulmer et al., 2019). We found no consistent changes in locomotion or shaking displays throughout observation times when infected termites were grouped with nestmates, which suggests that these behaviors are not used by workers to signal this infection to nestmates. These results were found under our experimental setup using early infected individuals; however, it is possible that changes in locomotion or shaking displays would be observed at later stages of the infection process, or that the dye used may influence shaking behavior. Nile blue was originally used to monitor termite colonies in the field and reportedly does not affect mortality, but it is unclear if it may affect behavior (Su, 1991). Additionally, further testing on a larger scale is necessary. However, reduced locomotion was observed after nestmate termites were added for the 15-min pathogen incubation treatment. Interestingly, this pattern was observed for both control and pathogen treated individuals held for a 15-min incubation period, although not significant for the control-treated group. The decrease in movement once nestmate workers were introduced may be attributed to nestmate worker inspection and grooming that naturally occurs once termites are faced with new individuals (Costa-Leonardo and Haifig, 2014). The occurrence of this pattern for both control-treated and pathogen-treated individuals suggests that isolation from the colony alone may already trigger this behavioral change, which may be used as a preventive inspection of reintegrated foragers. Potentially, if reduced locomotion does not rely on the presence of nestmates, then it may be a symptom of deteriorating health. This difference may not be possible to discern from behavioral studies and may require a more thorough analysis of physiological, chemical or transcriptional changes in infected individuals. For example, infected individuals of bees and ants exhibit change in cuticular hydrocarbons (CHCs) profiles suggesting their potential role in signaling immune status (Richard et al., 2008; Baracchi et al., 2012; Cappa et al., 2016; López et al., 2017; Pull et al., 2018; Cappa et al., 2019).
Behavioral changes after infection are not always the result of social immune defenses. Several pathogens can manipulate their host’s behavior to benefit their own transmission. More complex examples of manipulation appear to require a degree of host specialization (Lafferty and Shaw, 2013). Carpenter ants infected with Ophiocordyceps fungi descend from arboreal nests to find optimal conditions for fungal growth (Hughes et al., 2011). Similarly, in honeybees, workers infected with Israeli acute paralysis virus show decreased aggression toward other colonies, increasing the chance of transmission to new hosts (Geffre et al., 2020). A reduction in movement is one of the most common examples of host manipulation, as it increases the chance of the current host being predated and further transmitting the disease (Lafferty and Shaw, 2013). If this is the case, the inhibitory strength of the termite gut may have accordingly evolved to prevent pathogen transmission during cannibalization (Chouvenc et al., 2009b).
We show that R. flavipes workers that are infected with a fungal pathogen do not self-isolate from the colony in the planar arenas. However, subterranean termite colonies and their spatial organization can be much more complex in nature. Foraging ranges can extend hundreds of square meters and mature colonies typically contain orders of magnitude more than the 500 individuals we used in our arenas (Vargo and Husseneder, 2009; Shults et al., 2021). While infected termites are uninhibited from dense pockets of workers, it is unknown if the same is true for reproductive chambers. Additionally, the concentration of pathogenic spores used in this study is much higher than what termites are expected to encounter naturally (Chouvenc et al., 2011; Loreto and Hughes, 2016). Lower pathogen titers are typically not used in studies of social immunity, as differences in colony survival are less likely to be seen. Potentially, termite workers may behave differently when exposed to less lethal pathogen concentrations. Expanding on these results by altering pathogen titers and tracking colony interactions on a smaller scale will increase our understanding of how individual behaviors translate to overall colony organization. Similarly, testing the reintegration of infected individuals at later stages of infection will allow us to tease apart whether infected and infectious individuals exhibit or elicit different behavioral responses. Finally, studying the connectivity network between every individual in the colony would permit testing whether the unrestrained contact of infected individuals also apply to highly valuable reproductive individuals and more susceptible juvenile individuals (Cole et al., 2020).
Data Availability Statement
The raw data supporting the conclusions of this article is available on Open Science Framework (DOI: 10.17605/OSF.IO/6NDC9).
Author Contributions
CA and EV: conceptualization. CA: data curation and methodology. MM and CA: formal analysis, investigation, and validation. EV: funding acquisition, project administration, and supervision. MM, CA, and P-AE: visualization. MM, CA, P-AE, and EV: writing—original draft. All authors have read and agreed to the published version of the manuscript.
Funding
This research was funded by the Urban Entomology Endowment at Texas A&M University.
Conflict of Interest
The authors declare that the research was conducted in the absence of any commercial or financial relationships that could be construed as a potential conflict of interest.
Publisher’s Note
All claims expressed in this article are solely those of the authors and do not necessarily represent those of their affiliated organizations, or those of the publisher, the editors and the reviewers. Any product that may be evaluated in this article, or claim that may be made by its manufacturer, is not guaranteed or endorsed by the publisher.
References
Aguero, C. M., Eyer, P.-A., and Vargo, E. L. (2020). Increased genetic diversity from colony merging in termites does not improve survival against a fungal pathogen. Sci. Rep. 10:4212. doi: 10.1038/s41598-020-61278-7
Aguero, C. M., Eyer, P.-A., Martin, J., Bulmer, M. S., and Vargo, E. L. (2021a). Natural variation in a subterranean termite’s individual immunity is not related to colony inbreeding. Ecol. Evol. 11, 3072–3083. doi: 10.1002/ece3.7233
Aguero, C. M., Eyer, P.-A., Crippen, T. L., and Vargo, E. L. (2021b). Reduced environmental microbial diversity on the cuticle and in the galleries of a subterranean termite compared to surrounding soil. Microb. Ecol. 81, 1054–1063. doi: 10.1007/s00248-020-01664-w
Alciatore, G., Ugelvig, L. V., Frank, E., Bidaux, J., Gal, A., Schmitt, T., et al. (2021). Immune challenges increase network centrality in a queenless ant. Proc. R. Soc. B. 288:20211456. doi: 10.1098/rspb.2021.1456
Almeida, F. C. R., Magalhães, D. M., Favaris, A. P., Rodríguez, J., Azevedo, K. E. X., Bento, J. M. S., et al. (2022). Side effects of a fungus-based biopesticide on stingless bee guarding behaviour. Chemosphere 287, 132–147. doi: 10.1016/j.chemosphere.2021.132147
Anderson, R. M., and May, R. M. (1985). Vaccination and herd immunity to infectious diseases. Nature 318, 323–329. doi: 10.1038/318323a0
Baracchi, D., Fadda, A., and Turillazzi, S. (2012). Evidence for antiseptic behaviour towards sick adult bees in honey bee colonies. J. Insect Physiol. 58, 1589–1596. doi: 10.1016/j.jinsphys.2012.09.014
Berrett, T. B., and Samworth, R. J. (2021). USP: an independence test that improves on Pearson’s chi-squared and the G-test. Proc. R. Soc. A. 477:20210549. doi: 10.1098/rspa.2021.0549
Bos, N., Lefèvre, T., Jensen, A., and d’Ettorre, P. (2012). Sick ants become unsociable. J. Evol. Biol. 25, 342–351.
Brahma, A., Leon, R. G., Hernandez, G. L., and Wurm, Y. (2021). Larger, more connected societies of ants have a higher prevalence of viruses. Mol. Ecol. 31, 859–865. doi: 10.1111/j.1420-9101.2011.02425.x
Bulmer, M. S., Franco, B. A., and Fields, E. G. (2019). Subterranean termite social alarm and hygienic responses to fungal pathogens. Insects 10:240. doi: 10.3390/insects10080240
Bulmer, M., Lay, F., and Hamilton, C. (2010). Adaptive evolution in subterranean termite antifungal peptides. Insect Mol. Biol. 19, 669–674. doi: 10.1111/j.1365-2583.2010.01023.x
Cappa, F., Bruschini, C., Protti, I., Turillazzi, S., and Cervo, R. (2016). Bee guards detect foreign foragers with cuticular chemical profiles altered by phoretic varroa mites. J. Apic. Res. 55, 268–277. doi: 10.1080/00218839.2016.1229886
Cappa, F., Petrocelli, I., Dani, F. R., Dapporto, L., Giovannini, M., Silva-Castellari, J., et al. (2019). Natural biocide disrupts nestmate recognition in honeybees. Sci. Rep. 9:3171. doi: 10.1038/s41598-019-38963-3
Chapuisat, M. (2010). Social evolution: sick ants face death alone. Curr. Biol. 20, R104–R105. doi: 10.1016/j.cub.2009.12.037
Chouvenc, T., Elliott, M. L., Šobotník, J., Efstathion, C. A., and Su, N.-Y. (2018). The termite fecal nest: a framework for the opportunistic acquisition of beneficial soil Streptomyces (Actinomycetales: Streptomycetaceae). Environ. Entomol. 47, 1431–1439. doi: 10.1093/ee/nvy152
Chouvenc, T., Su, N.-Y., and Grace, J. K. (2011). Fifty years of attempted biological control of termites–Analysis of a failure. Biol. Control 59, 69–82. doi: 10.1016/j.biocontrol.2011.06.015
Chouvenc, T., Su, N.-Y., and Robert, A. (2009b). Inhibition of Metarhizium anisopliae in the alimentary tract of the eastern subterranean termite Reticulitermes flavipes. J. Invertebr. Pathol. 101, 130–136. doi: 10.1016/j.jip.2009.04.005
Chouvenc, T., Su, N.-Y., and Robert, A. (2009a). Cellular encapsulation in the eastern subterranean termite, Reticulitermes flavipes (Isoptera), against infection by the entomopathogenic fungus Metarhizium anisopliae. J. Invertebr. Pathol. 101, 234–241. doi: 10.1016/j.jip.2009.05.008
Cini, A., Bordoni, A., Cappa, F., Petrocelli, I., Pitzalis, M., Iovinella, I., et al. (2020). Increased immunocompetence and network centrality of allogroomer workers suggest a link between individual and social immunity in honeybees. Sci. Rep. 10:8928. doi: 10.1038/s41598-020-65780-w
Cole, E. L., Bayne, H., and Rosengaus, R. B. (2020). Young but not defenceless: antifungal activity during embryonic development of a social insect. R. Soc. Open Sci. 7:191418. doi: 10.1098/rsos.191418
Costa-Leonardo, A. M., and Haifig, I. (2014). “Termite communication during different behavioral activities,” in Biocommunication of Animals, ed. G. Witzany (Dordrecht: Springer), doi: 10.1007/978-94-007-7414-8_10
Cremer, S., Armitage, S. A., and Schmid-Hempel, P. (2007). Social immunity. Curr. Biol. 17, R693–R702.
Cremer, S., Pull, C. D., and Fuerst, M. A. (2018). Social immunity: emergence and evolution of colony-level disease protection. Annu. Rev. Entomol. 63, 105–123. doi: 10.1146/annurev-ento-020117-043110
Davis, H. E., Meconcelli, S., Radek, R., and McMahon, D. P. (2018). Termites shape their collective behavioural response based on stage of infection. Sci. Rep. 8:14433. doi: 10.1038/s41598-018-32721-7
DeHeer, C. J., and Vargo, E. L. (2004). Colony genetic organization and colony fusion in the termite Reticulitermes flavipes as revealed by foraging patterns over time and space. Mol. Ecol. 13, 431–441. doi: 10.1046/j.1365-294x.2003.2065.x
DeHeer, C., Kutnik, M., Vargo, E., and Bagneres, A. (2005). The breeding system and population structure of the termite Reticulitermes grassei in Southwestern France. Heredity 95, 408–415. doi: 10.1038/sj.hdy.6800744
Drees, B. M., Miller, R. W., Vinson, B. S., and Georgis, R. (1992). Susceptibility and behavioral response of red imported fire ant (Hymenoptera: Formicidae) to selected entomogenous nematodes (Rhabditida: Steinernematidae & Heterorhabditidae). J. Econ. Entomol. 85, 365–370. doi: 10.1093/jee/85.2.365
Durrer, S., and Schmid-Hempel, P. (1994). Shared use of flowers leads to horizontal pathogen transmission. Proc. R. Soc. B: Biol. Sci. 258, 299–302. doi: 10.1098/rspb.1994.0176
Epsky, N. D., and Capinera, J. L. (1988). Efficacy of the entomogenous nematode Steinernema feltiae against a subterranean termite, Reticulitermes tibialis (Isoptera: Rhinotermitidae). J. Econ. Entomol. 81, 1313–1317. doi: 10.1093/jee/81.5.1313
Eyer, P.-A., Salin, J., Helms, A. M., and Vargo, E. L. (2021). Distinct chemical blends produced by different reproductive castes in the subterranean termite Reticulitermes flavipes. Sci. Rep. 11:4471. doi: 10.1038/s41598-021-83976-6
Geffre, A. C., Gernat, T., Harwood, G. P., Jones, B. M., Gysi, D. M., Hamilton, A. R., et al. (2020). Honey bee virus causes context-dependent changes in host social behavior. PNAS 117, 10406–10413. doi: 10.1073/pnas.2002268117
Godfrey, S. S., Bull, C. M., Murray, K., and Gardner, M. G. (2006). Transmission mode and distribution of parasites among groups of the social lizard Egernia stokesii. Parasitol. Res. 99, 223–230. doi: 10.1007/s00436-005-0120-9
Guo, X., Chen, J., Azizi, A., Fewell, J., and Kang, Y. (2020). Dynamics of social interactions, in the flow of information and diseases preading in social insects colonies: Effects of environmental events and spatial heterogeneity. J. Theor. Biol. 492:110191. doi: 10.1016/j.jtbi.2020.110191
Hamilton, C., Lay, F., and Bulmer, M. S. (2011a). Subterranean termite prophylactic secretions and external antifungal defenses. J. Insect Physiol. 57, 1259–1266. doi: 10.1016/j.jinsphys.2011.05.016
Hamilton, C., Lejeune, B. T., and Rosengaus, R. B. (2011b). Trophallaxis and prophylaxis: social immunity in the carpenter ant Camponotus pennsylvanicus. Biol. Lett. 7, 89–92. doi: 10.1098/rsbl.2010.0466
Hamilton, W. (1987). “Kinship, recognition, disease, and intelligence: constraints of social evolution,” in Animal Societies: Theories and Facts, eds Y. Ito, J. L. Brown, and J. Kikkawa (Tokyo: Japan Scientific Societies Press), 81–100.
Heinze, J., and Walter, B. (2010). Moribund ants leave their nests to die in social isolation. Curr. Biol. 20, 249–252. doi: 10.1016/j.cub.2009.12.031
Hill, P. S. M. (2009). How do animals use substrate-borne vibrations as an information source? Naturwissenschaften 96, 1355–1371. doi: 10.1007/s00114-009-0588-8
Hughes, D. P., Andersen, S. B., Hywel-Jones, N. L., Himaman, W., Billen, J., and Boomsma, J. J. (2011). Behavioral mechanisms and morphological symptoms of zombie ants dying from fungal infection. BMC Ecol. 11:13. doi: 10.1186/1472-6785-11-13
Hughes, W. O., Eilenberg, J., and Boomsma, J. J. (2002). Trade-offs in group living: transmission and disease resistance in leaf-cutting ants. Proc. R. Soc. B: Biol. Sci. 269, 1811–1819. doi: 10.1098/rspb.2002.2113
Hussain, A., Tian, M.-Y., He, Y.-R., Bland, J. M., and Gu, W.-X. (2010). Behavioral and electrophysiological responses of Coptotermes formosanus Shiraki towards entomopathogenic fungal volatiles. Biol. Control 55, 166–173. doi: 10.1016/j.biocontrol.2010.08.009
Lafferty, K. D., and Shaw, J. C. (2013). Comparing mechanisms of host manipulation across host and parasite taxa. J. Exp. Biol. 216, 56–66. doi: 10.1242/jeb.073668
Liu, L., Zhao, X.-Y., Tang, Q.-B., Lei, C.-L., and Huang, Y.-Q. (2019b). The mechanisms of social immunity against fungal infections in eusocial insects. Toxins 11:244. doi: 10.3390/toxins11050244
Liu, L., Wang, W., Liu, Y., Sun, P., Lei, C., and Huang, Q. (2019a). The influence of allogrooming behavior on individual innate immunity in the subterranean termite Reticulitermes chinensis (Isoptera: Rhinotermitidae). J. Insect Sci. 19:6. doi: 10.1093/jisesa/iey119
López, J. H., Riessberger-Gallé, U., Crailsheim, K., and Schuehly, W. (2017). Cuticular hydrocarbon cues of immune-challenged workers elicit immune activation in honeybee queens. Mol. Ecol. 26, 3062–3073. doi: 10.1111/mec.14086
Loreto, R., and Hughes, D. P. (2016). Disease dynamics in ants: a critical review of the ecological relevance of using generalist fungi to study infections in insect societies. Adv. Genet. 94, 287–306. doi: 10.1016/bs.adgen.2015.12.005
Natsopoulou, M. E., McMahon, D. P., and Paxton, R. J. (2016). Parasites modulate within-colony activity and accelerate the temporal polyethism schedule of a social insect, the honey bee. Behav. Ecol. Sociobiol. 70, 1019–1031. doi: 10.1007/s00265-015-2019-5
Naug, D., and Camazine, S. (2002). The role of colony organization on pathogen transmission in social insects. J. Theor. Biol. 215, 427–439. doi: 10.1006/jtbi.2001.2524
Naug, D., and Smith, B. (2007). Experimentally induced change in infectious period affects transmission dynamics in a social group. Proc. R. Soc. B: Biol. Sci. 274, 61–65. doi: 10.1098/rspb.2006.3695
Nunn, C., Altizer, S., and Altizer, S. M. (2006). Infectious Diseases in Primates: Behavior, Ecology and Evolution. Oxford: Oxford University Press.
Oi, D. H., and Pereira, R. M. (1993). Ant behavior and microbial pathogens (Hymenoptera: Formicidae). Fla. Entomol. 76, 63–74. doi: 10.2307/3496014
Pailler, L., Desvignes, S., Ruhland, F., Pineirua, M., and Lucas, C. (2021). Vibratory behaviour produces different vibration patterns in presence of reproductives in a subterranean termite species. Sci. Rep. 11:9902. doi: 10.1038/s41598-021-88292-7
Peng, Y.-S., Fang, Y., Xu, S., and Ge, L. (1987). The resistance mechanism of the Asian honey bee, Apis cerana Fabr., to an ectoparasitic mite, Varroa jacobsoni Oudemans. J. Invertebr. Pathol. 49, 54–60. doi: 10.1016/0022-2011(87)90125-x
Pull, C. D., Ugelvig, L. V., Wiesenhofer, F., Grasse, A. V., Tragust, S., Schmitt, T., et al. (2018). Destructive disinfection of infected brood prevents systemic disease spread in ant colonies. ELife 7:e32073. doi: 10.7554/eLife.32073
Richard, F.-J., Aubert, A., and Grozinger, C. M. (2008). Modulation of social interactions by immune stimulation in honey bee, Apis mellifera, workers. BMC Biol. 6:50. doi: 10.1186/1741-7007-6-50
Rosengaus, R. B., Maxmen, A. B., Coates, L. E., and Traniello, J. F. (1998b). Disease resistance: a benefit of sociality in the dampwood termite Zootermopsis angusticollis (Isoptera: Termopsidae). Behav. Ecol. Sociobiol. 44, 125–134. doi: 10.1007/s002650050523
Rosengaus, R. B., Guldin, M. R., and Traniello, J. F. (1998a). Inhibitory effect of termite fecal pellets on fungal spore germination. J. Chem. Ecol. 24, 1697–1706.
Rosengaus, R. B., Jordan, C., Lefebvre, M. L., and Traniello, J. F. A. (1999). Pathogen alarm behavior in a termite: a new form of communication in social insects. Naturwissenschaften 86, 544–548. doi: 10.1007/s001140050672
Sah, P., Mann, J., and Bansal, S. (2018). Disease implications of animal social network: a synthesis across social systems. J. Anim. Ecol. 87, 546–558. doi: 10.1111/1365-2656.12786
Schmid-Hempel, P. (2021). Sociality and parasite transmission. Behav. Ecol. Sociobiol. 75:156. doi: 10.1007/s00265-021-03092-3
Schmid-Hempel, P., and Schmid-Hempel, R. (1984). Life duration and turnover of foragers in the ant Cataglyphis bicolor (Hymenoptera, Formicidae). Insectes Soc. 31, 345–360. doi: 10.1007/bf02223652
Schrank, A., and Vainstein, M. H. (2010). Metarhizium anisopliae enzymes and toxins. Toxicon 56:1267F1274. doi: 10.1016/j.toxicon.2010.03.008
Seeley, T. D. (1982). Adaptive significance of the age polyethism schedule in honeybee colonies. Behav. Ecol. Sociobiol. 11, 287–293. doi: 10.1007/bf00299306
Shults, P., Richardson, S., Eyer, P.-A., Chura, M., Barreda, H., Davis, R. W., et al. (2021). Area-wide elimination of subterranean termite colonies using a Novaluron bait. Insects 12:192. doi: 10.3390/insects12030192
Shykoff, J. A., and Schmid-Hempel, P. (1991). Parasites and the advantage of genetic variability within social insect colonies. Proc. R. Soc. B: Biol. Sci. 243, 55–58. doi: 10.1098/rspb.1991.0009
Stroeymeyt, N., Casillas-Pérez, B., and Cremer, S. (2014). Organisational immunity in social insects. Curr. Opin. Insect. Sci. 5, 1–15. doi: 10.1016/j.cois.2014.09.001
Stroeymeyt, N., Grasse, A. V., Crespi, A., Mersch, D. P., Cremer, S., and Keller, L. (2018). Social network plasticity decreases disease transmission in a eusocial insect. Science 362, 941–945. doi: 10.1126/science.aat4793
Su, N.-Y. (1991). Evaluation of dye markers for population studies of the eastern and Formosan subterranean termite (Isoptera: Rhinotermitidae). Sociobiology 19, 349–362.
Sun, Q., and Zhou, X. (2013). Corpse management in social insects. Int. J. Biol. 9, 313–321. doi: 10.7150/ijbs.5781
Tranter, C., LeFevre, L., Evison, S. E. F., and Hughes, W. O. H. (2014). Threat detection: contextual recognition and response to parasites by ants. Behav. Ecol. 26, 396–405. doi: 10.1093/beheco/aru203
Vargo, E. L. (2003). Hierarchical analysis of colony and population genetic structure of the eastern subterranean termite, Reticulitermes flavipes, using two classes of molecular markers. Evolution 57, 2805–2818. doi: 10.1111/j.0014-3820.2003.tb01522.x
Vargo, E. L., and Husseneder, C. (2009). Biology of subterranean termites: insights from molecular studies of Reticulitermes and Coptotermes. Annu. Rev. Entomol. 54, 379–403. doi: 10.1146/annurev.ento.54.110807.090443
Walker, T. N., and Hughes, W. O. (2009). Adaptive social immunity in leaf-cutting ants. Biol. Lett. 5, 446–448. doi: 10.1098/rsbl.2009.0107
Wang, D.-I., and Mofller, F. (1970). The division of labor and queen attendance behavior of Nosema-infected worker honey bees. J. Econ. Entomol. 63, 1539–1541. doi: 10.1093/jee/63.5.1539
Wilson, E. O. (1990). Success and Dominance in Ecosystems: The Case of the Social Insects. In Excellence in Ecology. Oldendorf: Ecology Institute.
Wilson-Rich, N., Stuart, R. J., and Rosengaus, R. B. (2007). Susceptibility and behavioral responses of the dampwood termite Zootermopsis angusticollis to the entomopathogenic nematode Steinernema carpocapsae. J. Invertebr. Pathol. 95, 17–25. doi: 10.1016/j.jip.2006.11.004
Yanagawa, A., and Shimizu, S. (2007). Resistance of the termite, Coptotermes formosanus Shiraki to Metarhizium anisopliae due to grooming. BioControl 52, 75–85. doi: 10.1007/s10526-006-9020-x
Yanagawa, A., Fujiwara-Tsujii, N., Akino, T., Yoshimura, T., Yanagawa, T., and Shimizu, S. (2012). Odor aversion and pathogen-removal efficiency in grooming behavior of the termite Coptotermes formosanus. PLoS One 7:e47412. doi: 10.1371/journal.pone.0047412
Yanagawa, A., Imai, T., Akino, T., Toh, Y., and Yoshimura, T. (2015). Olfactory cues from pathogenic fungus affect the direction of motion of termites, Coptotermes formosanus. J. Chem. Ecol. 41, 1118–1126. doi: 10.1007/s10886-015-0649-8
Keywords: Reticulitermes flavipes, social immunity, social insect, Blattodea, rhinotermitidae, Metarhizium
Citation: Moran MN, Aguero CM, Eyer P-A and Vargo EL (2022) Rescue Strategy in a Termite: Workers Exposed to a Fungal Pathogen Are Reintegrated Into the Colony. Front. Ecol. Evol. 10:840223. doi: 10.3389/fevo.2022.840223
Received: 20 December 2021; Accepted: 03 May 2022;
Published: 30 May 2022.
Edited by:
Solange Del Carmen Issa Ponce, Simón Bolívar University, VenezuelaReviewed by:
Federico Cappa, University of Florence, ItalyUlrich Rainer Ernst, University of Hohenheim, Germany
Brian R. Johnson, University of California, Davis, United States
Copyright © 2022 Moran, Aguero, Eyer and Vargo. This is an open-access article distributed under the terms of the Creative Commons Attribution License (CC BY). The use, distribution or reproduction in other forums is permitted, provided the original author(s) and the copyright owner(s) are credited and that the original publication in this journal is cited, in accordance with accepted academic practice. No use, distribution or reproduction is permitted which does not comply with these terms.
*Correspondence: Megan N. Moran, bWVnYW4ubi5tb3Jhbi4xNEBnbWFpbC5jb20=