- 1Pollinator Health Lab, Department of Horticulture, Oregon State University, Corvallis, OR, United States
- 2Honey Bee Lab, Department of Horticulture, Oregon State University, Corvallis, OR, United States
Estimates of pesticide application hazards have grown to be one of the most common methodologies for evaluating the impact of pest management practices on honey bees. Typically, hazards are estimated by calculating a Hazard Quotient (HQ), which is based on acute toxicity data for different pesticides and the quantity of those pesticides applied to a field or detected on bees and matrices associated with their hive (honey, wax, pollen, and/or bee bread). Although use of HQ is widespread, there have been few reviews of this methodology, particularly with focus on how effective this method is at predicting effects of pesticides on hives. We evaluated 36 relevant papers, containing calculations of HQ to estimate hazards to honey bees. We observed that HQ was primarily calculated using two different approaches: (1) from the concentration of pesticides in the food, hive, or tissues of honey bees or (2) using the field application rate of the active ingredient as the estimation of pesticide hazard. Within and between HQ calculation methods, thresholds vary widely with some HQ thresholds set below 1 and others set at 10,000. Based on our review we identify key weakness with current HQ methodology and how studies relate HQ to honey bee health endpoints. First, HQ thresholds from studies of pesticides in hives are not based on the same pesticide consumption models from the EPA, potentially overestimating the risk of impacts to colonies. Conversely, HQ estimates calculated from field application rates are not based on eco-toxicological estimates of field exposure, resulting in an overestimation of pesticide reaching colonies. We suggest it is for these reasons that there is poor correspondence between HQ and field-level honey bee health endpoints. Considering these challenges, HQ calculations should be used cautiously in future studies and more research should be dedicated to field level exposure models.
Introduction
Environmental hazards and risks are key concepts in quantifying how dangerous pesticides are to honey bees. These concepts are frequently confused, particularly, as we demonstrate in this paper, across the growing number of studies looking to quantify the effects of field applied pesticides on honey bees.
Environmental risk assessment is the process of determining the consequences of pesticide applications for environmental quality, including non-target organisms like honey bees. The risk of a pesticide to honey bees represents the likelihood that that the colony will be negatively impacted by the treatment when applied to a given crop at a given rate. Case in point is a recent risk assessment framework developed jointly by the United States Environmental Protection Agency (EPA), California Department of Pesticide Regulation (CDPR) and the Canadian Pest Management Regulatory Agency (PMRA) (US EPA, 2012; EPA, 2014). The framework allows for the estimation of risk as the likelihood that a bee will visit the treated crop and collect sufficient pesticide that it will be harmful to the colony. The estimate relies on a combination of exposure models, laboratory tests, and if necessary, tests that represent an increasingly realistic exposure a colony would experience if situated adjacent to a treated crop. EPA risk assessment involves tiers, beginning with the most simplistic and conservative estimates which generate expected environmental concentrations and toxicity estimates from lab studies on individual bees (tier one). Higher tiers (two and three) refine both the expected environmental concentration and estimate effects on the colony level. The goal of each tier is to be conservative with risk estimations that maximize potential environmental concentrations and to use higher tiers to refine exposure estimates (US EPA, 2012; EPA, 2014). Notably, this framework replaced an earlier method of evaluation that estimated the hazards of a pesticide, defined as the potential for harm. Unlike risk assessment, estimates of the hazard of a pesticide to honey bees is based on the laboratory toxicity of the product alone, and does not incorporate information about the likelihood of exposure and how exposure translates into harm of the colony.
In parallel to the regulatory shift in assessing the risks of pesticides to honey bees, there has been intense interest in quantifying the effects of pesticides in terms of a hazard quotient (HQ). HQ quantifies the total hazards associated with actual or expected concentrations of pesticides in the environment or bee matrices. These amounts are then related to the LD50 values of the pesticides detected (Thompson, 2021). The widespread use of this method in honey bee toxicology followed Stoner and Eitzer (2013) who calculated HQ for pollen trapped from honey bee hives. In order to discern which levels of pesticides were of concern, they assigned threshold values, above which harm to honey bees would be expected. Thompson (2021), however, noted that the threshold values from HQ studies frequently do not align with levels deemed of concern using the risk assessment framework from regulators. Thompson concluded that HQ thresholds likely overestimate the risk of pesticides to honey bees, casting doubt on the validity of HQ.
Although the purpose of this review is not to compare the EPA’s BeeREX Risk Quotient (RQ) with the use of HQ in the literature (see Thompson, 2021 for this analysis), it is important to note key similarities and differences between RQ and HQ. Both HQ and RQ are assessments intended to trigger more investigation if a particular threshold is exceeded. However, unlike HQ, RQ estimates exposure from a dietary consumption model which incorporates the expected levels of pesticide in bee diets with the chronic and acute toxicity and feeding rates of each bee caste (EPA, 2014). HQ uses thresholds derived, in most cases, from a 10-day nursing period of adult bees and only adult acute toxicity data. The insights of Thompson (2021) point to deeper issues associated with the widespread use of HQ in the ecotoxicological literature. While Thompson identified the failure of literature which focuses on pesticide contaminated hive matrices to account for actual consumption patterns, we believe there are further problems associated with the current interpretation of HQ estimations.
This review will provide additional information on the role of HQ within the literature and the challenges associated with using HQ to understand how management practices are linked to changes in pesticide risk. As indicated by Thompson (2021), RQ may be a more appropriate method of estimating pesticide risk to bees from detections in bee food resources. However, while RQ provides an understanding of the dietary impacts of single chemicals on honey bee health, HQ can be used to understand factors which RQ does not consider (i.e., wax and bee bodies), including additive hazard from multiple chemical residues. Contextualizing HQ calculations and providing insight into the limitations of HQ as it related to hive health and landscape use patterns can help future authors refine questions around HQ. Our analysis reviews each paper for how HQ was calculated, the way HQ calculations incorporated landscape level honey bee foraging patterns and interpreted the impacts of specific HQ levels on honey bee colony health.
Review Process and Methods
This review assesses the use of HQ in understanding pesticide risk to the European honey bee, Apis mellifera. A literature search was performed with three search engines; the resulting publications were filtered for inclusion.
First, papers were retrieved using searches for “Hazard Quotient,” “Apis mellifera,” “Hazard Ratio,” and “honey bee” in PubMed, Web of Science, and Google Scholar. Literature referencing Stoner and Eitzer (2013) was included in the review by searching for papers which cited this paper. In total, this process produced 306 papers.
Next, criteria for inclusion in this review were developed. First, all papers included in this review were interested in pesticide hazard to Apis mellifera. Each paper included in the review calculated HQ and provided adequate information on how HQ was calculated. This reduced the number to about 150 papers. Second, all papers included in this review were published in peer reviewed journals. Those in industry publications, reports, or meeting notes were excluded. Duplicates were removed at this stage, resulting in 44 papers. Next, papers which did not provide enough information about HQ calculations in relation to study design were excluded from this review, narrowing the pool to 36 total papers included in this review.
Each HQ calculation within this review is considered distinct. This choice was made because many papers included HQ calculations for multiple bee matrixes or scenarios. Separating these calculations allowed individual analysis of HQ calculations and threshold values within each matrix analyzed.
How Hazard Quotient was Calculated
Hazard Quotient (HQ) was calculated across all papers using two parameters: quantity of pesticide in the bee environment and the toxicity of the pesticide (in micrograms per bee).
The actual concentration of pesticide is most commonly parts per billion of pesticide within bee matrices. The expected concentration of pesticide is most commonly the field application rate (grams of active ingredient/hectare). However, as noted in Thompson (2021), HQ is considered a unitless value. Toxicity was estimated either as oral or contact acute toxicity, represented as the dose required to kill 50% of bees in laboratory assays (LD50; Table 1). Although HQ was occasionally estimated for single pesticides, most studies calculated HQ across multiple pesticides by summing together the HQ for each pesticide (HQsum).
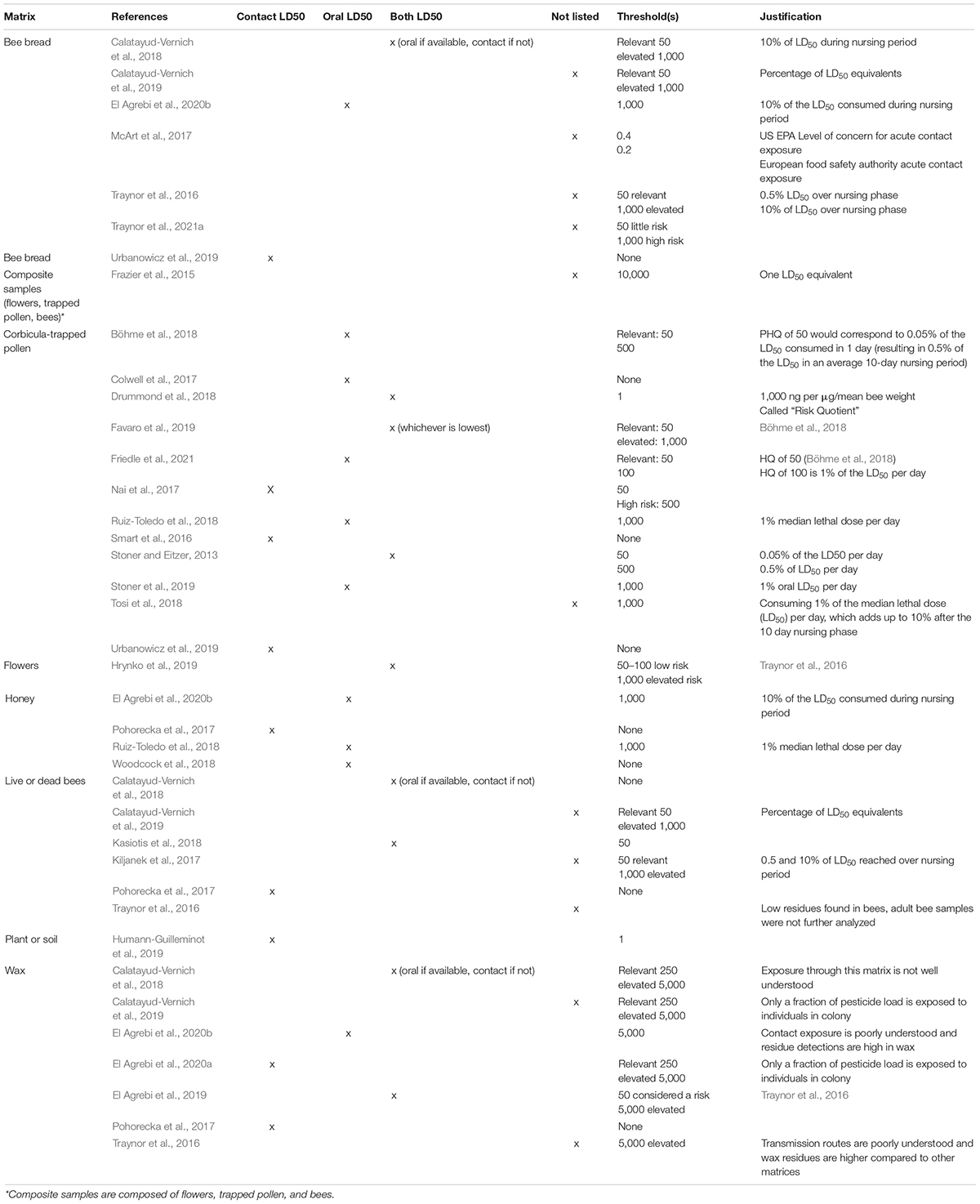
Table 1. A table displaying the contact or oral LD50 value, thresholds associated, and justifications for the thresholds, if provided.
Thresholds were commonly used to indicate the hazards that would likely have negative impacts to honey bee health (Tables 1, 2).
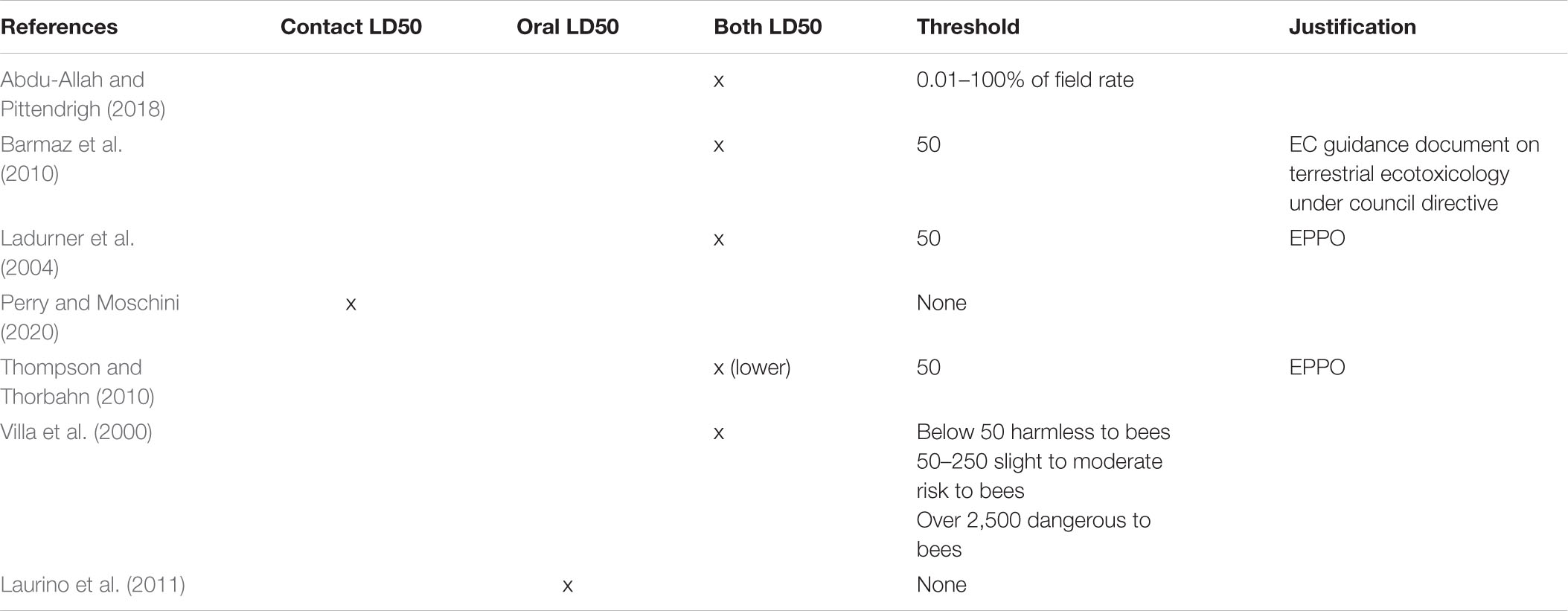
Table 2. A table displaying the contact or oral LD50 value, thresholds associated, and justifications for the thresholds, if provided for HQ calculations from the field application rate.
Hazard Quotient Calculated From Pesticide Detections in Bee Matrices
The most common way HQ was calculated across the studies was by measuring pesticide residue(s) within a bee matrix (i.e., wax, pollen, honey). This type of calculation was performed in 28 studies that were reviewed (Table 1). The pesticide residue was calculated from concentrations of pesticide found either in the locations that bees are likely to visit (i.e., nectar and pollen collected from flowers in Hrynko et al., 2019; foliage in Humann-Guilleminot et al., 2019) or from bee matrices.
Four matrices are commonly focused in the literature were pollen (33% of studies), bee bread (17%), wax (17%), and live or dead bee bodies (15%) (Table 1). Approximately one third of papers in this review calculate HQ in more than one bee matrix. For each of these matrices pesticide hazard is estimated by taking the concentration of pesticide in the matrix and relating that to the adult LD50 of the pesticide; this is a measurement of acute toxicity. There are also differences in the use of an oral or contact LD50 of a pesticide to calculate HQ. Contact LD50 values have historically been more readily available and were therefore used in the 23% of HQ studies. Some studies chose to use the lower, more conservative LD50 value for a pesticide when available, regardless of the likelihood of oral or contact exposure (Traynor et al., 2016). Finally, it is important to note that studies which test bee matrices for pesticides are primarily observational studies which monitor for pesticides in the bee environment, which have recognized limitations and biases; primarily that inferences are weaker in observational studies and replication is challenging to achieve (Eberhardt and Thomas, 1991).
Thresholds differ significantly in the literature for HQ calculated from honey bee matrices (Table 1). Many studies, approximately 65%, set no threshold for at least one level and at least one matrix (e.g., Böhme et al., 2018); sets a relevant threshold of 50 for pollen detections of HQ but no elevated threshold. Papers frequently set different thresholds for each matrix calculated for HQ. The most common thresholds set are HQ 50 as a relevant threshold (37%) and HQ 1,000 as an elevated threshold (37%). The heterogeneity of threshold values seen in this review is indicative of the lack of mechanistic understanding of how a HQ value moves from a pesticide detection to a potential impact on hive health.
Biases Associated With Different Bee Matrices
Two different terms around threshold HQ values were introduced by Traynor et al. (2016) to provide more nuanced understanding of HQ; relevant and elevated threshold values. The authors consider HQ value at or below the relevant threshold is considered harmlessness. HQ values above the relevant threshold are considered potentially harmful. The authors designated elevated thresholds to indicate unacceptable levels of risk. These thresholds demonstrate one way in which HQ studies attempt to estimate risk; thresholds implicitly make a connection between a pesticide detection level and the likelihood that a given pesticide is likely to cause harm.
The thresholds set for HQ at the hive are based on the percent of the LD50 reached in an approximated bee diet, as discussed in Stoner and Eitzer (2013). Stoner and Eitzer (2013) assumed the following: a honey bee adult consumes 9.5 mg of pollen per day throughout her 10-day nursing period; if the bee consumes a pollen diet of HQ 50, a bee will consume 0.05% of her LD50 each day; Kasiotis et al. (2018) built on this, noting this consumption would result an in an accumulated risk of death of 0.5% over her nursing period (Kasiotis et al., 2018). It is concerning to use acute LD50 values to understand metrics of chronic exposure as there is a mismatch in the toxicity metric of an acute LD50 and a threshold based on 10-day period. As discussed in Thompson (2021), these calculations and thresholds do not align with exposure models based on average pollen consumption rates, such as EPA’s BeeREX model and are instead based on other metrics of individual or colony health. While LD50 equivalents used in HQ thresholds are based on similar feeding models for adult nurse bees used in the Bee-REX model, Thompson (2021) found that using the same detections in bee matrices, HQ overestimated hazard when compared to EPA standards. Justifications for thresholds are based in percentages of LD50 equivalents (Calatayud-Vernich et al., 2019) or an expectation of imminent colony death due to high worker mortality (Drummond et al., 2018).
Studies commonly set different thresholds values for different types of matrices. The reason for these differences is rooted in a recognition of potential unequal exposure of bees to pesticides contained in different matrices. For example, relevant and elevated thresholds used for beeswax are commonly set higher than thresholds in other matrix types, owing to the slower release of pesticides to bees in wax compared to pesticides obtained from eating contaminated honey or pollen (Traynor et al., 2016; Pohorecka et al., 2017; Calatayud-Vernich et al., 2018, 2019). Wax relevancy thresholds are commonly set at 1,000 and wax elevated thresholds at 5,000 (Traynor et al., 2016; Calatayud-Vernich et al., 2017, 2018, 2019; Pohorecka et al., 2017). Notably, none of the papers base their threshold values on empirical estimates of the relative or absolute exposure of bees to pesticides in wax compared to other matrices.
The most common way of estimating pesticide hazard in terms of HQ is by trapping pollen from bees as they return to the hive using external pollen trapping equipment. By intercepting pollen before it reaches the hive environment, this matrix may best represent the external pesticide hazard across the bee’s foraging environment. Honey bees are generalist foragers known to travel 3 km away from the hive and trapped pollen can be used to sample the landscape for pesticide usage (Couvillon et al., 2014; Richardson et al., 2015). Trapped pollen can be sorted by color, homogenizing pollen species within the color group (Böhme et al., 2018; Stoner et al., 2019) and can be identified through microscopy and acetolysis (Topitzhofer et al., 2019). However, measuring pesticide residues from pollen in this way has limitations; low pollen availability or poor foraging weather can lead to insufficient pollen collected from traps (Topitzhofer et al., 2019). Moreover, as pollen traps or only engaged for short periods of time (typically 24–48 h) they may over or underestimate prolonged exposure to a pesticide, depending on whether traps are engaged when pesticide application is taking place. For example, Drummond et al. (2018) trapped pollen for a week of each 2-month period, which may not be reflective of the pesticide detections throughout the entire season; it is possible to miss pesticide emissions or to capture rare pesticide emissions and generalize these to the entire study period.
Collection of comb-stored pollen (bee bread) provides an alternative method of sampling pollen that estimates of pesticide hazard over a longer period than is possible using pollen trapping. Comb-stored pollen is processed by bees for long-term storage in the hive; it is packed into cells for storage and mixed with a small amount of nectar (Winston, 1987). Comb-stored pollen is most often collected by opening the hive and scraping “fresh” pollen out of the comb (Traynor et al., 2016; Drummond et al., 2018) and extracting the desired quantity. Bee bread becomes the food for the larvae, nurse bees, and the queen within the colony; therefore, using bee bread for estimation of hazard provides an estimate of pesticide load for the bees consuming this matrix; however, HQ detections from bee bread lack a mechanistic model of the inter-hive mechanisms through which the social aspects of honey bee feeding occurs (Sponsler and Johnson, 2017).
Wax is the structural matrix of the hive secreted by bees used to both store food and rear larvae; frames of drawn comb are commonly exchanged between hives in beekeeping practices (Winston, 1987; Calatayud-Vernich et al., 2017). Wax has a higher lipid content than pollen or honey and may be able to accumulate pesticides more readily (Mullin et al., 2010); even if environmental exposure is low, pesticides can accumulate in wax comb. Using wax to understand pesticide hazard provides valuable insights into an exposure pathway that is currently absent in risk assessment models. El Agrebi et al. (2020b) found that brood comb wax had the highest HQ values of all wax types they studied, indicating potential for exposure of developing larvae. Wax may become contaminated in several ways. First, beekeepers routinely apply miticides in the hive to control Varroa destructor and these chemicals have been detected at potentially concerning levels (El Agrebi et al., 2019). Understanding how pesticides in wax may become bioavailable to bees is nuanced; in vitro studies which examine realistic pesticide exposure in wax have done so through contaminated diet fed to larval honey bees where diet concentrations of pesticides were based off detections in wax and pollen, resulting in reduced survival of larvae and altering gene expression of detoxifying enzymes (Tomé et al., 2020). However, in a study where wax was removed from contaminated colonies and brood development was tracked, no significant impacts on larvae were found (Alkassab et al., 2020). Wax may be contaminated with pesticides via food sources (i.e., wax absorbs pesticides from contaminated pollen and nectar). There is evidence that bee bread and honey have higher HQ values after contact with contaminated wax due to the lipophilic nature of wax and the high levels of contamination common in honey bee wax (Calatayud-Vernich et al., 2017). Finally, even new wax secreted by bees has detectable pesticide loads, indicating that bees may be excreting pesticides from their bodies into this matrix (Calatayud-Vernich et al., 2017). Therefore, wax may be a pesticide sink where bees excrete pesticides into their environment and simultaneously wax may be a source of contamination increasing pesticide residues in bee diets.
Bee bodies can be sampled from within the hive or taken from suspected pesticide poisoning events. Both sampling scenarios present significant biases that are recognized in the literature (Traynor et al., 2016; Pohorecka et al., 2017). The amount of pesticide found on bees likely varies by the age of bees, given that older foraging bees are more likely to have direct contact with pesticides than nurse bees, which have never left the hive. Yet, determining the age of bees while sampling is nearly impossible; commonly, bees are sampled from the broodnest in order to standardize these factors (Traynor et al., 2016). Finally, HQ studies based on bee cadavers vs. live bees are expected to yield different results given that the metabolic processes within live bees begins degrading the pesticide rapidly (Magesh et al., 2017) and therefore any residues left over in the bee body could underestimate pesticide exposure.
Hazard Quotient Calculated From Field Application Rates
HQ was calculated from field application rates in eight studies that were reviewed (Table 2).
Studies calculating HQ from field application rates used both a combination of oral and contact LD50 values (Table 2). Thompson and Thorbahn (2010) advocate for the use of whatever LD50 is lower (oral or contact) in order to be as conservative as possible.
Thresholds set for HQ calculated from field application rates also vary within the literature (Table 2). Elevated thresholds are uncommon in this methodology; only two of the eight studies included in this review set elevated thresholds. In six of the eight studies included, the relevant threshold of HQ was set at 50. HQ values of 50 are rooted in EPPO regulatory guidelines (European and Mediterranean Plant Protection Organization [EPPO], 2010; US EPA, 2012) and Thompson and Thorbahn (2010) which used HQ calculations and poisoning events to validate thresholds in relation to poisoning events. The EPPO regulations outline a threshold below which a product is not deemed in need of risk assessment. It is, at its core, a conservative filter to remove relatively non-toxic or non-attractive products out of the framework for approval.
Hazard Quotient and Agronomic Management Practices
In current literature, HQ and landscape analysis are used in combination to address: (1) where is pesticide exposure occurring in the landscape and/or (2) what blooming plant species are primarily associated with pesticide detections. Some papers (n = 17, Table 3) used a geo-spatial component in their analysis of HQ. Authors account for the variation in landcover in a foraging landscape by: (1) classifying a site on important characteristic/management technique (e.g., designate a location as “organic” or “conventional” as in Humann-Guilleminot et al., 2019), (2) determining relative composition of land-use categories surrounding apiaries. These are questions of management practices around an apiary and how different land cover classes or crops may contribute pesticides to detections in bee matrices or dilute pesticide detections in bee matrices.
Most studies that considered landscape composition found it was unclear how crop-specific pesticide use patterns were associated with HQ (Table 3). In some studies, HQ estimates were so high that pesticide use patterns from crop areas adjacent to apiaries could not be discerned. For example, Tosi et al. (2018) presents a detailed analysis of HQ detections in trapped pollen over 3 years; this study examined different HQ risk at organically and conventionally managed sites. The study demonstrated that pesticide contamination is widespread throughout Italy and that low-impact agricultural practices do not necessarily reduce pesticide risk to pollinators. Similarly, Humann-Guilleminot et al. (2019) found pesticide hazard to pollinators was high, even at organically managed sites and habitat set aside as unsprayed refuge. Drummond et al. (2018) used the percent of different land classes to contextualize HQ detections within foraging radii and found that HQ was significantly correlated with agricultural land cover. Urbanowicz et al. (2019) investigated the relationship between HQ detections and the prevalence of corn within a landscape. The authors addressed this with two different levels of temporal resolution. Maize is a wind pollinated crop, moderately attractive to honey bees, and is treated with neonicotinoids (United States Department of Agriculture [USDA], 2015; Urbanowicz et al., 2019). The authors found that neither percent maize within the foraging radius of the hive, nor percent maize pollen collected by bees in bee bread was significantly correlated with higher HQ detections.
In some instances, management practices have provided insight into how pesticide hazards are distributed through the landscape. Colwell et al. (2017) found that HQ was associated with site type (fallow, blueberry, cranberry, and apple sites), but that HQ was also associated with local floral diversity. Notably, the sites with the highest floral diversity had the lowest HQ values, and metrics were associated with fallow sites, suggesting that diversity of available forage may reduce pesticide hazard. However, in apple pollination systems, McArt et al. (2017) intensively sampled bee bread from thirty orchards to understand how pesticide risk accumulates in fresh bee bread. Over 60% of the pesticide hazard did not come from pesticides known to be used in apple orchards. This suggests that other cropping systems nearby may be disproportionately contributing to pesticide loads.
Taken in aggregate, however, these studies largely show poor correlation between HQ and specific crop pesticide use patterns. HQ is not reliably correlated with landscape designations around the apiary being monitored or the percentage of land surrounding the apiary (within a reasonable foraging distance) (Table 3). One explanation for this poor association is that it assumes bees forage uniformly across all habitat types, providing equal sampling of pesticide residues in the surrounding environment. Yet, dance language analysis has demonstrated that this is not the case (Couvillon et al., 2015; Samuelson et al., 2019). In dance analysis, bees are recorded performing waggle dances and the dance is decoded to determine where the bee is recruiting her sisters to forage within the landscape (Couvillon and Ratnieks, 2015). Bees prioritize resources close to the hive and foraging locations change with fluctuations in floral resources; bees will forage farther from the hive in times of floral dearth (Couvillon et al., 2014). In some cases, the change in floral resources result in shifts in foraging behavior which results in bees spending more time in crops with elevated pesticide use, like oilseed rape (Garbuzov et al., 2015), resulting in disproportionate exposure to pesticides relative to the aggregate in the landscape.
Hazard Quotient and Land Use Changes
In some situations, analysis of HQ from management of a single field is inadequate and an understanding of an aggregate exposure pattern is needed on a landscape level. In this respect, HQ has been used to understand both validate thresholds and to understand changes in pesticide use patterns over time. HQ calculations from the application rate have also been used to validate current thresholds for regulatory decision making around potential honey bee poisoning events (Mineau et al., 2008; Thompson and Thorbahn, 2010). In these studies, HQ thresholds appear validated; that is, thresholds were exceeded during poisoning events. This indicates that poisoning events are not occurring below relevant thresholds.
Perry and Moschini (2020) used HQ at the emissions point to understand how pesticide risk to bees (and other organisms) changed over time in corn cropping systems. During their study period, 1998 to 2014, authors found that while more pesticide treatments were being applied, the HQ risk to bees from these applications remained relatively consistent while risk to fish, mammals, and birds decreased. Notably, Perry and Moschini (2020) does not consider the attractiveness of corn to honey bees, nor the timing of the application of the pesticide. However, such conclusions are at odds with other studies using of HQ to infer historical trends. Two other papers have examined how hazard calculations may underestimate risk during a similar time period using an estimation of toxicity, Acute Insecticide Toxic Load (AITL), DiBartolomeis et al. (2019) factored in the environmental half-life of insecticides and found a 4 and 48-fold increase in acute insecticide toxic load for contact and oral toxicity due to the use of neonicotinoids in agriculture in the United States. Douglas et al. (2020) found a 9-fold increase in oral toxicity to bees with some regions showing a 121-fold increase in toxicity insecticide load driven by the use of seed treatments in corn and soy. Both HQ and AITL do not account for the actual exposure dynamics of honey bees foraging on contaminated crops or contacting residues lingering in soil and plants. Actual exposure is the result of the combination of foraging dynamics and pesticide applications to bee attractive crops (Sponsler et al., 2019), and simply estimating the hazards within the environment through either HQ or other metrics does not capture this process.
Temporal Dimensions of Hazard Quotient
When pesticide hazard is estimated using HQ at the hive, it reflects the potential dietary exposure of bees within a specific environment. Exposure at the hive has detected banned or misused pesticides in bee products (Ruiz-Toledo et al., 2018; Woodcock et al., 2018). In some cases, studies report the detection of illegal pesticide use (Woodcock et al., 2017; Tosi et al., 2018). HQ at the hive uses honey bee colonies as ecological sensors which collect and aggregate information from a landscape and report it to scientists (Richardson et al., 2015). These detections are snapshots of exposure at a given time, and sampling at different times of year can produce variation in pesticide residues (Böhme et al., 2018). This specificity in time-bound measurements can demonstrate where pesticide exposures may be taking place. For example, Böhme et al. (2018) sampled pollen every day and stratified within samples to determine the relative pesticide contributions of specific taxa to the HQ value of the sample. This methodology was able to identify that the sub-fraction of grapevine pollen (Vitis vinifera L.) was disproportionately contributing pesticide residues to the composite sample. Similarly, Stoner et al. (2019) found that Spiraea spp. L. pollen had high concentrations of pesticide relative to the other pollen in their samples. Favaro et al. (2019) examined changes in HQ before and after apple bloom; however, the high variability in HQ resulted in no association between HQ values and timing of trapping. Favaro et al. (2019) also divided pollen into colors and found no associations between HQ and pollen color, which they attributed to the potential contamination of pollen before color sorting occurred or that pesticide contamination was high in both apple orchards and the surrounding environment.
When pesticide hazard is calculated using HQ from the field application rate, estimations are based on application rate of pesticide for a given crop type. Because LD50 values and land use information are readily available through public agencies (Douglas et al., 2020), this method of HQ could allow models of past or future pesticide use patterns to understand pesticide hazard. As in Chen et al. (2017), this also allows the potential to make recommendations to land managers interested in reducing pesticide exposure to bees by identifying hazard-risk scenarios and taking mitigating action. This method assumes that bees will contact the full application rate and is potentially useful as a worst-case-scenario estimation of hazard. The difficulty of connecting mitigating measures at a field-level (i.e., avoiding sprays to bee attractive crops or spraying at night when bees are not foraging) to hazard calculations from the application rate, as foraging behavior is not accounted for (Sponsler et al., 2019).
Hazard Quotient and Hive Health Endpoints
A key limitation of HQ estimates is that they attempt to evaluate the likelihood of negative impacts to colony health based on two strongly mediated points of data; laboratory acute and chronic toxicity tests or field application rates. In contrast, risk assessment integrates both types of data into a framework that links exposure and toxicity. This poses considerable challenges. Five studies included in this review link colony health outcomes to HQ values: Traynor et al. (2016) in the eastern United States, Traynor et al. (2021a) in the United States, Lee et al. (2019) in the United States, Smart et al. (2016) in the northern great plains, and El Agrebi et al. (2019) in Belgium. Of these five, only one study found clear association of colony health with HQ and two found a weak association.
Traynor et al. (2016) examined how colony death and queen events were related to HQ detections by collecting matrices from commercial colonies providing migratory pollination services. Colony health and colony loss were associated with a higher number of generally relevant HQ detections (HQ > 50) and HQ values with large contributions from fungicides. These associations were stronger than actual HQ values; the number of pesticides detected within a sample was a stronger predictor of colony death than the total HQ additive value. In a study tracking HQ in bee bread over 7 years and across the entire United States, Traynor et al. (2021a) found no statistically significant associations when tracking how HQ changed over time.
HQ can only be considered additively; HQ cannot be used to understand synergistic pesticide hazard without modification. Although multiple papers assessing risk with HQ note this (e.g., Colwell et al., 2017; Stoner et al., 2019), few adjust HQ. Adjustments may be unnecessary as Belden and Brain (2018) has suggested that testing of tank mixtures of multiple chemicals is not warranted; instead suggesting a focus on the chemical that dominates toxicity. Conversely, Sanchez-Bayo and Goka (2014) suggest addressing these underestimations of risk by including a synergistic factor in the estimation of the LD50 of pesticide mixtures. However, determining synergistic factors is time-intensive and must be computed for each combination of chemicals (Sanchez-Bayo and Goka, 2014).
Fungicide and insecticide synergies may be one area where HQ chronically underestimates risk, warranting further exploration of how this has been demonstrated in HQ literature. The use of insecticides and fungicides in almond pollination systems is wide-spread; from 2007 to 2015, acres of almond crop treated with insecticide and fungicide has increased (Wade et al., 2019). Fungicides are generally considered low-toxicity for contact to pollinators and have high LD50 values, indicating that bees can be exposed to comparatively large doses of fungicide with little acute toxic effects (Ladurner et al., 2004).
Fungicide and insecticide synergism, while documented at field-realistic exposure levels (Wade et al., 2019), is still concentration dependent and cannot be assumed to occur based on the presence of two pesticides in a sample or a system. In both larval and adult toxicity tests, combinations of fungicide and insecticides increased acute bee mortality compared to controls (Iverson et al., 2019; Wade et al., 2019). Field trials which exposed bees to combinations of insecticides and fungicides have shown negative effects on both larvae and adults, indicating a likelihood that at high concentrations these chemistries could impact colony population size and adult foraging force (Fisher et al., 2021). Even in isolation, fungicide exposure is associated with brood loss, queen events, and reduced hypopharyngeal gland size (Traynor et al., 2021b). These interactions, which are known to increase mortality in bees, would not be captured in a HQ value, as HQ is only capable of capturing additive effects.
Despite its difficulty, understanding synergy in pesticide risk is a critical missing piece of understanding realistic pesticide risk to pollinators. Several classes of insecticides (carbamate, organophosphates, and pyrethroids) and azole fungicides are known to be over represented in synergistic interactions in pesticide mixtures (Cedergreen, 2014); all of these are commonly detected in bee matrices. The mechanism behind this synergy is rooted in the potential for triazole fungicides to inhibit detoxifying enzymes of the honey bee, increasing toxicity of insecticides when these pesticides co-occur (Haas and Nauen, 2021; Haas et al., 2022). Within the studies covered in this review, several pesticide combinations known to synergize co-occurred within a system or sample; although it is beyond the scope of this review to address every instance, these examples demonstrate how common it is to underestimate pesticide risk when relying on HQ alone. Colwell et al. (2017) report that two combinations of pesticides: chlorothalonil and coumaphos, chlorothalonil and fluvalinate, are known to have synergistic effects and at least one of these combinations occur at every site the authors sampled. Frazier et al. (2015) determined that pumpkin pollination systems contain the highest or second highest concentrations of chlorothalonil, coumaphos, and fluvalinate-tau. Despite this, pumpkin had a moderate total HQ compared to other systems but exhibited a steep drop-off in adult bee foragers, indicating colony-level impacts from pesticide stress. This provides evidence of a mismatch between HQ values within the system (low) and potential stress on the honey bee colony due to a decrease in foraging force. As noted in Sponsler and Johnson (2017), less foraging bees can reduce the potential exposure of the colony to pesticides, through reduced incoming contaminated pollen and nectar. Traynor et al. (2016) also noted that increased chlorothalonil HQ values in bee bread were specifically associated with colony death, while HQ detections in general were not. It is possible that the increased colony death associated with this detection may be due to the likelihood of chlorothalonil to synergize with other pesticides. Traynor et al. (2021b) found that fungicide residues present in bee bread were significantly associated with disease (Nosema infection and brood disease) and queen issues. These co-occurrences, while interesting, do not directly indicate synergism is occurring, however they do point to the potential for synergistic toxicity to occur if each pesticide is present in a high enough concentration.
Lee et al. (2019) analyzed the relationship between complete and unbroken brood pattern and patchy brood pattern and found that HQ was not correlated with brood pattern. However, the number of pesticides detected was significantly correlated with brood pattern in at least 1 year. Notably, Lee et al. (2019) found much lower HQ values in wax throughout the study compared to Traynor et al. (2016) which may explain the lack of connection with brood pattern.
The remaining two studies which examined colony health parameters did not find any significant correlation with HQ detections. Smart et al. (2016) examined the percent loss of colonies in six apiaries over 3 years. A strong relationship was found between percent uncultivated forage land and apiary survival; pollen quantity was also found to influence apiary survival more than pollen diversity and did not appear to be related to HQ values at the same sites. This suggests that the forage quantity (and to a lesser degree, quality) had a larger impact on colony survival than HQ detections. Similarly, El Agrebi et al. (2019) did not find any link between HQ detections of flumethrin and apiaries where colony losses exceeded 10%. As this study examined only one pesticide at a county-wide scale, it is possible that other pesticide detections or management practices had stronger impacts on colony health than HQ of a single pesticide.
Other factors beyond HQ values may have a direct impact on the success of the colony, confounding the relationship between HQ detections and hive health. For example, mite levels of Varroa destructor and viruses associated with this parasite are well documented to have impacts on honey bee colony health and have been consistently identified as one of the major drivers of annual colony losses. Traynor et al. (2021a) noted that higher HQ scores were associated with both the extreme high and extreme low ends of Varroa levels; that is, HQ values were highest in colonies with very little mite presence or 10 + mites per 100 bees. The authors interpret this as evidence that either Varroa are more fit in environments of high pesticide residues or the adult bee population has been reduced by contamination of the pollen (Traynor et al., 2021a).
Synthesis
Calculating HQ is a growing practice among researchers and it is used to make inferences on the risk of specific pesticides to honey bees. We found that HQ is currently being calculated from two points along the path of a pesticide from application to bee, from the amount of pesticide accumulating in bee matrices and dead bees or from the rate of the pesticide applied to a crop. Thresholds are then used to move discussions of hazard into the terminology of risk. Yet, thresholds in HQ calculations are inconsistent across studies and HQ is not consistently associated with hive health measurements.
One concern which has been presented throughout this review is the lack of a full, mechanistic model for understanding pesticide exposure both as it relates to foraging dynamics and pesticide emissions (Sponsler and Johnson, 2017; Sponsler et al., 2019) and transfer of pesticides within the social structure of the hive itself (Sponsler and Johnson, 2017). This is perhaps illuminated when comparing honey bee ecotoxicology with another area—aquatic toxicology. For example, in aquatic toxicology, mechanistic models exist to predict impacts of pesticide applications to organism by integrating key factors associated with the application such as landscape composition, weather, and other abiotic factors (Janney and Jenkins, 2022). These models can, and have, been validated with continuous water sampling, even though “grab” sample detections do not accurately represent the system (Janney and Jenkins, 2022).
Models like these and continuous sampling can be used to answer central questions of risk assessment: for a given application of pesticide, at a given rate, on a particular crop—is this pesticide safe? HQ is not capable of answering that question for several reasons. Most importantly, as mentioned above, we lack an understanding of the dynamic mechanism by which a pesticide makes its way from a pesticide sprayer to a colony. This limitation expands on Thompson (2021) who observed that HQ calculations from contaminated pollen fail to take advantage of known about the consumption rates of developing larvae. Our observation goes further to point out that the process by which a larva becomes exposed to contaminated pollen is but one segment of the larger pathway by which a bee becomes exposed. HQ has considerable difficulty connecting field applied pesticide rates to residues found in colonies, providing descriptive, rather than predictive power. Second, HQ remains rooted in acute, individual bee toxicity rather than chronic hive toxicity, which is a problem when thresholds set in the literature assume accumulation of toxic load over days to weeks. HQ is based off of contact or oral LD50 values, however, actual exposure mechanisms are more complex than these toxicity metrics would imply. It is for these reasons that we believe that HQ is not reliably linked to hive health outcomes in short (one season) or long (years) time frames. It is often difficult to use HQ to understand or predict colony health outcomes; in some ways, this is to be expected as HQ is a tier-one assessment tool; however, in its role as a monitoring or observational tool, there are not clear connections between HQ and hive-level health metrics. Risk estimation would benefit greatly from a mechanistic model that could use lab assessments to predict risk in the field. Tools regarding these models may be emerging in the form of predictive, mechanistic models that demonstrate increased likelihood of synergy between compounds (Haas and Nauen, 2021; Haas et al., 2022). However, HQ has been used to link pesticide detections in bee tissues with negative outcomes for colony-level health. Moreover, the disconnection between specific pesticide uses means HQ provides little insights into how a pesticide use could be mitigated to reduce risk (e.g., by changing the application rate or formulation or timing of treatment).
While many of the studies we reviewed use HQ to predict the risk of pesticides to bees, they do so in a way that diverges from how regulatory agencies assess risk, which relies on predicting the quantity of pesticide likely to be collected by and consumed by bees. Where the goal of hazard estimation is to understand dietary risk through consumption of contaminated nectar and pollen, RQ calculated using BeeREX may be the most appropriate model (Thompson, 2021). However, while Thompson (2021) identified the need to incorporate consumptive models of exposure, there are additional issues with HQ calculations identified in this review. As HQ is currently used in the literature, it is difficult to connect hazards to specific pesticide use practices. It is assumed, for example, when HQ is calculated from an application rate that all the pesticide reaches a foraging bee. In contrast, while HQ calculated from hive matrices can aggregate pesticide hazard, it has proven unable to trace these hazards back to specific pesticide uses. What becomes clear from this review is that the use of HQ misses a centerpiece of pesticide eco-toxicology, between point of emission and pesticide accumulation in the hive—field level exposure. Given this limitation, HQ methodologies have proven inadequate to addressing key questions around mitigating hazards, most prominently how hazards might be reduced using modified pesticide use practices (e.g., restricting sprays to the evening, spraying at lower rates, using precision spray technology).
This further highlights a difficulty within HQ literature—the difference between regulatory risk and the consequences of pesticide exposure for an individual hive. In linking HQ to hive health outcomes, researchers may be able to connect health impacts to relevant levels of pesticide in a hive (Traynor et al., 2016). However, this does not illustrate how pesticide use patterns could change to reduce those negative impacts, and therefore reduce risk. It is interesting and compelling to understand that certain levels of pesticide within a hive are associated with queen events or hive death. However, this cannot provide information on how bees are exposed (on what crop, at what time, under what use practices). Therefore, there is considerable need to understand the limitations of using HQ to predict the true risk of specific pesticide use to honey bees. Finally, our review points in the direction of the need to address the missing element in HQ studies, namely a more mechanistic and empirically grounded model of how bees are exposed to specific pesticide under field conditions.
Author Contributions
EC, AM, and RS contributed to conception and design of the review. EC organized the review process and wrote the first draft of the manuscript. AM and RS provided feedback and edited the manuscript. All authors contributed to manuscript revision, read, and approved the submitted version.
Funding
This work was supported by the National Science Foundation Graduate Research Fellowship and the Foundation for Food and Agricultural Research (Grant no. 549024).
Conflict of Interest
The authors declare that the research was conducted in the absence of any commercial or financial relationships that could be construed as a potential conflict of interest.
Publisher’s Note
All claims expressed in this article are solely those of the authors and do not necessarily represent those of their affiliated organizations, or those of the publisher, the editors and the reviewers. Any product that may be evaluated in this article, or claim that may be made by its manufacturer, is not guaranteed or endorsed by the publisher.
Acknowledgments
We would like to acknowledge the assistance of the Honey Bee Lab and Pollinator Health Lab members who provided feedback and support on the drafts.
References
Abdu-Allah, G. A. M., and Pittendrigh, B. R. (2018). Lethal and sub-lethal effects of select macrocyclic lactones insecticides on forager worker honey bees under laboratory experimental conditions. Ecotoxicology 27, 81–88. doi: 10.1007/s10646-017-1872-6
Alkassab, A. T., Thorbahn, D., Frommberger, M., Bischoff, G., and Pistorius, J. (2020). Effect of contamination and adulteration of wax foundations on the brood development of honeybees. Apidologie 51, 642–651. doi: 10.1007/s13592-020-00749-2
Barmaz, S., Potts, S. G., and Vighi, M. (2010). A novel method for assessing risks to pollinators from plant protection products using honeybees as a model species. Ecotoxicology 19, 1347–1359. doi: 10.1007/s10646-010-0521-0
Belden, J. B., and Brain, R. A. (2018). Incorporating the joint toxicity of co-applied pesticides into the ecological risk assessment process. Integr. Environ. Assess. Manag. 14, 79–91. doi: 10.1002/ieam.1957
Böhme, F., Bischoff, G., Zebitz, C. P. W., Rosenkranz, P., and Wallner, K. (2018). Pesticide residue survey of pollen loads collected by honeybees (Apis mellifera) in daily intervals at three agricultural sites in South Germany. PLoS One 13:e0199995. doi: 10.1371/journal.pone.0199995
Calatayud-Vernich, P., Calatayud, F., Simó, E., Pascual Aguilar, J. A., and Picó, Y. (2019). A two-year monitoring of pesticide hazard in-hive: high honey bee mortality rates during insecticide poisoning episodes in apiaries located near agricultural settings. Chemosphere 232, 471–480. doi: 10.1016/j.chemosphere.2019.05.170
Calatayud-Vernich, P., Calatayud, F., Simó, E., and Picó, Y. (2017). Occurrence of pesticide residues in Spanish beeswax. Sci. Total Environ. 605–606, 745–754. doi: 10.1016/j.scitotenv.2017.06.174
Calatayud-Vernich, P., Calatayud, F., Simó, E., and Picó, Y. (2018). Pesticide residues in honey bees, pollen and beeswax: assessing beehive exposure. Environ. Pollut. 241, 106–114. doi: 10.1016/j.envpol.2018.05.062
Cedergreen, N. (2014). Quantifying synergy: a systematic review of mixture toxicity studies within environmental toxicology. PLoS One 9:e96580. doi: 10.1371/journal.pone.0096580
Chen, X. D., Gill, T. A., Pelz-Stelinski, K. S., and Stelinski, L. L. (2017). Risk assessment of various insecticides used for management of Asian citrus psyllid, Diaphorina citri in Florida citrus, against honey bee, Apis mellifera. Ecotoxicology 26, 351–359. doi: 10.1007/s10646-017-1768-5
Colwell, M. J., Williams, G. R., Evans, R. C., and Shutler, D. (2017). Honey bee-collected pollen in agro-ecosystems reveals diet diversity, diet quality, and pesticide exposure. Ecol. Evol. 7, 7243–7253. doi: 10.1002/ece3.3178
Couvillon, M. J., and Ratnieks, F. L. W. (2015). Environmental consultancy: dancing bee bioindicators to evaluate landscape “health.” Front. Ecol. Evol. 3:44. doi: 10.3389/fevo.2015.00044
Couvillon, M. J., Riddell Pearce, F. C., Accleton, C., Fensome, K. A., Quah, S. K. L., Taylor, E. L., et al. (2015). Honey bee foraging distance depends on month and forage type. Apidologie 46, 61–70. doi: 10.1007/s13592-014-0302-5
Couvillon, M. J., Schürch, R., and Ratnieks, F. L. W. (2014). Waggle dance distances as integrative indicators of seasonal foraging challenges. PLoS One 9:e93495. doi: 10.1371/journal.pone.0093495
DiBartolomeis, M., Kegley, S., Mineau, P., Radford, R., and Klein, K. (2019). An assessment of acute insecticide toxicity loading (AITL) of chemical pesticides used on agricultural land in the United States. PLoS One 14:e0220029. doi: 10.1371/journal.pone.0220029
Douglas, M. R., Sponsler, D. B., Lonsdorf, E. V., and Grozinger, C. M. (2020). County-level analysis reveals a rapidly shifting landscape of insecticide hazard to honey bees (Apis mellifera) on US farmland. Sci. Rep. 10:797. doi: 10.1038/s41598-019-57225-w
Drummond, F. A., Ballman, E. S., Eitzer, B. D., Du Clos, B., and Du, Dill, J. (2018). Exposure of honey bee (Apis mellifera L.) colonies to pesticides in pollen, a statewide assessment in Maine. Environ. Entomol. 47, 378–387. doi: 10.1093/ee/nvy023
Eberhardt, L. L., and Thomas, J. M. (1991). Designing environmental field studies. Ecol. Monogr. 61, 53–73.
El Agrebi, N., Tosi, S., Wilmart, O., Scippo, M. L., de Graaf, D. C., and Saegerman, C. (2020b). Honeybee and consumer’s exposure and risk characterisation to glyphosate-based herbicide (GBH) and its degradation product (AMPA): residues in beebread, wax, and honey. Sci. Total Environ. 704:135312. doi: 10.1016/j.scitotenv.2019.135312
El Agrebi, N., Traynor, K., Wilmart, O., Tosi, S., Leinartz, L., Danneels, E., et al. (2020a). Pesticide and veterinary drug residues in Belgian beeswax: occurrence, toxicity, and risk to honey bees. Sci. Total Environ. 745:141036. doi: 10.1016/j.scitotenv.2020.141036
El Agrebi, N., Wilmart, O., Urbain, B., Danneels, E. L., de Graaf, D. C., and Saegerman, C. (2019). Belgian case study on flumethrin residues in beeswax: possible impact on honeybee and prediction of the maximum daily intake for consumers. Sci. Total Environ. 687, 712–719. doi: 10.1016/j.scitotenv.2019.05.493
EPA (2014). Guidance for Assessing Pesticide Risks to Bees, Vol. 59. (Washington, DC: Environmental Protection Agency, 20460).
European and Mediterranean Plant Protection Organization [EPPO] (2010). Environmental risk assessment scheme for plant protection products Chapter 10: honeybees. EPPO Bull. 40, 323–331. doi: 10.1111/j.1365-2338.2010.02419.x
Favaro, R., Bauer, L. M., Rossi, M., D’Ambrosio, L., Bucher, E., and Angeli, S. (2019). Botanical origin of pesticide residues in pollen loads collected by honeybees during and after apple bloom. Front. Physiol. 10:1069. doi: 10.3389/fphys.2019.01069
Fisher, A., DeGrandi-Hoffman, G., Smith, B. H., Ozturk, C., Kaftanoglu, O., Fewell, J. H., et al. (2021). Field cross-fostering and in vitro rearing demonstrate negative effects of both larval and adult exposure to a widely used fungicide in honey bees (Apis mellifera). Ecotoxicol. Environ. Saf. 217:112251. doi: 10.1016/j.ecoenv.2021.112251
Frazier, M. T., Mullin, C. A., Frazier, J. L., Ashcraft, S. A., Leslie, T. W., Mussen, E. C., et al. (2015). Assessing honey bee (Hymenoptera: Apidae) foraging populations and the potential impact of pesticides on Eight U.S. Crops. J. Econ. Entomol. 108, 2141–2152. doi: 10.1093/jee/tov195
Friedle, C., Wallner, K., Rosenkranz, P., Martens, D., and Vetter, W. (2021). Pesticide residues in daily bee pollen samples (April–July) from an intensive agricultural region in Southern Germany. Environ. Sci. Pollut. Res. 28, 22789–22803. doi: 10.1007/s11356-020-12318-2
Garbuzov, M., Couvillon, M. J., Schürch, R., and Ratnieks, F. L. W. (2015). Honey bee dance decoding and pollen-load analysis show limited foraging on spring-flowering oilseed rape, a potential source of neonicotinoid contamination. Agric. Ecosyst. Environ. 203, 62–68. doi: 10.1016/j.agee.2014.12.009
Haas, J., Glaubitz, J., Koenig, U., and Nauen, R. (2022). A mechanism-based approach unveils metabolic routes potentially mediating chlorantraniliprole synergism in honey bees, Apis mellifera L., by azole fungicides. Pest Manag. Sci. 78, 965–973. doi: 10.1002/ps.6706
Haas, J., and Nauen, R. (2021). Pesticide risk assessment at the molecular level using honey bee cytochrome P450 enzymes: a complementary approach. Environ. Int. 147:106372. doi: 10.1016/j.envint.2020.106372
Hrynko, I., Łozowicka, B., and Kaczyński, P. (2019). Comprehensive analysis of insecticides in melliferous weeds and agricultural crops using a modified QuEChERS/LC-MS/MS protocol and of their potential risk to honey bees (Apis mellifera L.). Sci. Total Environ. 657, 16–27. doi: 10.1016/j.scitotenv.2018.11.470
Humann-Guilleminot, S., Binkowski, ŁJ., Jenni, L., Hilke, G., Glauser, G., and Helfenstein, F. (2019). A nation-wide survey of neonicotinoid insecticides in agricultural land with implications for agri-environment schemes. J. Appl. Ecol. 56, 1502–1514. doi: 10.1111/1365-2664.13392
Iverson, A., Hale, C., Richardson, L., Miller, O., and McArt, S. (2019). Synergistic effects of three sterol biosynthesis inhibiting fungicides on the toxicity of a pyrethroid and neonicotinoid insecticide to bumble bees. Apidologie 50, 733–744. doi: 10.1007/s13592-019-00681-0
Janney, P., and Jenkins, J. (2022). Passive sampling and ecohydrologic modeling to investigate pesticide surface water loading in the Zollner Creek watershed, Oregon, USA. Sci. Total Environ. 819:152955. doi: 10.1016/j.scitotenv.2022.152955
Kasiotis, K. M., Tzouganaki, Z. D., and Machera, K. (2018). Chromatographic determination of monoterpenes and other acaricides in honeybees: prevalence and possible synergies. Sci. Total Environ. 625, 96–105. doi: 10.1016/j.scitotenv.2017.12.244
Kiljanek, T., Niewiadowska, A., Gaweł, M., Semeniuk, S., Borzęcka, M., Posyniak, A., et al. (2017). Multiple pesticide residues in live and poisoned honeybees – Preliminary exposure assessment. Chemosphere 175, 36–44. doi: 10.1016/j.chemosphere.2017.02.028
Ladurner, E., Bosch, J., Kemp, W. P., and Maini, S. (2004). Assessing delayed and acute toxicity of five formulated fungicides to Osmia lignaria Say and Apis mellifera. Apidologie 35, 3–13. doi: 10.1051/apido
Laurino, D., Proporato, M., Patetta, A., and Manino, A. (2011). Toxicity of neonicotinoid insecticides to honey bees: laboratory tests. Bull. Insectol. 64, 107–113.
Lee, K. V., Goblirsch, M., McDermott, E., Tarpy, D. R., and Spivak, M. (2019). Is the brood pattern within a honey bee colony a reliable indicator of queen quality? Insects 10, 1–17. doi: 10.3390/insects10010012
Magesh, V., Zhu, Z., Tang, T., Chen, S., Li, L., Wang, L., et al. (2017). Toxicity of neonicotinoids to honey bees and detoxification mechanism in honey bees. IOSR J. Environ. Sci. Toxicol. Food Technol. 11, 102–110. doi: 10.9790/2402-110401102110
McArt, S. H., Fersch, A. A., Milano, N. J., Truitt, L. L., and Böröczky, K. (2017). High pesticide risk to honey bees despite low focal crop pollen collection during pollination of a mass blooming crop. Sci. Rep. 7:46554. doi: 10.1038/srep46554
Mineau, P., Harding, K. M., Whiteside, M., Fletcher, M. R., Garthwaite, D., and Knopper, L. D. (2008). Using reports of bee mortality in the field to calibrate laboratory-derived pesticide risk indices. Environ. Entomol. 37, 546–554. doi: 10.1093/ee/37.2.546
Mullin, C. A., Frazier, M., Frazier, J. L., Ashcraft, S., Simonds, R., Vanengelsdorp, D., et al. (2010). High levels of miticides and agrochemicals in North American apiaries: implications for honey bee health. PLoS One 5:e9754. doi: 10.1371/journal.pone.0009754
Nai, Y. S., Chen, T. Y., Chen, Y. C., Chen, C. T., Chen, B. Y., and Chen, Y. W. (2017). Revealing pesticide residues under high pesticide stress in Taiwan’s agricultural environment probed by fresh honey bee (Hymenoptera: Apidae) Pollen. J. Econ. Entomol. 110, 1947–1958. doi: 10.1093/jee/tox195
Perry, E. D., and Moschini, G. C. (2020). Neonicotinoids in U.S. maize: insecticide substitution effects and environmental risk. J. Environ. Econ. Manage. 102:102320. doi: 10.1016/j.jeem.2020.102320
Pohorecka, K., Szczęsna, T., Witek, M., Miszczak, A., and Sikorski, P. (2017). The exposure of honey bees to pesticide residues in the hive environment with regard to winter colony losses. J. Apic. Sci. 61, 105–125. doi: 10.1515/jas-2017-0013
Richardson, R. T., Lin, C.-H., Sponsler, D. B., Quijia, J. O., Goodell, K., and Johnson, R. M. (2015). Application of ITS2 metabarcoding to determine the provenance of pollen collected by honey bees in an agroecosystem. Appl. Plant Sci. 3:1400066. doi: 10.3732/apps.1400066
Ruiz-Toledo, J., Vandame, R., Castro-Chan, R. A., Penilla-Navarro, R. P., Gómez, J., and Sánchez, D. (2018). Organochlorine pesticides in honey and pollen samples from managed colonies of the honey bee Apis mellifera linnaeus and the stingless bee scaptotrigona Mexicana guérin from southern, Mexico. Insects 9:54. doi: 10.3390/insects9020054
Samuelson, A. E., Schürch, R., and Leadbeater, E. (2019). Dancing bees evaluate agricultural forage resources as inferior to central urban land. bioRxiv [Preprint] doi: 10.1101/2019.12.19.882076
Sanchez-Bayo, F., and Goka, K. (2014). Pesticide residues and bees - a risk assessment. PLoS One 9:e94482. doi: 10.1371/journal.pone.0094482
Smart, M. D., Pettis, J. S., Euliss, N., and Spivak, M. S. (2016). Land use in the Northern great plains region of the U.S. influences the survival and productivity of honey bee colonies. Agric. Ecosyst. Environ. 230, 139–149. doi: 10.1016/j.agee.2016.05.030
Sponsler, D. B., Grozinger, C. M., Hitaj, C., Rundlöf, M., Botías, C., Code, A., et al. (2019). Pesticides and pollinators: a socioecological synthesis. Sci. Total Environ. 662, 1012–1027. doi: 10.1016/j.scitotenv.2019.01.016
Sponsler, D. B., and Johnson, R. M. (2017). Mechanistic modeling of pesticide exposure: the missing keystone of honey bee toxicology. Environ. Toxicol. Chem. 36, 871–881. doi: 10.1002/etc.3661
Stoner, K. A., Cowles, R. S., Nurse, A., and Eitzer, B. D. (2019). Tracking pesticide residues to a plant genus using palynology in pollen trapped from honey bees (Hymenoptera: Apidae) at ornamental plant nurseries. Environ. Entomol. 48, 351–362. doi: 10.1093/ee/nvz007
Stoner, K. A., and Eitzer, B. D. (2013). Using a hazard quotient to evaluate pesticide residues detected in pollen trapped from honey bees (Apis mellifera) in Connecticut. PLoS One 8:e77550. doi: 10.1371/journal.pone.0077550
Thompson, H. M. (2021). The use of the hazard quotient approach to assess the potential risk to honeybees (Apis mellifera) posed by pesticide residues detected in bee-relevant matrices is not appropriate. Pest Manag. Sci. 77, 3934–3941. doi: 10.1002/ps.6426
Thompson, H. M., and Thorbahn, D. (2010). Review of honeybee pesticide poisoning incidents in Europe – evaluation of the hazard quotient approach for risk assessment. Julius Kühn Arch. 103, 103–108. doi: 10.1002/ps.6426
Tomé, H. V. V., Schmehl, D. R., Wedde, A. E., Godoy, R. S. M., Ravaiano, S. V., Guedes, R. N. C., et al. (2020). Frequently encountered pesticides can cause multiple disorders in developing worker honey bees. Environ. Pollut. 256:113420. doi: 10.1016/j.envpol.2019.113420
Topitzhofer, E., Lucas, H., Chakrabarti, P., Breece, C., Bryant, V., Sagili, R. R., et al. (2019). Assessment of pollen diversity available to honey bees (Hymenoptera: Apidae) in major cropping systems during pollination in the western United States. J. Econ. Entomol. 112, 2040–2048. doi: 10.1093/jee/toz168
Tosi, S., Costa, C., Vesco, U., Quaglia, G., Guido, G., Simone, T., et al. (2018). A 3-year survey of Italian honey bee-collected pollen reveals widespread contamination by agricultural pesticides. Sci. Total Environ. 615, 208–218. doi: 10.1016/j.scitotenv.2017.09.226
Traynor, K. S., Pettis, J. S., Tarpy, D. R., Mullin, C. A., Frazier, J. L., Frazier, M., et al. (2016). In-hive pesticide exposome: assessing risks to migratory honey bees from in-hive pesticide contamination in the Eastern United States. Sci. Rep. 6:33207. doi: 10.1038/srep33207
Traynor, K. S., Tosi, S., Rennich, K., Steinhauer, N., Forsgren, E., Rose, R., et al. (2021a). Pesticides in honey bee colonies: establishing a baseline for real world exposure over seven years in the USA. Environ. Pollut. 279:116566. doi: 10.1016/j.envpol.2021.116566
Traynor, K. S., vanEngelsdorp, D., and Lamas, Z. S. (2021b). Social disruption: sublethal pesticides in pollen lead to Apis mellifera queen events and brood loss. Ecotoxicol. Environ. Saf. 214:112105. doi: 10.1016/j.ecoenv.2021.112105
United States Department of Agriculture [USDA] (2015). Attractiveness of Agricultural Crops to Pollinating Bees for the Collection of Nectar and/or Pollen. Washington, DC: USDA.
Urbanowicz, C., Baert, N., Bluher, S. E., Böröczky, K., Ramos, M., and McArt, S. H. (2019). Low maize pollen collection and low pesticide risk to honey bees in heterogeneous agricultural landscapes. Apidologie 2011, 379–390. doi: 10.1007/s13592-019-00655-2
US EPA (2012). White Paper in Support of the Proposed Risk Assessment Process for Bees, Vol. 275. Washington, DC: Office of Chemical Safety and Pollution Prevention.
Villa, S., Vighi, M., Finizio, A., and Serini, G. B. (2000). Risk assessment for honeybees from pesticide-exposed pollen. Ecotoxicology 9, 287–297.
Wade, A., Lin, C. H., Kurkul, C., Regan, E. R., and Johnson, R. M. (2019). Combined toxicity of insecticides and fungicides applied to California almond orchards to honey bee larvae and adults. Insects 10:20. doi: 10.3390/insects10010020
Woodcock, B. A., Bullock, J. M., Shore, R. F., Heard, M. S., Pereira, M. G., Redhead, J., et al. (2017). Country-specific effects of neonicotinoid pesticides on honey bees and wild bees. Science 356, 1393–1395. doi: 10.1126/science.aaa1190
Keywords: honey bee, hazard quotient, HQ, ecotoxicology, Apis mellifera, pollen hazard quotient
Citation: Carlson EA, Melathopoulos A and Sagili R (2022) The Value of Hazard Quotients in Honey Bee (Apis mellifera) Ecotoxicology: A Review. Front. Ecol. Evol. 10:824992. doi: 10.3389/fevo.2022.824992
Received: 29 November 2021; Accepted: 05 May 2022;
Published: 06 June 2022.
Edited by:
Christopher Williams, Liverpool John Moores University, United KingdomReviewed by:
Daniel Schmehl, Bayer CropScience, United StatesHelen Thompson, Syngenta Jealotts Hill, United Kingdom
Kimberly Ann Stoner, Connecticut Agricultural Experiment Station, United States
William G. Meikle, Agricultural Research Service (USDA), United States
Chuleui Jung, Andong National University, South Korea
Copyright © 2022 Carlson, Melathopoulos and Sagili. This is an open-access article distributed under the terms of the Creative Commons Attribution License (CC BY). The use, distribution or reproduction in other forums is permitted, provided the original author(s) and the copyright owner(s) are credited and that the original publication in this journal is cited, in accordance with accepted academic practice. No use, distribution or reproduction is permitted which does not comply with these terms.
*Correspondence: Emily A. Carlson, RW1pbHkuQ2FybHNvbkBvcmVnb25zdGF0ZS5lZHU=