- 1School of Life and Environmental Sciences, University of Sydney, Sydney, NSW, Australia
- 2Flora and Fauna Division, Department of Environment, Parks and Water Security, Northern Territory Government, Arid Zone Research Institute, Alice Springs, NT, Australia
- 3CSIRO, Land and Water, Darwin, NT, Australia
Urban environments provide the only or best habitats that are left for wildlife in many areas, promoting increased interest in urban conservation and a need to understand how wildlife cope with urban stressors, such as altered predator activity and human disturbance. Here, we used filmed giving-up density experiments to investigate behavioral coping responses of foraging small prey animals at three sites (close, mid, and far) along an urban disturbance gradient. Our study design included “natural” and experimentally added stressor cues of predators and/or human disturbance. We observed small mammal foraging behaviors, particularly: the common brushtail possum (Trichosurus vulpecula), northern brown bandicoot (Isoodon macrourus), brown antechinus (Antechinus stuartii), black rat (Rattus rattus), and brown rat (Rattus norvegicus), and to a lesser degree several species of native birds. We found that at the close urban-edge environment, coping responses to human disturbances were most pronounced, and predator cues from the red fox (Vulpes vulpes) were perceived as least risky. However, at the mid environment, red fox cues were perceived as most risky, especially when combined with human disturbance. At the far environment, domestic cat (Felis catus) cues were perceived as most risky, again when combined with human disturbance. Impacts from the combined stressors of predator and human disturbance cues appeared to be additive, with higher risk being perceived with increasing distance from urban build-up. Behavioral adjustments were observed to be the primary response to stressors by small prey animals in the close environment. In the mid environment, slight temporal shifts in activity across the night were more evident. In the far environment, habitat components were likely being used differently as the primary coping response to stressors. As mostly the same species were observed along the disturbance gradient, our results suggest a level of response plasticity that is calibrated to the level of exposure to a stressor and the stressor type. To maximize conservation outcomes in urban habitats, we therefore propose that management should be sensitive to the level and history of human disturbance, as this affects the coping responses of wildlife that remain.
Introduction
Urban areas support many flora and fauna species of conservation concern (Cincotta et al., 2000; Seto et al., 2012; Ives et al., 2016; Wintle et al., 2019), and often provide the only suitable habitat that is left (Hobbs et al., 2013). Conservation of biodiversity may therefore become increasingly reliant upon effective management of urban environments (Dearborn and Kark, 2010; Soanes and Lentini, 2019). Urban environments present benefits to wildlife such as year-round supplementary resources, which may be beneficial even to the soil microbiome (Delgado-Baquerizo et al., 2021; Lerman et al., 2021). However, urban environments also present stressors that wildlife have not coevolved with, such as introduced species (Doherty et al., 2016, 2017; Legge et al., 2020), human activity (Fernández-Juricic and Tellería, 2000; Frid and Dill, 2002), introduced light and sound (Navara and Nelson, 2007; Shannon et al., 2016), pollutants (Schwarzenbach et al., 2006), and traffic (Taylor and Goldingay, 2010). Further, humans often exert predator-like pressures on wildlife (Frid and Dill, 2002; Rehnus et al., 2014; Clinchy et al., 2016; Patten and Burger, 2018), which may particularly affect predators (Suraci et al., 2019). Predator activity in urban environments may itself be influenced by supplementary resources (Newsome et al., 2015; Reshamwala et al., 2018) or introduced predators, including domestic pets (Twardek et al., 2017). The “landscapes of fear” concept represents the perception by prey of their foraging habitat in terms of high and low predation risk, affecting how and where they forage in an environment, which can consequentially have population level impacts (Laundré et al., 2001). It follows then that in urban environments, landscapes of fear may be shaped by human activity in combination with altered or novel pressures from predators (Fardell et al., 2021a).
Urban ecology is a developing subdiscipline within conservation science (Magle et al., 2012; Collins et al., 2021). This new focus reflects a growing awareness that urban biodiversity conservation requires an understanding of the cumulative impacts of introduced and/or novel stressors on wildlife (McPhearson et al., 2016). Habitat structure can potentially be managed to reduce the impacts of combined stressors, such as predator presence and human disturbance (Fardell et al., 2021a), and has proven successful in increasing biodiversity and resources for urban wildlife (Threlfall et al., 2017). To reduce stress impacts on urban wildlife, it is first important to determine how animals alter their behavior when stressed. Behaviors that are consistently correlated with a stressor are defined as coping responses, and can be classified as passive/reactive or active/proactive (Koolhaas et al., 1999). A passive response describes conservation-withdrawal and is characterized by immobility and low aggression, whereas an active response is fight-or-flight and is characterized by territoriality and aggression (Koolhaas et al., 1999). Coping responses can also be understood as a spectrum that allows for threat detection and downgrading through behavioral adjustments, rather than being expressed categorically (Boulton et al., 2015), and may develop under consistent exposure to stressors across generations (Koolhaas et al., 1999). Rapid or dynamic changes in the levels or types of stressors in a system could therefore detrimentally affect animal fitness.
Introduced stressors in ecosystems may interact among themselves and with natural stressors (Côté et al., 2016). Exposure to stressors that are persistent and strongly negative can overwhelm an animal’s allostatic load and impair its ability to maintain homeostasis (McEwen and Wingfield, 2003; Dantzer et al., 2014). Persistently negative stressors may affect vital behaviors (Mineur et al., 2006), reproduction and immunity (Sapolsky et al., 2000), and also impair neurological/cardiovascular/musculoskeletal physiological systems (Roozendaal, 2000; McEwen, 2004). When glucocorticoids and other hormones in the hypothalamo-pituitary-adrenal axis elevate in response to a stressor, rapid physiological or behavioral responses, such as increased foraging, can reduce the allostatic load and enhance fitness and survival (Wingfield et al., 1998; McEwen and Wingfield, 2003). Elevated food consumption can occur under heavy stressor loads, as the energy required to forage increases, and increased glucocorticosteroid levels also increase appetite (McEwen and Wingfield, 2003). Impacts from a stressor may not subside until the threat is removed (Sapolsky et al., 2000). Responses to persistent novel stressors may, therefore, need to be developed to allow normal function (McEwen and Wingfield, 2003). Understanding whether or not wildlife is coping with exposure to ambient stressors and can successfully conduct vital behaviors like foraging, especially in highly disturbed environments, will allow better allocation of conservation efforts (Fardell et al., 2020).
Wildlife persistence in urban habitats may be promoted by access to supplementary resources (Boutin, 1990; Prevedello et al., 2013; Cox and Gaston, 2018) and changes in behavior (Lowry et al., 2013; Sih, 2013; Dammhahn et al., 2020) and/or temporal activity (Lendrum et al., 2017; Shamoon et al., 2018; Nickel et al., 2020). Such changes may reflect phenotypic plasticity (Alberti et al., 2017) or rapid adaptations driven by “human-altered selection pressures” (Otto, 2018). Phenotypic plasticity refers to the ability of a genotype to produce different phenotypes, and allows animals to adjust their behavior or morphology in response to changed environmental conditions; it is observed commonly in nature (Thibert-Plante and Hendry, 2011). Conversely, an inability to produce different phenotypes may limit population persistence in disturbed environments (Badyaev, 2005). Much research shows that phenotypic plasticity underpins the ability of wildlife to exploit urban environments (Donihue and Lambert, 2015). However, evolution that is adaptive (based on natural and sexual selection) and non-adaptive (based on genetic drift and gene flow) (Stearns and Hoekstra, 2000) also occurs in both flora and fauna in response to the pressures of human disturbed environments (Johnson and Munshi-South, 2017; Otto, 2018). Such evolution may occur more rapidly in urban dwelling species compared to their further removed counterparts (Yeh, 2004). Rapid change may, however, in some cases, ultimately lead to fitness loss (Otto, 2018).
Stress responses can be innate or acquired (Lambert et al., 2006) and can vary at the species level depending on differences in species’ morphology and historical exposure to stressors (Brodie and Brodie, 1999), as well as to different levels of disturbance in urban environments (Walker et al., 2005; Ditchkoff et al., 2006; Tang et al., 2020). However, increased familiarity with human disturbances may allow for persistent behavior-mediated responses to stressors. In certain bird species, for example, this reduces the need for ongoing responses and permits animals to persist in disturbed environments without experiencing high energy costs (Blumstein, 2014). Supplementary resources, including food, shelter, and water run-off to green spaces (Cox and Gaston, 2018), may also alter wildlife behavioral responses to stressors as they affect competitor and predator activity (Shochat et al., 2010; Newsome et al., 2015). In short, urban wildlife are required to exhibit spatially and temporally varied trade-offs to reduce stress while attaining sufficient resources, such that coping responses may differ along urban disturbance gradients.
Vigilance behavior can be used in response to stressors to detect approaching threats like predators (Lima and Dill, 1990) or people (Frid and Dill, 2002). However, it is context and experience dependent and may be used more under safe conditions than those perceived as risky (Brown, 1999; Kotler and Brown, 2017). “Optimal use of vigilance theory” proposes that, for an individual, use of vigilance is relative to previous encounter rates with predators, predator lethality, its effectiveness in reducing mortality, and food harvest rates if not vigilant (Lima, 1987; Brown, 1999). Vigilance may therefore differ in different environments or with access to different habitat components that modulate threats. Under conditions where vigilance has proven effective, i.e., in reducing risk whilst allowing for sufficient foraging success, the value of this behavior may be perceived as high (Brown, 1999). If risk levels remain high regardless of vigilance, vigilance may be perceived as less valuable and hence be used less (Brown, 1999; Kotler and Brown, 2017). For example, squirrels are more vigilant when foraging near to than away from trees; given that they can readily escape up trees, the vigilance value is increased (Kotler and Brown, 2017). Vigilance value can also be higher in less disturbed compared to heavily disturbed environments, as the cost of being vigilant to the many persistent disturbances would greatly reduce foraging time and impact fitness. For example, animals in urban environments can be comparatively bolder, compared to their more wild counterparts, owing to habituation to disturbances resulting in reduced vigilance (Uchida et al., 2019). Vigilance behaviors may also be reduced when an animal is highly stressed or fearful owing to the need for food taking priority (Beauchamp, 2017). Whilst vigilance behaviors may be used as a stress response, they may also be used to detect food or in social interactions (Beauchamp, 2017). Therefore, measurements in combination with food consumption rates offer a more reliable insight into fear and stress impacts and the relative use of vigilance (Kotler and Brown, 2017).
When predation risk is perceived as high, foraging prey species may benefit from employing a shuttling strategy of sourcing and removing food items to consume in safer conditions (Ovadia et al., 2001). Other behaviors, or physiological stress responses, may also be employed when foraging under stress, including increasing latency (Sterlemann et al., 2008), or time allocated to foraging (Brown, 1999). Indeed, such responses may even occur within a single night to meet animals’ nutritional needs if a stressor is detected but no additional information is then presented (Fardell et al., 2021b). Animals exposed to chronic stressors during adolescence may show phenotypic changes that enhance their function under increased or novel threats, through the development of the foraging behaviors of increased transitions across patches, reduced/increased latency, and increased food consumption (Chaby et al., 2015). Although such adjustments allow wildlife to persist in disturbed environments, there may be lasting impacts on their health and function, including over- and under-reacting to stimuli, reducing their quality of life (Wulsin et al., 2016; Smith et al., 2021).
Temporal changes in activity may also occur if perceived risks from stressors are high and resources are available at alternative times (e.g., Makin et al., 2017; Wu et al., 2018; Smith et al., 2019). However, high activity overlaps between species, including predators and prey, are likely if the ecosystem can support the diversity of species present (Kronfeld-Schor and Dayan, 2003). Heterogeneity, complexity, size, number and connectedness of vegetated habitat patches likely aid this (Bazzaz, 1975; Brown, 2000; Pianka, 2011; Shanahan et al., 2011; Nielsen et al., 2014), and could increase suitable conditions in urban areas. Still, human disturbances have been observed to drive activity shifts in mammals from primarily diurnal to nocturnal (Gaynor et al., 2018), although this response is not common. Anthropogenic sound and light can, similarly, affect wildlife behavior shifts, depending on when these sensory pollutants occur (Dominoni et al., 2020). As such chronic stressors are prevalent in urban environments, such developments may be more evident in urban-edge wildlife than in their further removed counterparts.
It is evident that wildlife species have numerous avenues by which they can survive in urban environments, and in some cases flourish (e.g., Lyons et al., 2017; Fingland et al., 2021). However, at what cost is this occurring, and by which mechanisms? The answers may be relevant in terms of the timing and direction of conservation efforts. In a companion study (Fardell et al., 2021a), we related foraging activity by small prey animals as giving-up density (GUD) measures to habitat component use and distances to predators and human disturbances, under both natural conditions and with introduced stressor cues of human disturbance (sound and light) and/or introduced predators (integumentary scent cues). Patch GUD measurements reveal the amount of food consumed in a grid of patches across a landscape and allow for investigation into factors that may impact optimal foraging behavior, as it is based on the theory that a patch will no longer be foraged once the combined energetic, missed opportunity costs and predation risks are higher than the harvest rate (Brown, 1988). In our companion study we found that, when foraging, habitat components were used differently in response to stressors. Consequently, we proposed that the observed differences in foraging activity could be attributed to differences in behavioral stress responses. In this present study we examine this idea in detail by observing small mammal, and to a lesser degree, bird stress response behaviors used whilst foraging. Our aim is to better understand their primary coping responses to the combined and singular stressors of altered/novel predator activity and human disturbance, along an urban disturbance gradient. To do so, we investigate four questions, relating to small prey animals:
(1) Do introduced stressors influence: (i) foraging efforts (GUD), (ii) number of visitors to food stations, (iii) time spent at food stations, and (iv) time spent in vigilance?
(2) Does proximity to urban build-up influence the level at which the observed behavioral stress responses are expressed?
(3) Are behavioral responses different among species?
(4) Do introduced stressors cause temporal shifts in times active?
In general, we expected that under experimental treatments that combined cues of predators and human disturbance, the perceived risk would be higher than treatments presenting singular cues, resulting in lower foraging efforts (higher GUDs) with fewer visits and time at food stations, but more vigilance. Further, that responses closer to houses would be more pronounced than in connected but more distant environments along the disturbance gradient. Additionally, that differences between the present species’ responses would be relative to morphology, size, and foraging mode. Finally, that differences in temporal activity would be relative to proximity to human disturbances and the level and type of human activity.
Materials and Methods
Study Area
As described in Fardell et al. (2021a,c), this study was undertaken in suburban Whitebridge in the Lake Macquarie district of New South Wales, Australia. The environment is biodiverse, interspersed with patches of remnant vegetation and bordered by Glenrock State Conservation Area (GSCA) and Awabakal Nature Reserve. We conducted our research along an urban disturbance gradient that is detailed and depicted in Fardell et al. (2021a). The gradient primarily differed in distance to urban build-up and consequent levels of human disturbance across three “environments”: (1) a “close environment” – a green space patch of dry sclerophyll forest surrounded by houses, a grass sports oval that was in use day and night, and roads; (2) a “mid environment” – a connected dry sclerophyll forest within an empty block of state-owned land that was seamlessly contiguous with the GSCA on the eastern side and had roads on the southern and western sides and a recreational path to the north; and (3) a “far environment” – a connected narrow remnant rainforest patch along an ephemeral creek line in GSCA, surrounded by dry sclerophyll forest. Upon trials to select these study sites, weather readings were taken, including: temperature, humidity, dew point, wet bulb, and wind speed. These readings varied only minimally between the three sites, and so were determined as unlikely to influence any responses observed. Habitat complexity and vegetation in the three environments was very similar. Human activity frequency ranged from highest at the close environment to lowest at the far environment, owing largely to the relative ease/difficulty of access. Throughout this area, the powerful owl (Ninox strenua, weight: 1240–1700 g, wingspan: 112–135 cm) is the native apex predator, and the lace monitor (Varanus varius, weight: 14 kg, snout-vent length: 76.5 cm) and diamond python (Morelia spilota spilota, weight: 15 kg, size: 2–4 m), are native mesopredators (Fardell et al., 2021b). Each of these reptiles feeds primarily on smaller animals, but occasionally consumes larger mammals, like possums (700–4500 g) and bandicoots (500–3100 g) (Slip and Shine, 1988; Jessop et al., 2010). Introduced predators, the feral or pet domestic cat (Felis catus, weight: 2.5–7.3 kg, snout-vent length: 38–74 cm) and European red fox (Vulpes vulpes, weight: 4–8.3 kg, snout-vent length: 57–74 cm), also occur commonly, adding to predation pressure (Lake Macquarie City Council [LMCC], 2012). The vegetated patches are frequently used by bike riders, horse riders, runners, walkers, and dog walkers (Department of Environment, Climate Change and Water [DECCW], 2010) by day and night (pers. obs. Fardell, 2019). These activities potentially affect wildlife stress levels and activity (Gander and Ingold, 1997; Frid and Dill, 2002; Larson et al., 2016; Bleicher and Rosenzweig, 2018).
Survey Methods
The monitoring of wildlife was conducted under animal ethics approval from the University of Sydney (2017/1275), and a New South Wales Scientific License (SL102024). Survey methods are described in detail in Fardell et al. (2021a). In summary, small mammal foraging behavior was examined using nocturnal GUD trials (Brown, 1988), four times between January and September 2019, at each of the three environments along the disturbance gradient. There were 24 GUD stations at each environment, each set with 20 mealworms (Tenebrio molitor larvae) in 2 L of fresh medium-grade vermiculite an hour before sunset every day, and closed and collected within an hour of sunrise each following morning (Fardell et al., 2021a). Trials comprised three components that ran consecutively across seven nights: (1) “acclimatization” – cameras on, with a peanut butter-honey-oat bait ball crumbled on top of a closed GUD tray, for one night (n = 96/environment); (2) “pre-treatment” – cameras on and stations set with fresh mealworms and vermiculite daily under natural conditions, for three nights (n = 288/environment); and (3) “treatment trials” – set as per pre-treatment but with six treatments added in an orthogonal design that allowed for four independent but randomly placed replicates per treatment, re-set with new materials daily for three nights (n = 48/treatment/environment) (Fardell et al., 2021a). Treatments consisted of: (1) “fox” – integumentary scent of red fox (scented towel that had been placed in red fox bedding for 2 weeks); (2) “cat” – integumentary scent of domestic cat (scented towel placed in domestic cat bedding for 2 weeks); (3) “human disturbance” (12 h of music and spoken-word playback and diffused torch light pointed at the ground); (4) “fox with human disturbance” cues combined; (5) “cat with human disturbance” cues combined; and (6) procedural “control” (non-scented towel, with the addition of an inactive torch and speaker unit) (Fardell et al., 2021a). Foraging behaviors at the GUD stations were filmed using Scoutguard infra-red motion-sensor cameras, set to take continuous 60 s videos, secured to a tree or post ∼20 cm above ground on a ∼10° angle (Fleming et al., 2014) at 150–200 cm from the GUD tray (Fardell et al., 2021a). Predator presence and active times were also observed using cameras. Here, six Reconyx motion sensor cameras, set to take a quick-burst of 10 images across both day and night, were positioned 20–50 m from the perimeter of each study site in each environment. These cameras were fixed to trees 1 m above ground at a 10° downward angle, and had a scent and bait lure of fish oil and a peanut butter-honey-oat mix scattered amongst leaf litter, which was reapplied after 3 days (Fardell et al., 2021a). Responses to abiotic events were controlled by ceasing research during extreme weather events and periods of 70 to 100% full moon (Fardell et al., 2021a), as high illumination moon-phases can affect small mammal foraging behavior (Navarro-Castilla and Barja, 2014).
Videos were scored manually using VLC Media Player playback and a Microsoft Office Excel spreadsheet to identify the species and measure the time spent (in seconds) in different behaviors. All videos were scored by a single observer (LF). An ethogram for scoring behaviors was constructed via observations of 500 random videos, from the total recorded, across all sites (Supplementary Table 1). To test recorder consistency 500 random videos were scored by the single observer on two separate occasions, the results were compared and found to be 98% similar. Consistency was also cross checked every 2 h by re-scoring a random selection of five videos scored in the past 2 h; if the percentage similarity was less than 95% then scoring would not be continued, and the videos that were not similar re-scored at another time. To reduce observer bias, each video was scored randomly under an identification number assigned blindly (by another) to the observer. This way the treatment, location, station number, date and time were unknown to the observer at the time of scoring. These data were added at completion of video scoring to estimate the number of animals that visited each GUD station during an overnight exposure period. If there were consecutive videos that started when the previous video concluded, then these videos were re-watched to determine if they could be the same animal, based on it being in the same location at the start and end of the sequential videos. Consistently observed behaviors, as listed in the ethogram (Supplementary Table 1), that were used to test the study predictions, include: time spent foraging at the GUD tray (at the edge and in the tray combined) – including eating and sniffing through tray materials with full body inside or from a perched position on the edge of the tray, and time spent vigilant – where animal stops a behavior suddenly to actively look and listen with eyes, ears, and nose alert and head and upper body upright or horizontal and stiff. The remaining behaviors of: inspecting bowl, inspecting treatment, cleans self or other, fights other, and rapid exit were less frequently observed across all species, stations and treatments, and thus could not be analyzed reliably. The behavior of foraging outside the tray was not analyzed as it was not directly relevant to the research questions. To observe temporal responses, times active were recorded when scoring all GUD station videos and predator camera station photos. Predator cameras were operational both day and night during survey periods, as were the GUD station video cameras during the acclimatization period only. During GUD surveys, including the pre-treatment conditions and treatment trials, the GUD video cameras were only operational between an hour before sunset and an hour from sunrise.
Statistical Methods
Generalized linear mixed models that allowed for zero inflation and dispersion parameters were constructed with the package glmmTMB (Brooks et al., 2017) in the statistical software R (R Core Team, 2020). This model design comprised three parts. The first was a model of the conditional mean (Brooks et al., 2017), indicating which predictors influence the response values. The second was a model for zero inflation (Brooks et al., 2017), which indicates which predictors increase the probability of a zero result. The third was a dispersion model (Brooks et al., 2017), which allows for a balance of dispersion patterns relative to the mean. The first and third parts are constrained to be positive through log links, and the second part is constrained between zero and one through a logit link (Brooks et al., 2017). The response variables of each model were (1) foraging effort (GUD, i.e., number of mealworms remaining in trays at morning checks), (2) the number of fauna visitors (count per night), (3) the average proportion of time that fauna spent foraging at a GUD tray, and (4) the average proportion of time that fauna spent vigilant on camera. Explanatory variables that showed variance in a response were included in the relative model’s dispersion formula. Explanatory variables that showed the highest deviation in a zero response result were included in the relative model’s zero inflation formula. Fixed explanatory variables included the treatment (seven levels: pre-treatment, control, fox, cat, human disturbance, fox with human disturbance, and cat with human disturbance) and environment (three environments along the urban disturbance gradient: close, mid, and far). For models of the proportion of time spent in a behavior, species was also included as an explanatory variable. The station number (24 per environment), survey night (1–6 per session), and survey session (1–4) were included in all models as random effects to account for repeated measures. An offset term of the number of species observed at each station each night was used in the proportion models to account for the uneven numbers of species across the foraging stations, per night, per environment.
Each model distribution and fit was assessed using observations and tests on the residuals in the package DHARMa (Hartig, 2021). For models based on counts, GUD and number of fauna visitors, a negative binomial 1 distribution was found to fit best. For models based on the average proportions of time spent in a behavior compared to not in the behavior, foraging and vigilant, a beta binomial distribution was the best fit (Kapourani, 2018). Between-pairs analyses were conducted on models to determine significant differences based on estimated marginal means odds ratios, or log odds ratios relevant to the model, using a P-value Tukey adjustment that considered the number of estimates compared in the family, through the package emmeans (Lenth, 2021). Raw data are displayed as boxplots of the explanatory variable effects on each observed response. Model results differ slightly to the box plot representations of the raw response data considering the random, dispersion and zero inflation effects included in the models. Further, data that were visually depicted as outliers in the box plots were included in the models. Overlap coefficients for activity times between each species across the three environments, and between each treatment for a species per environment, were calculated from the predator and GUD camera data and were plotted using the package overlap, which works in radians to fit density curves via trigonometric functions (Ridout and Linkie, 2009).
Results
The most frequently encountered species during the study were the common brushtail possum (Trichosurus vulpecula), northern brown bandicoot (Isoodon macrourus), brown antechinus (Antechinus stuartii), black rat (Rattus rattus), and brown rat (Rattus norvegicus). The latter two rodents were processed together as both are introduced species, and display common behaviors in urbanized areas (Feng and Himsworth, 2014). Nine species of bird were also frequently recorded (see Fardell et al., 2021a). As these birds frequently ate from the GUD trays, they were grouped together here for comparative analyses alongside the small mammals to which they would be potential competitors. Potential stressors that were frequently encountered included people (walking, running, and cycling), and several mesopredators: lace monitor, red fox, domestic cat, and pet dog. Each of the potential prey species and stressors was observed each study session in all environments along the urban disturbance gradient, with a few exceptions: northern brown bandicoots were observed only at the mid environment during the third study session and on only one occasion at the far environment during acclimatization; domestic cats were observed only at the close environment, and lace monitors were observed only on camera at the mid and far environments but were seen opportunistically at the close environment on two occasions (Fardell et al., 2021a). Potential prey species that were less frequently encountered included the common ringtail possum (Pseudocheirus peregrinus) at both the close and far environments, and the short-beaked echidna (Tachyglossus aculeatus) at both the mid and far environments (Fardell et al., 2021a).
The numbers of videos of each species per study session at each environment are detailed in Fardell et al. (2021a). In total 113,979 videos and photos were scored for the purposes of this study, of which the frequently encountered prey species and stressors comprised 106,051. The highest number of videos across the GUD feeding stations was obtained at the far environment, the mid environment videos totalled ∼33% of the far total, and the close environment videos totalled ∼43% of the far total (Fardell et al., 2021a). Human related stressors – people, pet dogs, and domestic cats – were encountered most at the close environment (Table 1). Human disturbance, in the form of paths, facilitated red fox movement in all environments but mostly in the mid environment (Table 1). Lace monitors were observed mostly in the mid environment too, as were common brushtail possums (Table 1). Brown antechinus, brown and black rats, and birds, all returned more video records in the far environment (Table 1). Northern brown bandicoots, however, were most active in the close environment (Table 1).
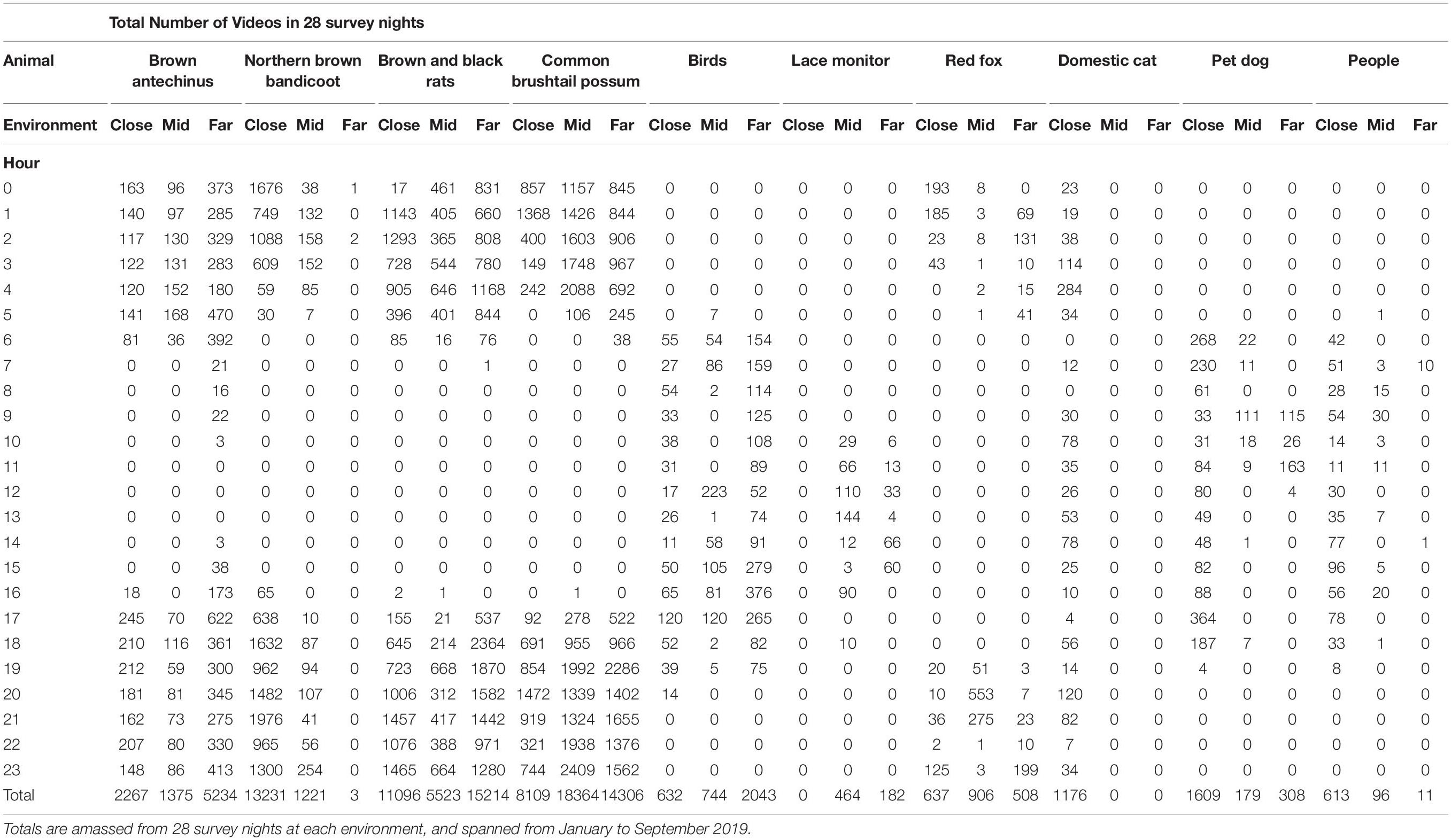
Table 1. Total number of videos and photos for each hour (0–23) for the most consistently observed potential prey species and their potential stressors, at each of the three environments along an urban disturbance gradient (close, mid, and far).
Model Factors: Zero Inflation and Dispersion
Foraging effort (GUD), and numbers of fauna visitors to the GUD trays, differed among the three environments along the disturbance gradient, and under the introduced stressor cues (Figure 1 and Supplementary Tables 2, 3). For both models, there was no significant influence of any treatment or environment on the probability of a zero GUD value (Supplementary Tables 2, 3). There was, however, significant dispersion across: survey sessions 1, 2, and 3 for both models, and between all three environments for the GUD model and the close and far environments for the number of visitors model (Supplementary Tables 2, 3).
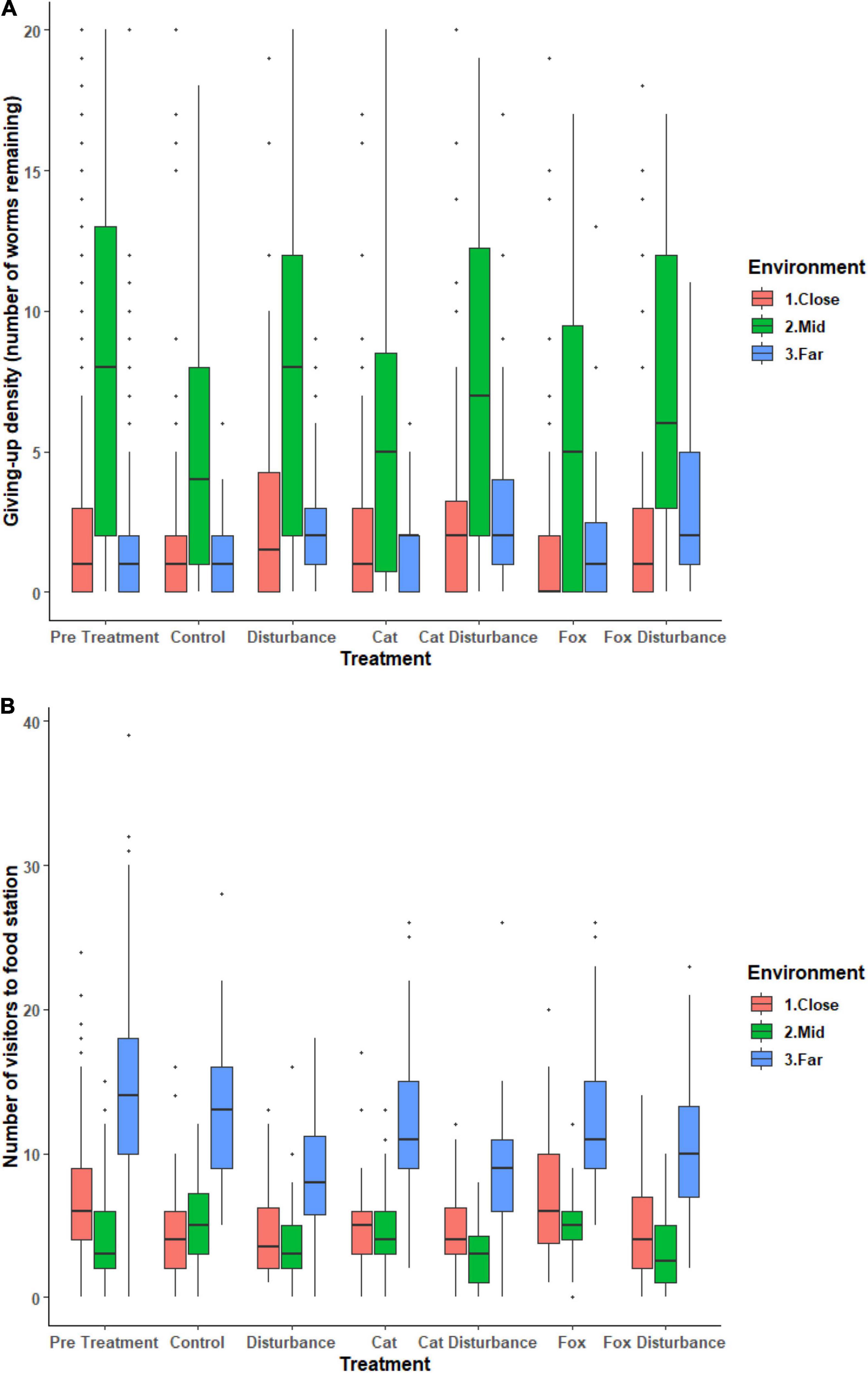
Figure 1. Boxplots of raw data for the (A) GUD (giving-up density: number of mealworms remaining after 12 h overnight exposure), and (B) number of fauna visitors to a giving-up density station for each environment and treatment. Vertical lines represent the standard error. Horizontal lines represent the mean. Dots represent measurements that were not consistently recorded but were retained in the models.
The average proportions of time that animals spent foraging at GUD stations and in vigilance also differed among the three environments along the disturbance gradient, among species, or under the different stressors (Figure 2 and Supplementary Tables 4, 5). For the time spent foraging model, it was significantly less probable for northern brown bandicoots, and brown and black rats, and more probable for common brushtail possums, to have spent zero time foraging in a GUD station when on camera (Supplementary Table 4). Further, there was significant dispersion across all survey sessions, under the control, pre-treatment, cat, and cat with human disturbance treatments, in all three environments, and for all species (Supplementary Table 4). For the time spent in vigilance model, it was significantly less probable for zero time to be spent in vigilant behavior under the pre-treatment conditions, or in the close environment (Supplementary Table 5). Further, there was significant dispersion across all survey sessions, all treatments except for the pre-treatment and cat treatment, at all environments, and for brown antechinus, birds, and common brushtail possums (Supplementary Table 5).
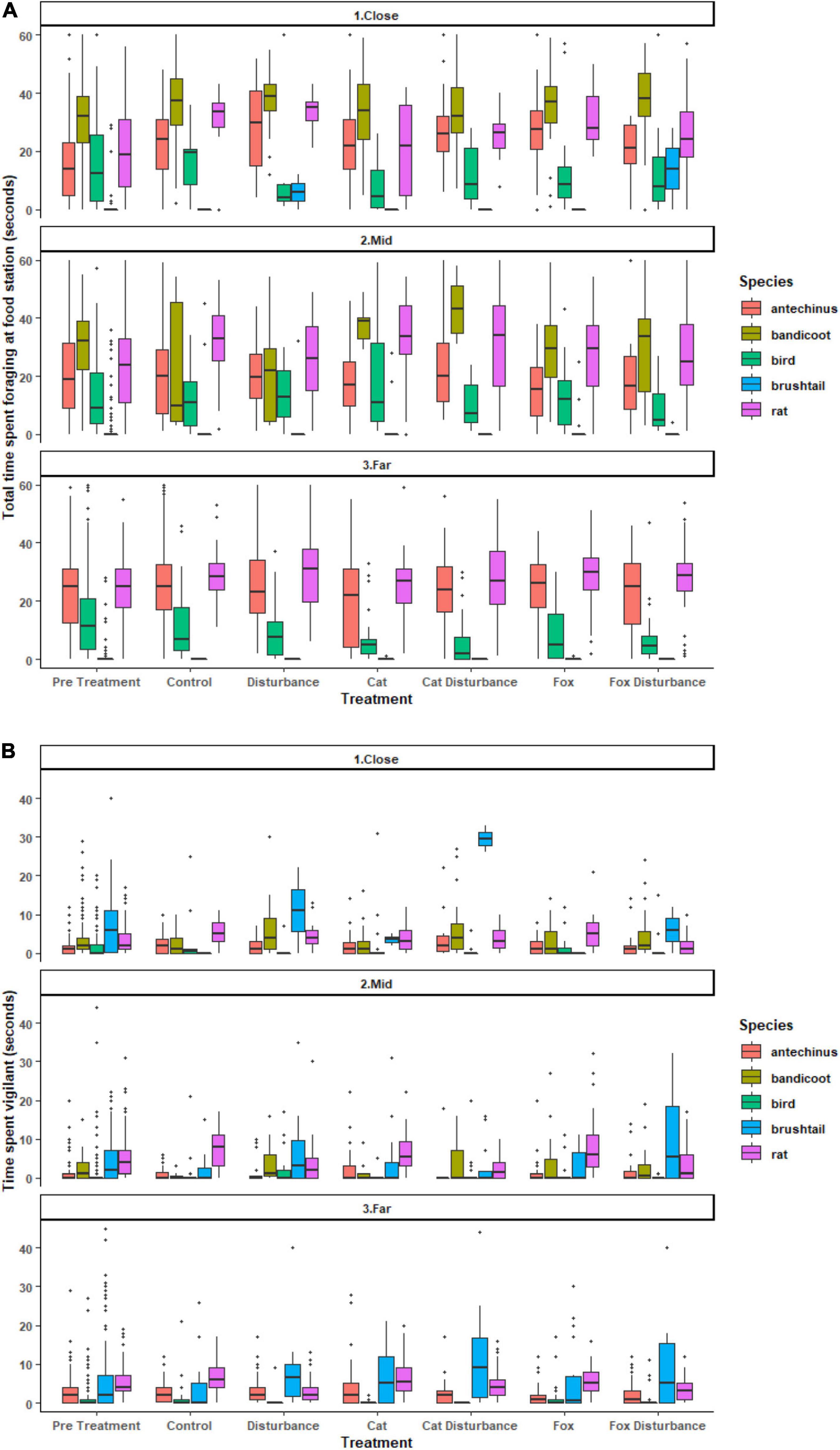
Figure 2. Boxplots of raw data for the (A) average amount of time (seconds) spent foraging at a giving-up density station, and (B) average amount of time (seconds) spent in vigilant behavior on camera, for each species by treatment and environment. Vertical lines represent the standard error. Horizontal lines represent the mean. Dots represent measurements that were not consistently recorded but were retained in the models.
Introduced Stressor Responses
Foraging Effort Giving-Up Density
Significant differences in the generalized linear mixed models considering GUDs by treatment and location are shown in Supplementary Table 2, and in the according pairwise estimated marginal means comparisons in Table 2. In all three environments there were similarities in the patterns of difference in foraging efforts among treatments. In general, GUDs were lower under the control compared to the pre-treatment, and lower under the control and predator-only treatments compared to those that contained human disturbance cues both singularly and when combined with a predator cue (Figure 1 and Table 2). The highest and lowest GUDs were observed under different treatments along the disturbance gradient, being: at the close, pre-treatment (high) and fox (low); at the mid, human disturbance and pre-treatment (highs) and control (low); and at the far, cat with human disturbance (high) and control (low) (Figure 1 and Table 2).
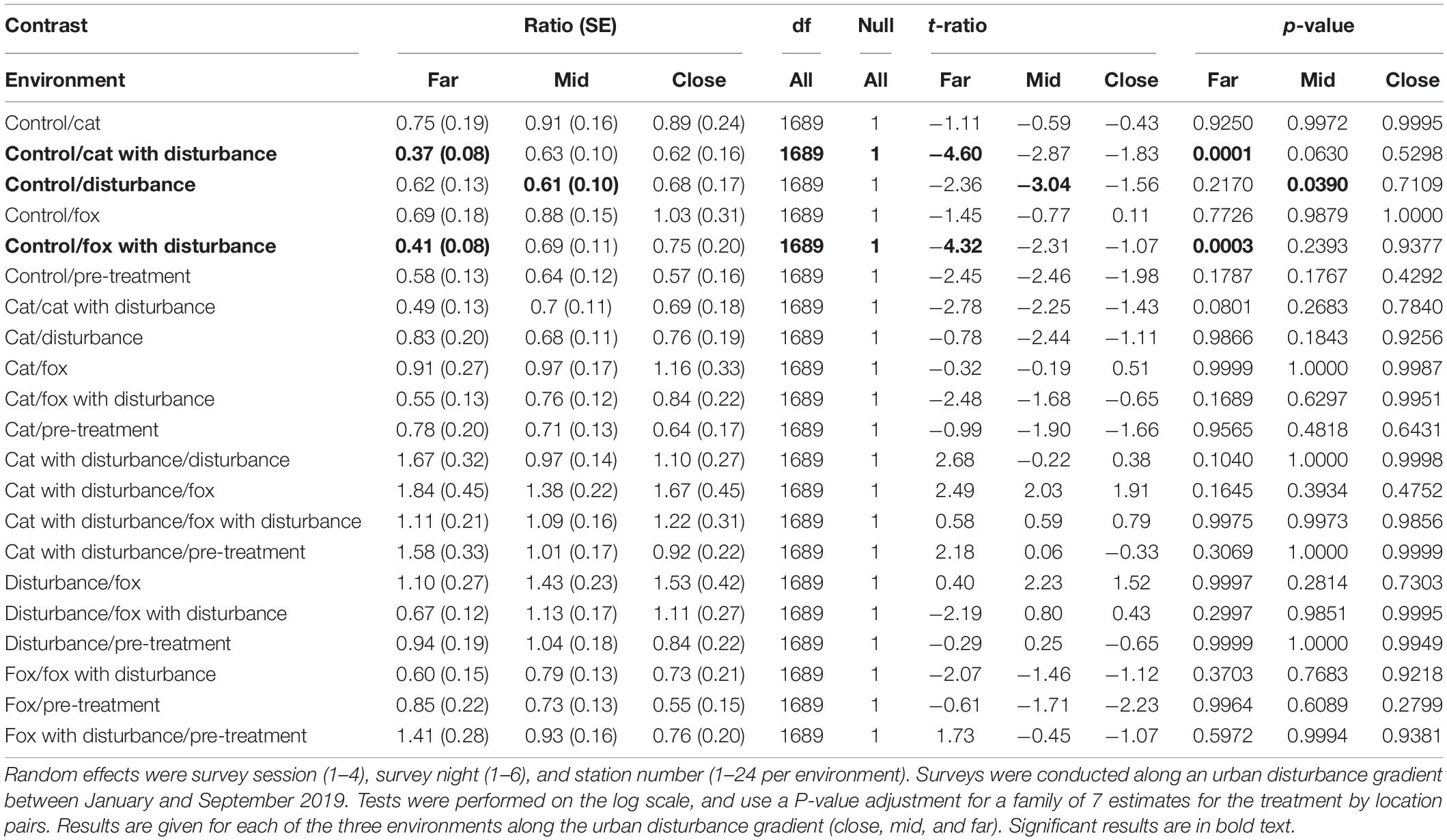
Table 2. Between treatment pairwise contrast ratios for estimated marginal means from generalized linear mixed models with zero inflation and dispersion parameters for giving-up density (GUD) responses, under the explanatory variables of treatment and environment.
The Number of Fauna Visitors
Significant differences in the generalized linear mixed models considering the number of fauna visitors to GUD stations by treatment and location are shown in Supplementary Table 3, and in the according pairwise estimated marginal means comparisons in Table 3. In all three environments, there was an observable trend of more visitors to the GUD stations under the control and single predator treatments compared to all treatments that contained human disturbance cues, although the difference was reduced in the close environment (Figure 1 and Table 3). The highest and lowest numbers of visitors were observed under different treatments along the disturbance gradient, being: at the close, pre-treatment and fox (highs) and control and human disturbance (lows); at the mid, control (high) and fox with human disturbance (low); and at the far, pre-treatment (high) and cat with human disturbance (low) (Figure 1 and Table 4).
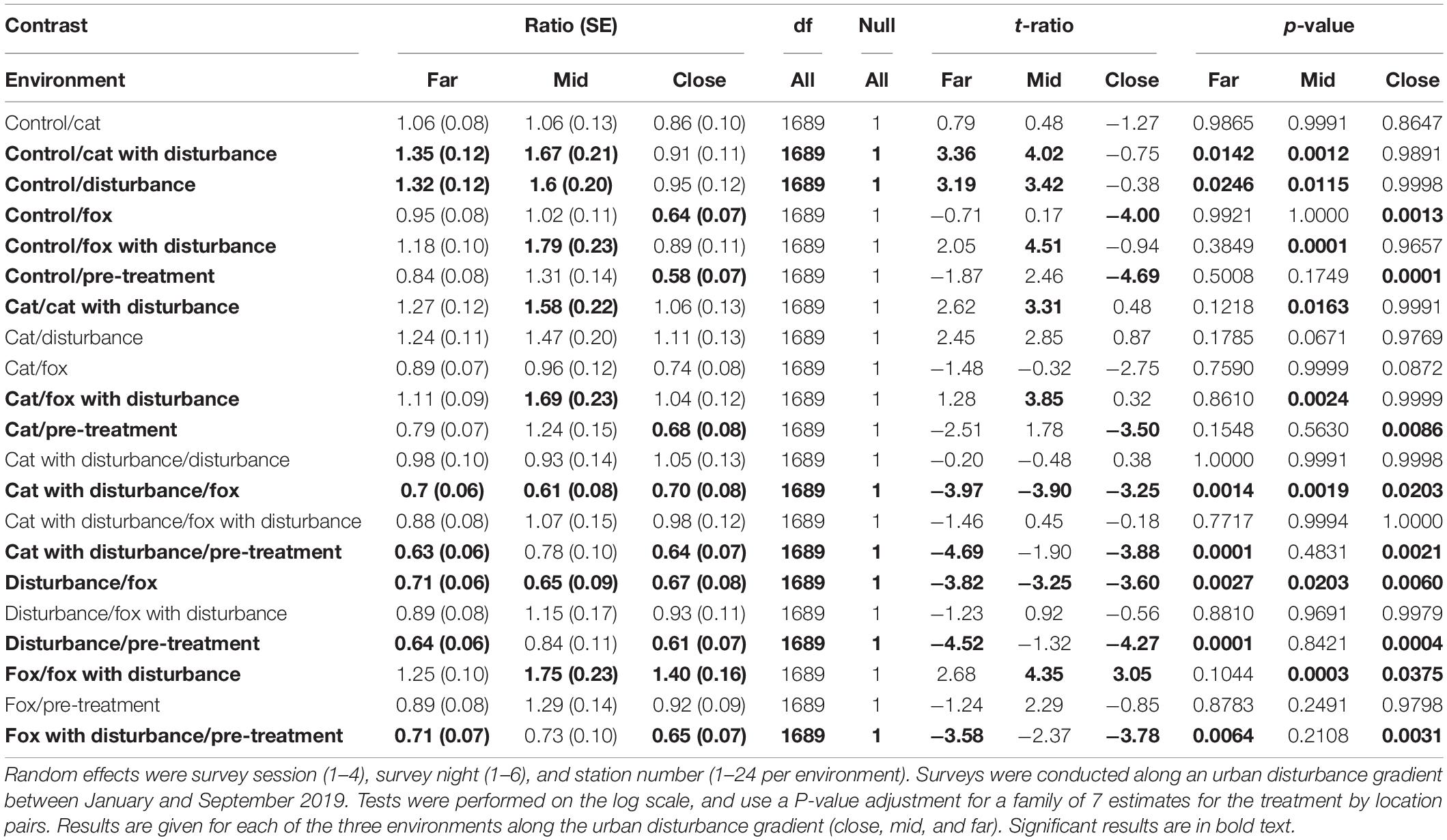
Table 3. Between treatment pairwise contrast ratios for estimated marginal means from generalized linear mixed models with zero inflation and dispersion parameters for number of fauna visitors to giving-up density (GUD) stations, under the explanatory variables of treatment and environment.
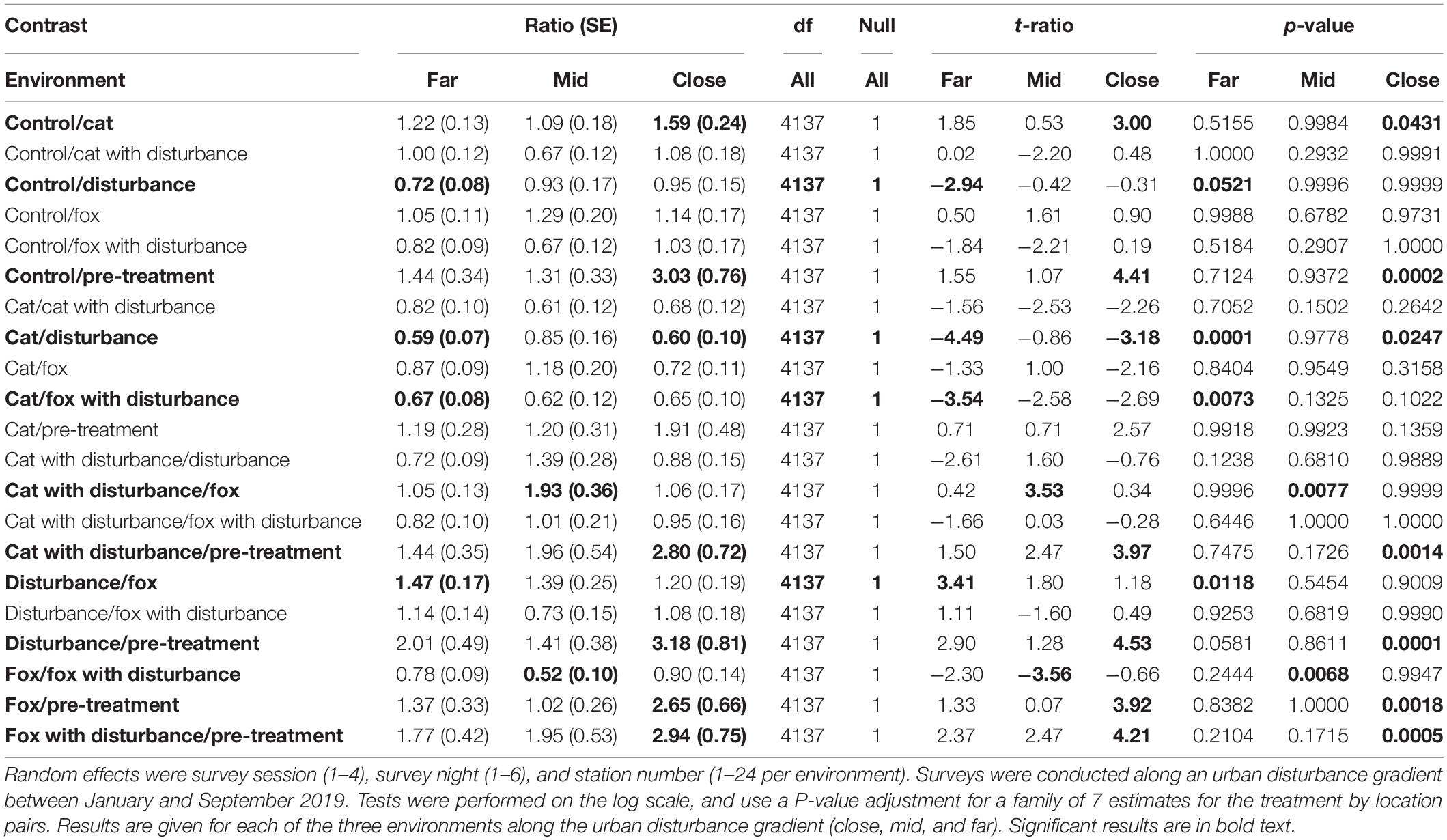
Table 4. Between treatment pairwise contrast ratios for estimated marginal means from generalized linear mixed models with zero inflation and dispersion parameters for the proportion of time spent foraging response, under the explanatory variables of treatment and environment.
The Time That Fauna Visitors Spent Foraging at Food Stations
Significant differences in the generalized linear mixed models considering the proportion of time that fauna visitors spent foraging at GUD stations by treatment and location are shown in Supplementary Table 4, and in the according pairwise estimated marginal means comparisons in Table 4. In all three environments, animals tended to spend less time foraging at the GUD stations under the control and single predator treatments compared to all treatments that contained human disturbance cues (Figure 2 and Table 4). The highest and lowest amounts of time spent foraging were observed under different treatments across the disturbance gradient, being: at the close, human disturbance (high) and pre-treatment (low); at the mid, cat/fox with human disturbance (highs) and pre-treatment and fox (lows); and at the far, human disturbance (high) and pre-treatment (low) (Figure 2 and Table 4).
The Time That Fauna Visitors Spent Vigilant
Significant differences in the generalized linear mixed models considering the proportion of time that fauna visitors spent in vigilance on camera by treatment and location are shown in Supplementary Table 5, and in the according pairwise estimated marginal means comparisons in Table 5. At both the mid and far environments, there was a trend of more time spent in vigilance under the control and single predator treatments compared to all treatments that contained human disturbance cues (Figure 2 and Table 5). The opposite was evident in the close environment, where less time was spent vigilant under the control and single predator treatments compared to all treatments that contained human disturbance cues (Figure 2 and Table 5). The highest and lowest amounts of time spent vigilant occurred under different treatments along the disturbance gradient, being: at the close, cat with human disturbance (high) and pre-treatment (low); at the mid, fox (high) and pre-treatment (low); and at the far, cat (high) and human disturbance and fox with human disturbance (lows) (Figure 2 and Table 5).
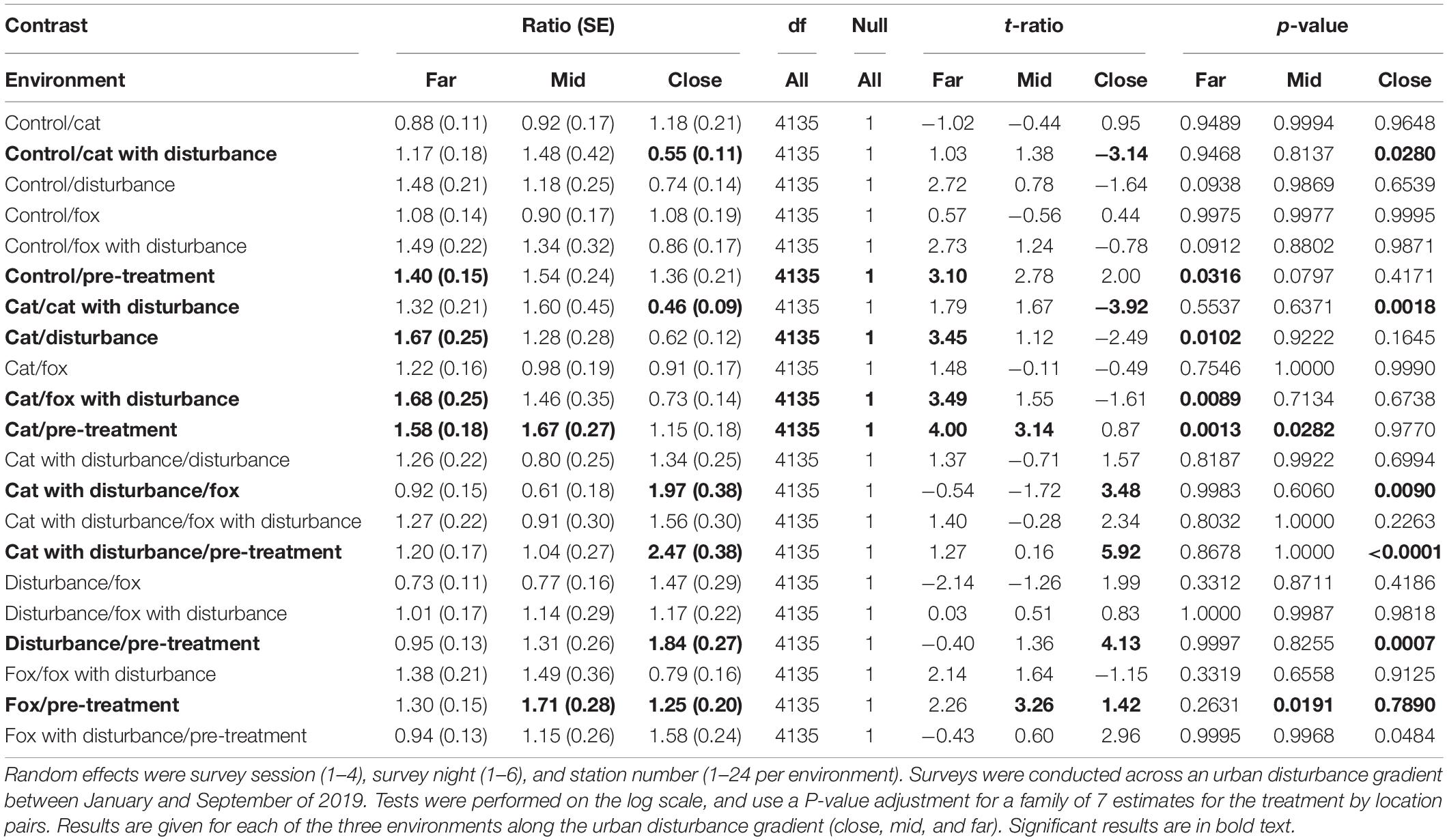
Table 5. Between treatment pairwise contrast ratios for estimated marginal means from generalized linear mixed models with zero inflation and dispersion parameters for the proportion of time spent vigilant, under the explanatory variables of treatment and environment.
Responses Along the Urban Disturbance Gradient
Along the disturbance gradient, GUD results were significantly highest at the mid environment under each of the treatments, and also lower at the far environment compared to the close environment for all treatments, except under the fox with human disturbance treatment where it was higher (Figure 1 and Table 6). The number of visitors was significantly highest at the far environment under all treatments, and at the close environment there were more visitors than at the mid environment under all treatments except cat and the control (Figure 1 and Table 6).
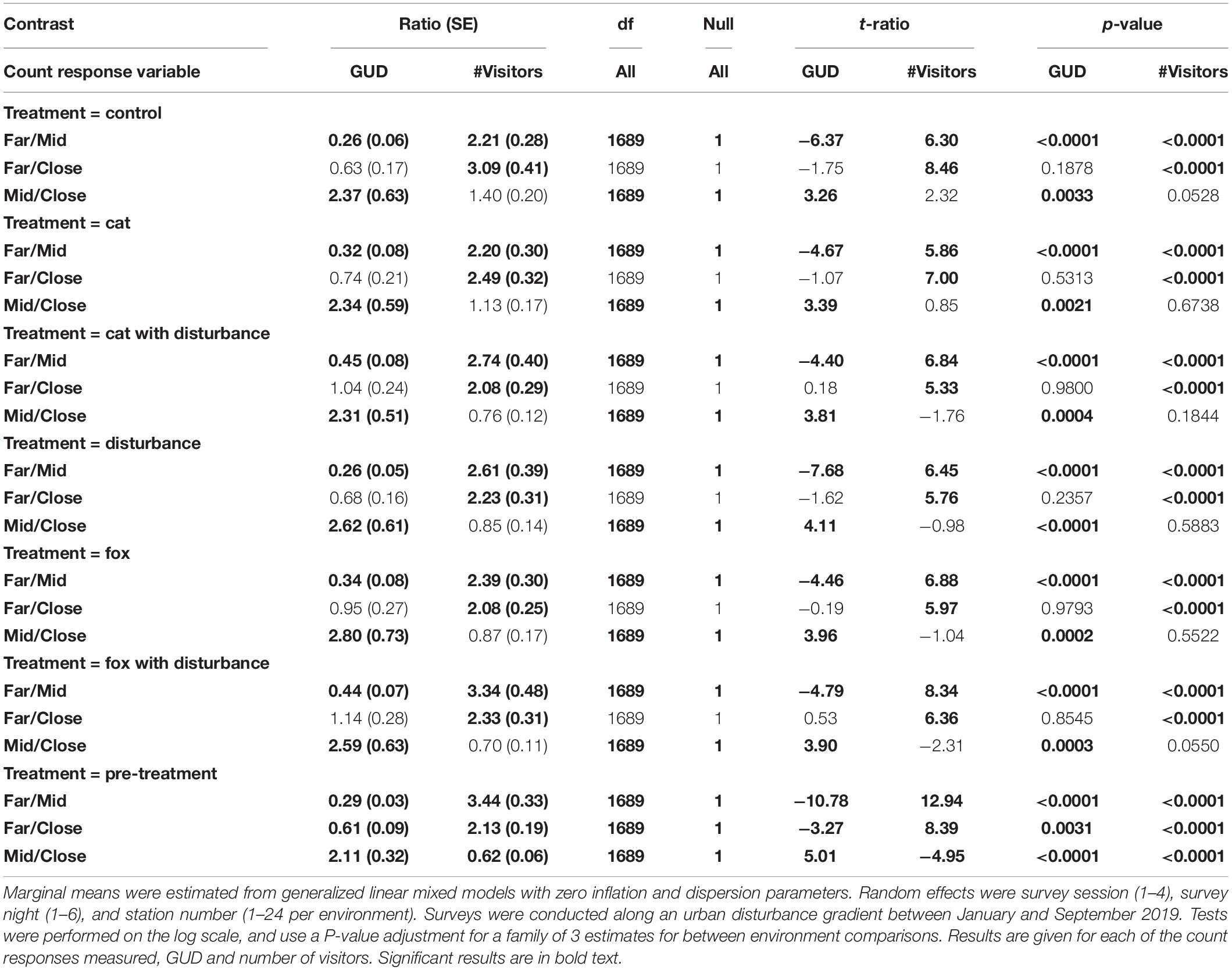
Table 6. Pairwise contrast ratios for estimated marginal means comparing environments (close, mid, and far) along an urban disturbance gradient for giving-up density (GUD) responses and number of visitor responses, under the explanatory variables of treatment and environment.
Significantly more time was spent foraging at the GUD stations, under the pre-treatment conditions at each of the far and mid environments, than at the close environment (Table 7). Additionally, under the cat with human disturbance treatment, animals spent less time foraging at the GUD stations at the far environment than at the mid environment (Table 7). Generally, less time was spent foraging under cat and cat/fox with human disturbance at the far environment compared to the other two, less time was spent foraging at the mid environment under human disturbance and fox than at the close environment, and more time was spent foraging at the close environment under fox compared to the other two environments (Table 7). Significantly less time was spent in vigilance at the close environment under the pre-treatment compared to the far environment, under the cat treatment compared to both environments, and under the fox treatment compared to the mid environment (Table 7). Comparatively, fauna visitors spent more time vigilant at the close environment under the treatments of human disturbance compared to the far environment, and the cat with human disturbance treatment compared to both environments (Table 7). Generally, more time was spent vigilant at the mid and far environments under the control and predator only treatments compared to the close environment, where comparatively more time was spent vigilant under all treatments that contained human disturbance (Table 7). Between the mid and far environments, more time was spent vigilant at the far environment under treatments with cat cues, and at the mid environment under treatments with fox cues (Table 7).
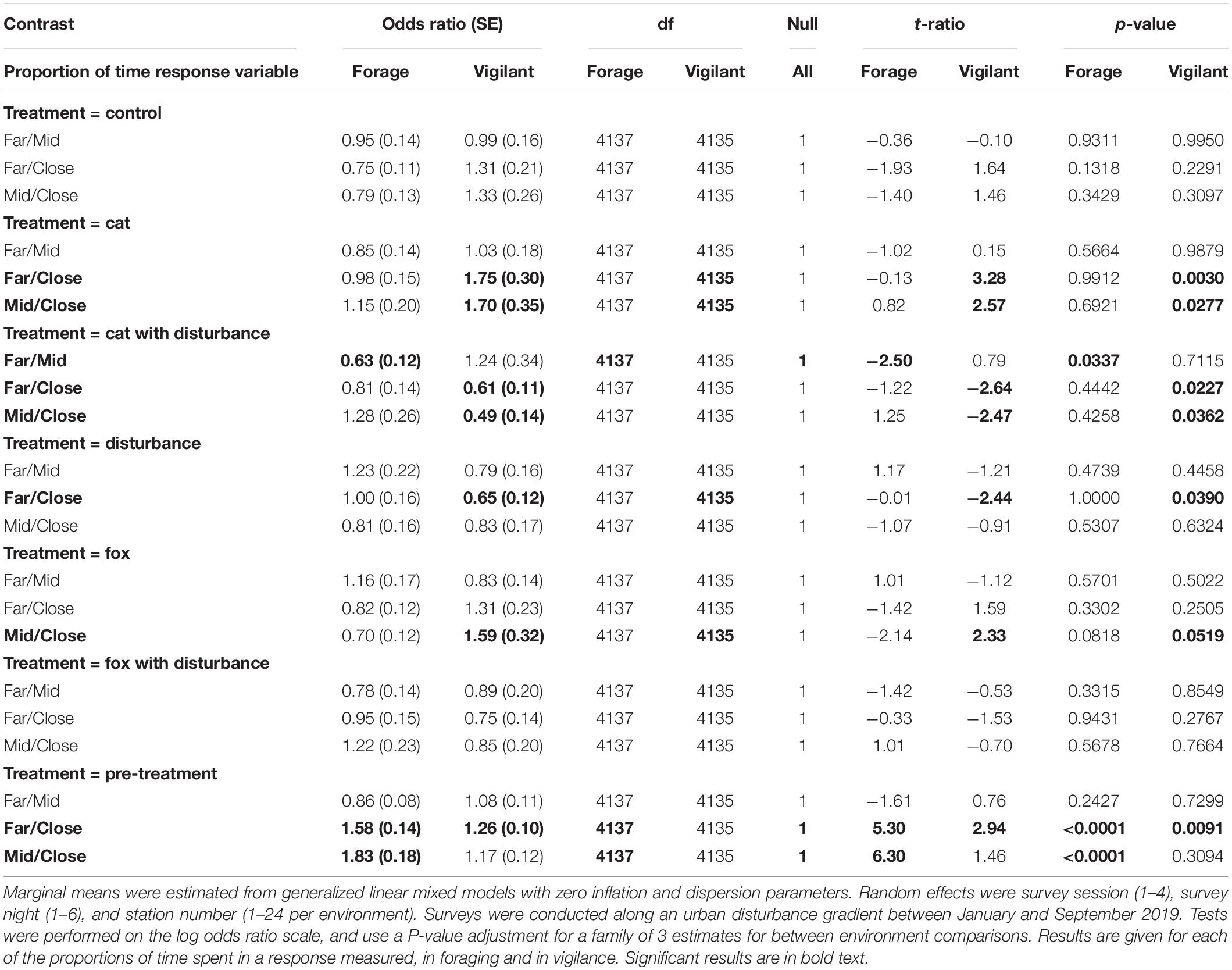
Table 7. Pairwise contrast ratios for estimated marginal means comparing environments (close, mid, and far) along an urban disturbance gradient for animal responses of time spent foraging and time spent vigilant, under the explanatory variables of treatment and environment.
Responses Among Small Animal Species
From pairwise analyses between the more frequently observed species among the combined treatments and environments, northern brown bandicoots spent the most time foraging, followed by the combined brown and black rats, then brown antechinus, birds, and finally common brushtail possums (Figure 2 and Table 8). Differences in the time that species spent foraging under the different treatments reduced along the disturbance gradient with increased distance from houses (Figure 2). Common brushtail possums spent most time being vigilant, followed by brown and black rats, then northern brown bandicoots, brown antechinus, and finally birds (Figure 2 and Table 8). Species’ vigilance patterns were similar along the disturbance gradient in response to the treatments in all three environments (Figure 2).
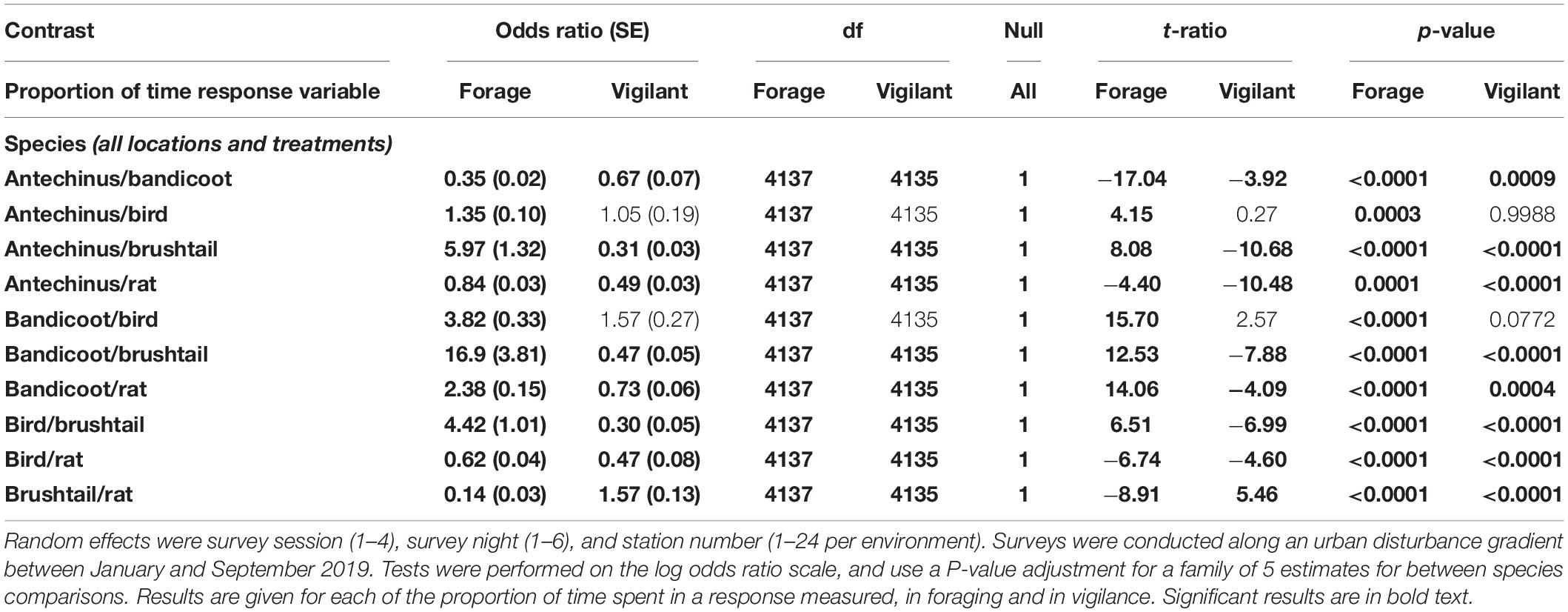
Table 8. Between species pairwise contrast ratios for estimated marginal means from generalized linear mixed models with zero inflation and dispersion parameters for each response of time spent foraging and time spent vigilant, under the explanatory variables of treatment and environment.
Temporal Activity Responses
Activity periods for small prey animals largely overlapped in each environment and under the different treatment conditions compared to the control (Figure 3 and Supplementary Table 6). The lowest overlap coefficients were observed in the mid environment, between the control and: fox with human disturbance for the brown antechinus; the pre-treatment, cat, cat with human disturbance, and fox, for the northern brown bandicoot; and the pre-treatment, cat, fox, and fox with disturbance for the common brushtail possum. The patterns of each were observed more closely using pairwise overlap plots (Figure 4).
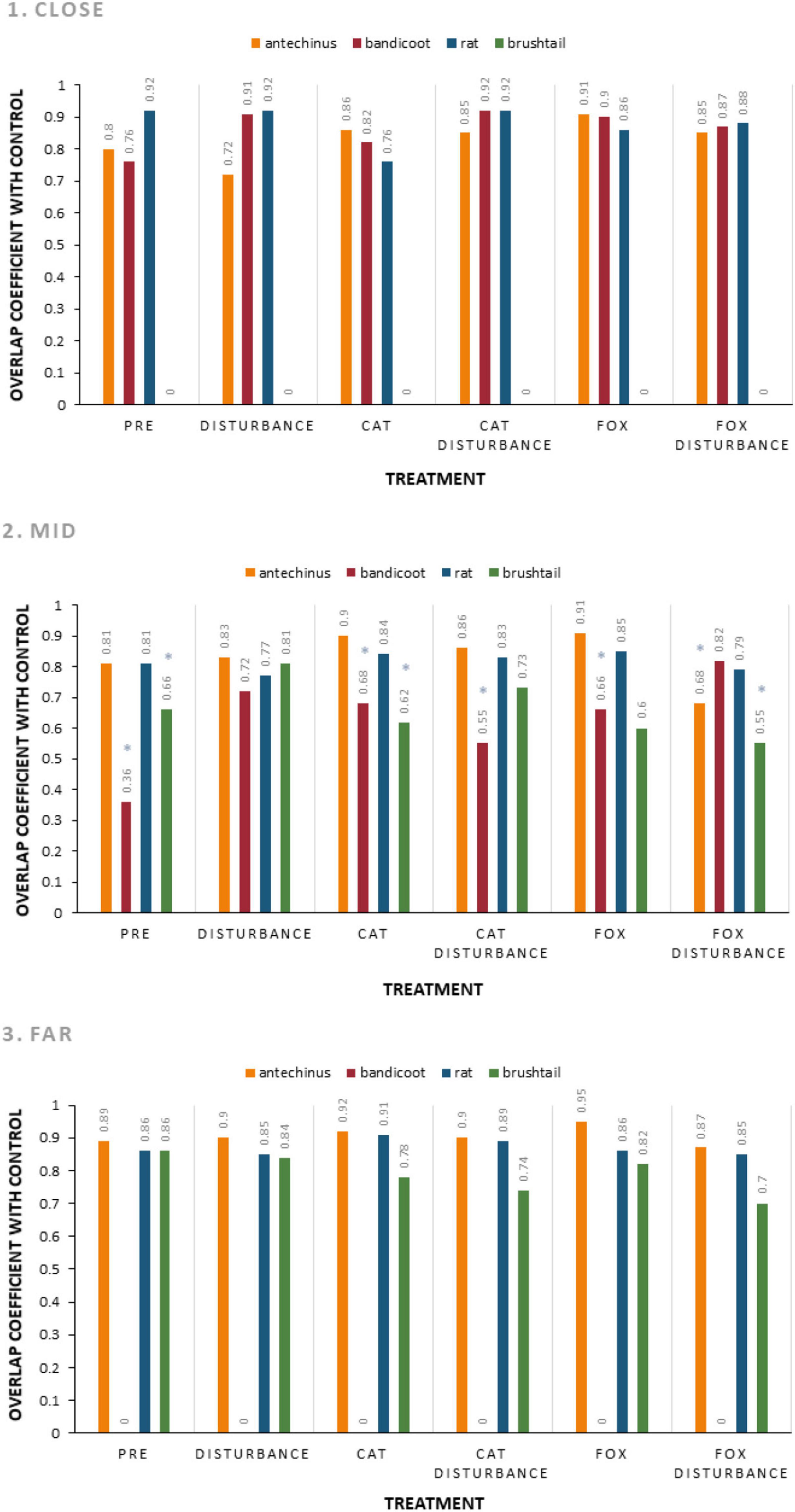
Figure 3. Overlap coefficient comparing each treatment to the control, for the hours active at giving-up density stations (baited with live mealworms) for each frequently observed small mammal species (brown antechinus, northern brown bandicoot, brown and black rats combined, and common brushtail possum) along the three urban disturbance gradient environments (1. Close, 2. Mid, and 3. Far). For each species, periods active under the procedural control are compared to those under each of the six treatment conditions (pre-treatment: three nights of trials under natural conditions before the treatment trials, disturbance: human disturbance cues of continual sound and light, cat: domestic cat cues of cat integumentary scent captured on towels placed in their bedding, cat with human disturbance: the combination of these stressors, fox: red fox cues of fox integumentary scent captured on towels placed in their bedding, fox with human disturbance: the combination of these stressors). Results with “*” are considered low and are investigated further in Figure 4.
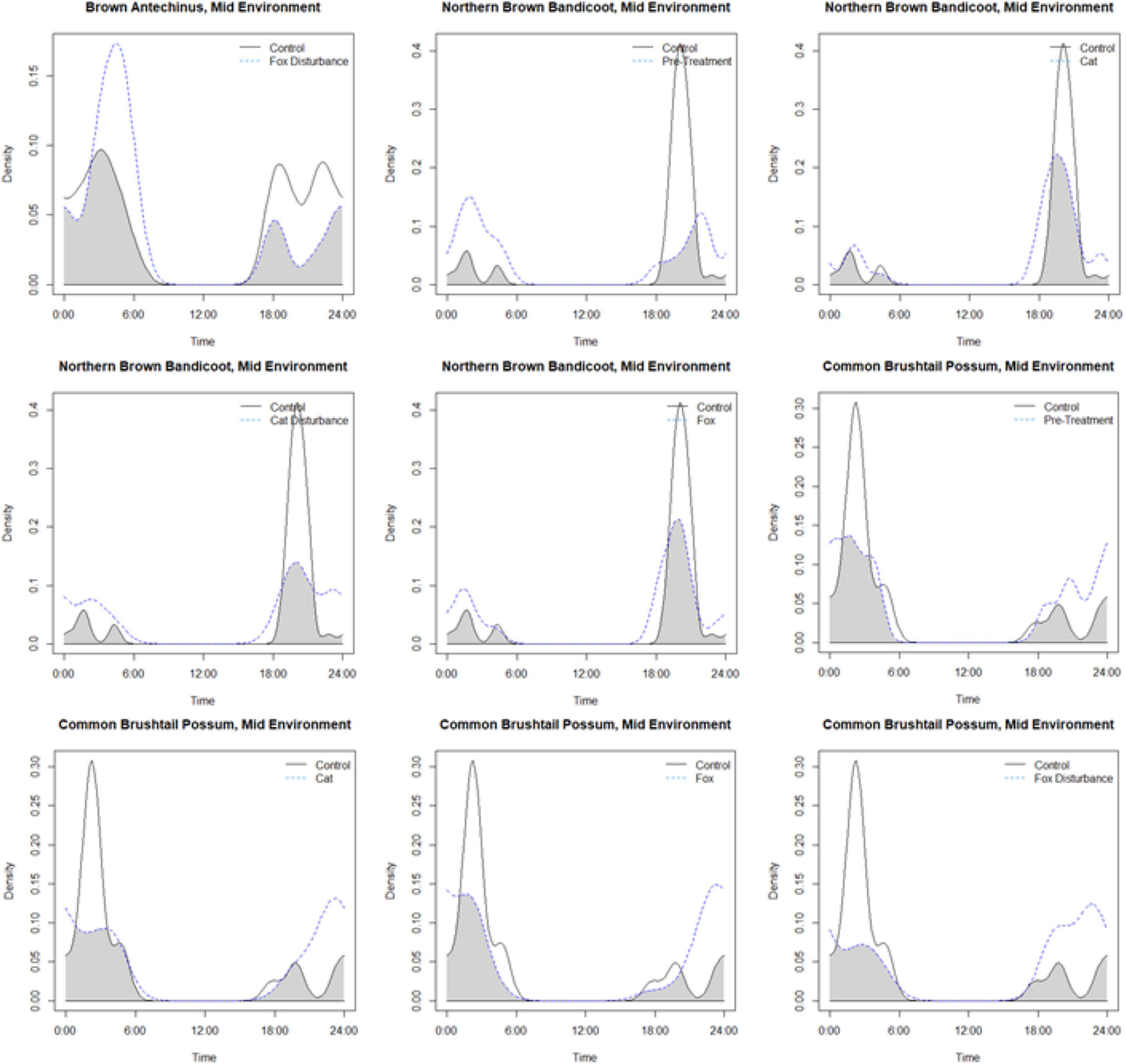
Figure 4. Density pair plots for the lowest observed overlap coefficients for hours active by small mammals at giving-up density stations (baited with live mealworms), for species across the treatments compared to the procedural control (pre-treatment: three nights of trials under natural conditions before the treatment trials, disturbance: human disturbance cues of continual sound and light, cat: domestic cat cues of cat integumentary scent captured on towels placed in their bedding, cat with human disturbance: the combination of these stressors, fox: red fox cues of fox integumentary scent captured on towels placed in their bedding, fox with human disturbance: the combination of these stressors). All of the lowest overlap calculations were observed at the mid environment on an urban disturbance gradient (of close, mid, and far).
Activity periods largely overlapped for the nocturnally active small mammal species, with overlaps generally highest at the close environment (Figure 5 and Supplementary Table 7). These species also overlapped with the red fox, especially in the far environment (Figure 5 and Supplementary Table 7). The highest overlaps in times active for each stressor (i.e., domestic cats, dogs, and people) with small mammals occurred in the close environment (Figure 5). Lace monitors overlapped little in activity with the mammals, slightly more so in the mid than in the other environments (Figure 5). Birds showed the highest overlap in activity with predators and people at the close environment (Figure 5). Common brushtail possums, and brown and black rats, had the highest observed frequency of activity peaks across the nocturnal period in all three environments, as did northern brown bandicoots at the close environment (Figure 5).
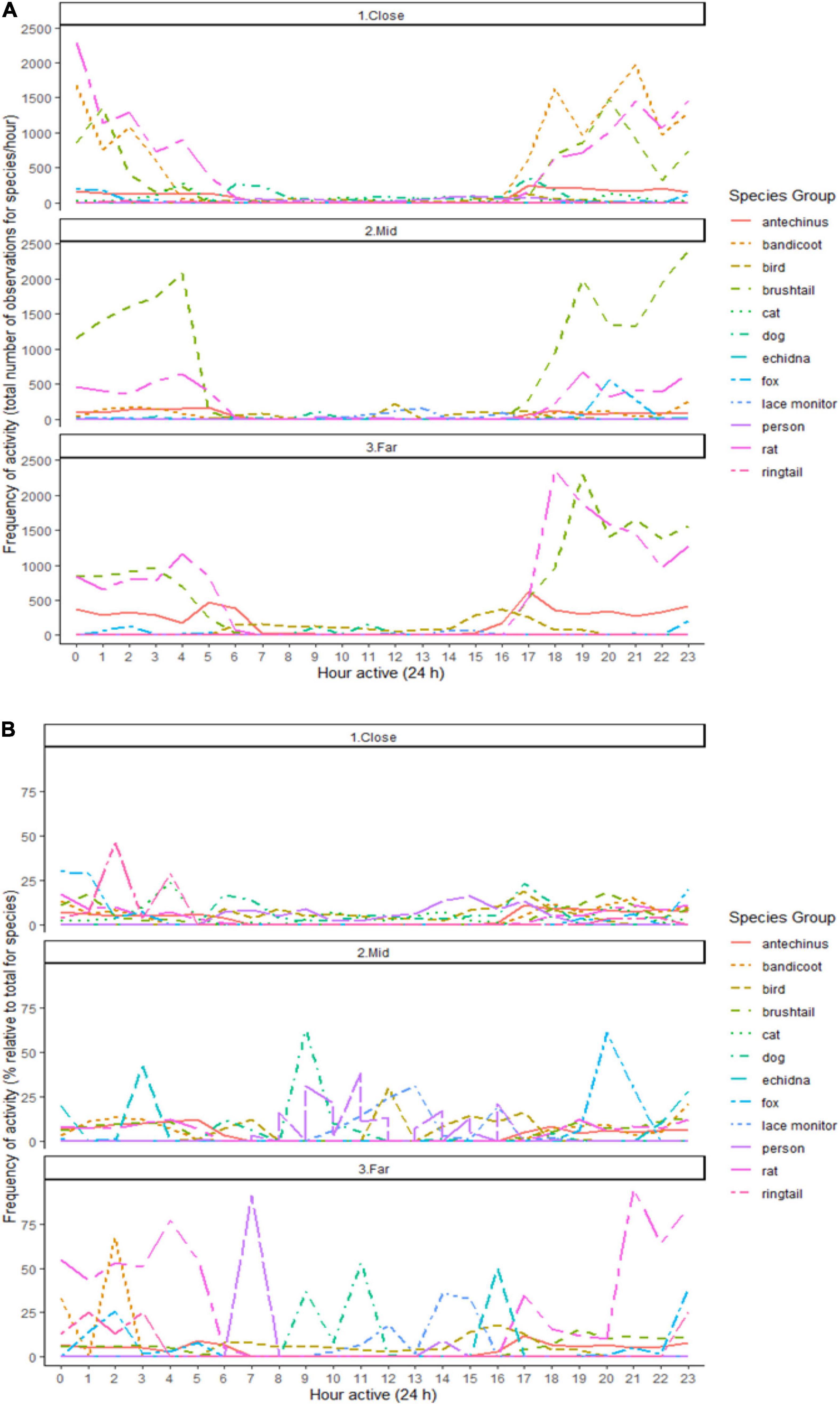
Figure 5. The frequency of activity across 24 h by the (A) total number of observations, and (B) percentage relative to the total number of observations, for each observed species (brown antechinus, northern brown bandicoot, birds, common brushtail possum, domestic cat, pet dogs, short-beaked echidna, red fox, lace monitor, person, brown and black rats combined, common ringtail possum) along an urban disturbance gradient with three environments (close, mid, and far), under the combined treatment conditions, at both giving-up density stations and baited predator cameras.
Discussion
Conservation management of wildlife in urban ecosystems (Seto et al., 2012; Wintle et al., 2019) requires an improved understanding of the coping mechanisms that wildlife employ in response to the attendant stressors (McPhearson et al., 2016; Fardell et al., 2020). Our results provide insight into how small prey animals, particularly small mammals and to a lesser degree birds, cope behaviorally with the urban stressors of novel predator activity and human disturbance along an urban disturbance gradient. We found some support for each of our four predictions. Urban stressors elicited different responses in foraging activity and behaviors, with those simulating human disturbance and particularly in combination with a predator cue showing the most obvious pattern in level of coping response. Notably though, the type and level of responses differed across the gradient. At the close environment, human disturbance elicited the strongest responses, but the highest vigilance response was in combination with cat odor. By comparison, in the mid environment, fox odor and/or human disturbance elicited the strongest responses, while in the far environment responses to cat odor and/or human disturbance were most evident. Under conditions with these stressors, generally more time was spent in vigilance and in foraging, with fewer visits made to GUD stations and higher GUDs resulting. Interestingly, at the close environment, the fox treatment was perceived as low risk for foraging. Different coping mechanisms were evident along the disturbance gradient, with behavioral adjustments at the close environment, minor temporal activity adjustments at the mid environment, and shifts in habitat component use at the far environment (Fardell et al., 2021a). Species foraging responses appeared to be relative to size, with larger animals spending more time vigilant than smaller species, and relative to history, movement, and morphology for time spent in vigilance. Temporal activity patterns largely coincided, with the greatest overlap between mammals and their stressors observed at the close environment. There were no large shifts in the hours active for the small prey animals, but there were some small differences in levels of temporal activity across the night in response to the stressors in the mid environment for small mammals.
Introduced Stressor Responses
Responses by small prey animals differed between the treatment types, suggesting a level of recognition of the domestic cat and red fox predator cues in all three environments. Our GUD results correlated with those reported in Fardell et al. (2021a), where GUD data were analyzed relative to habitat component use and distances to human disturbances and predators. However, some trends were more evident here when modeling the GUD directly against the treatments and between locations. At all three environments, the pre-treatment conditions resulted in generally high GUDs with animals spending the least amount of time foraging. At both the close and mid environments, these were the highest overall GUDs with the least amount of time spent vigilant per visit. Interestingly though, at the close environment, the number of fauna visitors was generally higher under the pre-treatment and fox treatment conditions. These results potentially reflect that when compared to the natural (pre-treatment) conditions, the introduced treatment stressors caused an ambient level of higher stress across the study area, which due to responsive hormone releases increased food consumption (refer McEwen and Wingfield, 2003). As urban wildlife has been observed to have lower or equal glucocorticoid levels compared to their rural counterparts (French et al., 2008; Łopucki et al., 2019; Iglesias-Carrasco et al., 2020), our results may be driven by a higher level of stressors than the wildlife across the disturbance gradient is accustomed to. This indicates that in response to increased stressors, there would be a period of chronic stress impacts. Further, the close environment results may offer some support for the theory that animals exposed to chronic stressors from birth develop foraging patterns of increased transitions between patches as part of threat mitigation (Chaby et al., 2015). At the far environment, the pre-treatment conditions contrastingly had the highest number of visitors. Likely, though, at the far environment the animals were less accustomed to introduced stressors and resources, and consequently took longer to acclimatize to the GUD trays. In this scenario animals conceivably moved back and forth between patches more often and for a reduced time each visit. We expect that the pattern in the far environment was driven by the higher density of brown and black rats, as both species are particularly neophobic (Barnett, 1958), with this wariness manifesting in shuttling foraging behavior (Ovadia et al., 2001).
In general, GUDs were lowest under the control compared to the pre-treatment and treatment conditions. The exception related to the fox treatment at the close environment. According to optimal patch use theory (Brown, 1988, 1999), the control and the fox treatment in the close environment would be perceived by foragers as low risk. Across all environments, under all treatments that contained human disturbance cues alone or combined with a predator cue, there was a general pattern of higher GUDs, fewer visits to each GUD station but greater time spent foraging per visit than under the control and predator-cue treatments alone. This pattern could indicate bolder individuals taking advantage of the available food, as has been observed, similarly, in gecko species exposed to urban disturbances (Short and Petren, 2008). Similarly, in the close environment there were fewer visitors that spent more time foraging under the control treatment, although this resulted in lower GUDs, suggesting more efficient foraging by animals in this instance when not in direct proximity to an introduced stressor.
Treatments containing human disturbance elicited the clearest coping responses at the close environment but, notably, these responses were greatly reduced in the presence of fox scent. Coping responses to mitigate the effects of human disturbance were most obvious near urban build-up, suggesting a level of familiarization and threat response development. Urban populations of birds have been found to habituate to human disturbances faster than their rural counterparts (Vincze et al., 2016). Similarly, squirrel responses to human cues are reduced at urban compared to less urban environments (Kittendorf and Dantzer, 2021). Notably though, in our study, we recorded increased vigilance to human disturbance at the close environment, which contrasts with other studies (e.g., Møller et al., 2015; Uchida et al., 2019). We propose that human disturbance was still perceived as risky despite the potentially longer and stronger exposure of animals to it. Our results support our previous finding that predator and human disturbance stressors are additive and produce the most developed and obvious responses in wildlife (Fardell et al., 2021a).
Under human disturbance, and more so under the combined treatments of human disturbance with a predator cue, less time was spent in vigilance per visit at the mid and far environments, while more time was spent vigilant at the close environment. Urban squirrels have also been found to increase vigilance when human and predator cues are combined compared to when these cues occur singly (Kittendorf and Dantzer, 2021). This response may be due to animals at the mid and far environments having experienced comparatively less exposure to human disturbance. Where this occurs, disturbance then is perceived as risky and induces a higher level of stress such that vigilance is no longer perceived to be beneficial (Brown, 1999; Kotler and Brown, 2017). The higher GUD results support this idea. At the close environment, mammals are likely to be more habituated to the stressors of human disturbance (Lowry et al., 2013; Møller et al., 2015; Uchida et al., 2019), and this is reflected in the lower GUD results under the combined treatments compared to the other environments. As such, vigilance was evidently beneficial to small mammals at the close environment under the treatments containing human disturbance cues, particularly as their auditory senses may have been dulled by urban noise, and olfactory predator cue treatments were pronounced.
The most obvious coping responses to the fox (predator cue) treatment were observed at the mid environment, where animals spent much time vigilant and least time foraging. Notably though, these responses increased under the combined fox with human disturbance treatment. The mid environment was likely subject to high red fox activity, as indicated by the large numbers of videos/photos and was due perhaps to a path that extended along the border of the mid environment from the road and houses to the conservation area. Red foxes frequently use anthropogenic paths (Towerton et al., 2016), and the narrow mid environment habitat next to the path would have facilitated opportunistic encounters based on red fox olfactory detection of the small mammals (Hughes et al., 2010). Interestingly though, at the close environment, fox cues were perceived to be low risk, and small prey animals at this treatment made many visits and foraged down to low GUDs. Considering the strong reaction of small prey animals to human disturbance cues, red foxes may be seen as a “shield” from human activity, with their presence indicating safe distances and times from people. This interpretation is supported by our previous study (Fardell et al., 2021a), where closer distances to predators were perceived to provide less risky foraging conditions and proximity to houses was perceived as highly risky. Red foxes are often commensal with people but stay at a safe distance that reduces direct interactions (Díaz-Ruiz et al., 2016; Moll et al., 2018). This “near-wariness” allows foxes to exploit supplementary food resources, which may in turn reduce predation pressure on local wildlife (Reshamwala et al., 2018). At the far environment, however, the fox treatment cue was perceived as less of a threat than the corresponding cat treatment cue.
The cat (predator cue) treatment appeared to have the strongest effect in the far environment. Here, time spent vigilant by small prey animals increased in both the individual cat treatment and the combined cat with human disturbance treatment. This result is interesting given that cats were not detected in this site. Various factors may contribute to it. First, the result may in part reflect the fact that both brown and black rats were present at the far site in high numbers; both share an evolutionary history with the domestic cat and would be vigilant on encountering cat cues. Second, other species at the site may have displayed neophobic responses to cat cues that were manifested as vigilance. Certainly, antechinus species display a form of initial neophobia to in situ experiments (Tasker and Dickman, 2001). However, this response was not detected in other treatments, and antechinus have previously shown little aversive response to the presence of introduced predator cues (Dickman, 1993; Russell and Banks, 2007). Alternatively, the native small prey animals at the far site may have had exposure to domestic cats at other times, as cats prefer to hunt in open grassy habitats (McGregor et al., 2014) that occur in patches at the far environment in our study. At the close environment, highest vigilance by small prey animals was recorded in response to the combined treatment of cat with human disturbance. This implies that vigilance can be an effective coping response. The same may be true at the mid environment, except when the cat treatment only was used, as it yielded more time in vigilance, albeit less compared to when under the fox treatment.
Responses Along the Urban Disturbance Gradient
Direct comparisons between the environments along the disturbance gradient showed that the number of fauna visitors was highest at the far environment across all treatments, and that generally GUDs and time spent foraging per visit were lower. However, GUDs at the far environment were higher than those at the close environment when under the combined cat/fox with human disturbance treatments. Considering the fewer disturbances and expanse of the conservation reserve in which the far environment was located, the higher number of videos, and visitors, at the far environment is likely due to more active or larger populations. Larger populations and competition for food could also lead to lower GUDs and reduce the time spent foraging and vigilant (Davidson and Morris, 2001). The mid environment had the highest GUDs and fewest visitors, but higher average times spent foraging than at the close environment, except under the disturbance and fox treatments. Despite the fragmentation and reduced size of the close environment it still had more videos than the mid, and the most northern brown bandicoot activity, suggesting that the benefits of urban-edge environments can be reaped if animals can cope with the presence of additional stressors there (Fardell et al., 2021a).
Our results show some indication of different coping responses along the disturbance gradient. Minor temporal activity shifts were observed at the mid environment. At the close environment, adjustments to behavior and sensitivity to predators were manifest, and at the far environment, animals used different habitat components more (Fardell et al., 2021a). These differences may reflect animals’ past experiences and the amount of stressor exposure in each environment, and the differences in them, including the higher exposure to human disturbances and supplementary resources in the close environment, to paths in the mid environment, and to the large and heterogeneous connected habitat in the far environment. Whether these differences also reflect differences in physiological stress loads still needs to be confirmed. This will be important to increase understanding of how well small prey animals in urban and adjacent environments cope with the stressors of predators and human disturbances at the physiological level.
Responses Among Small Prey Animal Species
Of the small mammal species observed, the common brushtail possum spent the most time being vigilant, which supports the idea that this species is generally cautious (Mella et al., 2014). Their high levels of vigilance displayed in response to predator cues also reflects their susceptibility to red fox and domestic cat predation (Molsher et al., 1999; Fleming et al., 2021). High vigilance habits may “pre-adapt” the common brushtail possum to urban life, owing to the success this behavior has afforded them in problem solving (Wat et al., 2020). Brown and black rats (95–400 g) displayed the next highest levels of vigilance, then northern brown bandicoots (500–3100 g). These patterns may reflect high levels of predation in urban areas by red foxes on bandicoots (Fleming et al., 2021) and domestic cats on rodents (Barratt, 1997). The larger sizes of each species would make them a reward worth pursuing. Brown antechinus (17–71g) may be less vigilant as their small size increases their options for hiding, and their ability to zig zag in a spiral motion when ascending trees reduces their chance of capture (Dickman, 1991). Limited vigilance by birds invites a similar explanation: flight is a primary means of avoiding predators. Times spent in vigilance may therefore be supported by reference to species’ history, movement, and morphology. As food consumption is relative to size (Case, 1979), it is no surprise that differences in time spent foraging may in part be related to species size, with the larger species generally spending more time foraging. Differences in species assemblages across the urban disturbance gradient, may therefore, contribute to some of the observed differences in behavioral responses to stressors.
Temporal Activity Responses
While there was no indication of large shifts in temporal activity among species or treatments, there was evidence that temporal activity overlapped most at the close environment between the small prey animals and their stressors. Small changes in temporal activity were observed only at the mid environment. Under the fox with human disturbance treatment, compared to the control, the activity of brown antechinus increased slightly in the second half of the night (the am period), perhaps suggesting threat downgrading in the absence of new threat cues, as observed previously in rodents (Bedoya-Pérez et al., 2019; Fardell et al., 2021b). Under the treatments of cat, fox, and cat with human disturbance, the northern brown bandicoot appeared to exercise some caution when foraging by spreading activity more evenly across the night, compared to intensive activity under control conditions in the first (pm) hours of the night. Such a response may be a common mammalian coping mechanism, as gerbils that are naturally most active in the early evening also alter their activity across the night in response to stressors (Kotler et al., 1994). Under the treatments of fox, cat, fox with human disturbance, and pre-treatment, the common brushtail possum was more active across the night but active largely in the latter half of the night under the control. As the common brushtail possum rarely foraged at the GUD stations, its pattern of activity probably reflects trade-offs between curiosity and caution while moving across each environment and its encounters with different stressor cues. This interpretation coincides with findings that common brushtail possums leave risky food patches early even if this comes at the cost of reduced food consumption (Mella et al., 2014).
Conclusion
Our findings here align with our previous results that the impacts of combined urban stressors of novel predator activity and human disturbance are additive (Fardell et al., 2021a), as differences in the levels of coping responses to the combined stressors were the most pronounced. We proposed previously that stress from novel predator activity and human disturbance could be reduced by appropriate management of habitat components that small prey animals perceive as less risky under the urban stressors (Fardell et al., 2021a). Our current findings suggest that behavioral adjustments are also used to cope with urban stressors and are calibrated according to the relative pressure of those stressors, i.e., human disturbance mostly in the close environment, fox with human disturbance in the mid environment, and cat with human disturbance in the far environment. As the same small mammal and bird species occurred along the urban gradient, this suggests a level of plasticity in these species, with those under increased levels of chronic stressors expressing the greatest behavioral changes. The differences in the levels and mechanisms of coping responses that we observed – behavioral adjustments in the close environment, temporal shifts in the mid environment, and altered habitat component use in the far environment (Fardell et al., 2021a), emphasize the need for management actions to be tailored to position along disturbance gradients. Our findings show that urban environments along a disturbance gradient are each valuable to wildlife, and that while animals persist in these habitats, they still perceive human disturbance as a threat. This applies even in the urban-edge environment that has had more exposure to such threats. Further, introduced predators are perceived as causing differing levels of threat along the gradient, likely relative to disturbances that facilitate or change predator behaviors. Our findings also hint that the availability of natural food resources is important when coping with increased stressors, as animals consumed more food when under increased stressor conditions. Overall, our work shows that successful conservation outcomes for focal species in urban settings require consideration of stressor impacts and coping responses relative to the levels and types of disturbance that are present.
Data Availability Statement
The raw data supporting the conclusion of this article will be made available by the authors, without undue reservation. Requests to access these data should be directed to LF, bG9yZW4uZmFyZGVsbEBnbWFpbC5jb20=.
Ethics Statement
The monitoring of wildlife in situ was conducted under the animal ethics approval from the University of Sydney (2017/1275) and under a New South Wales Scientific License (SL102024). Written informed consent was obtained from the owners of the animals used to collect scent cues for this study.
Author Contributions
LF, CD, CP, and CN conceived the study. LF performed the field work and statistical analyses and wrote the initial manuscript draft. All authors edited and contributed to the subsequent drafts.
Funding
This research was supported by a Holsworth Wildlife Research Endowment, and a Lake Macquarie Environmental Research Grant funded by Lake Macquarie City Council and other sponsors, which in 2018–2019 included Hunter Water Corporation, Delta Electricity and Origin Energy. LF was supported by an Australian Government Research Training Program Stipend, and a University of Sydney Merit Award Scholarship.
Conflict of Interest
The authors declare that the research was conducted in the absence of any commercial or financial relationships that could be construed as a potential conflict of interest.
Publisher’s Note
All claims expressed in this article are solely those of the authors and do not necessarily represent those of their affiliated organizations, or those of the publisher, the editors and the reviewers. Any product that may be evaluated in this article, or claim that may be made by its manufacturer, is not guaranteed or endorsed by the publisher.
Acknowledgments
We acknowledge the traditional owners of country throughout Australia and recognize their continuing connection to lands, waters and communities. We pay our respect to Aboriginal and Torres Strait Islander cultures; and to Elders past, present and emerging. We recognize and acknowledge the Aboriginal people, known today as the Awabakal, as the traditional Custodians of the land presently known as the suburb of Whitebridge. We are grateful to Associate Professor John Clulow for stimulating discussions on urban wildlife in the Lake Macquarie area and for his direction to sites of high wildlife activity. We are also grateful to Dr. Lauren Young for stimulating discussions on spatial and statistical analyses. We are also grateful to Bobby Tamayo for his endless support and direction both logistically and in creative problem solving for in-field research.
Supplementary Material
The Supplementary Material for this article can be found online at: https://www.frontiersin.org/articles/10.3389/fevo.2022.805891/full#supplementary-material
References
Alberti, M., Marzluff, J., and Hunt, V. M. (2017). Urban driven phenotypic changes: empirical observations and theoretical implications for eco-evolutionary feedback. Philos. Trans. R. Soc. B Biol. Sci. 372:20160029. doi: 10.1098/rstb.2016.0029
Badyaev, A. V. (2005). Stress-induced variation in evolution: from behavioural plasticity to genetic assimilation. Proc. R. Soc. B-Biol. Sci. 272, 877–886. doi: 10.1098/rspb.2004.3045
Barnett, S. A. (1958). Experiments on ‘neophobia’ in wild and laboratory rats. Br. J. Psychol. 49, 195–201. doi: 10.1111/j.2044-8295.1958.tb00657.x
Barratt, D. G. (1997). Predation by house cats, Felis catus (L.), in Canberra, Australia. I. Prey composition and preference. Wildl. Res. 24, 263–277. doi: 10.1071/WR96020
Bazzaz, F. A. (1975). Plant species diversity in old-field successional ecosystems in southern Illinois. Ecology 56, 485–488. doi: 10.2307/1934981
Beauchamp, G. (2017). What can vigilance tell us about fear? Anim. Sentience 15:1. doi: 10.51291/2377-7478.1203
Bedoya-Pérez, M. A., Smith, K. L., Kevin, R. C., Luo, J. L., Crowther, M. S., and McGregor, I. S. (2019). Parameters that affect fear responses in rodents and how to use them for management. Front. Ecol. Evol. 7:136. doi: 10.3389/fevo.2019.00136
Bleicher, S. S., and Rosenzweig, M. L. (2018). Too much of a good thing? A landscape-of-fear analysis for collared peccaries (Pecari tajacu) reveals hikers act as a greater deterrent than thorny or bitter food. Can. J. Zool. 96, 317–324. doi: 10.1139/cjz-2017-0158
Blumstein, D. T. (2014). “Attention, habituation, and antipredator behaviour: implications for urban birds,” in Avian Urban Ecology, eds D. Gil and H. Brumm (New York: Oxford University Press). doi: 10.1093/acprof:osobl/9780199661572.003.0004
Boulton, K., Couto, E., Grimmer, A. J., Earley, R. L., Canario, A. V., Wilson, A. J., et al. (2015). How integrated are behavioral and endocrine stress response traits? A repeated measures approach to testing the stress-coping style model. Ecol. Evol. 5, 618–633. doi: 10.1002/ece3.1395
Boutin, S. (1990). Food supplementation experiments with terrestrial vertebrates: patterns, problems, and the future. Can. J. Zool. 68, 203–220. doi: 10.1139/z90-031
Brodie, E. D. III, and Brodie, E. D. Jr. (1999). Predator-prey arms races: asymmetrical selection on predators and prey may be reduced when prey are dangerous. Bioscience 49, 557–568. doi: 10.2307/1313476
Brooks, M. E., Kristensen, K., van Benthem, K. J., Magnusson, A., Berg, C. W., Nielsen, A., et al. (2017). GlmmTMB balances speed and flexibility among packages for zero-inflated generalized linear mixed modelling. R J. 9, 378–400. doi: 10.32614/RJ-2017-066
Brown, J. S. (1988). Patch use as an indicator of habitat preference, predation risk, and competition. Behav. Ecol. Sociobiol. 22, 37–47. doi: 10.1007/BF00395696
Brown, J. S. (1999). Vigilance, patch use and habitat selection: foraging under predation risk. Evol. Ecol. Res. 1, 49–71.
Brown, J. S. (2000). “Foraging ecology of animals in response to heterogeneous environments,” in The Ecological Consequences of Environmental Heterogeneity, eds M. J. Hutchings, E. A. John, and A. J. Stewart (Oxford: Blackwell), 181–214.
Case, T. J. (1979). Optimal body size and an animal’s diet. Acta Biotheor. 28, 54–69. doi: 10.1007/BF00054680
Chaby, L. E., Sheriff, M. J., Hirrlinger, A. M., and Braithwaite, V. A. (2015). Does early stress prepare individuals for a stressful future? Stress during adolescence improves foraging under threat. Anim. Behav. 105, 37–45. doi: 10.1016/j.anbehav.2015.03.028
Cincotta, R. P., Wisnewski, J., and Engelman, R. (2000). Human population in the biodiversity hotspots. Nature 404, 990–992. doi: 10.1038/35010105
Clinchy, M., Zanette, L. Y., Roberts, D., Suraci, J. P., Buesching, C. D., Newman, C., et al. (2016). Fear of the human “super predator” far exceeds the fear of large carnivores in a model mesocarnivore. Behav. Ecol. 27, 1826–1832. doi: 10.1093/beheco/arw117
Collins, M. K., Magle, S. B., and Gallo, T. (2021). Global trends in urban wildlife ecology and conservation. Biol. Conserv. 261:109236. doi: 10.1016/j.biocon.2021.109236
Côté, I. M., Darling, E. S., and Brown, C. J. (2016). Interactions among ecosystem stressors and their importance in conservation. Proc. R. Soc. B Biol. Sci. 283:20152592. doi: 10.1098/rspb.2015.2592
Cox, D. T., and Gaston, K. J. (2018). Human–nature interactions and the consequences and drivers of provisioning wildlife. Philos. Trans. R. Soc. B Biol. Sci. 373:20170092. doi: 10.1098/rstb.2017.0092
Dammhahn, M., Mazza, V., Schirmer, A., Göttsche, C., and Eccard, J. A. (2020). Of city and village mice: behavioural adjustments of striped field mice to urban environments. Sci. Rep. 10:13056. doi: 10.1038/s41598-020-69998-6
Dantzer, B., Fletcher, Q. E., Boonstra, R., and Sheriff, M. J. (2014). Measures of physiological stress: a transparent or opaque window into the status, management and conservation of species? Conserv. Physiol. 2:cou023. doi: 10.1093/conphys/cou023
Davidson, D. L., and Morris, D. W. (2001). Density-dependent foraging effort of deer mice (Peromyscus maniculatus). Funct. Ecol. 15, 575–583. doi: 10.1046/j.0269-8463.2001.00569.x
Dearborn, D. C., and Kark, S. (2010). Motivations for conserving urban biodiversity. Conserv. Biol. 24, 432–440. doi: 10.1111/j.1523-1739.2009.01328.x
Delgado-Baquerizo, M., Eldridge, D. J., Liu, Y. R., Sokoya, B., Wang, J. T., Hu, H. W., et al. (2021). Global homogenization of the structure and function in the soil microbiome of urban greenspaces. Sci. Adv. 7:eabg5809. doi: 10.1126/sciadv.abg5809
Department of Environment, Climate Change and Water [DECCW] (2010). Glenrock State Conservation Area Plan of Management. New South Wales: DECCW.
Díaz-Ruiz, F., Caro, J., Delibes-Mateos, M., Arroyo, B., and Ferreras, P. (2016). Drivers of red fox (Vulpes vulpes) daily activity: prey availability, human disturbance or habitat structure? J. Zool. 298, 128–138. doi: 10.1111/jzo.12294
Dickman, C. R. (1991). “Use of trees by ground-dwelling mammals: implications for management,” in Conservation of Australia’s forest fauna, ed. D. Lunney (Sydney: Royal Zoological Society of New South Wales), 125–136. doi: 10.7882/RZSNSW.1991.012
Dickman, C. R. (1993). Raiders of the last ark: cats in island Australia. Aust. Nat. Hist. 24, 44–52.
Ditchkoff, S. S., Saalfeld, S. T., and Gibson, C. J. (2006). Animal behavior in urban ecosystems: modifications due to human-induced stress. Urban Ecosyst. 9, 5–12. doi: 10.1007/s11252-006-3262-3
Doherty, T. S., Dickman, C. R., Glen, A. S., Newsome, T. M., Nimmo, D. G., Ritchie, E. G., et al. (2017). The global impacts of domestic dogs on threatened vertebrates. Biol. Consev. 210, 56–59. doi: 10.1016/j.biocon.2017.04.007
Doherty, T. S., Glen, A. S., Nimmo, D. G., Ritchie, E. G., and Dickman, C. R. (2016). Invasive predators and global biodiversity loss. Proc. Natl. Acad. Sci. U. S. A. 113, 11261–11265. doi: 10.1073/pnas.1602480113
Dominoni, D. M., Halfwerk, W., Baird, E., Buxton, R. T., Fernández-Juricic, E., Fristrup, K. M., et al. (2020). Why conservation biology can benefit from sensory ecology. Nat. Ecol. Evol. 4, 502–511. doi: 10.1038/s41559-020-1135-4
Donihue, C. M., and Lambert, M. R. (2015). Adaptive evolution in urban ecosystems. Ambio 44, 194–203. doi: 10.1007/s13280-014-0547-2
Fardell, L. L., Nano, C. E. M., Pavey, C. R., and Dickman, C. R. (2021a). Small prey animal habitat use in landscapes of fear: effects of predator presence and human activity along an urban disturbance gradient. Front. Ecol. Evol. Urban Ecol. 9:750094. doi: 10.3389/fevo.2021.750094
Fardell, L. L., Bedoya-Pérez, M. A., Dickman, C. R., Crowther, M. S., Pavey, C. R., and Narayan, E. J. (2021b). Are physiological and behavioural responses to stressors displayed concordantly by wild urban rodents? Sci. Nat. 108:5. doi: 10.1007/s00114-020-01716-8
Fardell, L. L., Young, L. I., Pavey, C. R., and Dickman, C. R. (2021c). Habitat use by wandering pet cats (Felis catus) in a patchy urban environment. J. Urban Ecol. 7:juab019. doi: 10.1093/jue/juab019
Fardell, L. L., Pavey, C. R., and Dickman, C. R. (2020). Fear and stressing in predator–prey ecology: considering the twin stressors of predators and people on mammals. PeerJ 8:e9104. doi: 10.7717/peerj.9104
Feng, A. Y., and Himsworth, C. G. (2014). The secret life of the city rat: a review of the ecology of urban Norway and black rats (Rattus norvegicus and Rattus rattus). Urban Ecosyst. 17, 149–162. doi: 10.1007/s11252-013-0305-4
Fernández-Juricic, E., and Tellería, J. L. (2000). Effects of human disturbance on spatial and temporal feeding patterns of Blackbird Turdus merula in urban parks in Madrid, Spain. Bird Study 47, 13–21. doi: 10.1080/00063650009461156
Fingland, K., Ward, S. J., Bates, A. J., and Bremner-Harrison, S. (2021). A systematic review into the suitability of urban refugia for the Eurasian red squirrel Sciurus vulgaris. Mamm. Rev. 52, 26–38. doi: 10.1111/mam.12264
Fleming, P. A., Crawford, H. M., Stobo-Wilson, A. M., Dawson, S. J., Dickman, C. R., Dundas, S. J., et al. (2021). Diet of the introduced red fox Vulpes vulpes in Australia: analysis of temporal and spatial patterns. Mamm. Rev. 51, 508–527. doi: 10.1111/mam.12251
Fleming, P., Meek, P., Ballard, G., Banks, P., Calridge, A., Sanderson, J., et al. (2014). Camera Trapping: Wildlife Management and Research. Clayton, VIC: CSIRO Publishing.
French, S. S., Fokidis, H. B., and Moore, M. C. (2008). Variation in stress and innate immunity in the tree lizard (Urosaurus ornatus) across an urban–rural gradient. J. Comp. Physiol. B 178, 997–1005. doi: 10.1007/s00360-008-0290-8
Frid, A., and Dill, L. (2002). Human-caused disturbance stimuli as a form of predation risk. Conserv. Ecol. 6:11. doi: 10.5751/ES-00404-060111
Gander, H., and Ingold, P. (1997). Reactions of male alpine chamois Rupicapra r. rupicapra to hikers, joggers and mountain bikers. Biol. Conserv. 79, 107–109. doi: 10.1016/S0006-3207(96)00102-4
Gaynor, K. M., Hojnowski, C. E., Carter, N. H., and Brashares, J. S. (2018). The influence of human disturbance on wildlife nocturnality. Science 360, 1232–1235. doi: 10.1126/science.aar7121
Hartig, F. (2021). DHARMa: Residual Diagnostics for Hierarchical (Multi-Level/Mixed) Regression Models. R package version 0.4.1. Available online at: http://florianhartig.github.io/DHARMa/ (accessed May 29, 2021).
Hobbs, R. J., Higgs, E. S., and Hall, C. (2013). Novel ecosystems: intervening in the new ecological world order. West Sussex: John Wiley and Sons. doi: 10.1002/9781118354186
Hughes, N. K., Price, C. J., and Banks, P. B. (2010). Predators are attracted to the olfactory signals of prey. PLoS One 5:e13114. doi: 10.1371/journal.pone.0013114
Iglesias-Carrasco, M., Aich, U., Jennions, M. D., and Head, M. L. (2020). Stress in the city: meta-analysis indicates no overall evidence for stress in urban vertebrates. Proc. Royal Soc. B. 287:20201754. doi: 10.1098/rspb.2020.1754
Ives, C. D., Lentini, P. E., Threlfall, C. G., Ikin, K., Shanahan, D. F., Garrard, G. E., et al. (2016). Cities are hotspots for threatened species. Glob. Ecol. Biogeogr. 25, 117–126. doi: 10.1111/geb.12404
Jessop, T. S., Urlus, J. A. K. E., Lockwood, T., and Gillespie, G. (2010). Preying possum: assessment of the diet of lace monitors (Varanus varius) from coastal forests in southeastern Victoria. Biawak 4, 59–63.
Johnson, M. T., and Munshi-South, J. (2017). Evolution of life in urban environments. Science 358:eaam8327. doi: 10.1126/science.aam8327
Kapourani, C. A. (2018). Beta Binomial for overdispersion, Rpubs for RStudio. Available online at: https://rpubs.com/cakapourani/beta-binomial (Accessed May 29, 2021).
Kittendorf, A., and Dantzer, B. (2021). Urban fox squirrels exhibit tolerance to humans but respond to stimuli from natural predators. Ethology 127, 697–709. doi: 10.1111/eth.13206
Koolhaas, J. M., Korte, S. M., De Boer, S. F., Van Der Vegt, B. J., Van Reenen, C. G., Hopster, H., et al. (1999). Coping styles in animals: current status in behavior and stress-physiology. Neurosci. Biobehav. Rev. 23, 925–935. doi: 10.1016/S0149-7634(99)00026-3
Kotler, B. P., Ayal, Y., and Subach, A. (1994). Effects of predatory risk and resource renewal on the timing of foraging activity in a gerbil community. Oecologia 100, 391–396. doi: 10.1007/BF00317860
Kotler, B. P., and Brown, J. S. (2017). Fear and loathing on the landscape: what can foraging theory tell us about vigilance and fear? Anim. Sentience 2:5. doi: 10.51291/2377-7478.1280
Kronfeld-Schor, N., and Dayan, T. (2003). Partitioning of time as an ecological resource. Annu. Rev. Ecol. Evol. Syst. 34, 153–181. doi: 10.1146/annurev.ecolsys.34.011802.132435
Lake Macquarie City Council [LMCC] (2012). City of Lake Macquarie City Council Vertebrate Pest Management Strategy 2012-2018. Lake Macquarie: Lakemac Print.
Lambert, K. G., Tu, K., Everette, A., Love, G., McNamara, I., Bardi, M., et al. (2006). Explorations of Coping Strategies, Learned Persistence, and Resilience in Long-Evans Rats: innate versus Acquired Characteristics. Ann. N. Y. Acad. Sci. 1094, 319–324. doi: 10.1196/annals.1376.042
Larson, C. L., Reed, S. E., Merenlender, A. M., and Crooks, K. R. (2016). Effects of recreation on animals revealed as widespread through a global systematic review. PLoS One 11:e0167259. doi: 10.1371/journal.pone.0167259
Laundré, J. W., Hernández, L., and Altendorf, K. B. (2001). Wolves, elk, and bison: re-establishing the “landscape of fear” in Yellowstone National Park, USA. Can. J. Zool. 79, 1401–1409. doi: 10.1139/z01-094
Legge, S., Woinarski, J. C., Dickman, C. R., Murphy, B. P., Woolley, L. A., and Calver, M. C. (2020). We need to worry about Bella and Charlie: the impacts of pet cats on Australian wildlife. Wildl. Res. 47, 523–539. doi: 10.1071/WR19174
Lendrum, P. E., Crooks, K. R., and Wittemyer, G. (2017). Changes in circadian activity patterns of a wildlife community post high-intensity energy development. J. Mammal. 98, 1265–1271. doi: 10.1093/jmammal/gyx097
Lenth, R. V. (2021). Emmeans: Estimated Marginal Means, aka Least-Squares Means. R package version 1.6.1. Available online at: https://CRAN.R-project.org/package=emmeans (accessed May 29, 2021).
Lerman, S. B., Narango, D. L., Andrade, R., Warren, P. S., Grade, A. M., and Straley, K. (2021). “Wildlife in the city: Human drivers and human consequences,” in Urban ecology: it’s nature and challenges, ed. B. Pedro (Boston: CABI Publishing), 37–66. doi: 10.1079/9781789242607.0037
Lima, S. L. (1987). Vigilance while feeding and its relation to the risk of predation. J. Theor. Biol. 124, 303–316. doi: 10.1016/S0022-5193(87)80118-2
Lima, S. L., and Dill, L. M. (1990). Behavioral decisions made under the risk of predation: a review and prospectus. Can. J. Zool. 68, 619–640. doi: 10.1139/z90-092
Łopucki, R., Klich, D., Ścibior, A., and Gołêbiowska, D. (2019). Hormonal adjustments to urban conditions: stress hormone levels in urban and rural populations of Apodemus agrarius. Urban Ecosyst. 22, 435–442. doi: 10.1007/s11252-019-0832-8
Lowry, H., Lill, A., and Wong, B. B. (2013). Behavioural responses of wildlife to urban environments. Biol. Rev. 88, 537–549. doi: 10.1111/brv.12012
Lyons, J., Mastromonaco, G., Edwards, D. B., and Schulte-Hostedde, A. I. (2017). Fat and happy in the city: eastern chipmunks in urban environments. Behav. Ecol. 28, 1464–1471. doi: 10.1093/beheco/arx109
Magle, S. B., Hunt, V. M., Vernon, M., and Crooks, K. R. (2012). Urban wildlife research: past, present, and future. Biol. Conserv. 155, 23–32. doi: 10.1016/j.biocon.2012.06.018
Makin, D. F., Chamaillé-Jammes, S., and Shrader, A. M. (2017). Herbivores employ a suite of antipredator behaviours to minimize risk from ambush and cursorial predators. Anim. Behav. 127, 225–231. doi: 10.1016/j.anbehav.2017.03.024
McEwen, B. S. (2004). Protection and damage from acute and chronic stress: allostasis and allostatic overload and relevance to the pathophysiology of psychiatric disorders. Ann. N.Y. Acad. Sci. 1032, 1–7. doi: 10.1196/annals.1314.001
McEwen, B. S., and Wingfield, J. C. (2003). The concept of allostasis in biology and biomedicine. Horm. Behav. 43, 2–15. doi: 10.1016/S0018-506X(02)00024-7
McGregor, H. W., Legge, S., Jones, M. E., and Johnson, C. N. (2014). Landscape management of fire and grazing regimes alters the fine-scale habitat utilisation by feral cats. PLoS One 9:e109097. doi: 10.1371/journal.pone.0109097
McPhearson, T., Pickett, S. T., Grimm, N. B., Niemelä, J., Alberti, M., Elmqvist, T., et al. (2016). Advancing urban ecology toward a science of cities. Bioscience 66, 198–212. doi: 10.1093/biosci/biw002
Mella, V. S. A., Banks, P. B., and McArthur, C. (2014). Negotiating multiple cues of predation risk in a landscape of fear: what scares free-ranging brushtail possums? J. Zool. 294, 22–30. doi: 10.1111/jzo.12146
Mineur, Y. S., Belzung, C., and Crusio, W. E. (2006). Effects of unpredictable chronic mild stress on anxiety and depression-like behavior in mice. Behav. Brain Res. 175, 43–50. doi: 10.1016/j.bbr.2006.07.029
Moll, R. J., Cepek, J. D., Lorch, P. D., Dennis, P. M., Robison, T., Millspaugh, J. J., et al. (2018). Humans and urban development mediate the sympatry of competing carnivores. Urban Ecosyst. 21, 765–778. doi: 10.1007/s11252-018-0758-6
Møller, A. P., Tryjanowski, P., Díaz, M., Kwieciński, Z., Indykiewicz, P., Mitrus, C., et al. (2015). Urban habitats and feeders both contribute to flight initiation distance reduction in birds. Behav. Ecol. 26, 861–865. doi: 10.1093/beheco/arv024
Molsher, R., Newsome, A., and Dickman, C. (1999). Feeding ecology and population dynamics of the feral cat (Felis catus) in relation to the availability of prey in central-eastern New South Wales. Wildl. Res. 26, 593–607. doi: 10.1071/WR98058
Navara, K. J., and Nelson, R. J. (2007). The dark side of light at night: physiological, epidemiological, and ecological consequences. J. Pineal Res. 43, 215–224. doi: 10.1111/j.1600-079X.2007.00473.x
Navarro-Castilla, Á, and Barja, I. (2014). Does predation risk, through moon phase and predator cues, modulate food intake, antipredatory and physiological responses in wood mice (Apodemus sylvaticus)? Behav. Ecol. Sociobiol. 68, 1505–1512. doi: 10.1007/s00265-014-1759-y
Newsome, T. M., Dellinger, J. A., Pavey, C. R., Ripple, W. J., Shores, C. R., Wirsing, A. J., et al. (2015). The ecological effects of providing resource subsidies to predators. Glob. Ecol. Biogeogr. 24, 1–11. doi: 10.1111/geb.12236
Nickel, B. A., Suraci, J. P., Allen, M. L., and Wilmers, C. C. (2020). Human presence and human footprint have non-equivalent effects on wildlife spatiotemporal habitat use. Biol. Conserv. 241:108383. doi: 10.1016/j.biocon.2019.108383
Nielsen, A. B., Van Den Bosch, M., Maruthaveeran, S., and van den Bosch, C. K. (2014). Species richness in urban parks and its drivers: a review of empirical evidence. Urban Ecosyst. 17, 305–327. doi: 10.1007/s11252-013-0316-1
Otto, S. P. (2018). Adaptation, speciation and extinction in the Anthropocene. Proc. R. Soc. B Biol. Sci. 285:20182047. doi: 10.1098/rspb.2018.2047
Ovadia, O., Ziv, Y., Abramsky, Z., Pinshow, B., and Kotler, B. P. (2001). Harvest rates and foraging strategies in Negev Desert gerbils. Behav. Ecol. 12, 219–226. doi: 10.1093/beheco/12.2.219
Patten, M. A., and Burger, J. C. (2018). Reserves as double-edged sword: avoidance behavior in an urban-adjacent wildland. Biol. Conserv. 218, 233–239. doi: 10.1016/j.biocon.2017.12.033
Prevedello, J. A., Dickman, C. R., Vieira, M. V., and Vieira, E. M. (2013). Population responses of small mammals to food supply and predators: a global meta-analysis. J. Anim. Ecol. 82, 927–936. doi: 10.1111/1365-2656.12072
R Core Team (2020). R: A language and environment for statistical computing. Vienna: R Foundation for Statistical Computing.
Rehnus, M., Wehrle, M., and Palme, R. (2014). Mountain hares Lepus timidus and tourism: stress events and reactions. J. Appl. Ecol. 51, 6–12. doi: 10.1111/1365-2664.12174
Reshamwala, H. S., Shrotriya, S., Bora, B., Lyngdoh, S., Dirzo, R., and Habib, B. (2018). Anthropogenic food subsidies change the pattern of red fox diet and occurrence across Trans-Himalayas. India J. Arid Environ. 150, 15–20. doi: 10.1016/j.jaridenv.2017.12.011
Ridout, M., and Linkie, M. (2009). Estimating overlap of daily activity patterns from camera trap data. J. Agric. Biol. Environ. Stat. 14, 322–337. doi: 10.1198/jabes.2009.08038
Roozendaal, B. (2000). Glucocorticoids and the regulation of memory consolidation. Psychoneuroendocrinology 25, 213–238. doi: 10.1016/S0306-4530(99)00058-X
Russell, B. G., and Banks, P. B. (2007). Do Australian small mammals respond to native and introduced predator odours? Austral Ecol. 32, 277–286. doi: 10.1111/j.1442-9993.2007.01685.x
Sapolsky, R. M., Romero, L. M., and Munck, A. U. (2000). How do glucocorticoids influence stress responses? Integrating permissive, suppressive, stimulatory, and preparative actions. Endocr. Rev. 21, 55–89. doi: 10.1210/edrv.21.1.0389
Schwarzenbach, R. P., Escher, B. I., Fenner, K., Hofstetter, T. B., Johnson, C. A., Von Gunten, U., et al. (2006). The challenge of micropollutants in aquatic systems. Science 313, 1072–1077. doi: 10.1126/science.1127291
Seto, K. C., Güneralp, B., and Hutyra, L. R. (2012). Global forecasts of urban expansion to 2030 and direct impacts on biodiversity and carbon pools. Proc. Natl. Acad. Sci. U. S. A. 109, 16083–16088. doi: 10.1073/pnas.1211658109
Shamoon, H., Maor, R., Saltz, D., and Dayan, T. (2018). Increased mammal nocturnality in agricultural landscapes results in fragmentation due to cascading effects. Biol. Conserv. 226, 32–41. doi: 10.1016/j.biocon.2018.07.028
Shanahan, D. F., Miller, C., Possingham, H. P., and Fuller, R. A. (2011). The influence of patch area and connectivity on avian communities in urban revegetation. Biol. Conserv. 144, 722–729. doi: 10.1016/j.biocon.2010.10.014
Shannon, G., McKenna, M. F., Angeloni, L. M., Crooks, K. R., Fristrup, K. M., Brown, E., et al. (2016). A synthesis of two decades of research documenting the effects of noise on wildlife. Biol. Rev. 91, 982–1005. doi: 10.1111/brv.12207
Shochat, E., Lerman, S. B., Anderies, J. M., Warren, P. S., Faeth, S. H., and Nilon, C. H. (2010). Invasion, competition, and biodiversity loss in urban ecosystems. Bioscience 60, 199–208. doi: 10.1525/bio.2010.60.3.6
Short, K. H., and Petren, K. (2008). Boldness underlies foraging success of invasive Lepidodactylus lugubris geckos in the human landscape. Anim. Behav. 76, 429–437. doi: 10.1016/j.anbehav.2008.04.008
Sih, A. (2013). Understanding variation in behavioural responses to human-induced rapid environmental change: a conceptual overview. Anim. Behav. 85, 1077–1088. doi: 10.1016/j.anbehav.2013.02.017
Slip, D. J., and Shine, R. (1988). Feeding habits of the diamond python, Morelia s. spilota: ambush predation by a boid snake. J. Herpetol. 22, 323–330. doi: 10.2307/1564156
Smith, J. A., Donadio, E., Pauli, J. N., Sheriff, M. J., and Middleton, A. D. (2019). Integrating temporal refugia into landscapes of fear: prey exploit predator downtimes to forage in risky places. Oecologia 189, 883–890. doi: 10.1007/s00442-019-04381-5
Smith, J. A., Gaynor, K. M., and Suraci, J. P. (2021). Mismatch Between Risk and Response May Amplify Lethal and Non-lethal Effects of Humans on Wild Animal Populations. Front. Ecol. Evol. 9:604973. doi: 10.3389/fevo.2021.604973
Soanes, K., and Lentini, P. E. (2019). When cities are the last chance for saving species. Front. Ecol. Environ. 17, 225–231. doi: 10.1002/fee.2032
Stearns, S. C., and Hoekstra, R. F. (2000). Evolution, an Introduction. Oxford: Oxford University Press.
Sterlemann, V., Ganea, K., Liebl, C., Harbich, D., Alam, S., Holsboer, F., et al. (2008). Long-term behavioral and neuroendocrine alterations following chronic social stress in mice: implications for stress-related disorders. Horm. Behav. 53, 386–394. doi: 10.1016/j.yhbeh.2007.11.001
Suraci, J. P., Clinchy, M., Zanette, L. Y., and Wilmers, C. C. (2019). Fear of humans as apex predators has landscape-scale impacts from mountain lions to mice. Ecol. Lett. 22, 1578–1586. doi: 10.1111/ele.13344
Tang, R., Li, W., Zhu, D., Shang, X., Guo, X., and Zhang, L. (2020). Raging elephants: effects of human disturbance on physiological stress and reproductive potential in wild Asian elephants. Conserv. Physiol. 8:coz106. doi: 10.1093/conphys/coz106
Tasker, E. M., and Dickman, C. R. (2001). A review of Elliott trapping methods for small mammals in Australia. Aust. Mammal. 23, 77–87. doi: 10.1071/AM01077
Taylor, B. D., and Goldingay, R. L. (2010). Roads and wildlife: impacts, mitigation and implications for wildlife management in Australia. Wildl. Res. 37, 320–331. doi: 10.1071/WR09171
Thibert-Plante, X., and Hendry, A. P. (2011). The consequences of phenotypic plasticity for ecological speciation. J. Evol. Biol. 24, 326–342. doi: 10.1111/j.1420-9101.2010.02169.x
Threlfall, C. G., Mata, L., Mackie, J. A., Hahs, A. K., Stork, N. E., Williams, N. S., et al. (2017). Increasing biodiversity in urban green spaces through simple vegetation interventions. J. Appl. Ecol. 54, 1874–1883. doi: 10.1111/1365-2664.12876
Towerton, A. L., Kavanagh, R. P., Penman, T. D., and Dickman, C. R. (2016). Ranging behaviour and movements of the red fox in remnant forest habitats. Wildl. Res. 43, 492–506. doi: 10.1071/WR15203
Twardek, W. M., Peiman, K. S., Gallagher, A. J., and Cooke, S. J. (2017). Fido, Fluffy, and wildlife conservation: the environmental consequences of domesticated animals. Environ. Rev. 25, 381–395. doi: 10.1139/er-2016-0111
Uchida, K., Suzuki, K. K., Shimamoto, T., Yanagawa, H., and Koizumi, I. (2019). Decreased vigilance or habituation to humans? Mechanisms on increased boldness in urban animals. Behav. Ecol. 30, 1583–1590. doi: 10.1093/beheco/arz117
Vincze, E., Papp, S., Preiszner, B., Seress, G., Bókony, V., and Liker, A. (2016). Habituation to human disturbance is faster in urban than rural house sparrows. Behav. Ecol. 27, 1304–1313. doi: 10.1093/beheco/arw047
Walker, B. G., Boersma, P. D., and Wingfield, J. C. (2005). Physiological and behavioral differences in Magellanic penguin chicks in undisturbed and tourist-visited locations of a colony. Conserv. Biol. 19, 1571–1577. doi: 10.1111/j.1523-1739.2005.00104.x
Wat, K. K., Banks, P. B., and McArthur, C. (2020). Linking animal personality to problem-solving performance in urban common brushtail possums. Anim. Behav. 162, 35–45. doi: 10.1016/j.anbehav.2020.01.013
Wingfield, J. C., Maney, D. L., Breuner, C. W., Jacobs, J. D., Lynn, S., Ramenofsky, M., et al. (1998). Ecological bases of hormone—behavior interactions: the “emergency life history stage”. Am. Zool. 38, 191–206. doi: 10.1093/icb/38.1.191
Wintle, B. A., Kujala, H., Whitehead, A., Cameron, A., Veloz, S., Kukkala, A., et al. (2019). Global synthesis of conservation studies reveals the importance of small habitat patches for biodiversity. Proc. Natl. Acad. Sci. U. S. A. 116, 909–914. doi: 10.1073/pnas.1813051115
Wu, Y., Wang, H., Wang, H., and Feng, J. (2018). Arms race of temporal partitioning between carnivorous and herbivorous mammals. Sci. Rep. 8:1713. doi: 10.1038/s41598-018-20098-6
Wulsin, A. C., Wick-Carlson, D., Packard, B. A., Morano, R., and Herman, J. P. (2016). Adolescent chronic stress causes hypothalamo–pituitary–adrenocortical hypo-responsiveness and depression-like behavior in adult female rats. Psychoneuroendocrinology 65, 109–117. doi: 10.1016/j.psyneuen.2015.12.004
Keywords: predator-prey, risk-sensitive foraging, urban biodiversity, green space, wild space, vigilance, time allocation foraging
Citation: Fardell LL, Nano CEM, Pavey CR and Dickman CR (2022) Small Prey Animal Foraging Behaviors in Landscapes of Fear: Effects of Predator Presence and Human Activity Along an Urban Disturbance Gradient. Front. Ecol. Evol. 10:805891. doi: 10.3389/fevo.2022.805891
Received: 31 October 2021; Accepted: 04 February 2022;
Published: 30 March 2022.
Edited by:
Serge Morand, Centre National de la Recherche Scientifique (CNRS), FranceReviewed by:
Bastiaan G. Meerburg, Wageningen University and Research, NetherlandsGuy Gilles Beauchamp, Concordia University, Canada
Copyright © 2022 Fardell, Nano, Pavey and Dickman. This is an open-access article distributed under the terms of the Creative Commons Attribution License (CC BY). The use, distribution or reproduction in other forums is permitted, provided the original author(s) and the copyright owner(s) are credited and that the original publication in this journal is cited, in accordance with accepted academic practice. No use, distribution or reproduction is permitted which does not comply with these terms.
*Correspondence: Loren L. Fardell, bG9yZW4uZmFyZGVsbEBnbWFpbC5jb20=