- 1Plant Quarantine and Pesticide Management Centre, Lalitpur, Nepal
- 2Gulbali Institute, Charles Sturt University, Orange, NSW, Australia
- 3Quantitative Consulting Unit, Charles Sturt University, Wagga Wagga, NSW, Australia
The benefits of non-crop vegetation to conservation biological control of insect pests in adjacent crops have often been demonstrated. Other studies have established that pesticide use can negatively impact natural enemies; but little is known about the outcomes from providing non-crop vegetation in systems with pesticide use. Here we conducted a natural experiment, sampling arthropods from within a set of four fields with varying pesticide use intensities that were otherwise similar and had perennial native vegetation adjacent to a single edge. Bayesian network analysis was applied to model the entire data set, then sensitivity analysis of numbers of arthropods captured in pitfall traps and sticky traps revealed that the overall effect of pesticide toxicity was large. Numbers of multiple arthropod taxa were especially strongly reduced in fields with pesticide regimes that had greater calculated toxicity scores. The effects on natural enemy numbers of the presence of adjacent perennial native vegetation was weaker than the effect of pesticide regime for all taxa except for Staphilinidae, for which it was equivalent. The benefit to in-crop numbers of natural enemies from the adjacent vegetation was strongest for ground active Araneae, Formicidae, and Dermaptera. Descriptive statistical analysis of the spatial distribution in the least heavily sprayed field suggested that the native vegetation was donor habitat for in-crop natural enemies, especially Hymenoptera, Dermaptera, and Formicidae, with numbers elevated close to the native vegetation, an effect that was apparent for around 100 m. Conservation of invertebrates in agricultural landscapes, including efforts to promote natural enemies for conservation biological control, are strongly impeded by “real world” pesticide regimes that include frequent applications and toxic compounds. Landscape features such as perennial native woody vegetation are potentially important refuges for a wide range of natural enemy taxa. The donor habitat effect of such refuges can elevate in-crop densities of these important ecosystem service providers over a scale of around 100 m, implying scope to enhance the strength of biological control in large fields (around 4 ha) by use of entirely wooded margins provided pesticide use is moderated.
Introduction
Crop fields surrounded by non-crop vegetation such as hedgerows, shelterbelts, and riparian vegetation can benefit from pest biological control provided by enhanced numbers and diversity of natural enemies compared to fields with no such refuge habitat (Landis and Marino, 1999; Bianchi et al., 2006; Chaplin-Kramer et al., 2013; Morandin et al., 2014; Heimoana et al., 2017). Non-crop vegetation can protect and enhance natural enemies through the provision of appropriate microclimates and overwintering sites for shelter and food in the form of nectar, pollen or alternative hosts (Gurr et al., 2017; Shields et al., 2019; Gardarin et al., 2021). Perennial vegetation can provide a stable habitat for natural enemies from which to recolonize the disturbed habitat of crop fields (Asbjornsen et al., 2014; Schellhorn et al., 2014), supporting pest suppression (Perović et al., 2011; Macfadyen et al., 2015; Heimoana et al., 2017).
Non-crop vegetation does not, however, always increase natural enemy abundance or provide biological pest control service in adjacent crop fields (Tscharntke et al., 2016). There are several factors that potentially account for this, such as absence of effective natural enemies in the region, insufficient or inefficient source habitat, or disruptive agricultural practices (Tscharntke et al., 2016; Karp et al., 2018). Chemical pesticide use on crop fields in particular can interrupt the biocontrol service provided by natural enemies adjacent to non-crop vegetation (Jonsson et al., 2012; Zhu et al., 2017; Gagic et al., 2019). Increased agricultural pesticide use in cropping systems is hostile for natural enemies and low abundance of natural enemies results in ineffective biological control (Mansfield et al., 2006; Thomson and Hoffmann, 2006; Bommarco et al., 2011; Whitehouse et al., 2018). However, population recovery of natural enemies in crop fields is possible as a result of immigration from nearby non-crop vegetation (Duffield et al., 1996; Heimoana et al., 2017). Increasing diversity in cropping systems and reduced or selective insecticide use can enhance natural enemy populations, suppress crop pests and improve agricultural sustainability (Lu et al., 2012; Torres and Bueno, 2018; Lykogianni et al., 2021; Tscharntke et al., 2021). A review by Bommarco et al. (2013) identified that perennial vegetation can be incorporated into agricultural systems to provide a diverse habitat for natural enemies which can result in the suppression of pest populations, and reduced environmental impacts due to decreased use of chemical pesticides. Currently, the effect of interactions between non-crop vegetation and pesticide use on natural enemy activity in crop fields is poorly understood (Zhu et al., 2017; Thomine et al., 2022).
Agricultural landscapes often include portions of perennial non-crop vegetation, some of which is remnant natural vegetation and some intentionally established to manage soil erosion, salinity or other agri-environmental benefit. The inclusion of non-crop native vegetation in agricultural landscapes has been recommended for biodiversity conservation of native flora and fauna (Burghardt et al., 2009; Landis et al., 2012; Chrobock et al., 2013; Gill et al., 2014). However, few studies have explored the effects of perennial, woody vegetation in commercial agricultural fields on natural enemy populations (Thomson and Hoffmann, 2010, 2013; Morandin et al., 2014; Bianchi et al., 2015; Shields et al., 2016; Retallack et al., 2019), despite the benefits evident in laboratory and small field trial studies (Fiedler and Landis, 2007; Isaacs et al., 2009; Pandey et al., 2018; Pandey and Gurr, 2019).
This study used a natural experiment approach to assess natural enemy densities in a set of four fields that were growing a common crop (brassica vegetable), in one region and 1 year, each had one margin that was adjacent to woody vegetation, but that were being managed with differing levels of pesticide use intensity. This uncommon alignment of characteristics (we used all sites that in the region that met the foregoing criteria) provided an opportunity to assess the effects on natural enemies in a system in which pesticide toxicity load and presence of woody vegetation on one edge could be discriminated. We hypothesized that the benefit of adjacent vegetation on in-crop natural enemy densities would be strongest in fields with the lowest pesticide use intensity.
Materials and Methods
Study Sites
The study sites consisted of four commercial brassica fields in Central West of New South Wales, Australia (Figure 1). This represented all possible available fields that met criteria for them to test the central hypothesis. Accordingly, sites each had an area of woody, perennial, native vegetation along one border with the opposite side of the field comprised of an unsealed farm road and another brassica crop. All fields were 3–4 ha in extent to allow for measurement of potential gradients in arthropod abundance in transects from the wooded margins. All four sites were in a simple landscape dominated by other commercial, conventionally managed (i.e., non-organic) crop fields. Each field contained a summer-autumn grown brassica vegetable crop (Brassica oleracea var. botrytis) that was managed by the farmer with conventional pesticides. Perennial woody native vegetation at all four sites was a Eucalyptus spp.-dominated zone, with a groundcover of grass family Cynodon dactylon, Digitaria spp., Paspalum dilatatum, Microlaena stipoides, Aristida spp., Bothriochloa spp., and herbaceous species Rubus sp., Foeniculum vulgare, Conium maculatum, Datura stramonium, Portulaca oleracea, Verbena bonariensis, Chondrilla juncea, Sisymbrium officinale, and Chenopodium album.
Pesticide Use
All crops were managed by the host farmer and for each site a “cumulative pesticide toxicity score” was calculated to measure pesticide load covering the time from field preparation to the last date of data collection. Crops were sprayed in response to the insect pest Plutella xylostella, Pieris rapae, Hellula hydralis, other lepidopteran pests and aphids. Pesticides were applied by farmers based on visual observations of pest infestation levels with decisions guided by their experience and consultation with their agronomist without influence from the researchers. Data on pesticide identity, rate and application frequency were collected from the spray application records provided by the farmers. Toxicity scores were calculated by multiplying a modified toxicity rating given to each pesticide chemical by the number of applications which was summed to give a “cumulative pesticide toxicity score” by following Thomson and Hoffmann (2006) and Markó et al. (2017). This approach has been found to be reasonable comparison of pesticide loads in fields with same type of crop and similar soil properties (Ockleford et al., 2017). The toxicity rating of each chemical was based on the International Organization for Biological Control (IOBC) Pesticide Side Effect Database,1 which divides the acute toxicity of pesticides for non-target organisms into four risk categories from harmless (1) to harmful (4) (Table 1). This was modified by changing the scores to 0–3 to include only the influence of chemical pesticides that harm natural enemies (Thomson and Hoffmann, 2006). We also considered application rate [grams of active ingredient (a.i.)/ha] for toxicity rating of each pesticide. For example, methomyl 500 g/ha was given maximum score of 3, while methomyl 90 g/ha was rated 2. These a.i./ha of sites were compared with a.i./ha of the IOBC database and relevant peer-reviewed research papers that considered natural enemies-insecticide toxicity (Leggett, 1990; Elzen et al., 1998; Galvan et al., 2006; Wanumen et al., 2016; Deekshita et al., 2017). Sampling of natural enemies was always carried out after the relevant re-entry interval after each pesticide application.
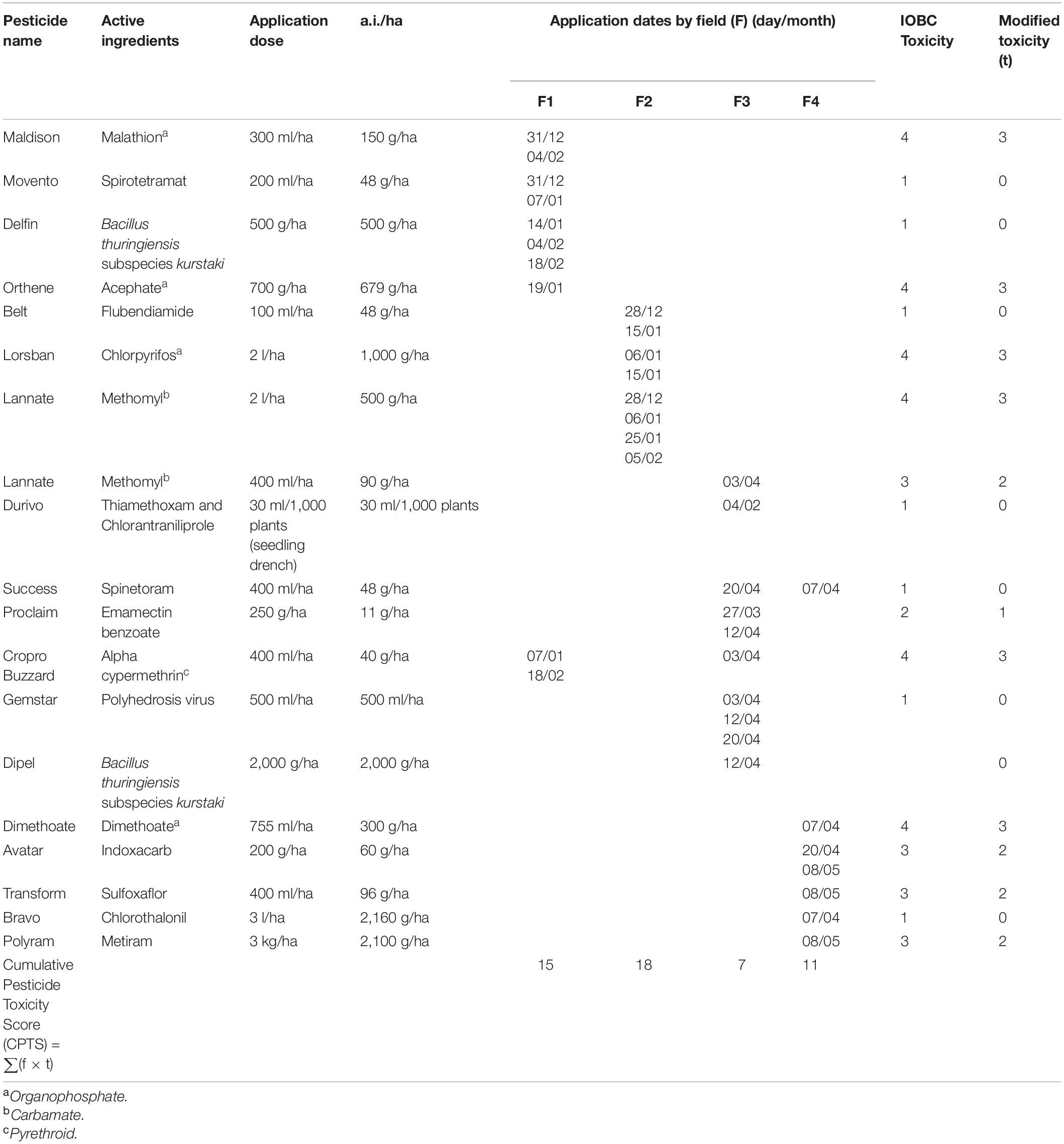
Table 1. Insecticides and fungicides applied during the growing season (Dec 2016–May 2017) and the toxicity as determined by IOBC and modified toxicity rating for each site (F1–F4).
Study Design
In each field, three parallel transects, 40 m apart, were established perpendicular from the woody vegetation toward the opposite edge of the field which was 140–180 m away depending on the field (Figure 2). For each transect there were 11 sampling points, which were positioned at 1, 5, 10, 20, and 50 m from each edge plus one point in the center of the field. The relative abundance of canopy and ground-active natural enemies was assessed at each sample point with a yellow sticky trap (17 × 10 cm) (Bugs for Bugs, Australia) mounted atop a 1,200 mm wooden stake and two round pitfall traps (12.5 cm deep and 8.5 cm diameter). Yellow sticky trapping is widely used for aerial arthropods such as parasitic Hymenoptera, reflecting efficacy, and low cost (Larsen et al., 2014; McCravy, 2018), whilst pitfall trapping is the most common sampling method for ground dwelling arthropods in cultivated land (McCravy, 2018). Pitfall traps were constructed using two cups, the outer cup was placed flush with the soil surface and inner cup was inserted into the first cup and filled with ∼250 ml of diluted ethylene glycol with a few drops of detergent. The traps were protected from rain and sun with a cover made from a plastic plate and wooden skewers. Traps were left in place for 4 days and then collected for quantification and identification of natural enemies. Natural enemy monitoring/sampling was carried out on four occasion’s commencing 30 days after crop planting-out and at approximately 15-day intervals taking into consideration the constraint of re-entry periods after pesticide applications. The last observation was made 2 weeks before crop harvest (March 2017 for field 1 and 2 and May 2017 for field 3 and 4).
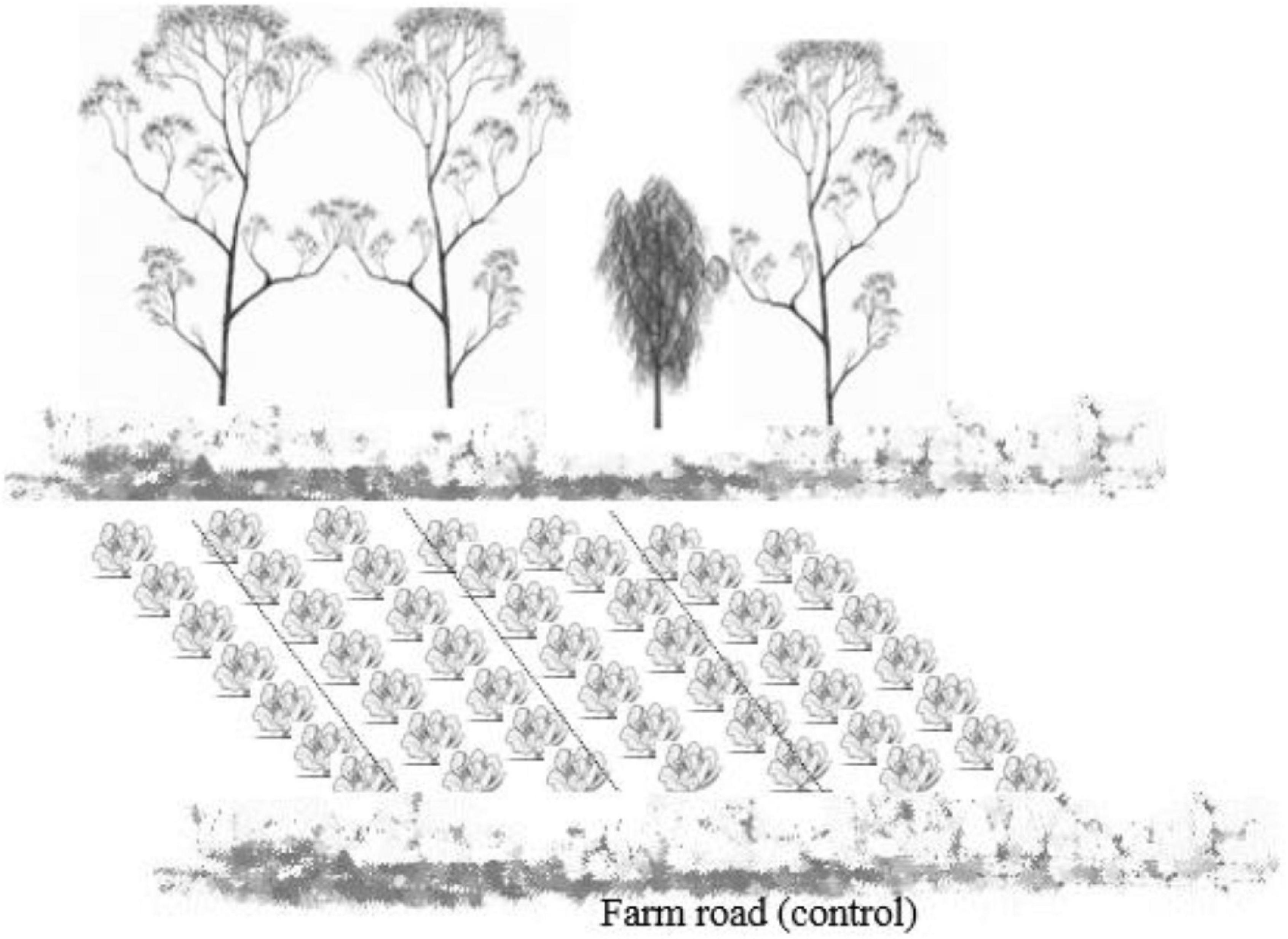
Figure 2. Schematic diagram of each field of study: The perennial native vegetation is considered as a “treatment” for attracting natural enemy insects for the benefit of growing brassica crops and farm road considered as control. The dotted line in the brassica field are transects were data collection was done in 11 distance points (1, 5, 10, 20, and 50 m from each edge and the field center).
Arthropods collected in pitfall traps at a given transect position were placed in a plastic container and labeled. Similarly, each yellow sticky trap was labeled and wrapped in plastic food wrap film. The arthropods from the pitfall traps (ground active predators) were cleaned, counted, identified to a family level and placed in ethyl alcohol filled glass vial for further inspection within 48 h from collection. Yellow sticky traps were initially placed in freezers and all natural enemies later identified to super family or genus level for some taxa. Parasitoids were identified by following (Goulet and Huber, 1993) using a stereo dissecting microscope (10–20 ×) (Leica, SE305-A, Mikrosysteme Vertrieb GmbH, Wetzlar, Germany). Arthropod counts were totaled across the corresponding positions (distance from edge) for the three transects in each field.
Data Analysis
A Bayesian Network (BN) approach was used for data analysis so that the nuances of the complex interrelationships between the variables could be captured. Originally developed as a modeling tool from artificial intelligence since late 1980s, BNs have found applications across the sciences, industries and government organizations (Pearl, 1988; Kjærulff and Madsen, 2008). As probabilistic graphical models, BNs allow for effective modeling of physical, biological, and social systems operating under uncertainty (Kjærulff and Madsen, 2008; Korb and Nicholson, 2011). Formally, a BN model is a graphical representation, i.e., a directed acyclic graph (DAG), of a joint probability distribution of a set of random variables in which each variable is represented by a node and the dependency relationship is represented by a link/edge for two associated variables (Pearl, 1988; Kjærulff and Madsen, 2008). BNs are based on Bayes Theorem, a mathematical statement which expresses the interrelationships between the conditional, marginal, and joint probability distributions of random variables as defined in the following formula (Upton and Cook, 2006):
where A and B are two random variables/events; Pr(A) and Pr(B) are the marginal probability distributions of A and B, respectively; Pr(B| A) is the conditional probability distribution of B given A; Pr(A| B) is the conditional probability distribution of A given B; and Pr(A, B) is the joint probability distribution of A and B.Since a BN model represents the joint distribution of all variables included in the model, anyone (or more than one) variable(s) may be selected as the target variable(s) (equivalent to the “outcome/dependent” variable in a regression model) to then perform inferential analysis by assuming different scenarios in terms of the “findings” of other variables. For example, by fixing the values of some variables (equivalent to those predictor variables in a regression model) we are able to estimate/predict the values (or the distribution of the values) of the remaining variable(s) (equivalent to the outcome/dependent variable in a regression model) in a BN model.
In the present study, the BN analysis investigated associations between field characteristics (pesticide toxicity score, distance from woody vegetation refuge, transect and sampling date) and number of arthropods caught of each taxonomic category or sampling method (pitfall trap or sticky trap) (Figure 3). The BN predictive model included the toxicity effect (reflecting the differing pesticide regimes among the four fields) and the refuge effect (representing the multiple sampling positions within each field at varying distances from the perennial native vegetation that bordered one edge and may have constituted a refuge for arthropods that later moved into the crop field). Other variables included the transect number and sample date as well as the natural enemy groups. By specifying different scenarios in terms of the toxicity effect and the refuge effect, this BN model could estimate/predict the abundance levels for each natural enemy groups. This BN model also allowed a sensitivity analysis to be run for each of the natural enemy groups to quantify the strength of the association between the abundance level and the toxicity effect and the refuge effect. Thus, the percentage values from such sensitivity analyses for a given variable are broadly analogous to the adjusted R2, the goodness-of-fit measure from a regression analysis that provide an indication of the explanatory strength of one variable for another. The proprietary BN software package, Netica (Norsys Software Corp, 2021), was used for model construction and sensitivity analyses.
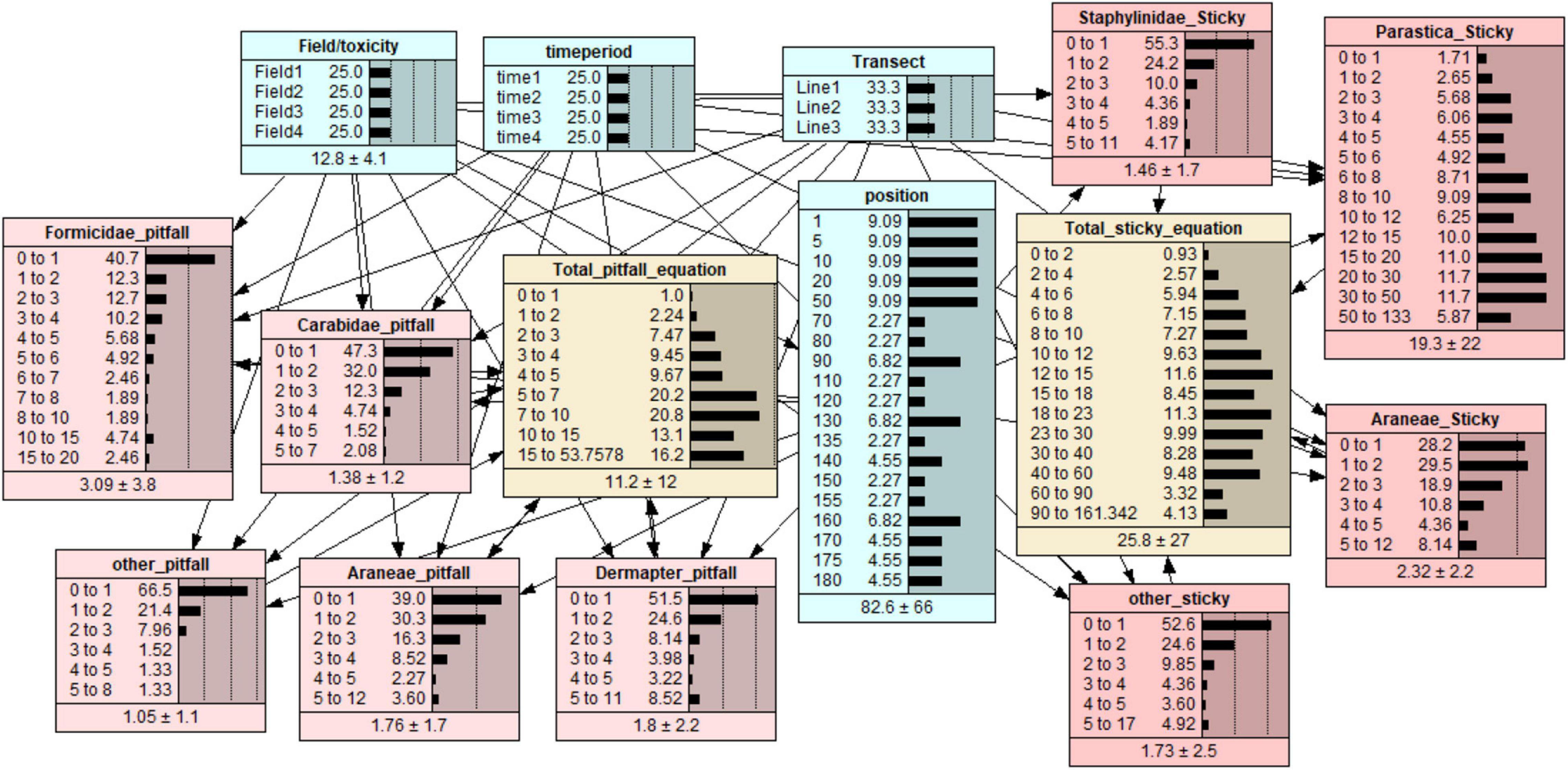
Figure 3. A predictive Bayesian Network model for the overall data set which represents the interrelationships between the pesticide toxicity scores calculated for four brassica vegetable fields, sample position effects resulting from perennial woody vegetation at one field edge, transect number (3 per field) and sample date on the abundance of various natural enemy groups sampled by pitfall and sticky traps.
Descriptive analyses were conducted in R (R Core Team, 2020) and were used to describe the overall spatial pattern of arthropod catches in relation to the fields with contrasting levels of calculated pesticide toxicity and spatial position within fields in relation to the adjacent perennial native vegetation.
Results
Natural Enemy Assemblages of Brassica Fields
A total of 14,459 natural enemies were caught; 11,123 on sticky traps and 3,336 in pitfall traps. Predators made up 34.7% (5,018) and hymenopteran parasitoids 65.3% (9,441). Predators were more numerous in pitfall traps (66.5%) compared to sticky traps (33.5%). All the parasitoids were caught on sticky traps. The most abundant predators comprised of Araneae (30.1%) (both canopy and ground), Formicidae (25.8%), Dermaptera (13.0%), Staphylinidae (12.8%), and Carabidae (9.3 %). Within Carabidae 95% of specimens were fully winged and small (<9 mm, length of the body excluding head). Less abundant taxa were Coccinellidae (1.41%), Syrphidae (0.5%), Dolichopodidae (0.33%), Melyridae (0.23%), Cantharidae (0.22%), Hemerobiidae (0.21%), Vespidae (0.09%), Nabidae (0.07%), and Cicindelinae (0.01%). Among total parasitoids, small-minute parasitoids comprised 97.3% followed by Diadegma spp. (1.62%), Aphidiinae (0.84%), Ichneumonoidea (0.12%), and Cotesia spp. (0.05%).
Effect of Pesticide Load and Perennial Native Vegetation on Natural Enemies
Descriptive data analysis for the overall numbers of arthropods captured in pitfall traps showed clear differences among the four fields with differing pesticide regimes. Numbers of these ground-active predators were uniformly low across the extent of transects in the field with highest toxicity score (Figure 4). Numbers in the field with the next most intense pesticide regime (toxicity score 15) were marginally higher but relatively uniform across the field. Arthropod numbers in the two least intensively sprayed fields were markedly higher (Table 2) and, in both cases, showed a pronounced elevation in proximity to the perennial native vegetation. This spatial trend was especially marked in field 3 which had the lowest toxicity score. Here, high predator densities were present in areas of the crop adjacent to perennial woody vegetation. Densities decayed over the range of 120 m from this margin, reaching levels consistent with the most heavily sprayed two fields.
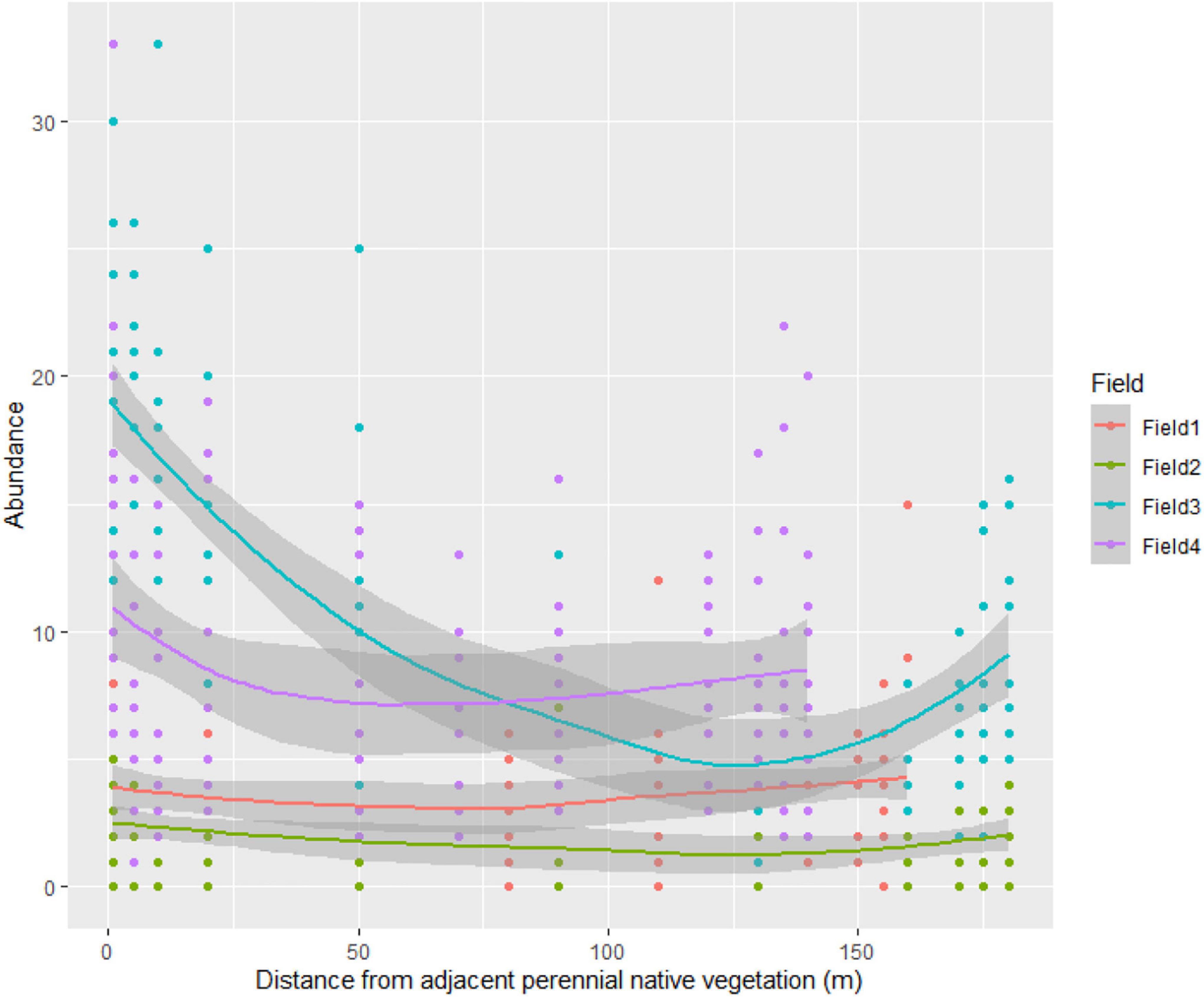
Figure 4. Spatial distribution of the ground active predators (pitfall trap catches) in fields with perennial woody vegetation adjacent to one margin (the zero position) but with contrasting levels of pesticide use quantified as a toxicity score (pale blue = low; purple = moderate; red = high; green = very high).
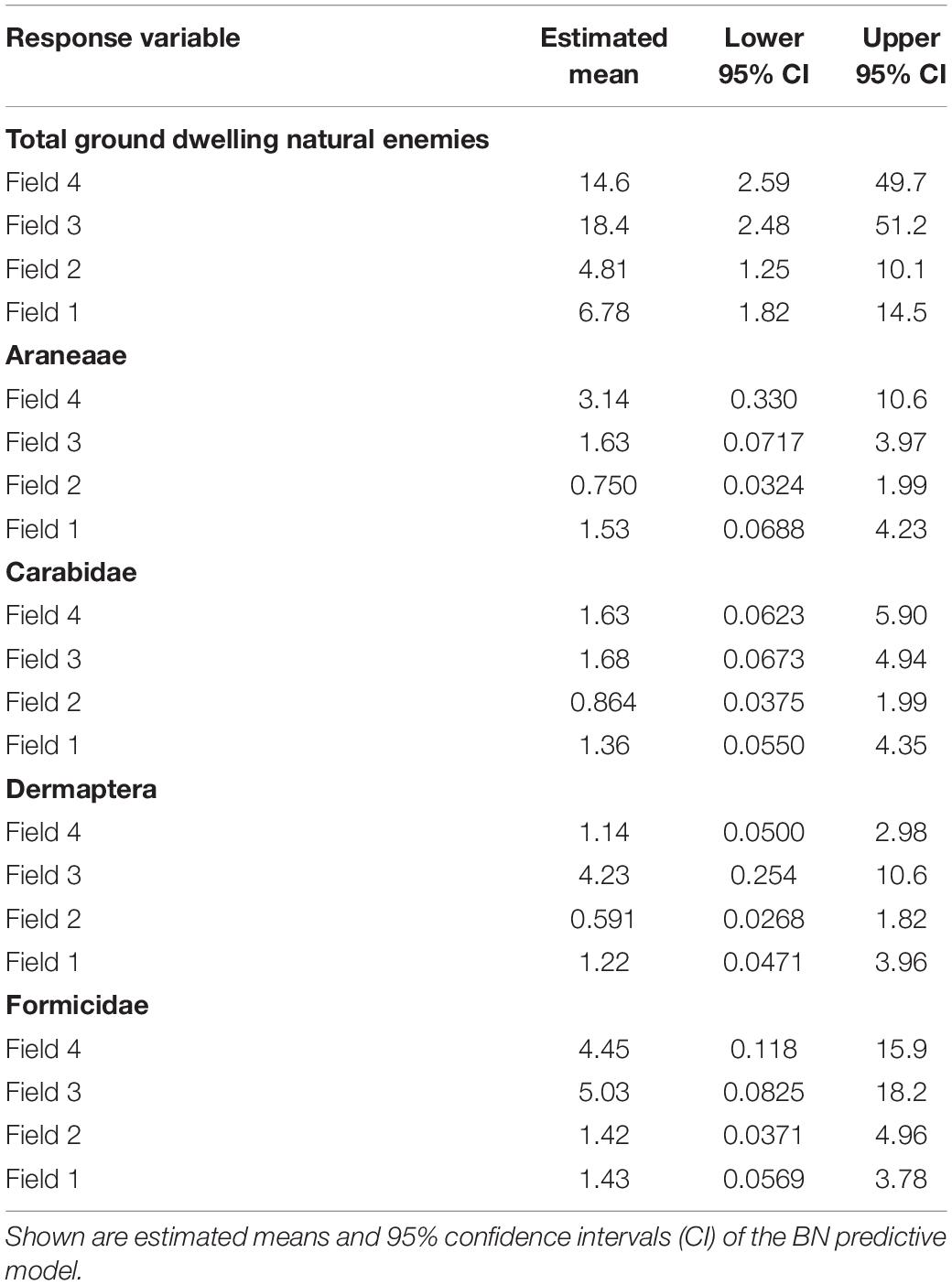
Table 2. Variables explaining the abundance of ground active natural enemies and their finer taxonomic group.
BN sensitivity analyses run for each of the natural enemy taxa and guilds allowed a quantitative comparison of the relative strength of the pesticide toxicity effect vs. the refuge effect. Overall, the effect of pesticide toxicity was dominant compared with the adjacent perennial native vegetation’s refuge effect. This was the case for all taxa and groups except for Staphilinidae, for which the two effects were equivalent (Table 3). Dermaptera, parasitoids, ground active Araneae and Formicidae were most strongly influenced by the toxicity effect and there was a strong effect for overall ground active arthropods. Less strong effects were evident for ground active Carabidae and aerial Aranae and Staphilinidae, all taxa within which some species are capable of flight or “ballooning” dispersal on silken threads. For all taxa, the refuge effect was never greater than 57% of that taxon’s toxicity effect, and often substantially smaller. In the case of Dermaptera for example, the refuge effect was 7.2 and the toxicity effect 41.7. This is despite the fact that Dermaptera, along with ground active Araneae and Formicidae, were the taxa that exhibited the strongest refuge effect. The taxa with weakest refuge effects were Carabidae and aerial Aranae and Staphilinidae, the same taxa that were least affected by pesticide toxicity. Collectively, this series of BN sensitivity analyses provides a clear indication that for a wide range of natural enemy taxa and guilds (e.g., ground active vs. aerial), the pesticide regime of a crop field is a stronger driver of population size than any potential effect of nearby donor habitat.
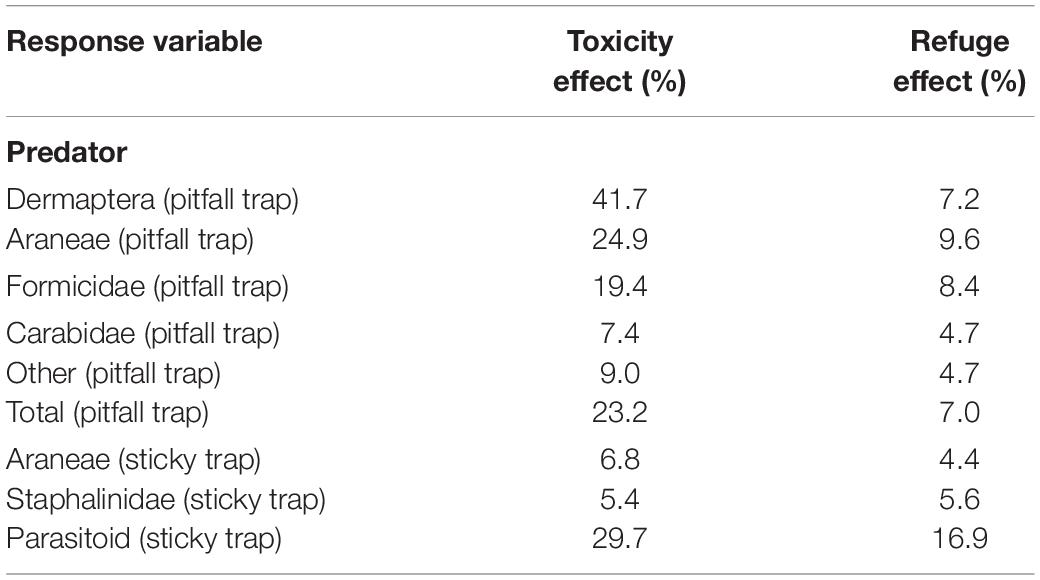
Table 3. Relative strength of effect from pesticide regime used in the field (“toxicity effect”) and from the presence of woody vegetation adjacent to one margin of field (“refuge effect”) on various natural enemy groups derived from Bayesian network sensitivity analysis.
The potential strength of benefit to in-crop densities of many natural enemies from perennial native vegetation was clearly apparent from descriptive data analysis of the least intensively sprayed field. For ground active taxa (Figure 5A), there were elevated numbers of individuals in areas of the crop closest to the woody vegetation consistent with it serving as donor habitat, especially for the more common taxa of Dermaptera, and Formicidae. Numbers of these insects were strongly elevated close to the native vegetation, an effect that was apparent for around 100 m. Weaker levels of elevation that tended to decay more rapidly were apparent for scarcer ground-active taxa: Araneae, Carabidae, and the category “other” (i.e., not among the afore-named taxa). For aerial arthropod arthropods, parasitic Hymenoptera were the most numerous overall and exhibited a very strong refuge effect that, like the ground active Dermaptera, and Formicidae, was apparent for 100 m into the field (Figure 5B). No refuge effect was apparent for other aerial natural enemies.
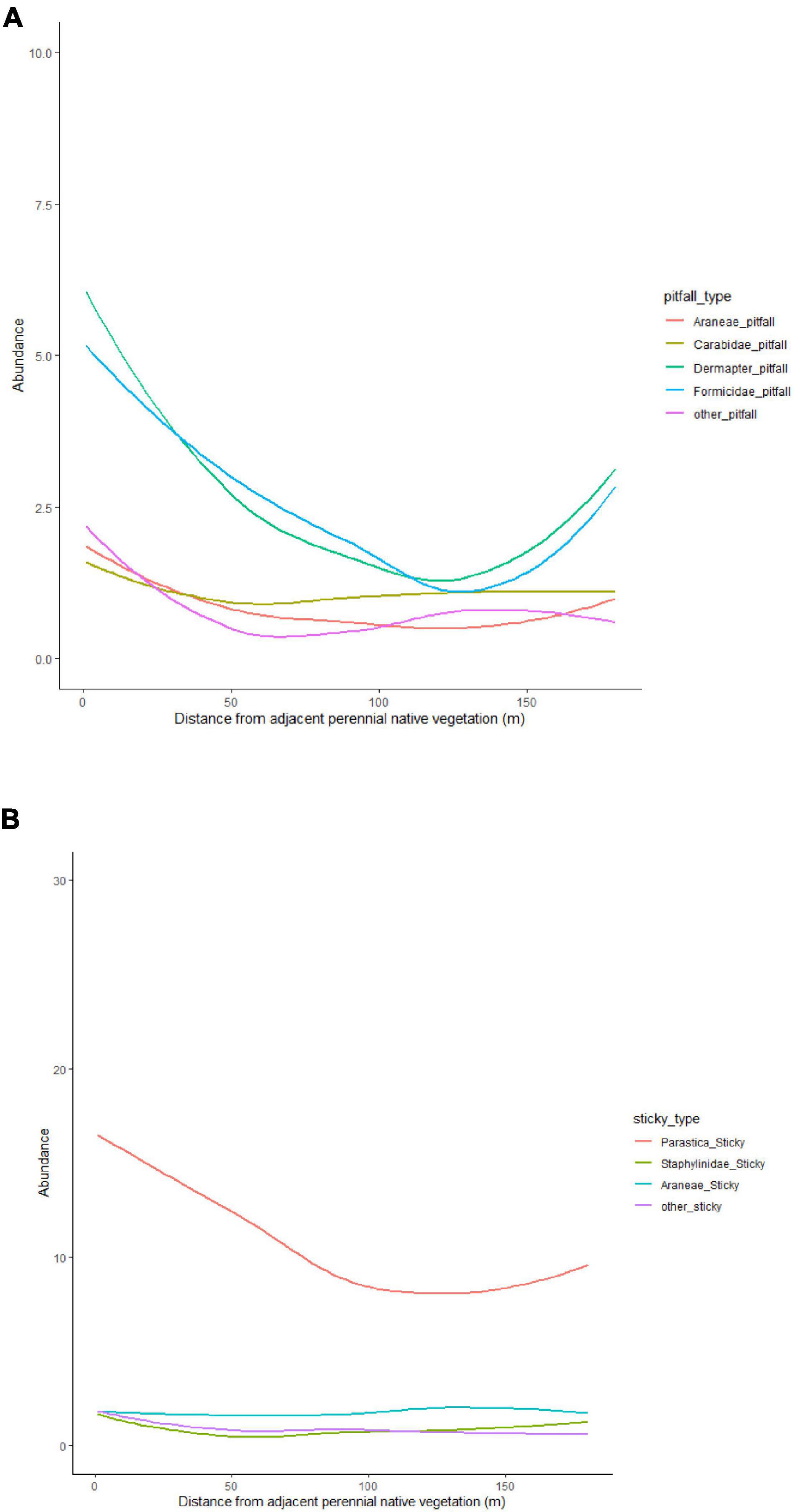
Figure 5. Effect on natural enemy groups of perennial woody vegetation adjacent to one margin of a brassica vegetable field with a pesticide regime calculated to have low toxicity. (A) Ground active predators (pitfall trap catches) and (B) aerial groups, including ballooning spiders (sticky trap catches).
Discussion
We found the abundance of ground active predators and constituent taxa were highly reduced in fields with high pesticide toxicity scores. In fields with lower toxicity scores, the presence of adjacent perennial native vegetation influenced in-field spatial distribution of ground active predators and constituent taxa. The abundance of natural enemies was higher adjacent to perennial native vegetation in the field with lower toxicity score whereas in the fields with relatively higher toxicity score no variation in numbers were observed according to the distance from perennial native vegetation. Importantly, however, intense insecticide use appeared to over-ride the benefits of adjacent woody vegetation in the fields with relatively higher toxicity score to the extent that in-crop abundance of these natural enemies close to the woody vegetation were as low as in remote parts of the field.
Generally, the presence of non-crop vegetation close to crops is widely observed in agroecosystems to offer potential support to natural enemies and biological pest control (Alignier et al., 2014; Schellhorn et al., 2014; Blaauw and Isaacs, 2015; Woodcock et al., 2016; Heimoana et al., 2017). However, inconsistent effects have also been reported in other studies (Blitzer et al., 2012; Jonsson et al., 2012; Parry et al., 2015) and a recent meta-analysis confirmed inconsistency in the response of natural enemy abundance as well as pest suppression and crop yield (Karp et al., 2018). Field management practices such as use of chemical pesticides have been proposed as one of the factors likely to account for the range of observed responses (Tscharntke et al., 2016; Begg et al., 2017) and the present study provides support for this effect to the extent that it overcame the effect of nearby woody vegetation which is likely to have provided resources (e.g., shelter, alternative foods) and served as donor habitat for enemies.
There are few studies of the role of non-crop vegetation on natural enemies in fields with pesticide sprayed crops (Lee et al., 2001; Zhu et al., 2017). The former of those studies suggested that refuge habitats can buffer the negative effects of insecticide use on carabid beetles in a temperate system but indicated that insecticide impact should be minimized during the period of carabid emergence to increase their activity in crops. The later study found, in sub-tropical rice, that the strong benefits to natural enemies of strips of flowering sesame were negated by insecticide application to the rice crop. This high level of disruption is likely to be accounted for by spatial scale. The crop margin flower strips in Zhu et al. (2017) were narrow and immediately adjacent to the sprayed crop so as not effective as a complete, spray-drift-free refuge for natural enemies. In the present study, the perennial woody vegetation present on one edge of all fields extended for at least 30 m from the crop margin. This, together with the height and structural complexity of the woody vegetation, would have greatly reduced the extent to which crop spraying penetrated and impacted natural enemies in the woodland, allowing it to serve more effectively as a refuge for, and source of, natural enemies. However, the adjacent crop fields in this study presented a hostile environment for natural enemies due to the use of broad-spectrum insecticides such as organophosphates, carbamates, and pyrethroids. Earlier studies have demonstrated impacts, including residual effects of these broad spectrum insecticides on predators such as beetles (O’Neal et al., 2005), spiders (Maloney et al., 2003), ladybugs and other predators (Roubos et al., 2014). Consistent with this, studies have concluded that pesticide toxicity is a major factor in disruption to biological control in crop fields despite the potential support of natural vegetation (Geiger et al., 2010; Jonsson et al., 2012; Gagic et al., 2019). The present study supports that general conclusion but suggests that natural enemy taxon and guild (e.g., dispersal capacity and life history omnivory) can strongly influence outcomes.
In contrast to several taxa of ground active predators captured in pitfall traps, sticky trap catches of Staphilinidae and Araneae were less strongly affected by toxicity scores of the contrasting fields. Many species of Staphilinidae fly and the spiders caught on sticky traps can be assumed to have been captured whilst undergoing ballooning dispersal. Among the predators captured in pitfall traps, Carabidae had the least strong toxicity effect in the BN sensitivity analysis and this family includes species capable of flight. Accordingly, the observed effects are likely to reflect their dispersal mode, an important factor in driving how natural enemies are affected by crop management practices and non-crop vegetation (Sorribas et al., 2016; Gagic et al., 2019). In this study, the vagility of the named taxa would allow them to recolonize a sprayed field more rapidly than taxa such as Dermaptera and ground-active spiders that are more likely dependent on walking. The same effects of dispersal capacity can explain the relative strength of refuge effects among the natural enemy taxa: those capable of flight or ballooning being less dependent on nearby donor habitats because they can reach all areas of a crop field equally well from even relatively remote refuges. Hymenopteran parasitoids, however, exhibited a strong toxicity effect and refuge effect despite flight capacity being virtual ubiquitous in this group. This apparent anomaly can be explained by the fact that the vast majority of the parasitoids captured were small or minute species that have relatively weak flight capacity. Additionally, adult parasitoids (including species of importance in brassica crops) exhibit life history omnivory, carnivores as immatures and nectar feeders as adults, sostrongly benefit from access to appropriate nectar plants (Pandey et al., 2018; Pandey and Gurr, 2019; Gardarin et al., 2021). Accordingly, the perennial native vegetation areas in the present study, that also had herbaceous plants in the groundcover vegetation, will have provided nectar in addition to physical shelter making these areas of double value to parasitoids. This contrasts with taxa such as Caribidae, Staphilinidae, and Araneae that do not feed on nectar and for which a shelter effect is more important.
In conclusion, perennial native vegetation has a good potential as a donor habitat for natural enemies in the studied crops, however, this can be negated by pesticide use. The abundance of ground active predators and parasitoids was markedly decreased in fields with higher levels pesticide use and the benefits provided by adjacent vegetation were evident only under conditions of low pesticide toxicity score. For the more vagile canopy dwelling predators, pesticide regime had a weaker effect on abundance and these were less likely to be reliant on nearby donor habitat. These results highlight the need for a nuanced approach to providing recommendations for conservation biological control, and possibly for arthropod conservation in farm landscapes more generally. Whilst providing donor habitats and moderating insecticide regimes are useful first approximations for promoting natural enemies in crop fields, there is a strong need to consider key ecological traits—especially those relating to dispersal capacity and life history omnivory of key natural enemies—when planning conservation biological control interventions.
Data Availability Statement
The raw data supporting the conclusions of this article will be made available by the authors, without undue reservation.
Author Contributions
SP and GG conceived the study. SP collected and identified samples. SP, GG, and GX analyzed the data. All authors wrote the manuscript.
Funding
SP was supported by an Australian Government Endeavor Postgraduate Scholarship.
Conflict of Interest
The authors declare that the research was conducted in the absence of any commercial or financial relationships that could be construed as a potential conflict of interest.
Publisher’s Note
All claims expressed in this article are solely those of the authors and do not necessarily represent those of their affiliated organizations, or those of the publisher, the editors and the reviewers. Any product that may be evaluated in this article, or claim that may be made by its manufacturer, is not guaranteed or endorsed by the publisher.
Acknowledgments
We are grateful to the farmers who allowed us onto their properties and provided crop management information and Pingyang Zhu for helping with field work.
Footnotes
References
Alignier, A., Raymond, L., Deconchat, M., Menozzi, P., Monteil, C., Sarthou, J. P., et al. (2014). The effect of semi-natural habitats on aphids and their natural enemies across spatial and temporal scales. Biol. Control 77, 76–82. doi: 10.1016/j.biocontrol.2014.06.006
Asbjornsen, H., Hernandez-Santana, V., Liebman, M., Bayala, J., Chen, J., Helmers, M., et al. (2014). Targeting perennial vegetation in agricultural landscapes for enhancing ecosystem services. Renew. Agric. Food Syst. 29, 101–125.
Begg, G. S., Cook, S. M., Dye, R., Ferrante, M., Franck, P., Lavigne, C., et al. (2017). A functional overview of conservation biological control. Crop Protect. 97, 145–158.
Bianchi, F. J. J. A., Booij, C. J. H., and Tscharntke, T. (2006). Sustainable pest regulation in agricultural landscapes: a review on landscape composition, biodiversity and natural pest control. Proc. R. Soc. Lond. B Biol. Sci. 273, 1715–1727. doi: 10.1098/rspb.2006.3530
Bianchi, F. J. J. A., Walters, B. J., Ten Hove, A. L. T., Cunningham, S. A., Van Der Werf, W., Douma, J. C., et al. (2015). Early-season crop colonization by parasitoids is associated with native vegetation, but is spatially and temporally erratic. Agric. Ecosyst. Environ. 207, 10–16.
Blaauw, B. R., and Isaacs, R. (2015). Wildflower plantings enhance the abundance of natural enemies and their services in adjacent blueberry fields. Biol. Control 91, 94–103.
Blitzer, E. J., Dormann, C. F., Holzschuh, A., Klein, A.-M., Rand, T. A., and Tscharntke, T. (2012). Spillover of functionally important organisms between managed and natural habitats. Agric. Ecosyst. Environ. 146, 34–43. doi: 10.1098/rspb.2016.0896
Bommarco, R., Kleijn, D., and Potts, S. G. (2013). Ecological intensification: harnessing ecosystem services for food security. Trends Ecol. Evol. 28, 230–238. doi: 10.1016/j.tree.2012.10.012
Bommarco, R., Miranda, F., Bylund, H., and Björkman, C. (2011). Insecticides suppress natural enemies and increase pest damage in cabbage. J. Econ. Entomol. 104, 782–791. doi: 10.1603/ec10444
Burghardt, K. T., Tallamy, D. W., and Gregory Shriver, W. (2009). Impact of native plants on bird and butterfly biodiversity in suburban landscapes. Conserv. Biol. 23, 219–224. doi: 10.1111/j.1523-1739.2008.01076.x
Chaplin-Kramer, R., De Valpine, P., Mills, N. J., and Kremen, C. (2013). Detecting pest control services across spatial and temporal scales. Agric. Ecosyst. Environ. 181, 206–212.
Chrobock, T., Winiger, P., Fischer, M., and Van Kleunen, M. (2013). The cobblers stick to their lasts: pollinators prefer native over alien plant species in a multi-species experiment. Biol. Invas. 15, 2577–2588.
Deekshita, K., Rao, C. R., Rani, C. S., and Kumari, V. P. (2017). Effect of new insecticide molecules on predators of rice ecosystem. J. Entomol. Zool. Stud. 5, 1617–1620.
Duffield, S. J., Jepson, P. C., Wratten, S. D., and Sotherton, N. W. (1996). Spatial changes in invertebrate predation rate in winter wheat following treatment with dimethoate. Entomol. Exp. Appl. 78, 9–17.
Elzen, G., Elzen, P., and King, E. (1998). Laboratory toxicity of insecticide residues to Orius insidiosus, Geocoris punctipes, Hippodamia convergens and Chrysoperla carnea. Southwest. Entomol. 23, 335–342.
Fiedler, A. K., and Landis, D. A. (2007). Attractiveness of Michigan native plants to arthropod natural enemies and herbivores. Environ. Entomol. 36, 751–765. doi: 10.1603/0046-225x(2007)36[751:aomnpt]2.0.co;2
Gagic, V., Hulthen, A. D., Marcora, A., Wang, X., Jones, L., and Schellhorn, N. A. (2019). Biocontrol in insecticide sprayed crops does not benefit from semi-natural habitats and recovers slowly after spraying. J. Appl. Ecol. 56, 2176–2185. doi: 10.1111/1365-2664.13452
Galvan, T. L., Koch, R. L., and Hutchison, W. D. (2006). Toxicity of indoxacarb and spinosad to the multicolored Asian lady beetle, Harmonia axyridis (Coleoptera: Coccinellidae), via three routes of exposure. Pest Manag. Sci. 62, 797–804. doi: 10.1002/ps.1223
Gardarin, A., Pigot, J., and Valantin-Morison, M. (2021). The hump-shaped effect of plant functional diversity on the biological control of a multi-species pest community. Sci. Rep. 11:21635. doi: 10.1038/s41598-021-01160-2
Geiger, F., Bengtsson, J., Berendse, F., Weisser, W. W., Emmerson, M., Morales, M. B., et al. (2010). Persistent negative effects of pesticides on biodiversity and biological control potential on European farmland. Basic Appl. Ecol. 11, 97–105.
Gill, K. A., Cox, R., and O’Neal, M. E. (2014). Quality over quantity: buffer strips can be improved with select native plant species. Environ. Entomol. 43, 298–311. doi: 10.1603/EN13027
Goulet, H., and Huber, J. T. (eds). (1993). Hymenoptera of the World: An Identification Guide to Families. Ottawa, ON: Agriculture Canada.
Gurr, G. M., Wratten, S. D., Landis, D. A., and You, M. S. (2017). Habitat management to suppress pest populations: progress and prospects. Annu. Rev. Entomol. 62, 91–109. doi: 10.1146/annurev-ento-031616-035050
Heimoana, V., Pilkington, L. J., Raman, A., Mitchell, A., Nicol, H. I., Johnson, A. C., et al. (2017). Integrating spatially explicit molecular and ecological methods to explore the significance of non-crop vegetation to predators of brassica pests. Agric. Ecosyst. Environ. 239, 12–19.
Isaacs, R., Tuell, J., Fiedler, A., Gardiner, M., and Landis, D. (2009). Maximizing arthropod-mediated ecosystem services in agricultural landscapes: the role of native plants. Front. Ecol. Environ. 7, 196–203. doi: 10.1890/080035
Jonsson, M., Buckley, H. L., Case, B. S., Wratten, S. D., Hale, R. J., and Didham, R. K. (2012). Agricultural intensification drives landscape-context effects on host-parasitoid interactions in agroecosystems. J. Appl. Ecol. 49, 706–714.
Karp, D. S., Chaplin-Kramer, R., Meehan, T. D., Martin, E. A., Declerck, F., Grab, H., et al. (2018). Crop pests and predators exhibit inconsistent responses to surrounding landscape composition. Proc. Natl. Acad. Sci. U.S.A. 115, E7863–E7870. doi: 10.1073/pnas.1800042115
Kjærulff, U. B., and Madsen, A. L. (2008). Bayesian Networks and Influence Diagrams: A Guide to Construction and Analysis. Cham: Springer.
Korb, K. B., and Nicholson, A. E. (2011). Bayesian Artificial Intelligence. Boca Raton, FL: CRC Press.
Landis, D. A., and Marino, P. C. (1999). “Landscape structure and extra-field processes: impact on management of pests and beneficials,” in Handbook of Pest Management, ed. J. Ruberson (New York, NY: Marcel Dekker), 79–104.
Landis, D. A., Gardiner, M. M., and Tompkins, J.-M. L. (2012). “Using native plant species to diversify agriculture,” in Biodiversity and Insect Pests: Key Issues for Sustainable Management, eds G. M. Gurr, S. D. Wratten, W. E. Snyder, and D. M. Y. Read (Chichester: Wiley-Blackwell), 276–292. doi: 10.1002/9781118231838.ch17
Larsen, N. J., Minor, M. A., Cruickshank, R. H., and Robertson, A. W. (2014). Optimising methods for collecting Hymenoptera, including parasitoids and Halictidae bees, in New Zealand apple orchards. J. Asia Pac. Entomol. 17, 375–381. doi: 10.1016/j.aspen.2014.03.004
Lee, J. C., Menalled, F. B., and Landis, D. A. (2001). Refuge habitats modify impact of insecticide disturbance on carabid beetle communities. J. Appl. Ecol. 38, 472–483. doi: 10.1046/j.1365-2664.2001.00602.x
Leggett, J. (1990). The influence of ULV malathion, applied for boll weevil control, on other pest and beneficial species in Arizona cotton fields. Cotton Coll. Agric. Rep. **VPQ,
Lu, Y. H., Wu, K. M., Jiang, Y. Y., Guo, Y. Y., and Desneux, N. (2012). Widespread adoption of Bt cotton and insecticide decrease promotes biocontrol services. Nature 487, 362–365. doi: 10.1038/nature11153
Lykogianni, M., Bempelou, E., Karamaouna, F., and Aliferis, K. A. (2021). Do pesticides promote or hinder sustainability in agriculture? The challenge of sustainable use of pesticides in modern agriculture. Sci. Total Environ. 795:148625. doi: 10.1016/j.scitotenv.2021.148625
Macfadyen, S., Hopkinson, J., Parry, H., Neave, M. J., Bianchi, F. J. J. A., Zalucki, M. P., et al. (2015). Early-season movement dynamics of phytophagous pest and natural enemies across a native vegetation-crop ecotone. Agric. Ecosyst. Environ. 200, 110–118.
Maloney, D., Drummond, F. A., and Alford, R. (2003). Spider Predation in Agroecosystems: Can Spiders Effectively Control Pest Population?, Vol. 190. Orono: Maine Agricultural and Forest Experiments Station.
Mansfield, S., Dillon, M. L., and Whitehouse, M. E. A. (2006). Are arthropod communities in cotton really disrupted? An assessment of insecticide regimes and evaluation of the beneficial disruption index. Agric. Ecosyst. Environ. 113, 326–335. doi: 10.1016/j.agee.2005.10.012
Markó, V., Elek, Z., Kovács-Hostyánszki, A., Kőrösi, Á, Somay, L., Földesi, R., et al. (2017). Landscapes, orchards, pesticides–Abundance of beetles (Coleoptera) in apple orchards along pesticide toxicity and landscape complexity gradients. Agric. Ecosyst. Environ. 247, 246–254.
McCravy, K. W. (2018). A review of sampling and monitoring methods for beneficial arthropods in agroecosystems. Insects 9:170. doi: 10.3390/insects9040170
Morandin, L. A., Long, R. F., and Kremen, C. (2014). Hedgerows enhance beneficial insects on adjacent tomato fields in an intensive agricultural landscape. Agric. Ecosyst. Environ. 189, 164–170.
O’Neal, M. E., Mason, K. S., and Isaacs, R. (2005). Seasonal abundance of ground beetles in highbush Blueberry (Vaccinium corymbosum) fields and response to a reduced-risk insecticide program. Environ. Entomol. 34, 378–384.
Ockleford, C., Adriaanse, P., Berny, P., Brock, T., Duquesne, S., Grilli, S., et al. (2017). Scientific opinion addressing the state of the science on risk assessment of plant protection products for in-soil organisms. EFSA J. 15:e04690. doi: 10.2903/j.efsa.2017.4690
Pandey, S., and Gurr, G. M. (2019). Conservation biological control using Australian native plants in a brassica crop system: seeking complementary ecosystem services. Agric. Ecosyst. Environ. 280, 77–84. doi: 10.1016/j.agee.2019.04.018
Pandey, S., Rahman, A., and Gurr, G. M. (2018). Australian native flowering plants enhance the longevity of three parasitoids of brassica pests. Entomol. Exp. Appl. 166, 265–276. doi: 10.1111/eea.12668
Parry, H. R., Macfadyen, S., Hopkinson, J. E., Bianchi, F. J. J. A., Zalucki, M. P., Bourne, A., et al. (2015). Plant composition modulates arthropod pest and predator abundance: evidence for culling exotics and planting natives. Basic Appl. Ecol. 16, 531–543. doi: 10.1016/j.baae.2015.05.005
Perović, D. J., Gurr, G. M., Simmons, A. T., and Raman, A. (2011). Rubidium labelling demonstrates movement of predators from native vegetation to cotton. Biocontrol Sci. Technol. 21, 1143–1146. doi: 10.1080/09583157.2011.607232
R Core Team (2020). R: A Language and Environment for Statistical Computing (version 4.0.3). Vienna: R foundation for statistical computing.
Retallack, M. J., Thomson, L. J., and Keller, M. A. (2019). Predatory arthropods associated with potential native insectary plants for Australian vineyards. Aust. J. Grape Wine Res. 25, 233–242.
Roubos, C. R., Rodriguez-Saona, C., Holdcraft, R., Mason, K. S., and Isaacs, R. (2014). Relative toxicity and residual activity of insecticides used in blueberry pest management: mortality of natural enemies. J. Econ. Entomol. 107, 277–285. doi: 10.1603/ec13191
Schellhorn, N. A., Bianchi, F. J. J. A., and Hsu, C. L. (2014). Movement of entomophagous arthropods in agricultural landscapes: links to pest suppression. Annu. Rev. Entomol. 59, 559–581. doi: 10.1146/annurev-ento-011613-161952
Shields, M. W., Johnson, A. C., Pandey, S., Cullen, R., González-Chang, M., Wratten, S. D., et al. (2019). History, current situation and challenges for conservation biological control. Biol. Control 131, 25–35. doi: 10.1016/j.biocontrol.2018.12.010
Shields, M. W., Tompkins, J. M., Saville, D. J., Meurk, C. D., and Wratten, S. (2016). Potential ecosystem service delivery by endemic plants in New Zealand vineyards: successes and prospects. PeerJ 4:e2042. doi: 10.7717/peerj.2042
Sorribas, J., González, S., Domínguez-Gento, A., and Vercher, R. (2016). Abundance, movements and biodiversity of flying predatory insects in crop and non-crop agroecosystems. Agron. Sustain. Dev. 36:34.
Thomine, E., Mumford, J., Rusch, A., and Desneux, N. (2022). Using crop diversity to lower pesticide use: socio-ecological approaches. Sci. Total Environ. 804:150156. doi: 10.1016/j.scitotenv.2021.150156
Thomson, L. J., and Hoffmann, A. A. (2006). Field validation of laboratory-derived IOBC toxicity ratings for natural enemies in commercial vineyards. Biol. Control 39, 507–515. doi: 10.1016/j.biocontrol.2006.06.009
Thomson, L. J., and Hoffmann, A. A. (2010). Natural enemy responses and pest control: importance of local vegetation. Biol. Control 52, 160–166.
Thomson, L. J., and Hoffmann, A. A. (2013). Spatial scale of benefits from adjacent woody vegetation on natural enemies within vineyards. Biol. Control 64, 57–65.
Torres, J. B., and Bueno, A. D. F. (2018). Conservation biological control using selective insecticides – A valuable tool for IPM. Biol. Control 126, 53–64.
Tscharntke, T., Grass, I., Wanger, T. C., Westphal, C., and Batáry, P. (2021). Beyond organic farming – harnessing biodiversity-friendly landscapes. Trends Ecol. Evol. 36, 919–930. doi: 10.1016/j.tree.2021.06.010
Tscharntke, T., Karp, D. S., Chaplin-Kramer, R., Batáry, P., Declerck, F., Gratton, C., et al. (2016). When natural habitat fails to enhance biological pest control – Five hypotheses. Biol. Conserv. 204, 449–458.
Wanumen, A. C., Carvalho, G. A., Medina, P., Viñuela, E., and Adán, Á (2016). Residual acute toxicity of some modern insecticides toward two mirid predators of tomato pests. J. Econ. Entomol. 109, 1079–1085. doi: 10.1093/jee/tow059
Whitehouse, T. S., Sial, A. A., and Schmidt, J. M. (2018). Natural enemy abundance in southeastern blueberry agroecosystems: distance to edge and impact of management practices. Environ. Entomol. 47, 32–38. doi: 10.1093/ee/nvx188
Woodcock, B. A., Bullock, J. M., Mccracken, M., Chapman, R. E., Ball, S. L., Edwards, M. E., et al. (2016). Spill-over of pest control and pollination services into arable crops. Agric. Ecosyst. Environ. 231, 15–23.
Keywords: conservation biological control, refuge habitat, pesticide toxicity, Bayesian network, predator, parasitoid, habitat management, brassica
Citation: Pandey S, Johnson AC, Xie G and Gurr GM (2022) Pesticide Regime Can Negate the Positive Influence of Native Vegetation Donor Habitat on Natural Enemy Abundance in Adjacent Crop Fields. Front. Ecol. Evol. 10:815162. doi: 10.3389/fevo.2022.815162
Received: 15 November 2021; Accepted: 16 February 2022;
Published: 07 April 2022.
Edited by:
James Moran, Galway-Mayo Institute of Technology, IrelandReviewed by:
Francisco Sánchez-Bayo, The University of Sydney, AustraliaMaría Pérez-Marcos, Instituto Murciano de Investigación y Desarrollo Agrario y Alimentario (IMIDA), Spain
Copyright © 2022 Pandey, Johnson, Xie and Gurr. This is an open-access article distributed under the terms of the Creative Commons Attribution License (CC BY). The use, distribution or reproduction in other forums is permitted, provided the original author(s) and the copyright owner(s) are credited and that the original publication in this journal is cited, in accordance with accepted academic practice. No use, distribution or reproduction is permitted which does not comply with these terms.
*Correspondence: Sunita Pandey, cGFuZGV5LnN1bml0YTIwMDlAZ21haWwuY29t