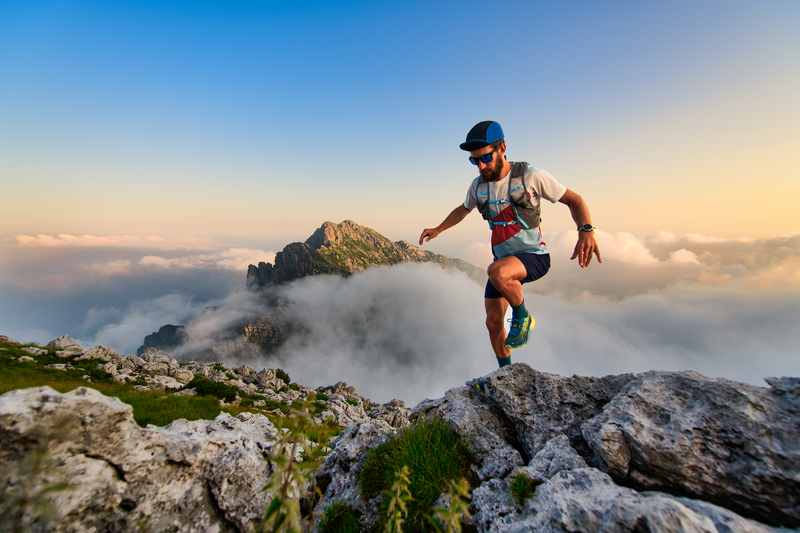
94% of researchers rate our articles as excellent or good
Learn more about the work of our research integrity team to safeguard the quality of each article we publish.
Find out more
ORIGINAL RESEARCH article
Front. Ecol. Evol. , 11 March 2022
Sec. Chemical Ecology
Volume 10 - 2022 | https://doi.org/10.3389/fevo.2022.833088
This article is part of the Research Topic Impacts of Pollution on Volatile-Mediated Interactions View all 5 articles
There is growing evidence to demonstrate that air pollution is affecting invertebrates both directly (e.g., causing physiological stress responses) and indirectly (e.g., via changes in host plant chemistry and/or by disruption of communication by volatile odours). Many of the studies to-date have focused upon winged insects and disruption of in-flight foraging. Therefore, in this study we investigated how the community composition of predominantly ground-dwelling invertebrates in fields of winter wheat are affected by two of the most ubiquitous lower tropospheric air pollutants, diesel exhaust emissions (including nitrogen oxides–NOx) and ozone (O3), both individually and in combination, over 2 years. Pitfall traps, located within the rings of a Free-Air Diesel and Ozone Enrichment (FADOE) facility, were used to sample invertebrates. The facility consisted of eight 8 m-diameter rings, which allowed elevation of the pollutants above ambient levels (ca 49–60 ppb NOx and 35–39 ppb O3) but within levels currently defined as safe for the environment by the Environmental Protection Agency. The invertebrates collected were taxonomically identified and characterised by diet specialisation, mobility and functional group. Taxonomic richness and Shannon’s diversity index were calculated. Even under the relatively low levels of air pollution produced, there were adverse impacts on invertebrate community composition, with greater declines in the abundance and taxonomic richness of invertebrates in the diesel exhaust treatment compared with O3 treatment. In the combined treatment, pollutant levels were lower, most likely because NOx and O3 react with one another, and consequently a lesser negative effect was observed on invertebrate abundance and taxonomic richness. Specialist-feeding and winged invertebrate species appeared to be more sensitive to the impacts of the pollutants, responding more negatively to air pollution treatments than generalist feeders and wingless species, respectively. Therefore, these results suggest a more severe pollution-mediated decline in specialist- compared with generalist-feeding invertebrates, and in more mobile (winged) individuals. Understanding how invertebrate communities respond to air pollutants alone and in combination will facilitate predictions of how terrestrial environments respond to changes in anthropogenic emissions, especially as we shift away from fossil fuel dependence and therefore manipulate the interactions between these two common pollutants.
Insects and other invertebrates provide a myriad of essential ecosystem services (e.g., nutrient cycling, maintenance of soil structure and fertility, pest control, pollination and seed dispersal) that create the biological foundation for all terrestrial ecosystems (Scudder, 2017). However, populations of many species are declining (van Klink et al., 2020; Wagner, 2020) and global invertebrate biodiversity is fundamentally threatened by human activities, such as agricultural intensification, introductions of invasive species and the use of pesticides (IPBES, 2019), which has reinforced interest in mitigating “global insect declines” (Didham et al., 2020). Recent research has proposed a role for common air pollutants, e.g., diesel exhaust [particularly nitrogen oxides—NOx, comprised of nitric oxide (NO) and nitrogen dioxide (NO2)] and ozone (O3), as a further threat to ecosystem function. These pollutants can impair the fitness of invertebrates, both directly via pollution-induced molecular and physiological changes (Leonard et al., 2019; Reitmayer et al., 2019; Thimmegowda et al., 2020; Vanderplanck et al., 2021), and indirectly via changes to the nutritional status of host plants or by chemically altering the odour compounds that invertebrates use for navigation (e.g., to locate plants or invertebrate hosts) and communication (Fuentes et al., 2016; Jamieson et al., 2017; Jürgens and Bischoff, 2017).
Individual invertebrate species and functional groups of those species (e.g., herbivore, pollinator and natural enemy feeding guilds) may differ in their responses to air pollutants depending on the foraging and feeding strategies they employ (McFrederick et al., 2009). Populations of some herbivores, for example, have been found to increase under air pollution (Whittaker, 2001; NECR199, 2016) and others, such as pollinating bees, are predicted to be more likely to decline (Lusebrink et al., 2015; Fuentes et al., 2016; Ryalls et al., 2022). Thus, recent and planned future governmental policy shifts away from the use of fossil fuels could alter the composition of air pollutants, potentially proving advantageous for some invertebrate species and feeding guilds, but disadvantageous for others. Moreover, specialist invertebrates that target an individual species or a few species as hosts or for food (i.e., plant or insect prey) may be more likely to respond to changes in air pollution, compared with generalist species that target multiple hosts. This is because most specialists do not adapt as easily to alternative plant defensive compounds and can thrive only within a narrow range of environmental conditions, compared with generalists that can be more capable of adapting to plant defences and thrive in a wide range of environmental conditions (Agathokleous et al., 2020). Moreover, during foraging, specialists are often dependent on a restricted set of odour stimuli, specific to their host, as opposed to generalists that respond primarily to less specific stimuli (Snyder and Ives, 2003; Holopainen, 2009). Whether specialist species are more likely to be directly affected by air pollution than generalists is unknown. A basic understanding of how air pollution alters invertebrate community composition and functional group (e.g., feeding guild) dynamics would enable us to predict effects of future pollution scenarios on plant–invertebrate systems, which could be used to influence policy to mitigate impacts on ecosystem function (Couture and Lindroth, 2013; Li et al., 2016).
Despite recent incentives to reduce fossil fuel emissions, NOx pollution is predicted to remain a serious problem for decades to come, with many of the latest diesel cars continuing to exceed air pollution limits (Brand, 2016; ACEA, 2019). Globally, increasing urbanisation and traffic congestion is also likely to result in higher NOx in peri-urban and rural areas, increasing the potential exposure of neighbouring agricultural land. Moreover, global tropospheric background O3 concentrations are rising and regular weather-induced episodic increases continue to occur (Hansen et al., 2019; Turnock et al., 2019). Ozone concentrations tend to be higher in rural areas because removal of O3 via its reaction with NOx is comparatively small in comparison to urban areas (Bae et al., 2020). The impact of atmospheric oxidants on invertebrates, and the ecosystem services they provide, is therefore of increasing concern (Li et al., 2016; Papazian and Blande, 2020).
While multiple air pollutants occur in tandem in the natural environment, most studies determining invertebrate responses to air pollution focus on the effects of individual pollutants on behavioural traits and are often confined to small-scale lab-based approaches (e.g., Girling et al., 2013; Farré-Armengol et al., 2016; Khaling et al., 2016; Reitmayer et al., 2019). Thus, field-based evidence of how air pollution impacts invertebrate communities is scarce, a significant oversight considering how important these communities are for the functioning of terrestrial ecosystems. Of the few studies that take a field-based approach, most have considered only one pollutant (Agathokleous et al., 2020) or have been observational (Thimmegowda et al., 2020), making it difficult to disentangle the effects of air pollution from other abiotic and biotic effects. Here, we take a balanced approach with a controlled study under field conditions, facilitating investigation into the effects of air pollution on invertebrates at an ecologically relevant scale.
Using a novel Free-Air Diesel and Ozone Enrichment (FADOE) facility, which emits regulated quantities of diesel exhaust and ozone from a series of 8 m-diameter rings, we investigated the field-scale effects of diesel exhaust (quantified as NO/NO2 concentration, but in reality a complex mix of pollutants including volatile organic compounds and particulates) and O3 pollution on invertebrate community composition in a wheat field after two summer seasons of fumigation. We determined how predominantly ground-active invertebrate feeding guilds and diversity metrics, including taxonomic richness and Shannon diversity index, responded to the individual and combined effects of diesel exhaust and O3. We also determined whether invertebrate responses to these pollutants varied with functional traits, including dietary specialisation (i.e., between specialist and generalist species) and mobility (i.e., between winged and non-winged species).
Full details of the FADOE system configuration are described and visualised in Ryalls et al. (2022). In short, eight FADOE rings (8 m in diameter) were distributed evenly in an octagonal formation within a field of winter wheat (cv. Skyfall sown in November 2017 at a seeding rate of 300 seeds m–2 and a row spacing of 166 mm) at the University of Reading’s Sonning farm, United Kingdom. The centre of each ring was located 50 m from the centre of the field (latitude 51.482853, longitude −0.897749), such that the edges of each ring were separated by a distance of at least 30 m to minimise cross-contamination. Two rings were assigned to each of four pollution treatments, comprising diesel exhaust (D), ozone (O3), diesel exhaust and ozone combined (D + O3) and a control treatment (CON; natural air pumped to each of the rings). Rings of the same treatment were positioned opposite each other in the field to minimise spatial effects. Heavy duty (50 mm ID) conduit connected to vacuum-blower pumps (R4110-2, Gast, United States) extended from one diesel (Hyundai DHY8000SELR 7.2 kVA, Genpower Ltd., United Kingdom) and two ozone (CD1500P, ClearWater Tech, United States) generators in the centre of the field to deliver elevated levels of diesel exhaust and ozone to the rings. Each ring was surrounded by conduit containing 120 (5 mm-diameter) holes to provide a diffuse plume of pollutant (or ambient air in the case of the Control treatment) directed toward the centre of the ring. Mixing ratios of NO, NO2, NOx, and O3 at the centre of each ring were monitored sequentially (every 120 s) via an automated switching system coupled to O3 (Model 49i, Thermo Scientific, United States) and NOx (Model 42C, Thermo Scientific, United States) analysers. Three-way mixing valves (VRG131 connected to ARA600 proportional actuators, ESBE, Sweden) and UV-light controllers (CD1500P 4–20 mA control board) were used to maintain levels of NOx and O3 at field-realistic doses below 120 ppb (based on average concentrations adjacent to major United Kingdom roadways and urban areas; Ares and Smith, 2017) and 90 ppb (based on peak concentrations recorded in rural European sites in 1990–2012; Colette et al., 2016), respectively. The D + O3 treatment rings were maintained at the same maximum concentration as those set for each pollutant ring individually. Winter wheat was re-sown in November 2018 in an adjacent field (latitude 51.482374, longitude −0.895855), where the FADOE facility was reassembled for the second year. Rings were fumigated for at least 10 h day–1 during two summer seasons (April-October 2018 and 2019) and 24 h day–1 during experimental data collection in September 2018 and 2019.
Six 2.5 L pitfall traps (196 mm diameter), evenly distributed within each ring, were dug into the ground, such that the lip of the trap was flush with the surface of the soil. Traps were positioned 1 or 3 m from the centre of the ring in the same orientation within each ring. During each pitfall event (run), traps (N = 48 per run) were opened for 1 week and 500 mL 20% propylene glycol (including detergent used to break the surface tension) was used as a non-toxic invertebrate capture liquid in each trap. Specimens were identified to a taxonomic level that was sufficient to establish feeding guild (i.e., predator, parasitoid, herbivore, fungivore, detritivore or pollinator). Taxa that could not be defined into individual feeding guilds, as well as those that were considered both predators and graminivores were defined as “Various.” Specimens were also characterised by diet specialisation (i.e., generalist or specialist-feeding) and mobility (i.e., winged or not winged). Generalists included invertebrates that consume multiple taxonomic orders and specialists included those that limit their diet to one or two taxonomic orders (Supplementary Table 1). Each pitfall event was run four times in total at the end of the summer season (twice in each of September 2018 and 2019), allowing more than 4 months of fumigation during wheat development prior to the experimental period.
All analyses were performed using the R statistical interface v3.6.3. Linear mixed effect models (LMMs) with a normal error distribution were used to analyse Shannon diversity index, taxonomic richness and pollutant metrics, whereas generalised linear mixed effect models (GLMMs) with a negative binomial distribution were used to analyse abundance metrics. All mixed models were analysed using the R package lme4 (Bates et al., 2014) and dependent variables were transformed, where appropriate. LMMs used to compare NO:NO2, NOx, and O3 mixing ratios between the four pollution treatments included “pollution treatment” as a fixed effect, with “ring location” nested within “year” as random effects to account for repeated measures and spatial differences between rings and years. The effects of air pollution on invertebrate abundance (i.e., the relative number of individuals caught per trap over 7 days) were determined with partial redundancy analysis (pRDA) using the R package “vegan” (Oksanen et al., 2017). pRDA models were run at three resolutions: (i) the most precise taxonomic resolution in the dataset, (ii) order-level (or class/sub-class for some non-insect invertebrates) and (iii) feeding guild. For each model, resolution was a response variable, “pollution treatment” was the explanatory variable, and “year” and “run” were conditioned (partialled-out) covariables. The response variables were Hellinger-transformed, giving low weights to variables with low counts and many zeros. The performance of the pRDAs were determined using adjusted R2 values along with F-statistics and P-values, based on 999 permutations. The vegan package (Oksanen et al., 2017) was used to calculate taxonomic richness and Shannon diversity from the most precise resolution available in the dataset, including specimens identified to levels above species (e.g., family), as described by Staton et al. (2021). The effects of air pollution, diet specialisation and mobility on the abundance of invertebrates that could be classified into specialist or generalist feeders and winged or not winged (mobility) were determined using negative binomial GLMMs with “pollution treatment,” “diet” and “mobility” (and their interactions) as fixed effects and “run” nested within “year” as random effects. For analysis purposes, invertebrates that were short-winged or part-winged (i.e., taxa that are only winged during some life stages or are dimorphic) were classified as winged and the few taxa identified as omnivorous were excluded (Supplementary Table 1). Two control models (negative binomial GLMMs) were used to determine whether changes in abundance under air pollution were driven by taxa capable of active flight (i.e., full-winged taxa). The first control model incorporated treatment and wing presence (i.e., wingless, part-winged or full-winged taxa), and their interaction, as fixed effects. Wingless and part-winged taxa were used as proxies for those that were not capable of active flight. The second control model determined the effects of air pollution on total invertebrate abundance, excluding those that were characterised as full-winged. Both models included “run” nested within “year” as random effects, as above. Post-hoc tests using the package emmeans (Lenth, 2021) were used for pairwise comparisons of means. Salient statistical results are reported in text and figures.
Within the FADOE rings, NOx and O3 mixing ratios (averaging 49–60 and 35–39 ppb, respectively) were maintained well below the maximum target levels (120 and 90 ppb, respectively) that are commonly recorded during natural elevations in the lower troposphere (Colette et al., 2016; Ares and Smith, 2017). Changes in mixing ratios were similar between the complete period (i.e., the entire exposure period over two full summer seasons) and the experimental (pitfall trapping) period. In particular, O3 mixing ratios were elevated by 69 and 79% during the complete and the experimental periods, respectively, compared with the Control treatment, and NOx (NO and NO2) mixing ratios increased by 6.5- and 6.6-fold during the complete and the experimental periods, respectively, compared with the Control treatment. Moreover, O3 mixing ratios in the D + O3 treatment were similar to those in the Control treatment during both time periods (Supplementary Figures 1C,D) and mixing ratios of NOx and the ratio of NO/NO2 significantly decreased under D + O3, compared with the D treatment, during the complete and the experimental periods (Supplementary Figures 1A,B, respectively).
A total of 27,071 specimens within 125 taxonomic groups were collected from the pitfall traps. Pollution treatment was a good predictor of all three resolutions according to pRDAs (Figure 1). In general, herbivores and pollinators responded negatively to diesel-polluted (D and D + O3) treatments (Figure 1C), but in contrast, predators, parasitoids, detritivores and fungivores responded positively to diesel-polluted treatments. Taxa and Class/Order-level resolutions (Figures 1A,B, respectively) highlighted some intra-functional group differences and were better predictors of pollution treatment effects than feeding guild. For example, Coleoptera (beetles) and Diplopoda (millipedes) tended to increase in response to D, whereas Hemiptera (true bugs), Lepidoptera (butterflies and moths), and Hymenoptera (bees, wasps and ants) tended to increase under the Control treatment.
Figure 1. Effects of exposure to air pollution (diesel exhaust and ozone, individually and in combination) on invertebrate abundance at three resolutions: (A) taxa (the most precise taxonomic resolution identified), (B) Order or Class and (C) feeding guild. Pollution treatments are displayed as centroids (blue). Adjusted R2 values represent the explanatory power of the three RDA models. F- and P-values represent model significance based on 999 permutations.
Pollution treatment had a significant effect on taxonomic richness (χ23,7 = 14.76, P = 0.002), which decreased in response to diesel pollution (D and D + O3) compared with the Control treatment. However, taxonomic richness did not vary significantly between O3 and Control treatments (Figure 2A). Pollution treatment had no significant effect on the Shannon diversity index (χ23,7 = 5.34, P = 0.149; Figure 2B). Total invertebrate abundance was significantly affected by air pollution (χ23,7 = 24.09, P < 0.001), whereby both D and D + O3 significantly decreased invertebrate abundance (Figure 3A). Diet in interaction with pollution treatment significantly impacted invertebrate abundance (χ23,11 = 85.44, P < 0.001), whereby those invertebrates with a specialist diet significantly decreased under all elevated pollution treatments, whereas generalist feeders only decreased significantly under D alone (Figure 3B). Moreover, a two-way interaction between mobility and pollution treatment (χ23,11 = 8.691, P = 0.034) showed that winged individuals responded more negatively to elevated air pollution treatments than those that were not winged (Figure 3C). There was no three-way interactive effect between pollution treatment, diet and mobility (χ23,19 = 6.177, P = 0.103). When accounting for differences in abundance between wingless, part-winged and full-winged taxa (i.e., to determine whether air pollution-mediated changes in abundance were driven by individuals that were capable of active flight), both part-winged and full-winged taxa showed similar responses to air pollution (treatment × wing presence χ23,11 = 36.82, P < 0.001; Supplementary Figure 2) and when full-winged taxa were excluded from analyses, air pollution had similar effects on total invertebrate abundance (χ23,7 = 52.20, P < 0.001; Supplementary Figure 3).
Figure 2. Effects of exposure to air pollution (diesel exhaust and ozone, individually and in combination) on (A) taxonomic richness and (B) Shannon diversity index. Crossbar values represent means ± SE, with dot plots representing the distribution of data points around the means. Values with the same letters were not significantly different (P < 0.05).
Figure 3. Effects of exposure to air pollution (diesel exhaust and ozone, individually and in combination) on (A) total invertebrate abundance (i.e., the total number of individual invertebrates collected in each pitfall trap), (B) the abundance of those identified as having a generalist and specialist diet and (C) the abundance of those identified as winged and not winged. Values are means ± SE. Total invertebrate abundance (A) includes taxa that were not characterised by diet and/or the presence of wings. Values with the same letters were not significantly different (P < 0.05).
This study took a novel field-based approach to investigate ecological effects of two of the most common interacting air pollutants (NOx, emitted from diesel exhaust, and O3) on invertebrate community composition. The mixing ratios of NOx and O3 recorded were well below the current United States Environmental Protection Agency’s National Ambient Air Quality Standards (O3 = 70 ppb averaged over 8 h, NO2 = 53 ppb averaged annually, values for NO are not stipulated), which specify the maximum outdoor pollutant levels for public health and environmental safety (EPA, 2021) and mixing ratios were lower still under the combined (D + O3) treatment. Both NOx and O3 are highly reactive gases associated with adverse short- and long-term human health outcomes (Rao et al., 2017; Nuvolone et al., 2018) and the ratio of these pollutants in an environment depends on whether a region or area is NOx- or VOC-limited at a particular time (Wang et al., 2019; Bae et al., 2020). NOx and O3 react with and often counteract each other in the atmosphere, whereby O3 can be consumed by reacting with NO to produce NO2 and oxygen. This was apparent from the decrease in NO:NO2 and lowered O3 concentrations in the combined treatment. Despite being exposed to only low to moderate levels of NOx and O3 (Connolly et al., 2013), invertebrate community composition (i.e., abundance and species richness) was deleteriously impacted by air pollution. In 2010, a meta-analysis on the effects of various point polluters (e.g., iron and steel factories, non-ferrous industries and power plants) on terrestrial arthropods (Zvereva and Kozlov, 2010) revealed no clear effects of air pollution on arthropod diversity but adverse effects on arthropod abundance. They also found that species richness of soil arthropods (i.e., those living in or on the soil surface) decreased, whereas herbivore species richness tended to increase under air pollution. In the current study, negative effects of air pollution on invertebrate abundance and taxonomic richness were mostly driven by exposure to diesel exhaust, with O3 exposure having a lesser negative effect on community composition.
Ecosystem stability (i.e., the ability of an ecosystem to resist and/or recover from environmental changes) tends to increase with higher invertebrate abundance and species richness (Peterson et al., 1998; Cleland, 2011). As such, ecosystems are likely to be less stable in polluted environments, especially in diesel-polluted areas close to roadways and point polluters. Moderate O3 concentrations at exposure levels similar to our study have previously demonstrated deleterious effects on invertebrate foraging (e.g., Khaling et al., 2020) but high levels of O3 (e.g., concentrations higher than 80 ppb) are likely to have a much greater impact on invertebrate communities (Fuentes et al., 2013; Farré-Armengol et al., 2016; Lemic et al., 2019; Vanderplanck et al., 2021). In the current study, O3 exposure reduced the negative effects of diesel exhaust on taxonomic richness and invertebrate abundance, even with the relatively low elevated levels of O3 concentrations emitted. This demonstrates the importance of incorporating multiple pollutants, as opposed to the exposure of individual pollutants, in studies into the ecological impacts of air pollution on biotic communities (Li et al., 2016; Papazian and Blande, 2020).
Exposure to both diesel exhaust and O3 pollution, individually and in combination, reduced the abundance of specialist-feeding and winged invertebrates by 29–34% and 18–35%, respectively. These negative responses to air pollution were not driven by species that are less likely to be caught in pitfall traps (i.e., species that undertake active flight) because part-winged and full-winged invertebrate taxa showed comparable responses to air pollution treatments. Furthermore, excluding full-winged (active-flight) taxa from the analysis of the effects of air pollution on total invertebrate abundance did not alter the experimental findings. There are several possible mechanisms that could contribute to the changes in invertebrate community composition we observed in this study. For example, these responses may be associated with qualitative or quantitative changes in the relative composition of volatile odour cues, as a result of their exposure to diesel exhaust and O3, which is known to adversely impact the learning and recognition of olfactory signals by pollinators (Girling et al., 2013; Fuentes et al., 2016) and other invertebrates (Fuentes et al., 2013; Khaling et al., 2016; Li et al., 2016). Many invertebrates, especially those that fly, depend on volatile chemical odour communication for critical interactions in their life histories, such as locating plants or insect hosts. Indeed, the relatively high abundance of winged invertebrates captured in the pitfall traps suggests that odour-mediated changes may have driven the observed differences in total invertebrate abundance between treatments. The use of other trapping methods, such as sticky traps and pan traps, including and excluding the presence of odour cues within the FADOE rings, would allow us to mechanistically quantify these changes while providing a more reliable estimate of active-flight invertebrates within and between pollution treatments. If the observed changes in invertebrate activity were odour-mediated, specialist invertebrates, which often show innate preferences toward specific volatile blends, as opposed to the learned responses of generalists, may be less adaptable to changes in the olfactory environment (Gols et al., 2012; Riffell et al., 2013; Farré-Armengol et al., 2016). This makes them more likely to be affected by air pollution-mediated signal degradation, thus reducing their foraging success in polluted environments (McFrederick et al., 2009; Gols et al., 2012). However, these differences could be associated with a combination of mechanisms, including pollution-mediated changes in plant nutritional quality or increases in plant defensive compounds, which specialists are less able to adapt to. It is also possible that diesel exhaust and O3 may have a greater negative impact on the physiology of specialist than of generalist invertebrates, because the latter are more commonly adaptable to a wider range of environmental conditions (Agathokleous et al., 2020).
Pitfall trapping is a measure of activity density because the numbers of individuals trapped depend on the population density of species as well as other factors such as locomotory activity (Thiele, 2012). If standardised procedures are used, numbers can be compared between species or taxa (relative differences), which is sufficient for most studies to estimate population size or community structure in time or space (Duelli et al., 1999; Perner and Schueler, 2004). In the current study, we cannot rule out the possibility that pollution-mediated decreases in abundance were associated with a decrease in locomotory activity within the rings, which is most likely to be the case for flying invertebrates (Vanderplanck et al., 2021). However, we previously demonstrated in a control study within the FADOE rings (Ryalls et al., 2022) that flying invertebrate pollinators were not inhibited from entering the pollution rings, whereby there were no significant differences in the abundance of flying invertebrate taxa between rings when olfactory cues were absent. Moreover, Vanderplanck et al. (2021) observed a decrease in motility of fig wasps under 80 ppb O3 but found no changes in motility under O3 levels equivalent to those we recorded in the O3-polluted rings (40 ppb), so direct changes in activity levels are unlikely to be driving the observed differences in flying invertebrate abundance between treatments. The effects of diesel exhaust and O3 pollution on some feeding guilds, such as herbivores, may depend more on changes in plant nutritional value and/or how their natural enemies respond to these pollutants (Fuentes et al., 2013; Campbell and Vallano, 2018; Khaling et al., 2020; Papazian and Blande, 2020). In this study, while the majority of invertebrates responded negatively to air pollution in general, the abundance of some groups (e.g., fungivores) and orders (e.g., Coleoptera) increased under diesel pollution, suggesting that these invertebrates are more resilient to air pollution or its wider effects. Alternatively, their responses to air pollution could be associated with wheat stress-related increases in secondary metabolites or a release from competitive constraints. For example, coleopteran and hemipteran herbivore populations, and their associated damage to plants, have been shown to increase in NO2- and O3-polluted atmospheres, often associated with stress-related increases in plant metabolites, and these pollution-mediated population increases may be exacerbated by concurrent decreases in populations of natural enemies or other herbivores that compete for the same plant (Whittaker, 2001; NECR199, 2016; Masui et al., 2021). While it can be challenging to classify invertebrate taxa into individual categories, a more detailed trait-based approach, incorporating foraging data on individual species, such as their dispersal ability, hunting strategy (including their ability to scavenge, which is often overlooked in entomological studies) and domain (e.g., ground-dwelling, foliar, aerial or within-plant) would help to identify functional changes in terrestrial invertebrate communities (Staton et al., 2021). Moreover, incorporating trophic complexity into field-based studies, at spatial and temporal scales that are relevant to the invertebrates, is key to understanding community-level responses to air pollution. This information can then feed into behavioural studies to evaluate the mechanisms underpinning such responses.
In general, our results demonstrated that both diesel exhaust and O3 pollution had adverse impacts on invertebrate community composition, although in the combined pollution treatment O3 exposure mitigated the negative effects of diesel exhaust emission on invertebrate abundance and taxonomic richness. Air pollution had a lesser negative effect on generalists compared with specialist feeders, suggesting that specialists are more at risk of air pollution-mediated declines. Holistic, field-based approaches such as this are key to understanding the broader ecological impacts of air pollution on insect communities (and the ecosystem services that they provide) before examining mechanisms and behavioural changes in bioassays, which may result in little or no ecological impacts in the field. As such, identifying these ecological impacts under real-world pollution scenarios could facilitate policy changes to mitigate deleterious impacts on invertebrate-provided ecosystem services.
Data for nitrogen oxide and ozone concentrations are available at the EIDC repository doi: 10.5285/d2e0cf65-010c-4206-8302-195449d0acba. Data for taxonomic composition and abundance of invertebrates are available at the EIDC repository doi: 10.5285/7262ce48-0cc3-4998-89ee-36d676bd057d. Traits for each taxon are detailed in Supplementary Table 1.
JR, NM, BL, CP, EN, JB, and RG contributed to conception and design of the study. NM and JR contributed to the maintenance of the FADOE facility. JR, TS, and LB collected and processed the data. JR and TS analysed the data. JR, TS, and RG wrote the first draft of the manuscript. All authors contributed to manuscript revision, read and approved the submitted version.
This work was supported by a British Ecological Society Large Research grant (LRB18/1009) awarded to JR and a Natural Environment Research Council grant (NE/P002404/1 and NE/P001971/2).
The authors declare that the research was conducted in the absence of any commercial or financial relationships that could be construed as a potential conflict of interest.
All claims expressed in this article are solely those of the authors and do not necessarily represent those of their affiliated organizations, or those of the publisher, the editors and the reviewers. Any product that may be evaluated in this article, or claim that may be made by its manufacturer, is not guaranteed or endorsed by the publisher.
We thank Richard Casebow, Caroline Hadley, David Casebow, Kaan Alkan, and Fiona Gierer for help with FADOE construction and field site preparation, and AMOF for the loan of the O3 analyser.
The Supplementary Material for this article can be found online at: https://www.frontiersin.org/articles/10.3389/fevo.2022.833088/full#supplementary-material
ACEA (2019). European Automobile Manufacurers Association Access to Euro 6 RDE Data. Available Online at: https://www.acea.be/publications/article/access-to-euro-6-rde-monitoring-data [accessed July 1, 2020].
Agathokleous, E., Feng, Z., Oksanen, E., Sicard, P., Wang, Q., Saitanis, C. J., et al. (2020). Ozone affects plant, insect, and soil microbial communities: a threat to terrestrial ecosystems and biodiversity. Sci. Adv. 6:eabc1176. doi: 10.1126/sciadv.abc1176
Ares, E., and Smith, L. (2017). Air Pollution: Meeting Nitrogen Dioxide Targets (Commons Library Briefing). London: House of Commons Library.
Bae, C., Kim, H. C., Kim, B.-U., and Kim, S. (2020). Surface ozone response to satellite-constrained NOx emission adjustments and its implications. Environ. Pollut. 258:113469. doi: 10.1016/j.envpol.2019.113469
Bates, D., Mächler, M., Bolker, B., and Walker, S. (2014). Fitting linear mixed-effects models using lme4. J. Stat. Softw. 67, 1–48. doi: 10.18637/jss.v067.i01
Brand, C. (2016). Beyond ‘Dieselgate’: implications of unaccounted and future air pollutant emissions and energy use for cars in the United Kingdom. Energy Policy 97, 1–12. doi: 10.1016/j.enpol.2016.06.036
Campbell, S. A., and Vallano, D. M. (2018). Plant defences mediate interactions between herbivory and the direct foliar uptake of atmospheric reactive nitrogen. Nat. Commun. 9:4743. doi: 10.1038/s41467-018-07134-9
Colette, A., Aas, W., Banin, L., Braban, C. F., Ferm, M., Gonzalez Ortiz, A., et al. (2016). Air Pollution Trends in the EMEP Region Between 1990 and 2012. Joint Report of the EMEP Task Force on Measurements and Modelling (TFMM), Chemical Co-Ordinating Centre (CCC), Meteorological Synthesizing Centre-East (MSC-E), Meteorological Synthesizing Centre-West (MSC-W). Kjeller: Norwegian Institute for Air Research.
Connolly, E., Fuller, G., Baker, T., and Willis, P. (2013). Update on Implementation of the Daily Air Quality Index. Available Online at: http://uk-air.defra.gov.uk/library/reports (accessed February 23, 2018).
Couture, J. J., and Lindroth, R. L. (2013). “Chapter 11 - impacts of atmospheric change on tree–arthropod interactions,” in Climate Change, Air Pollution and Global Challenges: Understanding and Perspectives from Forest Research, eds R. Matyssek, N. Clarke, P. Cudlin, T. N. Mikkelsen, J. P. Tuovinen, G. Wieser, et al. (Amsterdam: Elsevier), 227–248. doi: 10.1002/etc.3484
Didham, R. K., Basset, Y., Collins, C. M., Leather, S. R., Littlewood, N. A., Menz, M. H. M., et al. (2020). Interpreting insect declines: seven challenges and a way forward. Insect Conserv. Divers. 13, 103–114. doi: 10.1111/icad.12408
Duelli, P., Obrist, M. K., and Schmatz, D. R. (1999). “Biodiversity evaluation in agricultural landscapes: above-ground insects,” in Invertebrate Biodiversity as Bioindicators of Sustainable Landscapes, ed. M. G. Paoletti (Amsterdam: Elsevier), 33–64. doi: 10.1371/journal.pone.0091575
EPA (2021). United States Environmental Protection Agency: Air Topics. Available Online at: http://www.epa.gov/air/criteria.html [accessed March 31, 2021].
Farré-Armengol, G., Peñuelas, J., Li, T., Yli-Pirilä, P., Filella, I., Llusia, J., et al. (2016). Ozone degrades floral scent and reduces pollinator attraction to flowers. New Phytol. 209, 152–160. doi: 10.1111/nph.13620
Fuentes, J. D., Chamecki, M., Roulston, T., Chen, B., and Pratt, K. R. (2016). Air pollutants degrade floral scents and increase insect foraging times. Atmos. Environ. 141, 361–374. doi: 10.1016/j.atmosenv.2016.07.002
Fuentes, J. D., Roulston, T. H., and Zenker, J. (2013). Ozone impedes the ability of a herbivore to find its host. Environ. Res. Lett. 8:014048. doi: 10.1088/1748-9326/8/1/014048
Girling, R. D., Lusebrink, I., Farthing, E., Newman, T. A., and Poppy, G. M. (2013). Diesel exhaust rapidly degrades floral odours used by honeybees. Sci. Rep. 3:2779. doi: 10.1038/srep02779
Gols, R., Veenemans, C., Potting, R. P. J., Smid, H. M., Dicke, M., Harvey, J. A., et al. (2012). Variation in the specificity of plant volatiles and their use by a specialist and a generalist parasitoid. Anim. Behav. 83, 1231–1242. doi: 10.1016/j.anbehav.2012.02.015
Hansen, E. M. Ø., Hauggaard-Nielsen, H., Launay, M., Rose, P., and Mikkelsen, T. N. (2019). The impact of ozone exposure, temperature and CO2 on the growth and yield of three spring wheat varieties. Environ. Exp. Bot. 168:103868. doi: 10.1016/j.envexpbot.2019.103868
Holopainen, J. K. (2009). “Plant-insect interactions and pollution,” in Physiology and Maintenance Volume V - Neurophysiology, Plant Physiology and Environment, eds O. O. P. Hanninen and M. Atalay (Oxford: Encyclopedia of Life Support Systems), 358–374.
IPBES (2019). Summary for Policymakers of the Global Assessment Report on Biodiversity and Ecosystem Services of the Intergovernmental Science-Policy Platform on Biodiversity and Ecosystem Services, eds J. S. S. Díaz, E. S. Brondízio, H. T. Ngo, M. Guèze, J. Agard, A. Arneth, et al. (Bonn: IPBES).
Jamieson, M. A., Burkle, L. A., Manson, J. S., Runyon, J. B., Trowbridge, A. M., and Zientek, J. (2017). Global change effects on plant–insect interactions: the role of phytochemistry. Curr. Opin. Insect Sci. 23, 70–80. doi: 10.1016/j.cois.2017.07.009
Jürgens, A., and Bischoff, M. (2017). Changing odour landscapes: the effect of anthropogenic volatile pollutants on plant–pollinator olfactory communication. Funct. Ecol. 31, 56–64. doi: 10.1111/1365-2435.12774
Khaling, E., Agyei, T., Jokinen, S., Holopainen, J. K., and Blande, J. D. (2020). The phytotoxic air-pollutant O3 enhances the emission of herbivore-induced volatile organic compounds (VOCs) and affects the susceptibility of black mustard plants to pest attack. Environ. Pollut. 265:115030. doi: 10.1016/j.envpol.2020.115030
Khaling, E., Li, T., Holopainen, J. K., and Blande, J. D. (2016). Elevated ozone modulates herbivore-induced volatile emissions of Brassica nigra and alters a tritrophic interaction. J. Chem. Ecol. 42, 368–381. doi: 10.1007/s10886-016-0697-8
Lemic, D., Jembrek, D., Bažok, R., and Pajač Živković, I. (2019). Ozone effectiveness on wheat weevil suppression: preliminary research. Insects 10:357. doi: 10.3390/insects10100357
Lenth, R. V. (2021). emmeans: Estimated Marinal Means, aka Least-Squares Means. R Package Version 1.7.0. Available Online at: https://CRAN.R-project.org/package=emmeans (accessed November 4, 2021).
Leonard, R. J., Pettit, T. J., Irga, P., McArthur, C., and Hochuli, D. F. (2019). Acute exposure to urban air pollution impairs olfactory learning and memory in honeybees. Ecotoxicology 28, 1056–1062. doi: 10.1007/s10646-019-02081-7
Li, T., Blande, J. D., and Holopainen, J. K. (2016). Atmospheric transformation of plant volatiles disrupts host plant finding. Sci. Rep. 6:33851. doi: 10.1038/srep33851
Lusebrink, I., Girling, R. D., Farthing, E., Newman, T. A., Jackson, C. W., and Poppy, G. M. (2015). The effects of diesel exhaust pollution on floral volatiles and the consequences for honey bee olfaction. J. Chem. Ecol. 41, 904–912. doi: 10.1007/s10886-015-0624-4
Masui, N., Agathokleous, E., Mochizuki, T., Tani, A., Matsuura, H., and Koike, T. (2021). Ozone disrupts the communication between plants and insects in urban and suburban areas: an updated insight on plant volatiles. J. For. Res. 32, 1337–1349. doi: 10.1007/s11676-020-01287-4
McFrederick, Q. S., Fuentes, J. D., Roulston, T., Kathilankal, J. C., and Lerdau, M. (2009). Effects of air pollution on biogenic volatiles and ecological interactions. Oecologia 160, 411–420. doi: 10.1007/s00442-009-1318-9
NECR199 (2016). The Ecological Effects of Air Pollution from Road Transport: an Updated Review (NECR199). York: Natural England.
Nuvolone, D., Petri, D., and Voller, F. (2018). The effects of ozone on human health. Environ. Sci. Pollut. Res. 25, 8074–8088. doi: 10.1007/s11356-017-9239-3
Oksanen, J., Blanchet, F. G., Friendly, M., Kindt, R., Legendre, P., McGlinn, D., et al. (2017). vegan: Community Ecology Package. R package version 2.4-2.
Papazian, S., and Blande, J. D. (2020). Dynamics of plant responses to combinations of air pollutants. Plant Biol. 22, 68–83. doi: 10.1111/plb.12953
Perner, J., and Schueler, S. (2004). Estimating the density of ground-dwelling arthropods with pitfall traps using a nested-cross array. J. Anim. Ecol. 73, 469–477.
Peterson, G., Allen, C. R., and Holling, C. S. (1998). Ecological resilience, biodiversity, and scale. Ecosystems 1, 6–18. doi: 10.1007/s100219900002
Rao, S., Klimont, Z., Smith, S. J., Van Dingenen, R., Dentener, F., Bouwman, L., et al. (2017). Future air pollution in the shared socio-economic pathways. Glob. Environ. Change 42, 346–358. doi: 10.1016/j.gloenvcha.2016.05.012
Reitmayer, C. M., Ryalls, J. M. W., Farthing, E., Jackson, C. W., Girling, R. D., and Newman, T. A. (2019). Acute exposure to diesel exhaust induces central nervous system stress and altered learning and memory in honey bees. Sci. Rep. 9:5793. doi: 10.1038/s41598-019-41876-w
Riffell, J. A., Lei, H., Abrell, L., and Hildebrand, J. G. (2013). Neural basis of a pollinator’s buffet: olfactory specialization and learning in Manduca sexta. Science 339, 200–204. doi: 10.1126/science.1225483
Ryalls, J. M. W., Langford, B., Mullinger, N. J., Bromfield, L. M., Nemitz, E., Pfrang, C., et al. (2022). Anthropogenic air pollutants reduce insect-mediated pollination services. Environ. Pollut. 297:118847. doi: 10.1016/j.envpol.2022.118847
Scudder, G. G. E. (2017). “The importance of insects,” in Insect Biodiversity, eds R. G. Foottit and P. H. Adler (Hoboken, NJ: Wiley), 9–43. doi: 10.1002/9781118945568.ch2
Snyder, W. E., and Ives, A. R. (2003). Interactions between specialist and generalist natural enemies: parasitoids, predators, and pea aphid biocontrol. Ecology 84, 91–107. doi: 10.1890/0012-9658(2003)084[0091:IBSAGN]2.0.CO;2
Staton, T., Walters, R. J., Smith, J., Breeze, T. D., and Girling, R. D. (2021). Evaluating a trait-based approach to compare natural enemy and pest communities in agroforestry vs. arable systems. Ecol. Appl. 31:e02294. doi: 10.1002/eap.2294
Thiele, H.-U. (2012). Carabid Beetles in their Environments: a Study on Habitat Selection by Adaptations in Physiology and Behaviour. Berlin: Springer Science & Business Media.
Thimmegowda, G. G., Mullen, S., Sottilare, K., Sharma, A., Mohanta, S. S., Brockmann, A., et al. (2020). A field-based quantitative analysis of sublethal effects of air pollution on pollinators. Proc. Natl. Acad. Sci. U.S.A. 117, 20653–20661. doi: 10.1073/pnas.2009074117
Turnock, S. T., Wild, O., Sellar, A., and O’Connor, F. M. (2019). 300 years of tropospheric ozone changes using CMIP6 scenarios with a parameterised approach. Atmos. Environ. 213, 686–698. doi: 10.1016/j.atmosenv.2019.07.001
van Klink, R., Bowler, D. E., Gongalsky, K. B., Swengel, A. B., Gentile, A., and Chase, J. M. (2020). Meta-analysis reveals declines in terrestrial but increases in freshwater insect abundances. Science 368, 417–420. doi: 10.1126/science.aax9931
Vanderplanck, M., Lapeyre, B., Lucas, S., and Proffit, M. (2021). Ozone induces distress behaviors in fig wasps with a reduced chance of recovery. Insects 12:995. doi: 10.3390/insects12110995
Wagner, D. L. (2020). Insect declines in the Anthropocene. Annu. Rev. Entomol. 65, 457–480. doi: 10.1146/annurev-ento-011019-025151
Wang, N., Lyu, X., Deng, X., Huang, X., Jiang, F., and Ding, A. (2019). Aggravating O3 pollution due to NOx emission control in eastern China. Sci. Total Environ. 677, 732–744. doi: 10.1016/j.scitotenv.2019.04.388
Whittaker, J. B. (2001). Insects and plants in a changing atmosphere. J. Ecol. 89, 507–518. doi: 10.1046/j.0022-0477.2001.00582.x
Keywords: air pollution, community structure, diesel exhaust, functional traits, insects, invertebrates, terrestrial ecosystem, tropospheric ozone
Citation: Ryalls JMW, Staton T, Mullinger NJ, Bromfield LM, Langford B, Pfrang C, Nemitz E, Blande JD and Girling RD (2022) Ozone Mitigates the Adverse Effects of Diesel Exhaust Pollutants on Ground-Active Invertebrates in Wheat. Front. Ecol. Evol. 10:833088. doi: 10.3389/fevo.2022.833088
Received: 10 December 2021; Accepted: 28 January 2022;
Published: 11 March 2022.
Edited by:
Juergen Gross, Julius Kühn-Institut, GermanyReviewed by:
Andrey S. Zaitsev, University of Giessen, GermanyCopyright © 2022 Ryalls, Staton, Mullinger, Bromfield, Langford, Pfrang, Nemitz, Blande and Girling. This is an open-access article distributed under the terms of the Creative Commons Attribution License (CC BY). The use, distribution or reproduction in other forums is permitted, provided the original author(s) and the copyright owner(s) are credited and that the original publication in this journal is cited, in accordance with accepted academic practice. No use, distribution or reproduction is permitted which does not comply with these terms.
*Correspondence: James M. W. Ryalls, ai5yeWFsbHNAcmVhZGluZy5hYy51aw==
Disclaimer: All claims expressed in this article are solely those of the authors and do not necessarily represent those of their affiliated organizations, or those of the publisher, the editors and the reviewers. Any product that may be evaluated in this article or claim that may be made by its manufacturer is not guaranteed or endorsed by the publisher.
Research integrity at Frontiers
Learn more about the work of our research integrity team to safeguard the quality of each article we publish.