- 1MTA-PE Evolutionary Ecology Research Group, University of Pannonia, Veszprém, Hungary
- 2Behavioral Ecology Research Group, Center for Natural Sciences, Faculty of Engineering, University of Pannonia, Veszprém, Hungary
- 3Fish and Conservation Ecology Research Group, Balaton Limnological Research Institute, Eötvös Loránd Research Network, Tihany, Hungary
- 4MTA-ELTE Comparative Ethology Research Group, Budapest, Hungary
- 5Theoretical Population Ecology and Evolution Group, Department of Biology, Lund University, Lund, Sweden
- 6Lendület Evolutionary Ecology Research Group, Plant Protection Institute, Centre for Agricultural Research, Eötvös Loránd Research Network, Budapest, Hungary
Climate change and urbanisation are among the most salient human-induced changes affecting Earth’s biota. Extreme weather events can have high biological impacts and are becoming more frequent recently. In cities, the urban heat island can amplify the intensity and frequency of hot weather events. However, the joint effects of heat events and urban microclimate on wildlife are unclear, as urban populations may either suffer more from increased heat stress or may adapt to tolerate warmer temperatures. Here, we test whether the effects of hot weather on reproductive success of great tits (Parus major) are exacerbated or dampened in urban environments compared to forest habitats. By studying 760 broods from two urban and two forest populations over 6 years, we show that 14–16 days-old nestlings have smaller body mass and tarsus length, and suffer increased mortality when they experience a higher number of hot days during the nestling period. The negative effects of hot weather on body mass and survival are significantly stronger in forests than in urban areas, where these effects are dampened or even reversed. These results suggest that urban nestlings are less vulnerable to extreme hot weather conditions than their non-urban conspecifics. This difference might be the result of adaptations that facilitate heat dissipation, including smaller body size, altered plumage and reduced brood size. Alternatively or additionally, parental provisioning and food availability may be less affected by heat in urban areas. Our findings suggest that adaptation to heat stress may help birds cope with the joint challenges of climate change and urbanisation.
Introduction
Current large-scale environmental changes are substantially influencing the ecological conditions for Earth’s biota. Climate change is one of the dominant global processes that affect a wide range of organisms. Its effects include systematic changes in the long-term average meteorological conditions and also increases in the frequency of extreme weather events like heatwaves, droughts, and heavy storms (Buckley and Huey, 2016; Drumond et al., 2020; Perkins-Kirkpatrick and Lewis, 2020). These climatic changes are occurring in a world that is experiencing global-scale transformation of natural habitats into heavily modified anthropogenic habitats. For example, current estimates show that 77% of the terrestrial habitats have already been modified by the direct effects of human activities such as agriculture and urbanisation (Watson et al., 2018), and the remaining pristine habitats are also disappearing at a high rate (Watson et al., 2016, 2018; Di Marco et al., 2019) forcing an increasing number of wildlife populations to persist in anthropogenic environments (Alberti and Marzluff, 2004). Although the ecological and evolutionary consequences of different global processes such as climate change and anthropogenic land-use conversion are most often investigated separately, they obviously do not act independently. Thus to better understand their current effects and to predict the changes likely induced in the future, we need to investigate their joint impacts in natural systems.
Heat events, including hot days and heatwaves, are among those extreme meteorological events that have become more frequent in the last few decades (Drumond et al., 2020). Extreme hot conditions can have strong biological impacts, affecting both survival and reproduction of organisms (Mora et al., 2017; Woodroffe et al., 2017; Conradie et al., 2019). For example, when hot days occurred for several consecutive days in Southern and Western Europe in 2003, it resulted in an estimated 70,000 heat-related human deaths (Robine et al., 2008) and was followed by detectable decreases in wild bird populations (Jiguet et al., 2006). However, even a single hot day can cause acute heat stress and have metabolic costs (Moagi et al., 2021). Extreme hot weather affects reproductive success negatively in a wide range of taxa, including both ectothermic (Zizzari and Ellers, 2011; Dayananda et al., 2017) and endothermic animals (Welbergen et al., 2008; Conrey et al., 2016; Marrot et al., 2017; Woodroffe et al., 2017; Van de Ven et al., 2020; Bourne et al., 2021; McCowan and Griffith, 2021; Oswald et al., 2021). These effects of extreme hot weather may be mediated by several, non-exclusive mechanisms, including both direct effects of heat stress on physiological and cognitive functioning (Welbergen et al., 2008; Danner et al., 2021; McCowan and Griffith, 2021) and indirect effects of heat via altered ecological conditions like food or water availability (du Plessis et al., 2012; Fuller et al., 2016).
Anthropogenic habitat change, especially urbanisation, is likely to interact with extreme weather events because it fundamentally alters several basic environmental conditions, including microclimate and food availability (Alberti and Marzluff, 2004; Seress and Liker, 2015). Urban heat island (UHI) refers to the generally higher ambient temperature in cities compared to the surrounding non-urban areas, which is largely generated by heat storage in buildings and sealed roads. Its intensity can be as high as +5°C in some metropolitan areas (Landsberg, 1981; Zhao et al., 2014; Central and Climate, 2021), so organisms in cities experience higher temperatures and more frequent heat events compared to organisms in non-urban areas. The UHI effect may interact with heat events in at least two ways. First, UHI can exacerbate the biological effects of extreme heat events. For example, models show that daytime temperature in temperate-zone cities during a heat event can be higher by, on average, 2.8°C as a result of the synergistic effect between UHI and heat events (Zhao et al., 2018). Indeed, heat stress in cities can be higher during a heat event than the sum of the background UHI effect and the heat-event effect (Li and Bou-Zeid, 2013). According to these model predictions, the number of hot days is higher in urban than in non-urban areas with an additional increase in UHI resulting in increased human mortality (Li and Bou-Zeid, 2013; Rizvi et al., 2019). Similarly, animals are more likely to reach their upper limit of thermal tolerance in cities during heat events than in non-urban areas (Krause et al., 2017). Thus, urban populations may suffer a stronger reduction in survival and reproductive success than non-urban populations due to hot days (“superimposed heat and UHI effects” hypothesis).
Second, it is also possible that during long-term exposure to UHI, urban populations become adapted to higher temperatures (“adaptation to UHI” hypothesis). For example, heat tolerance (expressed as the thermal maximum where animals become unable to coordinate their motor performance) is higher in urban compared to rural populations in various ectotherm species (Brans et al., 2017; Diamond et al., 2017; Campbell-Staton et al., 2020), and these local thermal adaptations can have consequences for their reproductive success (Meineke et al., 2013; Diamond et al., 2017; Martin et al., 2021). However, endotherm species have different constraints and mechanisms for thermoregulation compared to ectotherms, as endotherms employ not only behavioural but also a variety of physiological mechanisms to regulate body temperature which have significant energetic and hydric costs (Levesque and Marshall, 2021). Although there is much less data on urban thermal adaptations in endotherms, the smaller body size of the rodent Peromyscus maniculatus (Guralnick et al., 2020) and reduced number of feathers in some birds (Sándor et al., 2021) in urban populations might also reflect adaptations to the UHI.
So far, only a few studies have tested the above hypotheses for joint effects of extreme hot weather events and habitat urbanisation. Their results show either additional mortality in cities in humans (Li and Bou-Zeid, 2013) or adaptation to UHI effects in water fleas (Brans et al., 2017). Importantly, there is no field study for endotherm animal populations, despite that these are the most frequent targets of conservation efforts and play crucial roles in urban ecosystems. To help fill this knowledge gap, we tested whether heat events influence avian breeding success differently in cities and forest habitats. We analysed 6 years of breeding biology data of two urban and two forest populations of great tits (Parus major), a small songbird that frequently breeds in both urban and forest habitats. In this species, similarly to several other birds, reproductive performance is typically lower in urban sites than in more natural areas (Bailly et al., 2016; Seress et al., 2018). We tested whether nestlings’ body mass, tarsus length, and survival are related to the number of hot days differently in urban and forest sites. According to the “superimposed heat and UHI effects” hypothesis, we expect that heat events would result in stronger negative impacts on reproduction in urban than forest habitats. Alternatively, the “adaptation to UHI” hypothesis predicts that heat events would have reduced impact in urban than forest populations.
Materials and Methods
Data Collection
We installed nest-boxes for great tits in an urban site (city of Veszprém 47°05′17″N, 17°54′29″E), and in two sites in natural habitats (forests near Vilma-puszta 47°05′06.7″N, 17°51′51.4″E and Szentgál 47°06′39″N, 17°41′17″E) in 2012, and additionally in another urban site (Balatonfüred 46°57′30″N, 17°53′34″E) in 2013. Urban nest-boxes are located mostly in public parks, university campuses, and a cemetery, where vegetation contains both native and introduced plant species. Forest study sites are located in deciduous woodlands, characterized by beech Fagus sylvatica and hornbeam Carpinus betulus (in Szengtál) or downy oak Quercus cerris and South European flowering ash Fraxinus ornus (in Vilma-puszta). See detailed description of study sites in Seress et al. (2018). In March 2013, we installed a WH 2080 weather station (Ambient, LLC, AZ, United States) at each study site that recorded hourly temperature (°C) data throughout the 6 years of the study.
Great tits usually raise one or two broods per breeding season. We collected data on all breeding attempts at each site from 2013 to 2018, by recording the number of eggs and nestlings in the nest-boxes every 3–4 days from March to the end of July. There were 48 to 108 nest-boxes available in each study site, and 12 to 77% of these nest-boxes was occupied by great tits during the study period (depending on the site and year). We captured parent birds using a nest-box trap 6–15 days after their first nestling had hatched. We determined parents’ sex based on their plumage characteristics and banded each bird with a unique combination of a numbered metal band and three plastic color bands. Breeding adult birds that had been color-banded on previous occasions were identified from recordings made during the nestling period by using a small, concealed video camera attached to the nest-boxes (Seress et al., 2017). In these video samples, we considered a color-banded individual to be a parent bird if it was recorded to enter the nest-box with food at least once. Close to fledging (at day 14–16 post-hatch; day 1 being the hatching day of the first nestling in the brood), we measured nestlings’ body mass with a Pesola spring balance (±0.1 g) and tarsus length with a Vernier caliper (±0.1 mm).
All procedures applied during our study were in accordance with the guidelines for animal handling outlined by ASAB/ABS1 and Hungarian laws. We have all the required permissions for capturing, measuring of the birds and monitoring their breeding from the Government Office of Veszprém County, Nature Conservation Division (former Middle Transdanubian Inspectorate for Environmental Protection, Natural Protection and Water Management; permission number: 24861/2014 and VE-09Z/03454-8/2018) and from the National Scientific Ethical Committee on Animal Experimentation (permission number: VE/21/00480-9/2019).
Data Processing
We used both the first and second annual broods of each pair in the study. We omitted broods where we could not precisely identify the nestling period due to unknown dates of hatching and/or the failure of the entire brood before hatching, and also those broods where we had gaps in the temperature data for more than 2 days during the nestling period (n = 190 broods). To characterize breeding success in each brood, we focused on the size and survival of nestlings. Offspring mass is a good indicator of post-fledging survival in birds (Ronget et al., 2018), so we preferred this variable over indices of “body condition” that express body mass in relation to length, because the latter indices can be inadequate for quantifying “fatness” in growing nestlings (Kraft et al., 2019).
For analysing the effects of weather on nestling size, we used the average body mass and the average tarsus length of each brood as response variables, and we included broods where at least one offspring was alive at the time of nestlings’ measuring and banding (i.e., the age of 14–16 days). Because chick size varies with age, we omitted those few broods where the nestlings were measured before or after 14–16 days of nestling age (n = 30); thus we had n = 674 broods for nestling size analyses.
For analysing the effects of weather on nestling mortality, we used broods in which at least one offspring was alive on the third day after the hatching of the first nestling. We excluded a small number of broods (n = 7) that failed within the first 3 days after hatching because the average interval between our nest monitoring visits was 3 days, so estimating the number of hatched chicks and their survival before the first nest check after hatching would be very uncertain. We also excluded those broods where chick mortality was likely unrelated to weather conditions (n = 7), i.e., occurred due to predation or human disturbance (i.e., recorded during the next observation after the occasion of capturing the parent bird on its nest). This yielded n = 760 broods for nestling mortality analyses, from which 385 had no chick mortality, 319 had partial mortality, and 56 had complete brood loss during the nestling period.
We calculated all weather variables (average temperature and number of hot days, detailed below) for the nestling period of each brood individually. For investigating nestling size, weather variables were calculated for the period from the day of hatching of the first chick to the day preceding the day of banding and measuring the nestlings. This sample included only broods where banding occurred at age 14–16 days (mean ± SE: 14.04 ± 0.03). For analysing nestling mortality, we calculated weather variables for the period from the day of hatching of the first offspring to either the day preceding the day of recording the last nestling’s death or the day preceding the day of banding and measuring the nestlings (mean ± SE: 13.72 ± 0.07, range: 3 – 18 days). We calculated the average temperature as the mean of hourly temperatures over the nestling period. There are several methods for quantifying extreme hot weather (Bailey and van de Pol, 2016), using various thresholds from 21 to 43°C depending on the geographic location. We calculated the number of hot days during each nestling period using a threshold specific to our region. To calculate a threshold for hot days, first, we defined a reference period from the earliest hatching date to the latest chick banding date recorded in our 6-year data set (pooling all sites and years), which ranged from 9th April to 15th July. Then, to estimate the typical temperatures in our geographic area during this reference period, we used a 26-years dataset from an external reference weather station located in Szentkirályszabadja, a small village near Veszprém (47°57’06″ É, 17°58’10″ K, ca. 9.5 – 22 km from our study sites). This weather station is maintained by the Hungarian Meteorological Service and its temperature data are available from the National Oceanic and Atmospheric Administration database2, with records of air temperature every 3 h (0, 3, 6, 12, 15, 18, 21 UTC) since 1993. Using this temperature dataset, we calculated the 90% percentile of all daily maximum temperatures for the reference period from this 26-year long dataset, which was 28.7°C. Finally, we calculated the number of hot days as the number of days when the daily maximum temperature was higher than the 28.7°C threshold during the nestling period for each brood. Fixed temperature thresholds for calculating hot days are widely used in ecological and evolutionary research (Bailey and van de Pol, 2016) based on the assumption of a fixed threshold for heat tolerance in adults and offspring (Anderson, 2006; Gardner et al., 2017; Marrot et al., 2017; McCowan and Griffith, 2021). Since there is no proper information about critical thermal tolerance values for great tit nestlings, we used the 28.7°C air temperature threshold to define hot days based on the logic that temperatures above this value have been rarely experienced (i.e., less than 10 % of time) by the studied populations over the latest decades (and given the long-term trend for climate warming, such high temperatures were likely even rarer during their earlier past).
Statistical Analyses
Analysing the effects of extreme weather is challenging. Extreme events are rare by definition, so their distribution is strongly skewed (for distribution of our variable, see Supplementary Material, Section 1). Furthermore, weather variables are often correlated with each other and with other variables influencing reproductive success (e.g., with calendar date), so multicollinearity can be a problem in models containing multiple predictors. Statistical methods that can handle multicollinearity, such as covariance-based structural equation modelling, are less well suited for handling non-normal residual distributions and the non-independence structure of ecological data (e.g., multiple broods per pair). Therefore, here we used general and generalized linear mixed-effects (LME and GLMM) models and Akaike’s information-criterion (AIC) based model selection (Burnham and Anderson, 2002) to focus on the interaction between habitat type and the number of hot days in explaining reproductive variables. Mixed-effects models can be applied appropriately to non-normal and non-independent data, while model selection based on measures of the models’ total explanatory power (model fit indices, like AIC) is not sensitive to multicollinearity (Graham, 2003).
All analyses were run in R (version 4.0.0) (R Core Team, 2020). First, to examine the temperature characteristics of our study sites, we compared the number of hot days and the average temperature in the nestling periods (calculated separately for each brood) between urban and forest habitats. We constructed a generalized linear model with the generalized Poisson distribution to handle overdispersion for the number of hot days using “glmmTMB” package (Brooks et al., 2017), and a linear model for the average temperatures. In both models, study site (4 sites) and year (6 years) were the predictors. To statistically compare the mean of each meteorological variable between the two habitat types, we calculated marginal means from the models for each study site, then calculated a linear contrast for habitat comparison as the difference of the average of the two urban sites from the average of the two forest sites, using the “emmeans” R package (Lenth, 2018). We used this approach rather than including habitat type as a fixed effect and site as a random effect in the models because variance estimations of random effects with few levels are unreliable (Piepho et al., 2003; Bolker et al., 2008), whereas including both habitat type and site as fixed effects would have resulted in strong collinearity between these two factors (Dormann et al., 2013). Instead, we treated the four sites as if they were two control groups and two treatment groups in an experiment, and we used a pre-planned comparison to test the prediction that the two treatment (i.e., urban) groups would differ from the two control (i.e., forest) groups. Such pre-planned comparisons are a powerful approach for testing a priori hypotheses (Ruxton and Beauchamp, 2008).
To analyse average nestling mass and average tarsus length as response variables, we used the “lme” function of the “nlme” R package (Pinheiro et al., 2013) which assumes Gaussian error. For nestling mortality (proportion of hatched chicks that died by the age of 14–16 days) as the response variable, we used GLMM models using the “glmer” function of the “lme4” R package (Bates et al., 2015) with binomial error distribution and logit link function. All of our models contained pair identity as a random factor to control for the non-independence of broods produced by the same pair. Broods got the same pair ID if both the female and the male parents were the same, and a new pair ID was assigned to the brood when it had at least one different parent. Pairs where one or both parents were unidentifiable (n = 43 and 139, respectively) got separate pair IDs (see Supplementary Material, Section 1, Supplementary Table 1 for random effect of pair ID). There were 111 pairs of parents that had more than one brood in our dataset, ranging 2–6 broods/pair. Additionally, to handle overdispersion in models of nestling mortality, we used an observation-level random effect (Maindonald and Braun, 2010) and ran the models with the BOBYQA optimizer (Bates et al., 2015).
First, we built a simple model for each of the three response variables (nestling mass, tarsus length, and mortality) including only the number of hot days (used as a numeric covariate) and study site (used as a factor with four levels), and their two-way interaction as predictors. We carefully inspected all relevant diagnostics to ensure that model fit was adequate for all models (see Supplementary Material, Sections 2, 3). Then, to statistically compare the effect of hot days on nestling size and mortality between the two habitat types, we calculated the slopes of the relationship between reproductive success measurements and the number of hot days for each study population, and we compared the average of the two urban slopes with the average of the two forest slopes using a linear contrast.
To infer the robustness of the results from the simple models, we also built multi-predictor models which included further potentially important predictor variables. In all models of our three response variables, we included year as a categorical variable, the average temperature during the nestling period and hatching date as numeric covariates, the latter defined as the number of days elapsed from the 1st January annually to the hatching of the first nestling in each brood. We also added the quadratic term of hatching date, because a preliminary inspection of diagnostic graphs suggested the possibility of non-linear seasonal changes in the response variables. For models of nestling characteristics (i.e., average body mass and average tarsus length of nestlings), we additionally included brood age (i.e., the number of days elapsed from the hatching of the first chick to the day of nestlings’ measuring, ranging from 14 to 16 days), and brood size (i.e., the number of offspring alive at measuring). We standardized all numeric predictors using z-transformation for our multi-predictor analyses to avoid model convergence problems due to different scales of variables. Based on variance inflation factor (VIF), we detected high levels of multicollinearity in these multi-predictor models (see Supplementary Material, Section 4, Supplementary Table 2). Because multicollinearity with VIF values > ca. 2 can lead to unreliable standard errors, non-significant parameter estimates from such models must be treated with caution (Graham, 2003). Therefore, such models cannot be used for quantifying the effects of each predictor: for example, the number of hot days systematically increases with date, so the effects of hot days and other seasonal changes cannot be separated or compared by using our observational data. However, the set of predictors that yields the best explanatory power can be correctly identified by model selection based on model fit statistics (like AIC), even if multicollinearity is present (Burnham and Anderson, 2002). Therefore, we compared multi-predictor models to test if inclusion of any predictor increases model fit considerably, and then to see if our interaction of interest (urbanisation × number of hot days) is qualitatively altered by taking into account those extra predictors. We created a candidate model set for each response variable using all combinations of predictors but always including the number of hot days, study site, and their interaction (because this interaction was the focus of our study, and our goal with the model-selection procedure was to see if this interaction yields similar habitat contrasts in the multi-predictor models as alone in the simple models). The model set contained 33 models for nestling size (Supplementary Material, Section 5, Supplementary Tables 3, 4) and 9 models for nestling mortality (Supplementary Material, Section 5, Supplementary Table 5). We compared the models in each model set based on their AICc (AIC corrected for sample size) to identify the most supported model(s) for each response variable (Burnham and Anderson, 2002) using the “model.sel” function of the “MuMIn” R package (Barton, 2009). From the model(s) with superior support based on ΔAICc and Akaike weight, we calculated the slope for each study site and the linear contrast that compares the effect of hot days between urban and forest sites the same way as from the simple models.
Results
Habitat Differences in Heat Events
Great tit offspring experienced markedly different temperature characteristics in urban and forest sites during the nestling periods (Figure 1). The incidence of hot days occurring during the nestling period was 2.7 times greater in the cities than in the forests (urban-forest difference on log scale, controlled for year effects: mean ± SE: 0.99 ± 0.13, t = 7.39, df = 750, p < 0.001; Figure 1). At least one hot day occurred in 179 out of 390 (45.9%) urban broods and in 77 out of 370 (20.8%) forest broods. The number of hot days ranged 0–13 in urban broods, taking up a maximum of 86.7% of the total length of individual nestling periods. In contrast, forest broods experienced only 0–5 hot days, which made up a maximum of 35.7% of the nestling period (see Supplementary Material, Section 1 for further details). The highest maximum temperature was 40.1°C in urban nestling periods (in June 2013) and 33.6°C in forest nestling periods (in May 2014). Hot days were often on consecutive days during the nestling periods: there were 197 broods where at least two hot days occurred, and in 107 of these broods all hot days were consecutive (out of these 107 cases of “heatwaves,” 71 were at least 3 days long). The average temperatures within the nestling periods were significantly higher in the urban habitat (mean ± SD: 16.62 ± 4.29°C) than in the forest habitat (14.55 ± 2.90°C; urban-forest difference controlled for year effects: 1.52 ± 0.27°C, t = 5.74, df = 751, p < 0.001; Figure 1).
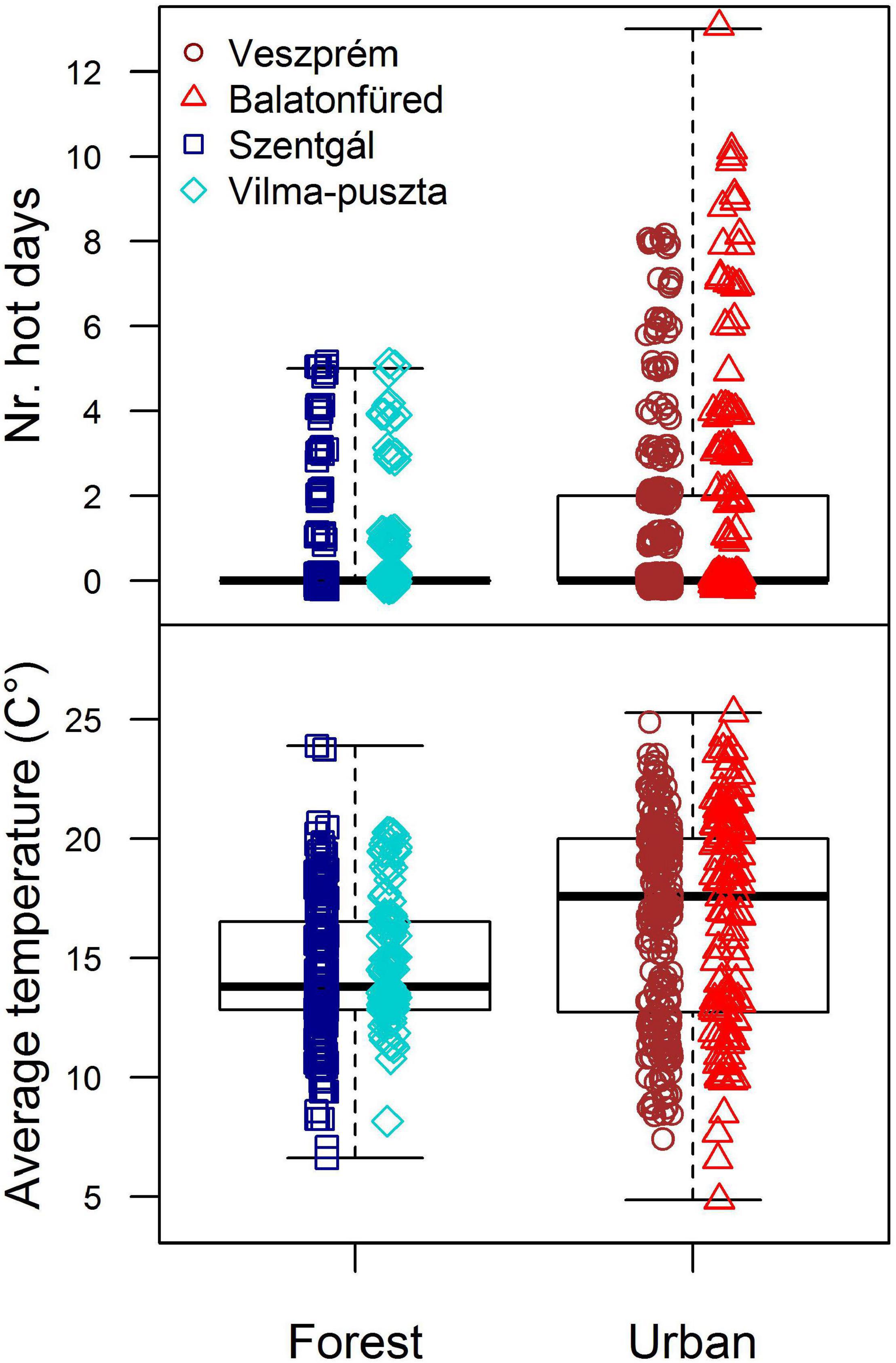
Figure 1. Distribution of the number of hot days and average temperature during the nestling periods in our study sites. Boxes show the interquartile range, the thick line is the median, and whiskers refer to the range of data distribution. Data points represent great tit broods (blue: forest, red: urban).
Effects of Hot Days on Reproductive Success
Nestling Body Mass
In the simple model where predictors were the number of hot days, study site and their two-way interaction, the effect of hot days on nestling mass differed between sites (as indicated by a significant interaction between sites and number of hot days, Table 1) and between urban and forest habitats (according to the linear contrast post hoc test comparing the effects between the two urban and the two forest populations, Table 2): increase in the number of hot days negatively affected nestling mass in forest broods while no such trend was present for the urban broods (Figure 2A). This result is consistent with the site-specific trends: the two forest populations had the most negative slopes for the relationship of nestling mass with the number of hot days, whereas the slope was flat or even positive in the two urban populations (Supplementary Material, Section 6, Supplementary Table 6). The habitat difference in heat effect remained significant when the analysis was restricted to the range of the number of hot days that occured in both habitats, i.e., to broods with a maximum of 5 hot days (Supplementary Material, Section, Supplementary Table 7).

Table 1. Relationship between the number of hot days during nestling development and nestlings’ body mass, tarsus length, and mortality, according to the simple models.
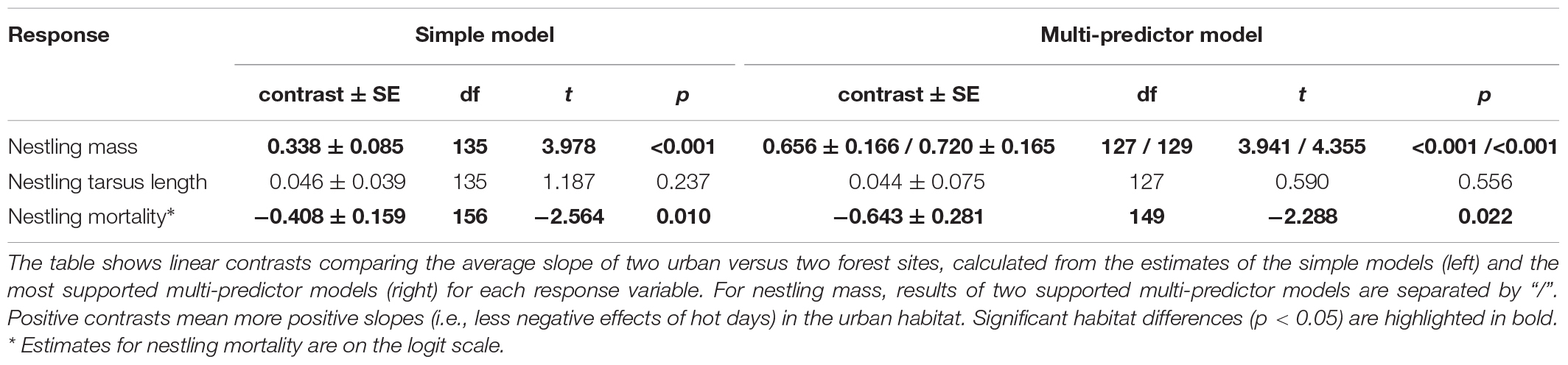
Table 2. Differences between urban and forest habitats in the effect of hot days on nestling’s body mass, tarsus length, and mortality.
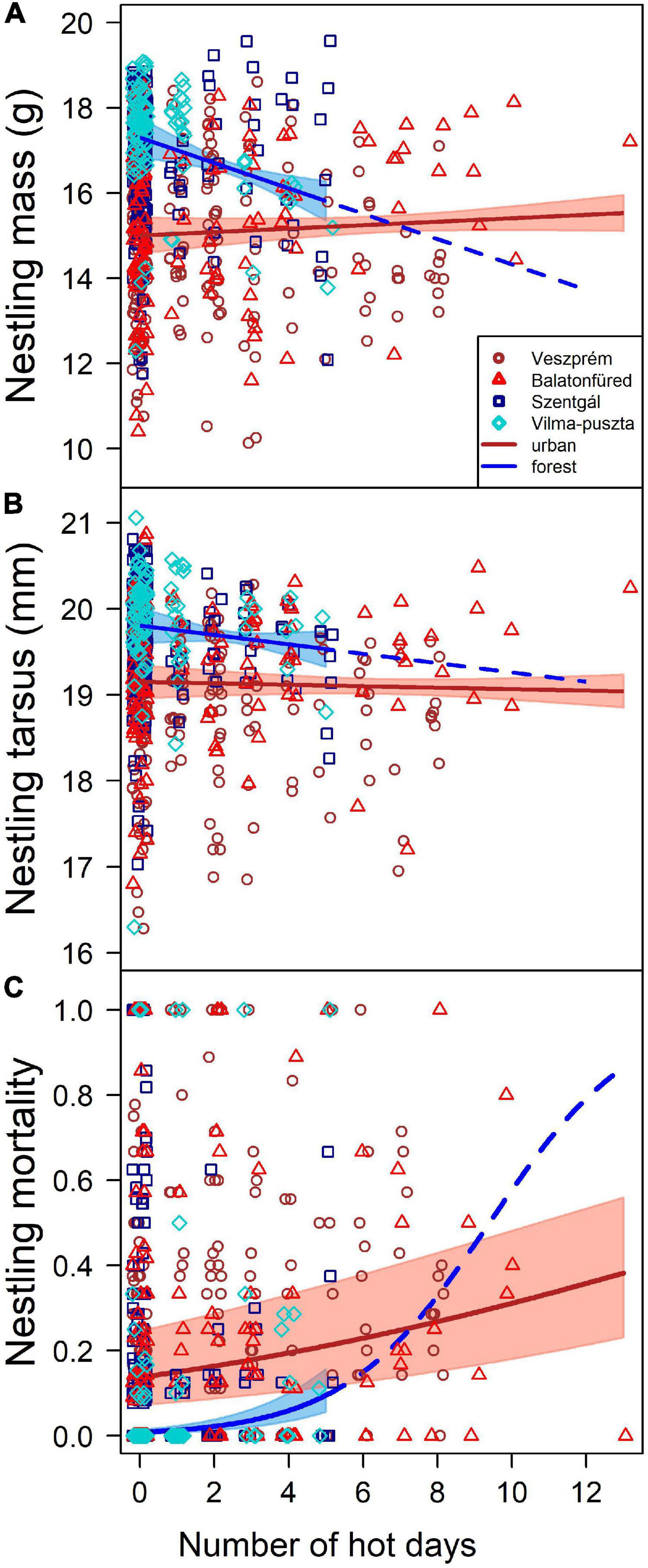
Figure 2. Relationships between the number of hot days during the nestling period and (A) body mass, (B) tarsus length, and (C) mortality of great tit nestlings. Symbols represent brood means for nestling mass and tarsus length. Colored stripes show the 95% confidence band of the slope estimated from the simple models (Supplementary Table 6). For forest broods, the fitted slopes are extrapolated for the range of 5–13 hot days (dashed lines) using the slopes estimated by the models from the observed data. Note that all urban-forest comparisons remained qualitatively the same when the analyses were restricted to a subset of data where the number of hot days ranged from 0 to 5 (see Supplementary Material, Section 7, Supplementary Table 7).
We also tested whether the effect of hot days persist when further potentially important variables (i.e., hatching date, year, brood size an brood age) are taken into account. We built a set of models containing various combinations of these variables in addition to the number of hot days, study site and their interaction, and we compared these models using the information-theoretic approach based on AICc. Model selection for these multi-predictor models resulted in two supported models for nestling mass (ΔAICc < 2; the model with the third best fit had ΔAICc = 4; Supplementary Table 3). The first supported model included year, brood age, and brood size besides the number of hot days, study sites, and their interaction; the second supported model contained the same predictors except brood size. Linear contrasts calculated from each of these supported multi-predictor models showed a significant overall habitat difference (Table 2) with a more negative effect of hot days in forest compared to urban populations (Supplementary Table 6), corroborating the results of the simple model.
Nestling Tarsus Length
In the simple model, the interaction between site and number of hot days was significant (Table 1), due to the difference between the two urban sites, with a positive slope in one site and negative in the other (Supplementary Table 6). The two forest sites both showed negative relationship between the number of hot days and nestling tarsus length (Supplementary Table 6). The linear contrast for urban-forest difference suggested no systematic habitat difference in the effect of hot days on the tarsus length of nestlings (Table 2 and Figure 2B).
Model selection for the multi-predictor model set resulted in a single supported model, which contained the number of hot days, study site, their interaction, the average temperature of the nestling period, brood age, and brood size (for the other models ΔAICc ≥ 6.68, Supplementary Table 4). The result of linear contrast comparing urban and forest slopes from this model was qualitatively identical to the result from the simple model, showing no consistent habitat difference in the effect of hot days on nestling tarsus length (Table 2 and Supplementary Table 6).
Nestling Mortality
The simple model showed that the effect of the number of hot days on nestling mortality differed significantly between study sites (Table 1). The slope of the relationship between nestling mortality and the number of hot days was positive in all populations, being highest in one of the forest sites and lowest in one of the urban sites (Supplementary Table 6). The slopes also differed between urban and forest populations with a stronger effect in the latter (Table 2 and Figure 2C), i.e., nestling mortality increased significantly more with the number of hot days in the forests than in the cities. The habitat difference in heat effect remained significant when the analysis was restricted to broods with a maximum of 5 hot days (Supplementary Table 7).
Multi-predictor model selection resulted in a single supported model, which was the full model of our model set excepting the quadratic hatching date (for the other models ΔAICc ≥ 42.87, Supplementary Table 5), containing the number of hot days, study site and their interaction, the average temperature of the nestling period, year and hatching date. The linear contrast calculated from this model showed a significantly steeper slope in forests compared to urban sites (Table 2), corroborating again the result of the simple model.
Discussion
Our study found clear differences between urban and non-urban populations of an urban exploiter passerine species in the effects of hot days on important fitness components. Although the influence of thermal environment on fitness has been shown to vary with habitat urbanisation in ectotherms (Martin et al., 2021), our study is the first to demonstrate such habitat-dependent responses to heat stress in an endothermic animal. While UHI caused a 1.19°C higher temperature on average and there were more hot days in the cities compared to forests (Supplementary Figure 1), hot days affected nestling mass and survival more negatively in the forest habitat type. These results are robust, as they are based on >600 broods from 6 years and four sites, and were qualitatively unchanged by taking into account several potentially confounding factors that may differ between urban and forest habitats, such as the start of breeding and brood size. These findings highlight that both lethal and sublethal effects of heat events (i.e., the proportion of surviving offspring and their quality in terms of body mass) can differ between urban and non-urban habitats. Although information about sublethal fitness costs of hot weather is scarce (Conradie et al., 2019), developing under hot weather conditions may have long-term consequences for fitness. Our results thus support the “adaptation to UHI” hypothesis and suggest that non-urban populations of birds (and possibly other vertebrates) are more vulnerable to extreme heat than urban populations.
Heat may affect the reproductive output of birds in at least two ways: directly through offspring physiology and indirectly through food availability. Firstly, the negative effects of hot days on offspring mass and survival may emerge due to the direct physiological consequences of heat stress. Nestlings usually cannot maintain stable body temperature in the first few days of their lives, and their metabolic processes also differ from those of adults (Price and Dzialowski, 2018) leaving them potentially more vulnerable to extreme heat than adults. The heat responses of dependent offspring are poorly known, although in birds the nestling phase seems to be the most relevant period during ontogeny regarding heat effects compared to the incubation and post-fledging periods (Marques-Santos and Dingemanse, 2020). In hot environments, individuals can less effectively dissipate the excess heat as a consequence of increased metabolic rate, leading to hyperthermia. Increasing metabolism causes decreased utilization of food and faster mobilization of energy reserves, thus it may cause lower body mass. Additionally, during heat stress, evaporative water loss is elevated as the organism tries to cool itself by evaporation to maintain body temperature in the physiologically normal range, causing dehydration (Weathers, 1972; Arad et al., 1989). Thus, increased metabolic rate and water loss both can lead to decreased body mass, as it has been shown in several bird species (Quinteiro-Filho et al., 2010; Kruuk et al., 2015; Corregidor-Castro and Jones, 2021; Oswald et al., 2021). Although parents can help their nestlings thermoregulate, great tits cannot shade their nest-boxes and they are not known to be able to cool their nestlings in other ways such as providing water.
These direct physiological effects of heat might be better tolerated by urban animals due to changes in their physiological and morphological characteristics. For example, we found in the same study populations that urban great tit nestlings have fewer feathers and increased bare body surfaces than forest nestlings (Sándor et al., 2021), which may facilitate heat dissipation. Reduced body size may also help to cope with the heat in cities because animals with smaller size have a higher surface-biomass ratio which facilitates heat loss. Bergmann’s rule (Bergmann, 1847) predicts that reduced size is beneficial against dehydration and overheating in warmer environments (Weathers, 1972; but see Whitfield et al., 2015) such as cities. This idea is supported by urban-rural comparisons of invertebrate communities (Merckx et al., 2018), as well as by a study of water flea populations which found that urban individuals were generally smaller than rural ones, and smaller individuals were more heat tolerant (Brans et al., 2017). In birds, a study of white-browed scrubwrens (Sericornis frontalis) also found that smaller individuals survived better when more extreme hot and dry events occurred (Gardner et al., 2017). Urban birds are usually smaller than their non-urban conspecifics (Liker et al., 2008; Chamberlain et al., 2009; Caizergues et al., 2021) and this is also the case with the great tits in our study populations (Seress et al., 2018). Although experimental evidence shows that low availability of nestling food is a major cause of the reduced size of urban great tit nestlings (Seress et al., 2020), selection for better heat tolerance might also contribute to it. Finally, life-history changes may also help animals to cope with the direct effects of urban heat. For example, urban birds usually have smaller clutch size than forest birds (Chamberlain et al., 2009), which is also typical in our study populations (Seress et al., 2018, 2021). Smaller clutch size may also be advantageous for heat dissipation when environmental temperature is high because fewer offspring produce less heat and have more space to maintain distance in the nest, which helps better heat conduction.
Secondly, indirect effects may also contribute to lower body mass and higher mortality in response to heat events. For example, hot weather may reduce food availability for insectivorous nestlings by affecting their parents and/or their prey. Caterpillars are the main source of nestling diet in great tits and many other birds, and increasing temperature strongly decreases the time to pupation in several lepidopteran species (Kingsolver et al., 1997; Lee and Roh, 2010; Lemoine et al., 2015), so optimal caterpillar food may be available for a shorter time in hot periods. Moreover, the growth of caterpillars declines rapidly above a critical temperature (Kingsolver et al., 1997) and their mortality increases when temperature is constantly high (York and Oberhauser, 2002; Lee and Roh, 2010). These heat effects may not only result in less food for nestlings but may also contribute to dehydration because caterpillars are the most water-rich items in the diet of nestlings (Zandt, 1997). These effects might be stronger in forests where the amount of available caterpillar prey is much higher in general (Seress et al., 2018) and the vast majority of nestling diet consists of caterpillars. In such environments, it might be more difficult for parent birds to compensate for a reduction in caterpillar biomass compared to cities, where nestlings are usually fed a greater proportion of other food types (Pollock et al., 2017; Jarrett et al., 2020). Water loss due to the combined effects of heat and reduced caterpillar availability might also be better compensated for in urban habitats if water is more accessible there, for example from public or garden ponds, bird water feeders, or puddles left behind after lawn irrigation. In our study, the nest-box colony in Balatonfüred is situated at ca. 700 m from Lake Balaton, and there is a small pond near some of our urban nest-boxes in Veszprém study site, while we are not aware of any permanent water sources in the forest study sites. However, we have no information on whether and how frequently the supplementary water sources are used by urban birds. Additionally, the foraging activity of parents may also decrease when temperature is high (Wiley and Ridley, 2016; Nilsson and Nord, 2018; Oswald et al., 2021), resulting in less food delivered to the offspring, and this effect might also be diminished in urban populations if parent birds have adapted to tolerate heat better (see above), or when they have more access to water than in non-urban forests. Further studies are required to test whether heat affects parental care differentially in urban and non-urban habitats.
Adaptation to constantly warmer urban environments may be achieved by microevolution, epigenetic changes, and/or phenotypic plasticity (Zhu et al., 2021). In some ectotherm species, artificial thermal selection, reciprocal transplant and common garden experiments have revealed that one or more of these mechanisms can play a role in the increased upper thermal tolerance of urban populations (Calosi et al., 2008; Brans et al., 2017; Campbell-Staton et al., 2020; Martin et al., 2021). To our knowledge, no such experiments have yet been done to identify the mechanisms of higher thermal tolerance in urbanised birds or other endothermic animals, which is an important knowledge gap in our understanding of urban adaptations. Such experiments are also needed for quantifying the relative importance of heat per se and other environmental effects that may change over the season and may differ between urban and non-urban habitats, including parental investment and the availability of food and water, which was not possible with our correlative data here. Therefore, there is a great need for further studies that explore the differences in thermal tolerance between different habitats to understand how wild animals are affected by, and may adapt to, the interacting challenges of climate change and urbanisation in the Anthropocene.
Data Availability Statement
The raw data supporting the conclusions of this article will be available from the authors upon request.
Ethics Statement
The animal study was reviewed and approved by the Government Office of Veszprém County, Nature Conservation Division (former Middle Transdanubian Inspectorate for Environmental Protection, Natural Protection and Water Management; permission number: 24861/2014 and VE-09Z/03454-8/2018), and the National Scientific Ethical Committee on Animal Experimentation (permission number: VE/21/00480-9/2019).
Author Contributions
IP, VB, and AL designed the study, did the statistical analyses, and wrote the manuscript. IP and VB made the data visualization. All authors collected data in the field and took part in finalizing the manuscript.
Funding
This project was primarily financed by the National Research, Development and Innovation Office of Hungary (NKFIH, grants K84132, K112838, and K132490 to AL and K135016 to VB), and by the Hungarian Academy of Sciences. IP was supported by the ÚNKP-20-4 New National Excellence Programme of the Ministry of Innovation and Technology from the Source of the National Research, Development and Innovation Fund. VB was supported by the János Bolyai Scholarship of the Hungarian Academy of Sciences. BP was supported by the 471-3/2021 grant of NKFIH. GS was supported by the FK137743 grant of NKFIH. EV was supported by the PD-134985 grant of NKFIH and by the MSCA EF Seal of Excellence IF-2019 grant by Vinnova, the Swedish Governmental Agency for Innovation Systems (grant number 2021-01102). KS was supported by MTA-ELTE Comparative Ethology Research Group (F01/031). Previous versions of this study have appeared in a doctoral thesis (Pipoly, 2020) and online as a preprint (https://www.biorxiv.org/content/10.1101/2020.01.29.924332v2).
Conflict of Interest
The authors declare that the research was conducted in the absence of any commercial or financial relationships that could be construed as a potential conflict of interest.
Publisher’s Note
All claims expressed in this article are solely those of the authors and do not necessarily represent those of their affiliated organizations, or those of the publisher, the editors and the reviewers. Any product that may be evaluated in this article, or claim that may be made by its manufacturer, is not guaranteed or endorsed by the publisher.
Acknowledgments
We thank earlier members and students of our research group, and several field assistants for helping during fieldwork.
Supplementary Material
The Supplementary Material for this article can be found online at: https://www.frontiersin.org/articles/10.3389/fevo.2022.825410/full#supplementary-material
Footnotes
References
Alberti, M., and Marzluff, J. M. (2004). Ecological resilience in urban ecosystems: linking urban patterns to human and ecological functions. Urban Ecosyst. 7, 241–265.
Anderson, T. (2006). Biology of the Ubiquitous House Sparrow: From Genes to Populations. Oxford: Oxford University Press.
Arad, Z., Horowitz, M., Eylath, U., and Marder, J. (1989). Osmoregulation and body fluid compartmentalization in dehydrated heat-exposed pigeons. Am. J. Physiol. Regul. Integr. Comp. Physiol. 257, 377–382. doi: 10.1152/ajpregu.1989.257.2.R377
Bailey, L. D., and van de Pol, M. (2016). Tackling extremes: challenges for ecological and evolutionary research on extreme climatic events. J. Anim. Ecol. 85, 85–96. doi: 10.1111/1365-2656.12451
Bailly, J., Scheifler, R., Berthe, S., Clément-Demange, V.-A., Leblond, M., Pasteur, B., et al. (2016). From eggs to fledging: negative impact of urban habitat on reproduction in two tit species. J. Ornithol. 157, 377–392.
Barton, K. (2009). Mu-MIn: Multi-model inference. R package. Available online at: https://CRAN.R-project.org/package=MuMIn
Bates, D., Maechler, M., Bolker, B., and Walker, S. (2015). Fitting linear mixed-effects models using lme4. J. Stat. Softw. 67, 1–48.
Bergmann, C. (1847). Ueber die verhältnisse der wärmeökonomie der thiere zu ihrer grösse. Gottinger Stud. 3, 595–708.
Bolker, B. M., Brooks, M. E., Clark, C. J., Geange, S. W., Poulsen, J. R., Stevens, M. H. H., et al. (2008). Generalized linear mixed models?: a practical guide for ecology and evolution. Trends Ecol. Evol. 24, 127–135. doi: 10.1016/j.tree.2008.10.008
Bourne, A. R., Cunningham, S. J., Nupen, L. J., Mckechnie, A. E., and Ridley, A. R. (2021). No sex-specific differences in the influence of high air temperatures during early development on nestling mass and fledgling survival in the Southern Pied Babbler (Turdoides bicolor). IBIS 164, 304–312. doi: 10.1111/ibi.12990
Brans, K., Jansen, M., Vanoverbeke, J., Tüzün, N., Stoks, R., and De Meester, L. (2017). The heat is on: genetic adaptation to urbanization mediated by thermal tolerance and body size. Glob. Change Biol. 23, 5218–5227. doi: 10.1111/gcb.13784
Brooks, M. E., Kristensen, K., van Benthem, K. J., Magnusson, A., Berg, C. W., Nielsen, A., et al. (2017). glmmTMB balances speed and flexibility among packages for zero-inflated generalized linear mixed modeling. R J. 9, 378–400.
Buckley, L. B., and Huey, R. B. (2016). Temperature extremes: geographic patterns, recent changes, and implications for organismal vulnerabilities. Glob. Change Biol. 22, 3829–3842. doi: 10.1111/gcb.13313
Burnham, K. P., and Anderson, D. R. (2002). Model Selection and Multi-Model Inference: A Practical Information-Theoretic Approach, 2nd Edn. New York, NY: Springer.
Caizergues, A. E., Charmantier, A., Lambrechts, M. M., Perret, S., Demeyrier, V., Lucas, A., et al. (2021). An avian urban morphotype: how the city environment shapes great tit morphology at different life stages. Urban Ecosyst. 24, 929–941.
Calosi, P., Bilton, D. T., and Spicer, J. I. (2008). Thermal tolerance, acclimatory capacity and vulnerability to global climate change. Biol. Lett. 4, 99–102. doi: 10.1098/rsbl.2007.0408
Campbell-Staton, S. C., Winchell, K. M., Rochette, N. C., Fredette, J., Maayan, I., Schweizer, R. M., et al. (2020). Parallel selection on thermal physiology facilitates repeated adaptation of city lizards to urban heat islands. Nat. Ecol. Evol. 4, 652–658. doi: 10.1038/s41559-020-1131-8
Central and Climate (2021). Hot Zones: Urban heat islands. Research Brief by Climate Central. Princeton, NJ: Central and Climate, 10.
Chamberlain, D. E., Cannon, A. R., Toms, M. P., Leech, D. I., Hatchwell, B. J., and Gaston, K. J. (2009). Avian productivity in urban landscapes: a review and meta-analysis. IBIS 151, 1–18.
Conradie, S. R., Woodborne, S. M., Cunningham, S. J., and McKechnie, A. E. (2019). Chronic, sublethal effects of high temperatures will cause severe declines in southern African arid-zone birds during the 21st century. Proc. Natl. Acad. Sci. U.S.A. 116, 14065–14070. doi: 10.1073/pnas.1821312116
Conrey, R. Y., Skagen, S. K., Yackel Adams, A. A., and Panjabi, A. O. (2016). Extremes of heat, drought and precipitation depress reproductive performance in shortgrass prairie passerines. IBIS 158, 614–629.
Corregidor-Castro, A., and Jones, O. R. (2021). The effect of nest temperature on growth and survival in juvenile Great Tits Parus major. Ecol. Evol. 11, 7346–7353. doi: 10.1002/ece3.7565
Danner, R. M., Coomes, C. M., and Derryberry, E. P. (2021). Simulated heat waves reduce cognitive and motor performance of an endotherm. Ecol. Evol. 11, 2261–2272. doi: 10.1002/ece3.7194
Dayananda, B., Murray, B. R., and Webb, J. K. (2017). Hotter nests produce hatchling lizards with lower thermal tolerance. J. Exp. Biol. 220, 2159–2165. doi: 10.1242/jeb.152272
Di Marco, M., Ferrier, S., Harwood, T. D., Hoskins, A. J., and Watson, J. E. M. (2019). Wilderness areas halve the extinction risk of terrestrial biodiversity. Nature 573, 582–585. doi: 10.1038/s41586-019-1567-7
Diamond, S. E., Chick, L., Perez, A. B. E., Strickler, S. A., and Martin, R. A. (2017). Rapid evolution of ant thermal tolerance across an urban-rural temperature cline. Biol. J. Linnean Soc. 121, 248–257. doi: 10.1111/eva.12826
Dormann, C. F., Elith, J., Bacher, S., Buchmann, C., Carl, G., Carré, G., et al. (2013). Collinearity?: a review of methods to deal with it and a simulation study evaluating their performance. Ecography 36, 27–46. doi: 10.1111/j.1600-0587.2012.07348.x
Drumond, A., Margarida, L. R., Reboita, M. S., and Taschetto, A. S. (2020). Weather and climate extremes: current developments. Atmosphere 11, 11–14.
du Plessis, K. L., Martin, R. O., Hockey, P. A. R., Cunningham, S. J., and Ridley, A. R. (2012). The costs of keeping cool in a warming world: Implications of high temperatures for foraging, thermoregulation and body condition of an arid-zone bird. Glob. Change Biol. 18, 3063–3070. doi: 10.1111/j.1365-2486.2012.02778.x
Fuller, A., Mitchell, D., Maloney, S. K., and Hetem, R. S. (2016). Towards a mechanistic understanding of the responses of large terrestrial mammals to heat and aridity associated with climate change. Clim. Change Responses 3, 1–19.
Gardner, J. L., Rowley, E., De Rebeira, P., De Rebeira, A., and Brouwer, L. (2017). Effects of extreme weather on two sympatric australian passerine bird species. Philos. Trans. R. Soc. B 372:20160148.
Graham, M. H. (2003). Confronting multicollinearity in ecological multiple regression. Ecology 84, 2809–2815.
Guralnick, R., Hantak, M. M., Li, D., and McLean, B. S. (2020). Body size trends in response to climate and urbanization in the widespread North American deer mouse, Peromyscus maniculatus. Sci. Rep. 10, 1–13. doi: 10.1038/s41598-020-65755-x
Jarrett, C., Powell, L. L., McDevitt, H., Helm, B., and Welch, A. J. (2020). Bitter fruits of hard labour: diet metabarcoding and telemetry reveal that urban songbirds travel further for lower-quality food. Oecologia 193, 377–388. doi: 10.1007/s00442-020-04678-w
Jiguet, F., Julliard, R., Thomas, C. D., Dehorter, O., Newson, S. E., and Couvet, D. (2006). Thermal range predicts bird population resilience to extreme high temperatures. Ecol. Lett. 9, 1321–1330. doi: 10.1111/j.1461-0248.2006.00986.x
Kingsolver, J. G., Woods, H. A., Kingsolver, J. G., and Woods, H. A. (1997). Thermal sensitivity of growth and feeding in manduca sexta caterpillars. Physiol. Zool. 70, 631–638. doi: 10.1086/515872
Kraft, F. L. O. H., Driscoll, S. C., Buchanan, K. L., and Crino, O. L. (2019). Developmental stress reduces body condition across avian life-history stages: a comparison of quantitative magnetic resonance data and condition indices. Gen. Comp. Endocrinol. 272, 33–41. doi: 10.1016/j.ygcen.2018.11.008
Krause, J. S., Chmura, H. E., Wingfield, J. C., Lisovski, S., Word, K. R., González-Gómez, P. L., et al. (2017). How birds cope physiologically and behaviourally with extreme climatic events. Philos. Trans. R. Soc. B 372, 20160140. doi: 10.1098/rstb.2016.0140
Kruuk, L. E. B., Osmond, H. L., and Cockburn, A. (2015). Contrasting effects of climate on juvenile body size in a Southern Hemisphere passerine bird. Glob. Change Biol. 21, 2929–2941. doi: 10.1111/gcb.12926
Lee, K. P., and Roh, C. (2010). Temperature-by-nutrient interactions affecting growth rate in an insect ectotherm. Entomol. Exp. Appl. 136, 151–163.
Lemoine, N. P., Capdevielle, J. N., and Parker, J. D. (2015). Effects of in situ climate warming on monarch caterpillar (Danaus plexippus) development. PeerJ 3:e1293.
Lenth, R. V. (2018). emmeans: Estimated Marginal Means, aka Least-Squares Means. R package version 1.2.3. Available online at: https://CRAN.R-project.org/package=emmeans
Levesque, D. L., and Marshall, K. E. (2021). Do endotherms have thermal performance curves? J. Exp. Biol. 224(Pt 3):jeb141309. doi: 10.1242/jeb.141309
Li, D., and Bou-Zeid, E. (2013). Synergistic interactions between urban heat islands and heat waves: the impact in cities is larger than the sum of its parts. J. Appl. Meteorol. Climatol. 52, 2051–2064.
Liker, A., Papp, Z., Bókony, V., and Lendvai, ÁZ. (2008). Lean birds in the city: Body size and condition of house sparrows along the urbanization gradient. J. Anim. Ecol. 77, 789–795. doi: 10.1111/j.1365-2656.2008.01402.x
Maindonald, J., and Braun, J. (2010). Data Analysis and Graphics Using R: An Example-Based Approach, 3rd Edn. Cambridge: Cambridge University Press.
Marques-Santos, F., and Dingemanse, N. J. (2020). Weather effects on nestling survival of great tits vary according to the developmental stage. J. Avian Biol. 51, 1–12.
Marrot, P., Garant, D., and Charmantier, A. (2017). Multiple extreme climatic events strengthen selection for earlier breeding in a wild passerine. Philos. Trans. R Soc. Lond. B Biol. Sci. 372:20160372. doi: 10.1098/rstb.2016.0372
Martin, R. A., Chick, L. D., Garvin, M. L., and Diamond, S. E. (2021). In a nutshell, a reciprocal transplant experiment reveals local adaptation and fitness trade-offs in response to urban evolution in an acorn-dwelling ant. Evolution 75, 876–887. doi: 10.1111/evo.14191
McCowan, L. S. C., and Griffith, S. C. (2021). Baked eggs: catastrophic heatwave induced reproductive failure in the desert-adapted Zebra Finch (Taeniopygia guttata). IBIS 163, 1207–1216.
Meineke, E. K., Dunn, R. R., Sexton, J. O., and Frank, S. D. (2013). Urban warming drives insect pest abundance on street trees. PLoS One 8:e59687. doi: 10.1371/journal.pone.0059687
Merckx, T., Souffreau, C., Kaiser, A., Baardsen, L. F., Backeljau, T., Bonte, D., et al. (2018). Body-size shifts in aquatic and terrestrial urban communities. Nature 558, 113–116. doi: 10.1038/s41586-018-0140-0
Moagi, L. L., Bourne, A. R., Cunningham, S. J., Jansen, R., Ngcamphalala, C. A., Ganswindt, A., et al. (2021). Hot days are associated with short-term adrenocortical responses in a southern African arid-zone passerine bird. J. Exp. Biol. 224, 1–6. doi: 10.1242/jeb.242535
Mora, C., Dousset, B., Caldwell, I. R., Powell, F. E., Geronimo, R. C., Bielecki, C. R., et al. (2017). Global risk of deadly heat. Nat. Clim. Change 7, 501–506.
Nilsson, J. -Å, and Nord, A. (2018). Testing the heat dissipation limit theory in a breeding passerine. Proc. R. Soc. B 285, 1–8. doi: 10.1098/rspb.2018.0652
Oswald, K. N., Smit, B., Lee, A. T. K., Peng, C. L., Brock, C., and Cunningham, S. J. (2021). Higher temperatures are associated with reduced nestling body condition in a range-restricted mountain bird. J. Avian Biol. 52, 1–10.
Perkins-Kirkpatrick, S. E., and Lewis, S. C. (2020). Increasing trends in regional heatwaves. Nat. Commun. 11, 1–8. doi: 10.1038/s41467-020-16970-7
Piepho, H. P., Büchse, A., and Emrich, K. (2003). A Hitchhiker’s guide to mixed models for randomized experiments. Agron. Crop Sci. 189, 310–322. doi: 10.1046/j.1439-037x.2003.00049.x
Pinheiro, J., Bates, D., DebRoy, S., and Sarkar, D. (2013). NLME: linear and nonlinear mixed effects models. Version 3, 1–336.
Pipoly, I. (2020). Environmental and Genetic Predictors of Reproductive Success and Sex Ratios in Vertebrates. Doctoral thesis, University of Pannonia.
Pollock, C. J., Capilla-Lasheras, P., McGill, R. A. R., Helm, B., and Dominoni, D. M. (2017). Integrated behavioural and stable isotope data reveal altered diet linked to low breeding success in urban-dwelling blue tits (Cyanistes caeruleus). Sci. Rep. 7:5014. doi: 10.1038/s41598-017-04575-y
Price, E. R., and Dzialowski, E. M. (2018). Development of endothermy in birds: patterns and mechanisms. J. Comp. Physiol. B 188, 373–391. doi: 10.1007/s00360-017-1135-0
Quinteiro-Filho, W. M., Ribeiro, A., Ferraz-de-Paula, V., Pinheiro, M. L., Sakai, M., Sá, L. R. M., et al. (2010). Heat stress impairs performance parameters, induces intestinal injury, and decreases macrophage activity in broiler chickens. Poultry Sci. 89, 1905–1914. doi: 10.3382/ps.2010-00812
Rizvi, S. H., Alam, K., and Iqbal, M. J. (2019). Spatio -temporal variations in urban heat island and its interaction with heat wave. J. Atmospheric Solar Terrestrial Phys. 185, 50–57.
Robine, J. M., Cheung, S. L. K., Le Roy, S., Van Oyen, H., Griffiths, C., Michel, J. P., et al. (2008). Death toll exceeded 70,000 in Europe during the summer of 2003. Comptes Rendus Biol. 331, 171–178. doi: 10.1016/j.crvi.2007.12.001
Ronget, V., Gaillard, J., Coulson, T., and Garratt, M. (2018). Causes and consequences of variation in offspring body mass?: meta-analyses in birds and mammals. Biol. Rev. 93, 1–27. doi: 10.1111/brv.12329
Ruxton, G. D., and Beauchamp, G. (2008). Time for some a priori thinking about post hoc testing. Behav. Ecol. 19, 690–693.
Sándor, K., Liker, A., Sinkovics, C., Péter, Á, and Seress, G. (2021). Urban nestlings have reduced number of feathers in Great Tits (Parus major). IBIS 163, 1369–1378.
Seress, G., and Liker, A. (2015). Habitat urbanization and its effects on birds. Acta Zool. Acad. Sci. Hungaricae 61, 373–408.
Seress, G., Hammer, T., Bókony, V., Vincze, E., Preiszner, B., Pipoly, I., et al. (2018). Impact of urbanization on abundance and phenology of caterpillars and consequences for breeding in an insectivorous bird. Ecol. Appl. 28, 1143–1156. doi: 10.1002/eap.1730
Seress, G., Sándor, K., Evans, K. L., and Liker, A. (2020). Food availability limits avian reproduction in the city: an experimental study on great tits Parus major. J. Anim. Ecol. 89, 1570–1580. doi: 10.1111/1365-2656.13211
Seress, G., Sándor, K., Vincze, E., Pipoly, I., Bukor, B., Ágh, N., et al. (2021). Contrasting effects of the COVID-19 lockdown on urban birds’ reproductive success in two cities. Sci. Rep. 11, 1–10. doi: 10.1038/s41598-021-96858-8
Seress, G., Vincze, E., Pipoly, I., Hammer, T., Papp, S., Preiszner, B., et al. (2017). Effects of capture and video-recording on the behavior and breeding success of Great Tits in urban and forest habitats. J. Field Ornithol. 88, 299–312. doi: 10.1111/jofo.12205
Van de Ven, T. M. F. N., Fuller, A., and Clutton-Brock, T. H. (2020). Effects of climate change on pup growth and survival in a cooperative mammal, the meerkat. Funct. Ecol. 34, 194–202.
Watson, J. E. M., Allan, J. R., Venter, O., Lee, J., Jones, K. R., Robinson, J. G., et al. (2018). Protect the last of the wild. Nature 563, 27–30.
Watson, J. E. M., Shanahan, D. F., Di Marco, M., Allan, J., Laurance, W. F., Sanderson, E. W., et al. (2016). Catastrophic declines in wilderness areas undermine global environment targets. Curr. Biol. 26, 2929–2934. doi: 10.1016/j.cub.2016.08.049
Weathers, W. W. (1972). Physiological thermoregulation in heat-stressed birds: consequences of body sizes. Physiol. Zool. 54, 345–361.
Welbergen, J. A., Klose, S. M., Markus, N., and Eby, P. (2008). Climate change and the effects of temperature extremes on Australian flying-foxes. Proc. R. Soci. B 275, 419–425. doi: 10.1098/rspb.2007.1385
Whitfield, M. C., Smit, B., Mckechnie, A. E., and Wolf, B. O. (2015). Avian thermoregulation in the heat?: scaling of heat tolerance and evaporative cooling capacity in three southern African arid-zone passerines. J. Exp. Biol. 218, 1705–1714. doi: 10.1242/jeb.121749
Wiley, E. M., and Ridley, A. R. (2016). The effects of temperature on offspring provisioning in a cooperative breeder. Anim. Behav. 117, 187–195.
Woodroffe, R., Groom, R., and McNutt, J. W. (2017). Hot dogs: high ambient temperatures impact reproductive success in a tropical carnivore. J. Anim. Ecol. 86, 1329–1338. doi: 10.1111/1365-2656.12719
York, A., and Oberhauser, K. (2002). Effects of duration and timing of heat stress on monarch butterfly (Danaus plexippus) (Lepidoptera?: Nymphalidae) development. J. Kansas Entomol. Soc. 75, 290–298.
Zandt, H. S. (1997). Water content of prey of nestling Blue tits in a Corsican habitat. Netherlands J. Zool. 47, 125–131.
Zhao, L., Lee, X., Smith, R. B., and Oleson, K. (2014). Strong contributions of local background climate to urban heat islands. Nature 511, 216–219. doi: 10.1038/nature13462
Zhao, L., Oppenheimer, M., Zhu, Q., Baldwin, J. W., Ebi, K. L., Bou-Zeid, E., et al. (2018). Interactions between urban heat islands and heat waves. Environ. Res. Lett. 13:034003.
Zhu, L., Hoffmann, A. A., Li, S. M., and Ma, C. S. (2021). Extreme climate shifts pest dominance hierarchy through thermal evolution and transgenerational plasticity. Funct. Ecol. 35, 1524–1537.
Keywords: climate change, heatwave, urban heat island (UHI), offspring size, offspring mortality, thermal tolerance
Citation: Pipoly I, Preiszner B, Sándor K, Sinkovics C, Seress G, Vincze E, Bókony V and Liker A (2022) Extreme Hot Weather Has Stronger Impacts on Avian Reproduction in Forests Than in Cities. Front. Ecol. Evol. 10:825410. doi: 10.3389/fevo.2022.825410
Received: 30 November 2021; Accepted: 23 February 2022;
Published: 23 March 2022.
Edited by:
Francois Vezina, Université du Québec à Rimouski, CanadaReviewed by:
Sylvie Massemin, UMR 7178 Institut Pluridisciplinaire Hubert Curien (IPHC), FranceMichał S. Wojciechowski, Nicolaus Copernicus University, Poland
Copyright © 2022 Pipoly, Preiszner, Sándor, Sinkovics, Seress, Vincze, Bókony and Liker. This is an open-access article distributed under the terms of the Creative Commons Attribution License (CC BY). The use, distribution or reproduction in other forums is permitted, provided the original author(s) and the copyright owner(s) are credited and that the original publication in this journal is cited, in accordance with accepted academic practice. No use, distribution or reproduction is permitted which does not comply with these terms.
*Correspondence: Ivett Pipoly, cGlwb2x5Lml2ZXR0QG1rLnVuaS1wYW5ub24uaHU=; András Liker, YWxpa2VyQGFsbW9zLnVuaS1wYW5ub24uaHU=
†These authors share senior authorship