Corrigendum: Food restriction reveals individual differences in insulin-like growth factor-1 reaction norms
- 1Department of Evolutionary Zoology and Human Biology, University of Debrecen, Debrecen, Hungary
- 2Pál Juhász-Nagy Doctoral School of Biology and Environmental Sciences, University of Debrecen, Debrecen, Hungary
- 3Department of Interdisciplinary Life Sciences, Konrad Lorenz Institute of Ethology, University of Veterinary Medicine, Vienna, Austria
Most organisms have to cope with unpredictable environmental challenges such as fluctuations in nutritional resources. Insulin-like growth factor-1 (IGF-1) is an evolutionarily conserved hormone that is highly sensitive to the individual nutritional status and regulates major life-history traits including lifespan and reproduction across vertebrates. We investigated the role of IGF-1 during periods of food shortages by altering between two feeding regimes (110 and 70% of daily food intake) after a period of ad libitum feeding in captive bearded reedlings (Panurus biarmicus). Each dietary treatment was repeated twice. Birds lost mass under food restriction, but the magnitude of mass change depended on the preceding dietary conditions. Moreover, bearded reedlings showed large, repeatable individual differences in their IGF-1 reaction norms with some individuals increasing IGF-1 levels in response to a restricted diet, whereas others showed no responses or decreased IGF-1 levels. This variation was explained by differences in average body mass: heavier individuals had higher IGF-1 levels during the control treatment and were more likely to decrease IGF-1 levels in response to the dietary restriction than did lighter ones. This result uncovers an individual by environment interaction (I × E) and may have important implications for the evolution of IGF-1 related hormonal phenotypes in this species.
Introduction
Unpredictable fluctuations in the availability of food (e.g., because of droughts, cold winters) are ubiquitous and organisms have developed an array of morphological, physiological and behavioral adaptations to cope with such environmental challenges (Groscolas and Robin, 2001; Harshman and Zera, 2007; Killen et al., 2011). The role of the endocrine system is particularly interesting in this context, because hormones are highly plastic traits that integrate external (environmental) and internal (e.g., nutritional status) information to respond to stochastic environments (Harshman and Zera, 2007; Regan et al., 2020). Across their responsiveness, hormones show a reaction norm (i.e., differential phenotypic expression of a given genotype due to changing environments), which is highly variable within and among individuals (Pigliucci, 2001; Cockrem, 2013). The among-individual variation in reaction norms (individual by environment interaction, I × E) shape phenotypically plastic responses to resource availability in a given environment (Williams, 2008; Baugh et al., 2014; Lendvai et al., 2014; Hau and Goymann, 2015; Madliger and Love, 2016; Vitousek et al., 2018; Houslay et al., 2019). The degree of plasticity influences not only the individual but also the ability of the populations to respond to small immediate changes, such as food shortages or predator attacks, and to long-term effects, such as climate change (Reed et al., 2006; Visser, 2008).
One of the hormonal systems that evolved to respond to the variation in food availability is the insulin/insulin-like signaling pathway (IIS) (Regan et al., 2020). IIS is known to have a prominent role in regulating energy metabolism and is directly integrated with nutrient-sensing cellular mechanisms (Saltiel and Kahn, 2001). In vertebrates, the main ligand of this system is insulin-like growth factor-1 (IGF-1), one of the key factors regulating the organism’s metabolism and development in relation to its nutritional status (Dantzer and Swanson, 2012; Lodjak and Mägi, 2017). These effects might be tightly linked to how IGF-1 determines the transition from the catabolic to the anabolic state. Low nutrient/energy availability is suggested to decrease IGF-1 production and secretion, which leads to increased cell recycling, autophagy, and apoptosis (Bitto et al., 2010; Adler and Bonduriansky, 2014). These processes can have direct and indirect fitness effects by down-regulating reproduction, growth, and the immune response (Wang and Levine, 2010; Gao et al., 2019). On the other hand, up-regulation of IGF-1 can delay muscle atrophy (i.e., an excessive amount of apoptosis of cells) during food restriction and reduce overall weight loss (O’Sullivan et al., 1989; Cleveland et al., 2009; Abe et al., 2019).
Even though fluctuations in food availability frequently occur in nature and IGF-1 might facilitate adaptations toward these conditions (O’Sullivan et al., 1989), our knowledge about the role of IGF-1 in shaping responses to such events in wild animals is limited. The majority of literature presents the findings of medical and agricultural sciences, focusing on humans and laboratory or farmed animals (Robinson et al., 2006; Berryman et al., 2008; Valente et al., 2013; Mauch et al., 2016; Rahmani et al., 2019). There are only a few examples from wild, free-living animals, such as a study in Eastern fence lizard (Sceloporus undulatus) which shows that food-restriction decreased plasma IGF-1 levels (Duncan et al., 2015). Another study on nestlings of a passerine species discusses the possibility that the growth-enhancing effects of IGF-1 during early development might be affected by parental food supply (Lodjak et al., 2014). Hence, to understand the variation in IGF-1 responses to current food availability and its contribution to shaping physiological phenotypes, it is important to aim for experimental studies on wild-type animals originating from natural populations.
To explore the role of the IGF-1 response to variation in food availability in a wild animal, we conducted a food-restriction experiment, using captive adult bearded reedlings (Panurus biarmicus). We repeatedly exposed individuals to changing dietary regimes, by alternating between control (110% of the daily intake) and restricted diet (70% of the daily intake). After each dietary treatment, we measured body mass and circulating IGF-1 levels of the birds. Our experimental design allowed us to disentangle among- and within-individual hormonal variation and to make predictions at both levels. Considering that IGF-1 levels are regarded to reflect the individual nutritional status, we made two predictions. First, we predicted that among individuals, larger (heavier) birds would have higher circulating IGF-1 levels. Second, we predicted that within individuals, circulating IGF-1 levels would decrease in response to the restricted dietary regime and this decrease would be proportional to body mass loss under the restricted diet.
Materials and Methods
General Methods
In July 2017, we caught 24 Bearded reedlings (Panurus biarmicus) at Hortobágy-Halastó, (47°38’13.7 N and 21°04’42.8 E, Hungary) using mist-nets. At the time of capture, all birds were juveniles (yearlings). The age and sex were determined by examining plumage and bill coloration (Svensson, 1992). All birds were ringed with a standard aluminum ring, and we measured tarsus length (to the nearest 0.01 mm) and body mass (to the nearest 0.1 g).
Immediately after the capture and the subsequent measurements, the birds were transferred into an outdoor aviary (3.65 × 3.35 × 2.75 [L × W × H] m) located at the University of Debrecen (47°33’32.9 N and 21°37’14.6 E). The aviary was furnished with reed bundles, branches, and a pool (1 m2 water surface). Food (a mixture of grated apple, carrot, quark, a commercial soft food mixture for insectivorous birds, ground dry cat food as a protein supplement and live mealworms) and water were provided ad libitum. The birds remained for seven months in the aviary before the onset of the experiment (5 February 2018) to habituate to the captive conditions. By the time the experiment started, all individuals had completed their post-juvenile molt and the reproductive period had not yet begun.
We followed all applicable international, national, and institutional guidelines for the use of animals. All procedures performed in studies involving animals were in accordance with the ethical standards of the institution and approved by the Regional government agency (license no HBB/17/00870-3/2015).
Experimental Protocol
The experimental protocol was based on Lendvai et al. (2014), with modifications. Two weeks prior to the experiment, the birds were captured, weighed and transferred into individual cages (measuring 30 × 26 × 32 [L × W × H] cm), located in the outdoor housing facilities.
During the acclimation period, individuals received ad libitum food (as described above) and water. Mealworms were not provided, because live mealworms can leave the feeder, which could have affected the dietary treatments (see below). After 2 weeks of acclimation in the individual cages, we measured the daily food intake (DFI) for each individual for five consecutive days. We fed the birds every morning between 09:00 and 10:00 by filling their feeder with 38 g of food. A 24 h later, we removed the bottom tray and the feeder from each cage and measured the weight of the remaining, and spilled food with a digital scale. The DFI was calculated as the difference between the initial weight in the feeder and the weight of remaining food plus spillage. This procedure was performed twice over 2 weeks, and the average daily food intake (ADFI) was calculated as the average of 10-day DFI values for each individual.
After measuring the food intake, birds were kept under ad libitum food regime for one additional week. After this period, individuals were randomly assigned to one of the treatment groups: food-restricted (70% of their individual ADFI) or control (110% of their individual ADFI). The treatment was based on previous studies showing that a 30% reduction in food is sufficient to trigger changes in hormone levels (e.g., Valle et al., 2015, 2020). The ad libitum diet differs from the control diet in that it is available in large quantities without any restriction throughout the day. In contrast, the control diet is only slightly more than the individuals’ daily food requirement measured under plentiful food conditions (note that food consumption was measured on an ad libitum diet). Therefore, the control diet (i.e., 110% of ADFI) can still be considered as limited dietary regime if an individual’s daily energy requirement is increased (e.g. for strategic fattening or regaining mass; Gosler, 1996). We used a randomized block design during the experiment, with each block containing one food-restricted and one control individual. We tested four blocks (i.e., 8 birds) per day, to minimize the bleeding time. Each treatment lasted for three consecutive days (trial; Figure 1).
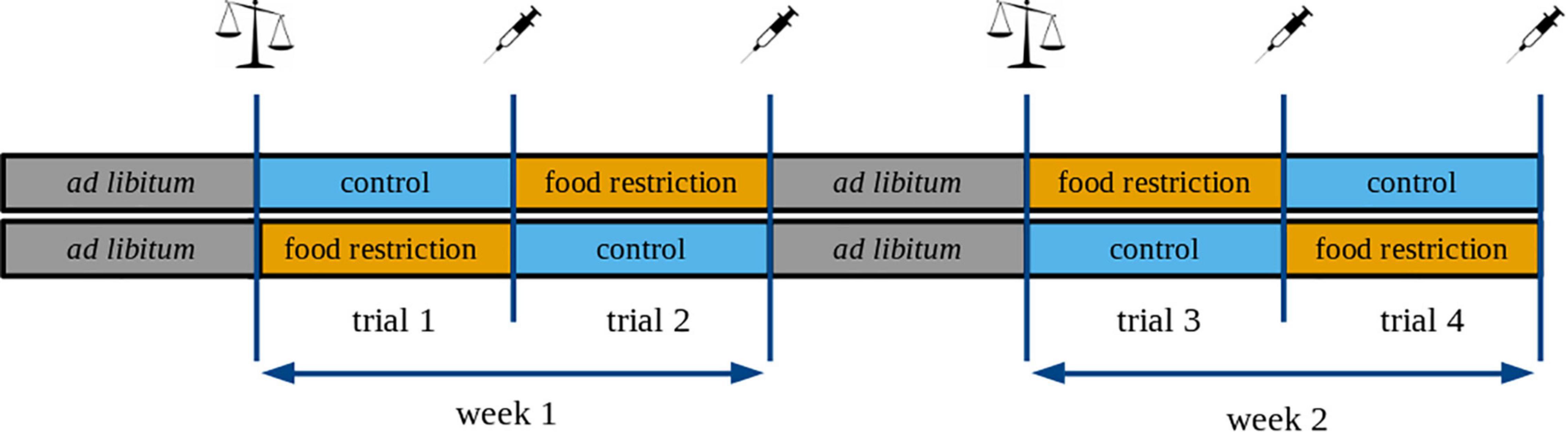
Figure 1. Schematic illustration of the experimental design. Every treatment lasted for three consecutive days, and we called these periods “trials”. Every bird received the control and the food-restricted treatment twice. We refer to the first replicate as “week 1” and to the second replicate as “week 2”. The syringe icon denotes blood sampling and body mass measurements, whereas the balance icon indicates only body mass measurements.
The order of treatments was randomized so that 12 birds received the control and 12 birds the food-restricted treatment during the first trial. After the first trial, each individual received the opposite treatment for three consecutive days (trial 2). After two trials, all birds received the resting diet (ad libitum food enriched with mealworms) for 3 days before trials 3 and 4, for which we reversed the order of the treatments for each individual to separate the effect of the treatment sequence from a group effect. At the end of each trial, we measured the body mass, and took a blood sample (into a heparinized capillary tube) for hormone analyses. We did not sample the birds at the end of the resting period to minimize the number of blood samplings while focusing on the hormonal responses to the restricted vs. the control diet. We refer to the first part of the experiment (trials 1-2) as “week 1” and the second part of the experiment (trials 3-4) as “week 2” (Figure 1).
On each sampling day, we randomized the sampling order for individuals. Blood sampling was always carried out by two experimenters and two assistants. The assistants caught the particular birds following the previously randomized sampling order and gave them to the experimenters, who were blind to the treatment. The assistants also recorded the bleeding time and morphometric variables measured by the experimenters. Bleeding was carried out by puncturing the brachial vein with a 26G sterile needle. We took a maximum of 140 μl blood from each individual, which corresponds with the international ethical standards in blood sampling (Owen, 2010). The bleeding of the first birds always started at 09:00. We recorded when the first person entered the housing facilities and when the blood sample was collected (bleeding time average: 5 min 36 s [min: 1 min 7 s, max: 11 min 48 s]). Preliminary analyses showed that IGF-1 levels were not related to bleeding time, and adding bleeding time never improved the model fit, therefore, this variable was discarded from subsequent analyses. All birds were fed immediately after blood sampling. Blood was kept on ice and centrifuged within one hour to separate plasma from erythrocytes. We stored the samples at −20°C until further processing.
To minimize observer bias, all measurements (during the experiment and hormone assays) were performed blindly for the individuals’ treatment.
IGF-1 Assay
Plasma IGF-1 levels were measured in a competitive ELISA developed in our laboratory at the University of Debrecen, which was validated for Bearded reedlings and described previously in Mahr et al. (2020). Briefly, 96-well microplates were coated overnight at 4°C with 100 μl of an antibody raised against IGF-1 in rabbits. The capture antibody was incubated for 2 h at room temperature (24°C) with 20 μl known concentrations of synthetic chicken IGF-1 in serial dilutions starting at 500 ng/ml or 20 μl of sample and 100 μl biotinylated IGF-1. After incubation, the microplate was washed three times with 250 μl of PBS buffer containing 0.025% Tween 20, and 100 μl of streptavidin-horseradish peroxidase conjugate was added to all wells and incubated at room temperature for 30 min. After washing, 100 μl of tetra-methyl-benzidine was added to the wells and incubated at room temperature for 30 min. The enzymatic reaction was stopped by adding 100 μl of 1 M H2SO4, and optical density (OD) was measured at 450 nm (reference at 620 nm) using a Tecan F50 microplate reader. We used chicken plasma in quadruplicates to determine intra- and inter-assay coefficient of variation (6.8 and 10.88%, respectively). We were not able to calculate the concentration from three samples of IGF-1 because of insufficient plasma volume.
Statistical Analysis
We analyzed our data in a Bayesian framework, using R version 3.6.2 (R core team, 2019) and the package “MCMCglmm” (Hadfield, 2010). First, we analyzed how the treatment affected the body mass of the birds. We fitted a trivariate mixed-effects model, where body mass measured after each treatment period (ad libitum, control, or food-restricted) was used as response variable. The experimental week and the order of treatments (food restriction followed by control “RC” or control followed by food restriction “CR”) and sex were used as fixed effects. Random intercepts were included for individual identity. This trivariate model allowed us to estimate the individual variance in body mass for ad libitum, control, and food-restricted diets separately, and the covariance between these terms. Based on these values, we calculated the among-individual cross-context (cross-treatment) correlations, which indicate intra-individual variation in the response given to the treatment (Dingemanse and Dochtermann, 2013; Houslay and Wilson, 2017). A high cross-context correlation (r close to 1) indicates consistent differences between individuals, e.g., a heavy bird under an ad libitum diet is likely to remain the heaviest (albeit with lower body mass) in the control and food-restricted diet as well. However, a lower r-value indicates that individuals react to different conditions differently (i.e., reaction norms cross over).
Second, we analyzed how the treatment affected IGF-1 concentrations. We used a univariate mixed-effects model with IGF-1 as the response variable, individual identity as the random intercept, and experimental week, treatment order group, treatment, and body mass as fixed effects. However, because body mass varies both among- and within-individuals, in this model, we partitioned the variance explained by body mass into among-individual and within-individual components (van de Pol and Wright, 2009). Among-individual body mass specifies the individual-specific average body mass, which explains variance due to consistent differences among individuals (e.g., a generally heavy vs. a light bird). On the other hand, the within-individual body mass component expresses the changes in body mass due to the specific dietary treatments.
Finally, we analyzed the covariation between IGF-1 and body mass. To do so, we built a multivariate model containing standardized body mass and IGF-1 (i.e., zero-centered and divided by the standard deviation), each included as a separate variable at each treatment level. Because blood samples were only collected after food-restricted and control (but not ad libitum) diets, this model contained four response variables (food-restricted and control treatment levels for both body mass and IGF-1). Individual identity was included as a random intercept. Experimental week, treatment order group, and sex were added as fixed factors. As above, in the trivariate model for body mass, this model also assumed a multivariate normal distribution full variance-covariance matrix that estimated all specific level variances and covariances. We also built alternative models with identical random and fixed structures, where specific parts of the variance-covariance matrix were constrained to zero. The model fit of these alternative models was compared using the Deviance Information Criterion, the Bayesian alternative of the Akaike Information Criterion. In this multivariate model, we also calculated the conditional among-individual variance for treatment-induced IGF-1 levels as variance in IGF-1 levels minus the square of the body mass∼IGF-1 covariance divided by the variance in body mass, for each food treatment level. This value represents the among-individual variance in IGF-1 that is not accounted for by variation in body mass due to the food restriction (Dingemanse and Dochtermann, 2013). When credible intervals of the estimate do not overlap zero, it can be interpreted as a “significant” variation among individuals. To facilitate interpretation, we also provide Bayesian p-values for fixed effects (Hadfield, 2010). As for the body mass model, we also estimated the cross-context correlation for the IGF-1 response, which indicates whether individual reaction norms cross over (if lower than 1).
Results
Body Mass
Males were heavier than females, but sex did not interact with any other variables. The dietary treatment affected body mass in a complex manner, where body mass differed between treatments, the week, the order of treatments and the interaction between order and treatment (Table 1). Experimental food restriction had a strong effect: body mass declined in all individuals (Figure 2 and Supplementary Figure 1). However, this body mass loss during food restriction was stronger when it followed the ad libitum diet than when it followed the control diet. This difference in the severity of body mass loss depending on the food availability in the preceding period was similar in both groups that differed in the order they received the treatments (Figure 2 and Supplementary Figure 1). The dietary conditions that the birds experienced in the preceding period also affected body mass change during the control treatment. Despite having access to 110% of their daily food requirement, birds lost body mass during control treatment if it followed the ad libitum diet. However, if the control treatment followed the restricted (70%) diet, birds regained mass during this period (Supplementary Figure 1). These effects resulted in a pattern that when birds received the control treatment after ad libitum (order “CR”), then body mass after control treatment was mid-way between ad libitum and restricted diet. However, when the control treatment followed the food restriction (order “RC”), the difference between body mass measured after control and restricted diet was more pronounced (Figure 2). Also, during the mid-experiment recovery (ad libitum) phase, birds increased their body mass so much that they became heavier by the beginning of week 2 compared to the beginning of the experiment (week 1). The latter effect was especially pronounced in the group that finished week 1 with the restricted diet (Supplementary Table 1; Figure 2; Supplementary Figure 1). These results were corroborated by univariate Bayesian analyses (Supplementary Table 1).
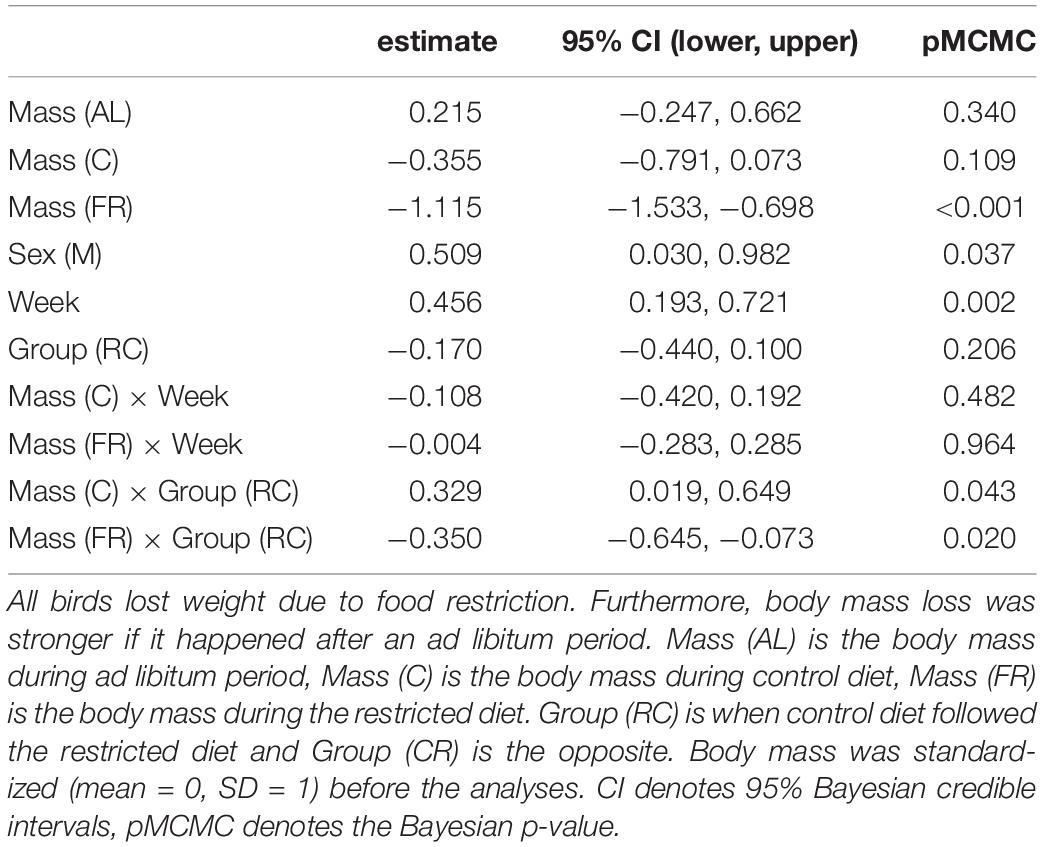
Table 1. The trivariate mixed-effects model showed that the experimental food restriction strongly affected the birds’ body mass.
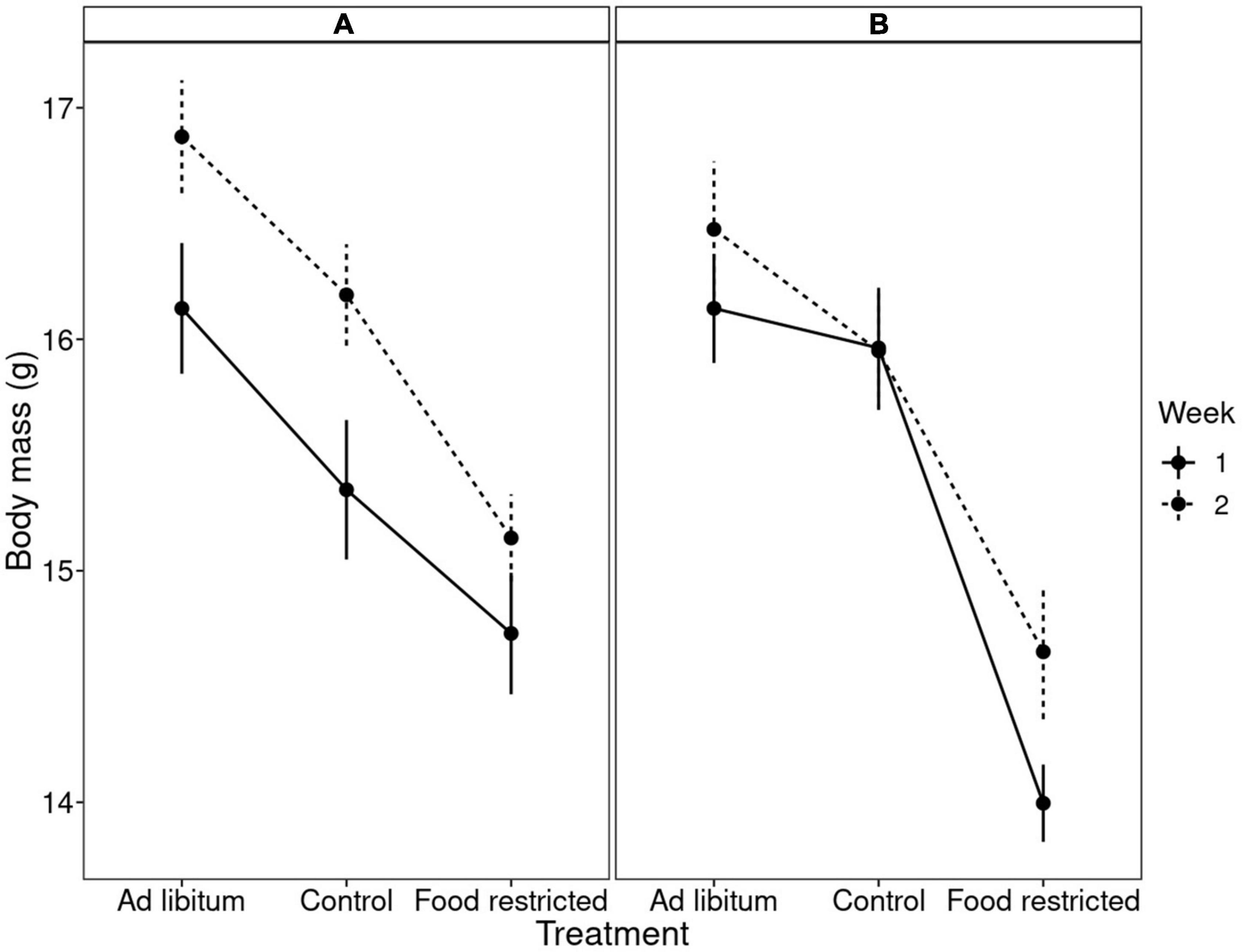
Figure 2. Mean ± SE body mass in bearded reedlings after different dietary treatments. The x-axis shows the type of dietary treatments while the two panels show in which order the birds received these treatments (see also Supplementary Figure 1). (A) Indicates control diet followed by restricted diet, and (B) means restricted diet followed by control diet. Solid and dashed lines indicate week 1 and 2 of the experiment, respectively. Note that body mass variation was affected by treatment, week, the order of treatments and the treatment × order interaction.
While individuals differed in their average body mass, the change in body mass was mostly parallel across feeding regimes among individuals (Supplementary Figure 2). Individual correlation of body mass across treatments was very high (ad libitum – control: 0.95, control - food-restricted: 0.94), indicating that consistent differences in body mass between the individuals remained unchanged despite the experimentally induced changes in body mass (Supplementary Figure 2).
IGF-1
Univariate analyses of IGF-1 showed that IGF-1 levels were higher during the food-restricted diet than during the control (4.40 [0.39; 8.22], p = 0.02) once we controlled for variation in body mass (Figure 3). To investigate this effect further, we divided body mass into two variance components: among-individual body mass (reflecting average mass differences among birds) and within-individual changes in body mass (reflecting experimentally induced loss and regain of body mass). This model showed that while among-individual body mass was positively related to IGF-1 (4.95 [0.75; 9.01], p = 0.02), within-individual changes in body mass tended to be in the opposite direction: i.e., body mass loss was associated with an increase in IGF-1 levels (resulting in a negative slope: −29.85 [−63.21; 2.05], p = 0.06), and this effect was the strongest in birds with low average body mass (1.81 [−0.27; 3.80], p = 0.07, Figure 4). However, the effects of within- and among individual body mass on IGF-1 had wide credible intervals that slightly encompassed 0 and were considered statistically marginally non-significant.
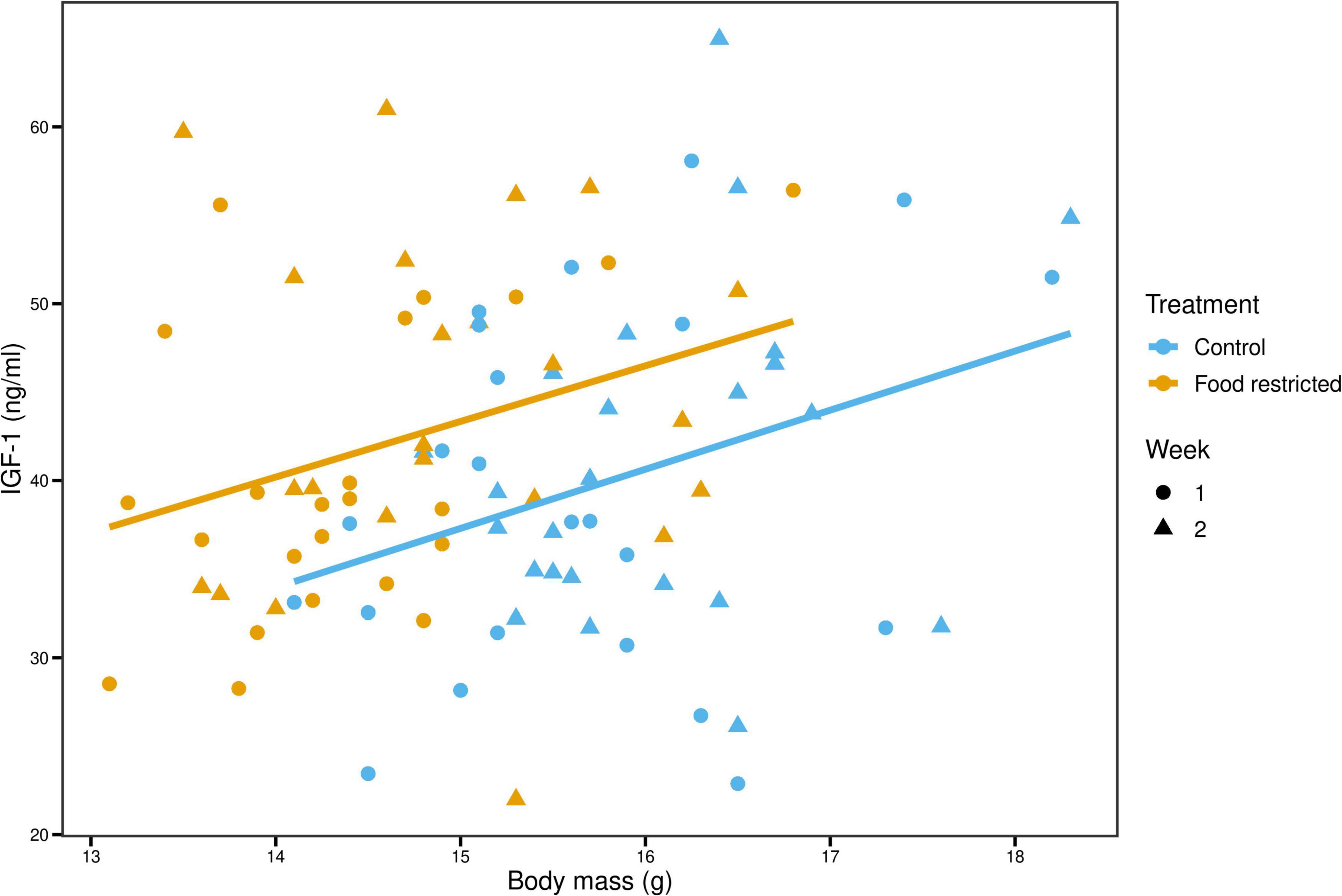
Figure 3. Birds with higher average body mass have higher IGF-1 levels. Blue and orange solid lines show the among-individual relationships for control and food-restricted treatment, respectively.
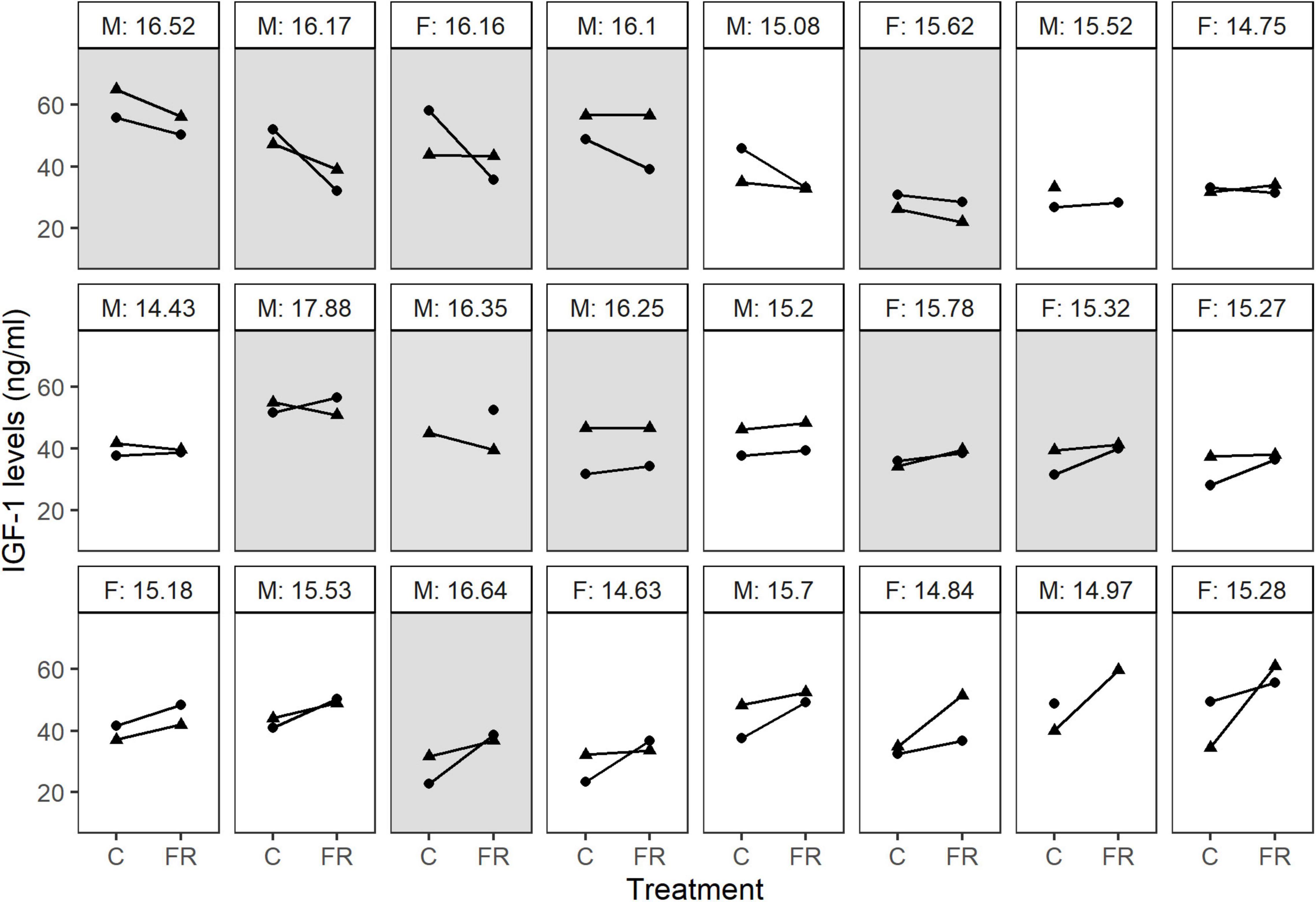
Figure 4. IGF-1 reaction norms in response to food restriction show large among-individual variation. Each panel represents an individual; dots or triangles connected by a line denote IGF-1 levels measured after control (C) or food-restricted (FR) treatment during the first and the second half of the experiment (week 1: dots, week 2: triangles). The text above the panel show the sex (M: males, F: females) and the average body mass (g) for each individual. Light and dark panels indicate individuals lighter and heavier than the median mass of their sex, respectively. For three individuals, one of the samples are missing.
Males tended to have higher IGF-1 levels than females (5.92 [−0.25; 12.15], p = 0.062). Since males were also heavier than females, we repeated the above model by including sex as an additional variable. Controlling for sex did not improve model fit (ΔDIC = −0.2), and sex did not explain significant variation in IGF-1 levels (4.17 [−2.6; 10.57], p = 0.182). At the same time, the other estimates remained similar, indicating that the effects of sex were driven mainly by the higher body mass of males. This was further supported by a model showing that individuals heavier than the median body mass of their sex were more likely to decrease their IGF-1 levels in response to the treatment (−6.87 [−12.12; −1.95], p = 0.009), whereas individuals lighter than the average showed the opposite pattern and were more likely to increase their IGF-1 levels (5.09 [1.66; 8.52], p = 0.003), (Figure 4).
When body mass and IGF-1 were analyzed together in a multivariate model (Table 2), we found significant positive covariance between body mass and IGF-1 under the control diet, corroborating results from the univariate analysis that birds with higher average body mass also had higher IGF-1 levels. The model that estimated covariance between body mass and IGF-1 had better support than the one where body mass was not allowed to covary with IGF-1 levels (ΔDIC = 5.2). IGF-1 levels after food restriction tended to be related to treatment-induced body mass, although this effect remained marginally non-significant (0.19 [−0.06; 0.49]). Furthermore, we found that individuals differed significantly in their IGF-1 levels in response to the food restriction. Still, this difference in individual IGF-1 response was independent of the body mass change caused by food restriction (conditional among-individual variance = 0.36 [0.01; 0.94], repeatability = 0.44). The analysis of change in IGF-1 levels from control to food restriction revealed significant individual variation and crossing reaction norms (Supplementary Figure 3), indicating that some individuals decreased, whereas others increased IGF-1 in response to the treatment (cross-context correlation: r = 0.70, this model is considerably better supported than the model, where the cross-context correlation was fixed to 1: ΔDIC = 19.6).

Table 2. Variance-covariance matrix from the multivariate MCMCglmm model including IGF-1 and body mass under each treatment (control, “C” or food-restricted, “FR”).
Discussion
Our experiment revealed that in response to food restriction, bearded reedlings showed marked individual differences in their IGF-1 reaction norms. While, as predicted, in some individuals, IGF-1 levels decreased in response to a restricted diet, the majority of the birds showed no response or even an increase. We also showed that heavier individuals had higher overall IGF-1 levels, and were more likely to decrease IGF-1 in response to the food-restriction. These results uncover the presence of an individual by environment interaction (I × E) and may have important implications for the evolution of IGF-1-related hormonal phenotypes in this species.
All birds lost weight during the restricted dietary regime, demonstrating that the experimental treatment was sufficient to simulate low food availability. Furthermore, during the mid-experiment ad libitum diet, birds increased their body mass to a higher level than their initial body mass at the beginning of the experiment. Finally, the decrease in body mass was stronger when the restricted diet followed the ad libitum feeding regime than when it followed the control regime (Figure 2). Our findings are in line with the adaptive regulation hypothesis, namely that individuals should maintain their current energetic status during low food availability and regain reserves during periods of high food availability to reduce the future risk of starvation (Witter et al., 1995; Fauchald et al., 2004).
The results on body mass resemble findings on House sparrows by Lendvai et al. (2014), even though the duration of the food-restriction period was shorter (3 days vs. 1 week) and the severity of the food restriction was lower in the current study (30 vs. 40% restriction). Our study design allowed us to separate the effects of the treatment sequence from a group effect because, at the midpoint of the experiment, the order of treatments was reversed in each experimental group (Figure 1). These results emphasize that individual responses to a standardized food restriction depend on previously experienced resource availability (Acquarone et al., 2002; Cucco et al., 2002; Fokidis et al., 2012). During the transition from ad libitum to control diet, birds were presented with less food in their feeder. Although they could still meet their daily energetic requirements (the control diet consisted of 110% of their individual daily food intake, measured under conditions when food was available in plentiful conditions), the lower amount of food left in the feeder at the end of the day may have been a visual cue for anticipating a deterioration of nutritional conditions. On the contrary, the 110% of daily food requirement may be seen as a significant improvement for birds who switched from the restricted treatment. As a consequence, while birds entering the control treatment from ad libitum conditions lost body mass during the control regime, birds previously experiencing food restriction regained body mass during the same treatment (Supplementary Figure 1). These responses indicate that birds may anticipate future resource availability based on previous experiences.
The perception of potential shortages in food resources might also determine individual physiological responses that allow individuals to mitigate the costs of low nutrient availability if they anticipate a decrease in nutritional conditions. While these dynamic changes in body mass were strong and consistent among individuals (Supplementary Figure 2), we found that changes in IGF-1 levels were markedly different among individuals (Figure 4 and Supplementary Figure 3). First, IGF-1 levels were repeatable within individuals and positively related to the average body mass, i.e., heavier birds had higher IGF-1 levels. Males were heavier than females and tended to have higher IGF-1 levels [as shown before in Tóth et al. (2018)], but once we controlled for body mass, the sex difference in hormone levels disappeared. Our findings on the positive relationship between body mass and IGF-1 are consistent with the available (albeit scarce) literature on fish (Cameron et al., 2007), reptiles (Crain et al., 1995; Sparkman et al., 2009), and mammals (Lewin et al., 2016; Tighe et al., 2016), although our result alone does not imply a causal relationship.
Second, individuals differed in their hormonal response to food restriction. While some individuals decreased their IGF-1 levels when food became scarce, others showed little response or even increased their IGF-1 levels (Figure 4). This difference remained after controlling for individual variation in body mass changes. Intriguingly, the variation in reaction norms was associated with the average body mass: relatively lighter birds were more likely to increase IGF-1 levels, while heavier birds were more likely to show a reduction in IGF-1. The restricted dietary regime is expected to decrease IGF-1 expression and secretion across invertebrates and vertebrates (Morishita et al., 1993; Schew et al., 1996). In contrast with our predictions and previous findings, we did not observe a consistent decrease in IGF-1 levels during food restriction, but we found significant among-individual variation (I × E) in the IGF-1 response to food restriction. Therefore, the question arises why food restriction had diverse effects on IGF-1 levels in adult bearded reedlings and whether this physiological response might facilitate survival under conditions of low food availability and constitute a possible coping mechanism to unpredictable environmental cues?
IGF-1 strongly affects energy metabolism including the elevation of glucose uptake without lowering free fatty acid levels (Kastin, 2013; Aguirre et al., 2016). It also promotes the formation of fat reserves via regulation of preadipocyte differentiation and increased lipogenesis (Smith et al., 1988; Scavo et al., 2004), which allows organisms to preserve energy to survive harsh environmental conditions. However, after preadipocytes differentiate, they stop expressing IGF-1 receptors. Therefore, in adipose tissues, only a high concentration of IGF-1 can effectively prevent lipolysis, and stimulate glucose transport (DiGirolamo et al., 1986). Based on our study and the role of IGF-1 in anabolic processes, we suggest that individuals might express different strategies when confronted with reduced food resources based on their initial energy status. Lighter (i.e., lean) individuals might produce more IGF-1 to maintain their energy homeostasis and mitigate the adverse effects of apoptosis and protein degradation, such as muscle atrophy (Musarò et al., 1999; Timmer et al., 2018). Furthermore, increasing IGF-1 during moderate or early stages of fasting might prevent protein degradation and facilitate the maintenance of cell growth and proliferation until energy becomes available (Scavo et al., 2004). On the contrary, heavier (i.e., fat) birds with decreased IGF-1 levels may be able to suppress insulin activity and increase blood glucose levels via gluconeogenesis, which is enough to maintain their normal life processes during harsh conditions and favor survival (Yakar et al., 2004). This hypothesis remains to be tested.
Only a few studies have suggested that food restriction may increase IGF-1. For example, Ayson et al. (2007) observed in rabbitfish that the hepatic IGF-1 mRNA level was higher in the starved (no food for 15 days) group than in the control group, albeit only in the early part of starvation (2nd and 3rd days). During prolonged starvation (15th –18th days), the IGF-1 mRNA level became significantly lower in the starved group compared with the controls. Ayson et al. (2007) suggested that previous studies may have missed the early increase of IGF-1 in response to starvation. However, another study on broiler chicken found that food restriction resulted in higher IGF-1 levels than controls throughout the study (from 15 to 28 weeks of age) (Hocking et al., 2007). We also found that female canaries responded to food restriction by increasing IGF-1 levels during breeding (Hargitai et al., 2022). These studies only analyzed the overall response to food-restriction, but here we show that within a single population, individuals may differ markedly in their physiological response to changes in nutritional conditions.
IGF-1 can also interact with other physiological parameters to modulate phenotypic responses. For example, Lodjak et al. (2016) showed in pied flycatcher nestlings that IGF-1 and glucocorticoid levels are positively related under high food abundance, while this relationship turns negative under low food abundance. They hypothesized the existence of a threshold level in physiological conditions over which the relationship between the two hormones can change. Our finding that heavier birds were more likely to decrease IGF-1 levels under food-restriction, whereas light birds were more likely to increase it may support the existence of such a physiological turning point, which may explain the variance of IGF-1 reaction norm during food-restriction. Accordingly, the relationships between IGF-1 and other physiological traits such as glucose levels, glucocorticoids and a marker of oxidative stress can be reorganized during environmental challenges (Vágási et al., 2020). These results suggest that the relationship between IGF-1 and other physiological factors is context- and condition-dependent and their joint effect on life-history or fitness-related traits is still unexplored in natural populations.
It should also be considered that with few exceptions, most of our knowledge about the effects of food availability on circulating IGF-1 levels comes from experiments conducted on model organisms (e.g., mice) in controlled laboratory settings and/or farm or breeding facilities (Puig and Tjian, 2006; Dantzer and Swanson, 2012). The feeding patterns of these animals (e.g., maintained unlimited access to food in lab condition) may not reflect the natural feeding habits of wild populations. Furthermore, laboratory and farm animals have been artificially selected on specific traits, such as rapid growth and an early onset of the reproduction, and therefore display different life histories and physiological phenotypes (e.g., metabolism) from wild animals (Petersson et al., 1996; Leili et al., 1997; Geiser et al., 2007; Auer et al., 2016; Bolstad et al., 2017). Considering that these traits correlate positively with IGF-1 levels (Frystyk et al., 1999), the measured physiological responses to low food availability may not reflect those of natural populations. Even though we conducted our experiments in captive animals, our study animals came from a natural population, therefore, it can represent the natural variation in IGF-1 response to food restriction.
Here, we uncovered large individual variation in the IGF-1 reaction norms, and the next step is to identify how these phenotypic differences are related to fitness. Dietary restriction is the most robust intervention that extends lifespan and delays ageing in various organisms, and it has been suggested that adaptive plasticity in the insulin/insulin-like signaling pathway underpins the physiological basis of this effect (Regan et al., 2020). Indeed, variation in IGF-1 levels has been connected to various life-history traits in vertebrates (Dantzer and Swanson, 2012). While IGF-1 is most often studied during post-natal growth, there is growing evidence that variation in IGF-1 is connected to fitness-related traits, such as lifespan and fecundity (Lodjak and Mägi, 2017), oxidative stress (Vágási et al., 2020) and ornament expression (Mahr et al., 2020; Lendvai et al., 2021) in adult birds. Given these relationships and that after an initial challenge IGF-1 levels do not return immediately to the baseline level (Gabillard et al., 2006), we expect that the way individuals react to variation in food availability may have longer-term, adaptive consequences. Therefore, the temporary lack of food could have a prolonged effect on reproduction via condition dependent IGF-1 regulation.
In conclusion, our study provides novel information on the existence of repeatable individual variation and multiple reaction norms in IGF-1 levels in response to food restriction. The variability of reaction norms can contribute to maintaining the genetic diversity within populations. This has a major ecological and evolutionary consequence because high genetic diversity reduces bottlenecks caused by environmental challenges and also involves the possibility of fast adaptation to the new circumstances (Fisher, 1930; Bouzat, 2010). IGF-1 showed a highly plastic response to one of the major environmental challenges (food deprivation). Therefore, we propose that IGF-1 might hold a prominent role in shaping adaptive responses to environmental changes, among other physiological variables, which remains to be tested.
Data Availability Statement
Data available on request from the authors.
Ethics Statement
The animal study was reviewed and approved by the Környezetvédelmi és Természetvédelmi Főosztály, Hajdú-Bihar Megyei Kormányhivatal.
Author Contributions
ZT and ÁZL designed the experimental protocol and conducted the statistical analyses. ZT, GÖ, LŐ, and ÁZL performed the experimental procedures with contribution from KM. ZT and ÁZL performed the lab analyses. ZT, KM, and ÁZL wrote the manuscript with contributions from GÖ and LŐ. All authors contributed to the article and approved the submitted version.
Funding
This study was financially supported by the Hungarian Scientific Research Fund (OTKA K113108 and K139021), the National Development, Research and Innovation Fund (2019-2.1.11-TÉT-2019-00043), and the European Union and the European Social Fund (EFOP-3.6.1-16-2016-00022). During manuscript preparation, KM was supported by Schrödinger Fellowship (J4235-B29) by the Austrian Science Fund (FWF) and by the Austrian Agency for International Cooperation in Education and Research (OeAD) WTZ grant (HU 05/2020). The funders had no role in study design, data collection, and analysis, decision to publish, or preparation of the manuscript.
Conflict of Interest
The authors declare that the research was conducted in the absence of any commercial or financial relationships that could be construed as a potential conflict of interest.
Publisher’s Note
All claims expressed in this article are solely those of the authors and do not necessarily represent those of their affiliated organizations, or those of the publisher, the editors and the reviewers. Any product that may be evaluated in this article, or claim that may be made by its manufacturer, is not guaranteed or endorsed by the publisher.
Acknowledgments
We thank Anna Anita Rácz, Dávid Radovics, and Csaba Péter Nagy for their help in the experiment and Jenny Q. Ouyang for discussion. We are grateful to the staff of the Botanical Garden of the University of Debrecen for supporting our work and for providing access to their facilities. We also thank the reviewers for their constructive comments on earlier versions of the manuscript.
Supplementary Material
The Supplementary Material for this article can be found online at: https://www.frontiersin.org/articles/10.3389/fevo.2022.826968/full#supplementary-material
References
Abe, T., Kazama, R., Okauchi, H., and Oishi, K. (2019). Food deprivation during active phase induces skeletal muscle atrophy via IGF-1 reduction in mice. Arch. Biochem. Biophys. 677:108160. doi: 10.1016/j.abb.2019.108160
Acquarone, C., Cucco, M., Cauli, S. L., and Malacarne, G. (2002). Effects of food abundance and predictability on body condition and health parameters: experimental tests with the Hooded Crow. Ibis 144, E155–E163. doi: 10.1046/j.1474-919X.2002.t01-2-00094_1.x
Adler, M. I., and Bonduriansky, R. (2014). Why do the well-fed appear to die young? BioEssays 36, 439–450. doi: 10.1002/bies.201300165
Aguirre, G. A., De Ita, J. R., de la Garza, R. G., and Castilla-Cortazar, I. (2016). Insulin-like growth factor-1 deficiency and metabolic syndrome. J. Transl. Med. 14:3. doi: 10.1186/s12967-015-0762-z
Auer, S. K., Bassar, R. D., Salin, K., and Metcalfe, N. B. (2016). Repeatability of metabolic rate is lower for animals living under field versus laboratory conditions. J. Exp. Biol. 219, 631–634. doi: 10.1242/jeb.133678
Ayson, F. G., de Jesus-Ayson, E. G. T., and Takemura, A. (2007). mRNA expression patterns for GH, PRL, SL, IGF-I and IGF-II during altered feeding status in rabbitfish, Siganus guttatus. Gen. Comp. Endocrinol. 150, 196–204. doi: 10.1016/j.ygcen.2006.08.001
Baugh, A. T., van Oers, K., Dingemanse, N. J., and Hau, M. (2014). Baseline and stress-induced glucocorticoid concentrations are not repeatable but covary within individual great tits (Parus major). Gen. Comp. End. 208, 154–163. doi: 10.1016/j.ygcen.2014.08.014
Berryman, D. E., Christiansen, J. S., Johannsson, G., Thorner, M. O., and Kopchick, J. J. (2008). Role of the GH/IGF-1 axis in lifespan and healthspan: lessons from animal models. Growth Horm. IGF Res. 18, 455–471. doi: 10.1016/j.ghir.2008.05.005
Bitto, A., Lerner, C., Torres, C., Roell, M., Malaguti, M., Perez, V., et al. (2010). Long-term IGF-I exposure decreases autophagy and cell viability. PLoS One 5:e12592. doi: 10.1371/journal.pone.0012592
Bolstad, G., Hindar, K., Robertsen, G., Jonsson, B., Sægrov, H., Diserud, O. H., et al. (2017). Gene flow from domesticated escapes alters the life history of wild Atlantic salmon. Nat. Ecol. Evol. 1:0124. doi: 10.1038/s41559-017-0124
Bouzat, J. L. (2010). Conservation genetics of population bottlenecks: the role of chance, selection, and history. Conserv. Genet. 11, 463–478. doi: 10.1007/s10592-010-0049-0
Cameron, C., Moccia, R., Azevedo, P. A., and Leatherland, J. F. (2007). Effect of diet and ration on the relationship between plasma GH and IGF-1 concentrations in Arctic charr, Salvelinus alpinus (L.). Aquac. Res. 38, 877–886. doi: 10.1111/j.1365-2109.2007.01747.x
Cleveland, B. M., Weber, G. M., Blemings, K. P., and Silverstein, J. T. (2009). Insulin-like growth factor-I and genetic effects on indexes of protein degradation in response to feed deprivation in rainbow trout (Oncorhynchus mykiss). Am. J. Phys. Reg. Int. Comp. Phys. 297, R1332–R1342. doi: 10.1152/ajpregu.00272.2009
Cockrem, J. F. (2013). Corticosterone responses and personality in birds: individual variation and the ability to cope with environmental changes due to climate change. Gen. Comp. End. 190, 156–163. doi: 10.1016/j.ygcen.2013.02.021
Crain, D. A., Bolten, A. B., Bjorndal, K. A., Guillerte, L. J. Jr., and Gross, T. S. (1995). Size-dependent, sex-dependent, and seasonal changes in insulin-like growth factor I in the loggerhead sea turtle (Caretta caretta). Gen. Comp. Endocrinol. 98, 219–226. doi: 10.1006/gcen.1995.1063
Cucco, M., Ottonelli, R., Raviola, M., and Malacarne, G. (2002). Variations of body mass and immune function in response to food unpredictability in magpies. Acta Oecologica 23, 271–276. doi: 10.1016/S1146-609X(02)01154-2
Dantzer, B., and Swanson, E. M. (2012). Mediation of vertebrate life histories via insulin-like growth factor-1. Biol. Rev. 87, 414–429. doi: 10.1111/j.1469-185X.2011.00204.x
DiGirolamo, M., Edén, S., Enberg, G., Isaksson, O., Lönnroth, P., Hall, K., et al. (1986). Specific binding of human growth hormone but not insulin-like growth factors by human adipocytes. FEBS Lett. 205, 15–19. doi: 10.1016/0014-5793(86)80856-0
Dingemanse, N. J., and Dochtermann, N. A. (2013). Quantifying individual variation in behaviour: mixed-effect modelling approaches. J. Anim. Ecol. 82, 39–54. doi: 10.1111/1365-2656.12013
Duncan, C. A., Jetzt, A. E., Cohick, W. S., and John-Alder, H. B. (2015). Nutritional modulation of IGF-1 in relation to growth and body condition in Sceloporus lizards. Gen. Comp. Endocrinol. 216, 116–124. doi: 10.1016/j.ygcen.2015.02.009
Fauchald, P., Tveraa, T., Henaug, C., and Yoccoz, N. (2004). Adaptive regulation of body reserves in reindeer, Rangifer tarandus: a feeding experiment. Oikos 107, 583–591. doi: 10.1111/j.0030-1299.2004.12945.x
Fokidis, H. B., des Roziers, M. B., Sparr, R., Rogowski, C., Sweazea, K., and Deviche, P. (2012). Unpredictable food availability induces metabolic and hormonal changes independent of food intake in a sedentary songbird. J. Exp. Biol. 215, 2920–2930. doi: 10.1242/jeb.071043
Frystyk, J., Delhanty, P. J. D., Skjærbæk, C., and Baxter, R. C. (1999). Changes in the circulating IGF system during short-term fasting and refeeding in rats. Am. J. Physiol. Endocrinol. Metab. 277, E245–E252. doi: 10.1152/ajpendo.1999.277.2.E245
Gabillard, J., Kamangar, B. B., and Montserrat, N. (2006). Coordinated regulation of the GH/IGF system genes during refeeding in rainbow trout (Oncorhynchus mykiss). J. End. 191, 15–24. doi: 10.1677/joe.1.06869
Gao, H., Khawar, M. B., and Li, W. (2019). “Autophagy in reproduction,” in Autophagy: Biology and Diseases. Advances in Experimental Medicine and Biology, ed. Z. H. Qin (Singapore: Springer). doi: 10.1007/978-981-15-0602-4_21
Geiser, F., Holloway, J. C., and Körtner, G. (2007). Thermal biology, torpor and behaviour in sugar gliders: a laboratory-field comparison. J. Comp. Physiol. B 177, 495–501. doi: 10.1007/s00360-007-0147-6
Gosler, A. G. (1996). Environmental and social determinants of winter fat storage in the great tit Parus major. J. Anim. Ecol. 65, 1–17. doi: 10.2307/5695
Groscolas, R., and Robin, J. P. (2001). Long-term fasting and re-feeding in penguins. Comp. Biochem. Physiol. A Mol. Integr. Physiol. 128, 643–653. doi: 10.1016/S1095-6433(00)00341-X
Hadfield, J. D. (2010). MCMC methods for multi-response generalized linear mixed models: the MCMCglmm R package. J. Stat. Soft. 33, 1–22. doi: 10.18637/jss.v033.i02
Hargitai, R., Boros, N., Tóth, Z., and Lendvai, ÁZ. (2022). Food restriction delays breeding and affects insulin-like growth factor-1, oxidative damage, and haematocrit value before egg-laying in female canaries. J. Avian Biol. 2022, e02866.
Harshman, L. G., and Zera, A. J. (2007). The cost of reproduction: the devil in the details. Trends Ecol. Evol. 22, 80–86. doi: 10.1016/j.tree.2006.10.008
Hau, M., and Goymann, W. (2015). Endocrine mechanisms, behavioral phenotypes and plasticity: known relationships and open questions. Front. Zool. 12:S7. doi: 10.1186/1742-9994-12-S1-S7
Hocking, P. M., Bernard, R., Wilkie, R. S., and Goddard, C. (2007). Plasma growth hormone and insulin-like growth factor-I (IGF-I) concentrations at the onset of lay in ad libitum and restricted broiler breeder fowl. B. Poul. Sci. 35, 299–308. doi: 10.1080/00071669408417694
Houslay, T. M., Earley, R. L., Young, A. J., and Wilson, A. J. (2019). Habituation and individual variation in the endocrine stress response in the Trinidadian guppy (Poecilia reticulata). Gen. Comp. Endocrinol. 270, 113–122. doi: 10.1016/j.ygcen.2018.10.013
Houslay, T. M., and Wilson, A. J. (2017). Avoiding the misuse of BLUP in behavioural ecology. Behav. Ecol. 28, 948–952. doi: 10.1093/beheco/arx023
Kastin, A. J. (2013). Handbook of Biologically Active Peptides, 2nd Edn. Cambridge, MA: Academic Press.
Killen, S. S., Marras, S., and McKenzie, D. J. (2011). Fuel, fasting, fear: routine metabolic rate and food deprivation exert synergistic effects on risk-taking in individual juvenile European sea bass. J. Anim. Ecol. 80, 1024–1033. doi: 10.1111/j.1365-2656.2011.01844.x
Leili, S., Buonomo, F. C., and Scanes, C. G. (1997). The effects of dietary restriction on insulin-like growth factor (IGF)-I and II, and IGF-binding proteins in chickens. Proc. Soc. Exp. Biol. Med. 216, 104–111. doi: 10.3181/00379727-216-44162A
Lendvai, ÁZ., Ouyang, J. Q., Schoenle, L. A., Fasanello, V., Haussmann, M. F., Bonier, F., et al. (2014). Experimental food restriction reveals individual differences in corticosterone reaction norms with no oxidative costs. PLoS One 9:e110564. doi: 10.1371/journal.pone.0110564
Lendvai, ÁZ., Tóth, Z., Mahr, K., Osváth, G., Vogel-Kindgen, S., and Gander, B. A. (2021). Effects of experimental increase in insulin-like growth factor 1 on feather growth rate, moult intensity and feather quality in a passerine bird. J. Exp. Biol. 224, jeb242481. doi: 10.1242/jeb.242481
Lewin, N., Swanson, E. M., Williams, B. L., and Holekamp, K. E. (2016). Juvenile concentrations of IGF-1 predict life-history trade-offs in a wild mammal. Funct. Ecol. 31, 894–902. doi: 10.1111/1365-2435.12808
Lodjak, J., and Mägi, M. (2017). Crosstalk between growth and somatic maintenance in young animals. J. Avian Biol. 48, 1360–1363. doi: 10.1111/jav.01519
Lodjak, J., Mägi, M., and Tilgar, V. (2014). Insulin-like growth factor 1 and growth rate in nestlings of a wild passerine bird. Funct. Ecol. 28, 159–166. doi: 10.1111/1365-2435.12164
Lodjak, J., Tilgar, V., and Mägi, M. (2016). Does the interaction between glucocorticoids and insulin-like growth factor 1 predict nestling fitness in a wild passerine? Gen. Comp. Endocrinol. 225, 149–154. doi: 10.1016/j.ygcen.2015.10.016
Madliger, C. L., and Love, O. P. (2016). Employing individual measures of baseline glucocorticoids as population-level conservation biomarkers: considering within-individual variation in a breeding passerine. Conserv. Physiol. 4:cow048. doi: 10.1093/conphys/cow048
Mahr, K., Vincze, O., Tóth, Z., Hoi, H., and Lendvai, ÁZ. (2020). Insulin-like growth factor 1 is related to the expression of plumage traits in a passerine species. Behav. Ecol. Sociobiol. 74, 39. doi: 10.1007/s00265-020-2821-6
Mauch, E., Serão, N., Wolc, A., Bunter, K., and Dekkers, J. (2016). Juvenile IGF-I: an early bio-marker for feed efficiency in pigs. Anim. Ind. Rep. 13:662. doi: 10.31274/ans_air-180814-247
Morishita, D., Sasaki, K., Wakita, M., and Hoshino, S. (1993). Effect of fasting on serum insulin-like growth factor-I (IGF-I) levels and IGF-I-binding activity in cockerels. J. Endocrinol. 139, 363–370. doi: 10.1677/joe.0.1390363
Musarò, A., McCullagh, K. J. A., Naya, F. J., Olson, E. N., and Rosenthal, N. (1999). IGF-1 induces skeletal myocyte hypertrophy through calcineurin in association with GATA-2 and NF-ATc1. Nature 400, 581–585. doi: 10.1038/23060
O’Sullivan, U., Gluckman, P. D., Breier, B. H., Woodall, S., Siddiqui, R. A., and McCutcheon, S. N. (1989). Insulin-like growth factor-1 (IGF-1) in mice reduces weight loss during starvation. Endocrinology. 125, 2793–2794. doi: 10.1210/endo-125-5-2793
Owen, J. C. (2010). Collecting, processing, and storing avian blood: a review. J. Field Ornithol. 82, 339–354. doi: 10.1111/j.1557-9263.2011.00338.x
Petersson, E., Jaurvi, T., Steffner, N. G., and Ragnarsson, B. (1996). The effect of domestication on some life history traits of sea trout and Atlantic salmon. J. Fish Biol. 48, 776–791. doi: 10.1111/j.1095-8649.1996.tb01471.x
Pigliucci, M. (2001). Phenotypic Plasticity: Beyond Nature and Nurture. Baltimore, MD: Johns Hopkins University Press.
Puig, O., and Tjian, R. (2006). Nutrient availability and growth: regulation of insulin signaling by dFOXO/FOXO1. Cell Cycle 5, 503–505. doi: 10.4161/cc.5.5.2501
R core team (2019). R: A Language And Environment For Statistical Computing. Vienna: R Foundation for Statistical Computing.
Rahmani, J., Varkaneh, H. K., Clark, C., Zand, H., Bawadi, H., Ryan, P. M., et al. (2019). The influence of fasting and energy restricting diets on IGF-1 levels in humans: a systematic review and meta-analysis. Ageing Res. Rev. 53:100910. doi: 10.1016/j.arr.2019.100910
Reed, T. E., Wanless, S., Harris, M. P., Frederiksen, M., Kruuk, L. E. B., and Cunningham, E. J. A. (2006). Responding to environmental change: plastic responses vary little in a synchronous breeder. Proc. Roy. Soc. B. 273, 2713–2719. doi: 10.1098/rspb.2006.3631
Regan, J. C., Froy, H., Walling, C. A., Moatt, J. P., and Nussey, D. H. (2020). Dietary restriction and insulin-like signalling pathways as adaptive plasticity: a synthesis and re-evaluation. Funct Ecol. 34, 107–128. doi: 10.1111/1365-2435.13418
Robinson, J. J., Ashworth, C. J., Rooke, J. A., Mitchell, L. M., and McEvoy, T. G. (2006). Nutrition and fertility in ruminant livestock. Anim. Feed Sci. Technol. 126, 259–276. doi: 10.1016/j.anifeedsci.2005.08.006
Saltiel, A. R., and Kahn, C. R. (2001). Insulin signalling and the regulation of glucose and lipid metabolism. Nature 414, 799–806. doi: 10.1038/414799a
Scavo, L. M., Karas, M., Murray, M., and Leroith, D. (2004). Insulin-like growth factor-I stimulates both cell growth and lipogenesis during differentiation of human mesenchymal stem cells into adipocytes. J. Clin. Endocrinol. Metab. 89, 3543–3553. doi: 10.1210/jc.2003-031682
Schew, W. A., McNabb, F. M. A., and Scanes, C. G. (1996). Comparison of the ontogenesis of thyroid hormones, growth hormone, and insulin-like growth factor-i inad libitumand food-restricted (altricial) European starlings and (precocial) Japanese quail. Gen. Comp. Endocrinol. 101, 304–316. doi: 10.1006/gcen.1996.0033
Smith, P. J., Wise, L. S., Berkowitz, R., Wan, C., and Rubin, C. S. (1988). Insulin-like growth factor-I is an essential regulator of the differentiation of 3T3-L1 adipocytes. J. Biol. Chem. 263, 9402–9408.
Sparkman, A. M., Vleck, C. M., and Bronikowski, A. M. (2009). Evolutionary ecology of endocrine-mediated life-history variation in the garter snake Thamnophis elegans. Ecology 90, 720–728. doi: 10.1890/08-0850.1
Svensson, L. (1992). Identification Guide to European Passerines, 4th Edn. Thetford: British Trust for Ornithology.
Tighe, R. L., Bonde, R. K., and Avery, J. P. (2016). Seasonal response of ghrelin, growth hormone, and insulin-like growth factor I in the free-ranging Florida manatee (Trichechus manatus latirostris). Mamm. Biol. 81, 247–254. doi: 10.1016/j.mambio.2016.02.006
Timmer, L. T., Hoogaars, W. M. H., and Jaspers, R. T. (2018). “The role of IGF-1 signaling in skeletal muscle atrophy,” in Muscle Atrophy, Advances in Experimental Medicine and Biology, ed. J. Xiao (Singapore: Springer), 109–137. doi: 10.1007/978-981-13-1435-3_6
Tóth, Z., Ouyang, J. Q., and Lendvai, ÁZ. (2018). Exploring the mechanistic link between corticosterone and insulin-like growth factor-1 in a wild passerine bird. PeerJ 6:e5936. doi: 10.7717/peerj.5936
Vágási, C. I., Tóth, Z., Pénzes, J., Pap, P. L., Ouyang, J. Q., and Lendvai, ÁZ. (2020). The relationship between hormones, glucose, and oxidative damage is condition and stress dependent in a free-living passerine bird. Phys. Biochem. Zool. 93, 466–476. doi: 10.1086/711957
Valente, L. M. P., Moutou, K. A., Conceição, L. E. C., Engrola, S., Fernandes, J. M. O., and Johnston, I. A. (2013). What determines growth potential and juvenile quality of farmed fish species? Rev. Aquac. 5, S168–S193. doi: 10.1111/raq.12020
Valle, S., Carpentier, E., Vu, B., Tsutsui, K., and Deviche, P. (2015). Food restriction negatively affects multiple levels of the reproductive axis in male house finches, Haemorhous mexicanus. J. Experiment. Biol. 218, 2694–2704. doi: 10.1242/jeb.123323
Valle, S., Eagleman, D., Kieffer, N., and Deviche, P. (2020). Disruption of energy homeostasis by food restriction or high ambient temperature exposure affects gonadal function in male house finches (Haemorhous mexicanus). J Comp Physiol B 190, 611–628. doi: 10.1007/s00360-020-01295-0
van de Pol, M., and Wright, J. (2009). A simple method for distinguishing within- versus between-subject effects using mixed models. Anim. Behav. 77, 753–758. doi: 10.1016/j.anbehav.2008.11.006
Visser, M. E. (2008). Keeping up with a warming world; assessing the rate of adaptation to climate change. Proc. Biol. Sci. 275, 649–659. doi: 10.1098/rspb.2007.0997
Vitousek, M. N., Taff, C. C., Hallinger, K. K., Zimmer, C., and Winkler, D. W. (2018). Hormones and fitness: evidence for trade-offs in glucocorticoid regulation across contexts. Front. Ecol. Evol. 6:42. doi: 10.3389/fevo.2018.00042
Wang, R. C., and Levine, B. (2010). Autophagy in cellular growth control. FEBS Lett. 584, 1417–1426. doi: 10.1016/j.febslet.2010.01.009
Williams, T. D. (2008). Individual variation in endocrine systems: moving beyond the ‘tyranny of the Golden Mean.’. Philos. Trans. R. Soc. B Biol. Sci. 363, 1687–1698. doi: 10.1098/rstb.2007.0003
Witter, M. S., Swaddle, J. P., and Cuthill, I. C. (1995). Periodic food availability and strategic regulation of body mass in the European starling, Sturnus vulgaris. Funct. Ecol. 9, 568–574. doi: 10.2307/2390146
Keywords: insulin-like growth factor-1 (IGF-1), stress, Panurus biarmicus, body mass, endocrinology, nutrition
Citation: Tóth Z, Mahr K, Ölveczki G, Őri L and Lendvai ÁZ (2022) Food Restriction Reveals Individual Differences in Insulin-Like Growth Factor-1 Reaction Norms. Front. Ecol. Evol. 10:826968. doi: 10.3389/fevo.2022.826968
Received: 01 December 2021; Accepted: 27 May 2022;
Published: 01 July 2022.
Edited by:
John Cockrem, Massey University, New ZealandReviewed by:
Christine Duncan, La Roche University, United StatesChristine Lattin, Louisiana State University, United States
Copyright © 2022 Tóth, Mahr, Ölveczki, Őri and Lendvai. This is an open-access article distributed under the terms of the Creative Commons Attribution License (CC BY). The use, distribution or reproduction in other forums is permitted, provided the original author(s) and the copyright owner(s) are credited and that the original publication in this journal is cited, in accordance with accepted academic practice. No use, distribution or reproduction is permitted which does not comply with these terms.
*Correspondence: Zsófia Tóth, aHlsaWRhZTkxQGdtYWlsLmNvbQ==; Ádám Zoltán Lendvai, YXoubGVuZHZhaUBnbWFpbC5jb20=