- 1Departamento de Ecologia, Universidade de São Paulo, São Paulo, Brazil
- 2Division of Biological Sciences, University of Montana, Missoula, MT, United States
- 3Departamento de Biodiversidade, Universidade Estadual Paulista (UNESP), Rio Claro, São Paulo, Brazil
Morphological attributes are important in determining the success of ecological interactions, such as the interactions between fleshy fruited plants and their seed dispersers. Morphological traits can present high levels of intraspecific variation both within and across populations. Such variation will not only mediate which interactions can be established locally but also the potential for populations to respond to perturbations and selective pressure. Here, we investigated patterns of morphological variation (body weight and beaks’ traits) among different populations of blue manakin (Chiroxiphia caudata), in the highly fragmented Atlantic Forest, Brazil, both in terms of mean trait values – that might mediate interactions locally – and in their habitat-level variation which influences their evolutionary potential. Using metrics of evolutionary potential derived from quantitative genetics theory, we hypothesized that ecological stress would lead to a decline in the overall evolutionary potential in manakin populations. We found that populations differ slightly in their mean morphological attributes, with the exception of a population that occurs in temperate Araucaria forests. Nevertheless, we found a striking difference in the evolutionary potential of populations from different vegetation types. Specifically, populations that occur in ecological stress areas, Araucaria forests, and forest fragments immersed in savanna presented a smaller overall and conditional evolvability, suggesting their lower ability to respond in the direction of selection. This pattern might have important implications for the role of this species as seed disperser, as populations under stress may lack morphological variation (and covariation) that can be important to allow a given population to disperse seeds of multiple types of fruits. Moreover, a smaller evolvability might impact the potential of these populations to persist and perform their ecosystem services in face of environmental changes.
Introduction
The evolutionary potential is the ability of a given population to respond to selective pressures (Hansen and Houle, 2008; Teplitsky et al., 2014; Milot et al., 2020). This ability depends upon available genetic (co)variance, as higher variation in a population makes the presence of specific and benefic trait combinations more likely (Walsh and Lynch, 2018). Moreover, the presence of a range of trait combinations within populations could also facilitate the establishment of a diverse set of ecological interactions (Bolnick et al., 2011), with consequences for ecosystem service provision. The structure of genetic and phenotypic (co)variance within populations, however, may be strongly affected by habitat fragmentation, which may isolate populations with contrasting phenotypes and evolutionary potentials. As a result, populations in fragmented landscapes could present contrasting ecological roles and abilities to respond to current and future selective pressures. In this sense, understanding how the morphology and evolutionary potential of populations are distributed in highly fragmented landscapes will help us to assess the conservation status of these systems and predict the ability of species to track future environmental disturbance.
The genetic architecture of traits is the amount of additive genetic variance of a given trait and the covariation among traits (Lande, 1979). Thus, the response of a population to selection depends not only upon selection – its direction and magnitude – but also upon the population-level genetic architecture underlying traits (Assis et al., 2016b; Milot et al., 2020). The nature of the association between traits, i.e., the strength and sign of the association, may impact not only the ability of a population to respond to diversified selective pressures but also their ability to interact with a diversity of other species. For instance, populations with a loose association among traits occupy a higher portion of the morphospace (Figure 1D), increasing the population’s ability to interact with a higher diversity of ecological partners that possess different morphologies (Dehling et al., 2016). Instead, populations with a tight association among traits will present lower morphological variation which could restrict their ecological interactions (Figure 1C). Morphological variation within populations, therefore, has implications both to the persistence of populations and potentially to their ecological role and contribution to ecosystem services.
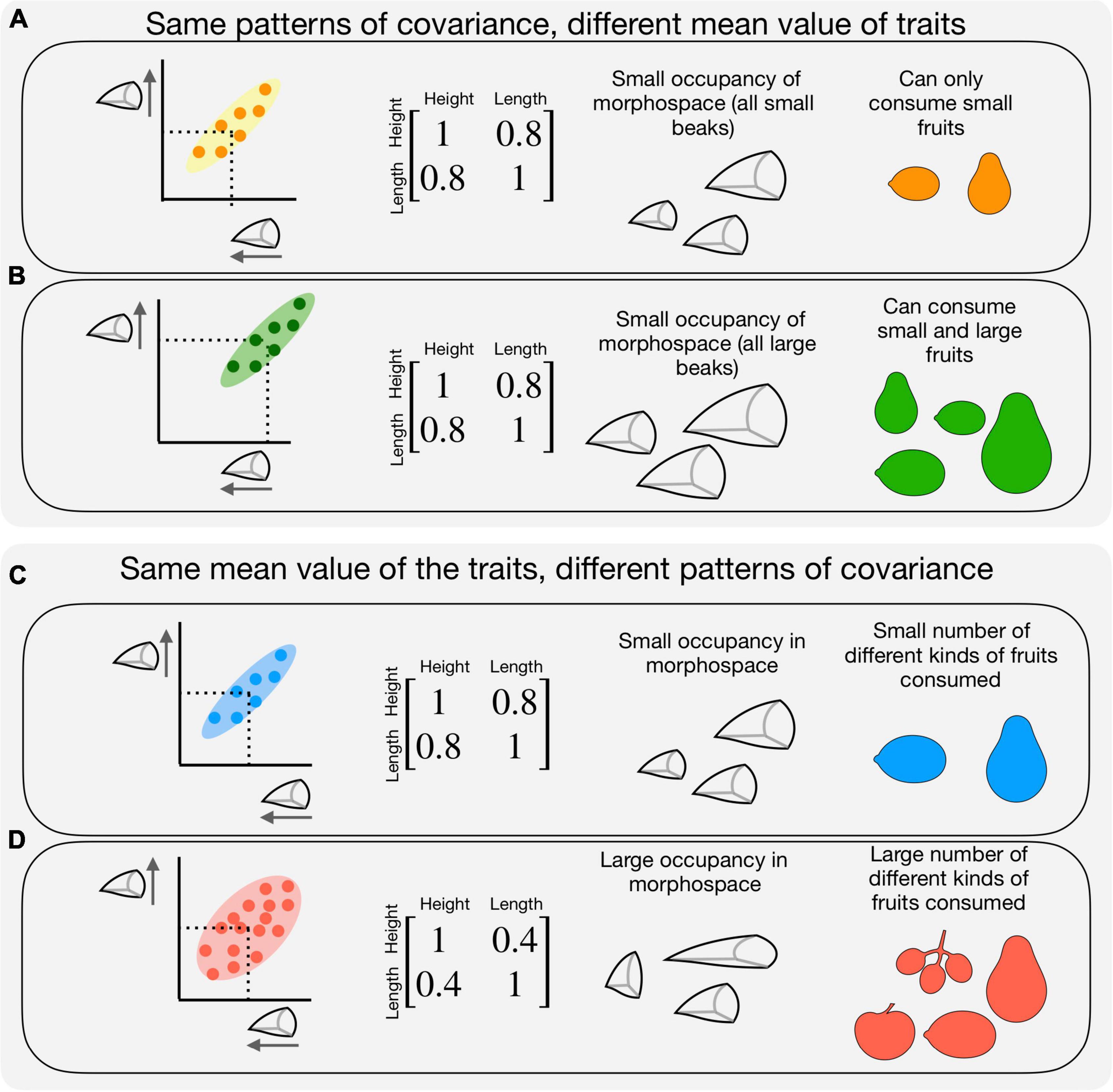
Figure 1. Theoretical examples on how differences in trait mean and variation may affect bird-fruit interactions. Plots in first column depicting beak length on X-axis and beak height on Y-axis, dots represent individuals. Matrices in the second column represent the covariance matrix exemplifying the differences in covariance structure (we assume trait variance to be 1). Third column exemplifies the occupancy of morphospace by birds and the last column exemplifies the variation of fruits a given occupancy of morphospace allows a frugivore to consume. Two populations that possess the same patterns of covariance between traits might present different mean population trait values (A,B). These difference in mean may impact the plant species a given bird might interact with. Where populations with larger trait values (B) might consume larger fruits. Conversely, (C,D) two populations may possess the same mean values for two traits, but still present different underlying patterns of covariance between the two traits, so that in one population the two traits are tightly correlated (C) while in the other the traits are loosely correlated (D), as represented on the left by the ellipses (the patterns of covariation in the population). In a population with a tight correlation between beak height and length (C) individuals with larger beak height necessarily present larger beak length, while a loose correlation between beak height and length results in a population with individuals presenting different combinations of the two traits (D). Differences in the population-level covariance structure might impact the patterns of interaction with plant species (the diversity of fruit types a population can eat).
An essential ecosystem service provided by animals is seed dispersal (Jordano, 2000). Among animals, birds are a major component of frugivore diversity (Fricke and Svenning, 2020), with their high levels of functional diversity being mapped accordingly in a broad range of ecological roles (Pigot et al., 2016). Birds’ gape size and body mass are important functional traits for frugivores, as they mediate birds’ abilities to interact with fruits and to disperse their seeds (Dehling et al., 2016): while small-gaped bird species are limited to a smaller range of fruit sizes and, consequently, species (Figure 1A), large-gaped birds are able to exploit a wide range of species and are the only ones to disperse species with larger seeds (Wheelwright, 1985; Levey, 1987) (Figure 1B). Our understanding on the relationships between functional diversity and plant-bird interactions is mostly restricted to the species level (but see Crestani et al., 2019). But there is mounting evidence showing that individual level diet preference might impact the species that a frugivore interacts with, for example, the helmeted manakin, which is a generalist species, presents varying degree of specialization among individuals (Pires and de Melo, 2020). In this sense, we can also expect trait variation across bird individuals to mediate the ecological and evolutionary consequences of plant-bird interactions (Guimarães, 2020), as particular trait combinations may constrain the fruits individual birds can eat (Figure 1). Understanding how birds’ traits are distributed across and within populations is, therefore, an essential step toward a better understanding on how phenotypic diversity shapes ecological interactions across different levels of ecological organization (Guimarães, 2020).
Manakins (Pipridae) are an endemic bird family from the Neotropical region and a dominant frugivore family in many forested communities (Terborgh et al., 1990). In the Brazilian Atlantic Forest, the blue manakin, Chiroxiphia caudata, is an important seed disperser of several plant species, feeding on a wide variety of fruits with different morphological attributes (Hasui et al., 2009). This species occurs throughout the Atlantic Forest, across several vegetation types but mainly associated with forest habitats. A study with four different populations found relatively stable and small effective population sizes, moderate population structure and very low distance-dependent gene flow among populations, suggesting that the neutral genetic variation in this species is in equilibrium (Francisco et al., 2007). Its habitat, however, is severely threatened as the Atlantic Forest – one of the world’s biodiversity hotspots (Myers et al., 2000) – currently occupies 28% of its biome area (Rezende et al., 2018). Even if higher when compared to previous estimates of habitat cover (11–16%, Ribeiro et al., 2009), older native forest is being replaced by secondary forest (Rosa et al., 2021), and most of its area is currently distributed across thousands of small, isolated and severely disturbed fragments (Ribeiro et al., 2009; Rosa et al., 2021).
Habitat loss and fragmentation have disrupted the movement of manakins (Hansbauer et al., 2008; Barbosa et al., 2017) affecting gene flow among populations (Francisco et al., 2007). If the blue manakin were now a collection of isolated populations, we would expect to observe (1) variation in the genetic structure across populations, and (2) variation in morphological traits. Moreover, different vegetation types might impose distinct selective pressures that could result in both different mean phenotypes through local adaptation, different patterns of genetic covariation, and different levels of evolutionary potential. In fact, local adaptation and variable selective pressures can produce strong phenotypic differentiation among populations, even in the presence of high gene flow (Thompson, 2005; Fitzpatrick et al., 2015). This indicates that even in the presence of high gene flow, the morphological attributes and evolutionary potential [i.e., the degree of genetic (co)variance] of populations might strikingly differ among populations, which can have cascading effects in the ecosystem services these populations provide.
Here, we investigated whether populations of blue manakin occurring in different vegetation types of the Atlantic Forest presented different mean morphological attributes of beak and body size. Moreover, we investigated whether populations occurring at different vegetation types presented different levels of evolutionary potential which might impact their potential to respond to novel selective pressures and their ability to perform their role as seed dispersers in both current and future ecological scenarios. To do so, we investigated the patterns of phenotypic variance and covariance among traits across distinct vegetation types. We hypothesized that populations of blue manakin under ecological stress would have lower evolutionary potential.
Materials and methods
Sampling procedures
We used trait data from C. caudata specimens derived from the Atlantic Birds dataset (Rodrigues et al., 2019), a dataset of morphological georeferenced bird specimens from the Atlantic Forest of Brazil. From the 1,661 specimens available we selected the specimens of C. caudata that had a complete description of the following morphological traits: body size (in grams), beak width, length and height (in mm). We chose these traits because they are important functional traits, mediating fruit consumption by C. caudata individuals, as beak shape and size directly impacts the plant species a bird can eat and, therefore, the seed dispersal services provided by C. caudata individuals (Jordano, 2000; Galetti et al., 2013). We also chose body size because body size is an important determinant of ecological interactions (Eklöf et al., 2013), and in several species it has been reported as a response trait to fragmentation (Warzecha et al., 2016; Tuff et al., 2019). We also restricted our sample to specimens that had reliable information on the specimen locality (Figure 2 and Supplementary Table 1). All the specimens used were collected between 1998 and 2001, which guarantees that the conditions they experienced on fragmentation and the forest distribution were similar to the observed nowadays.
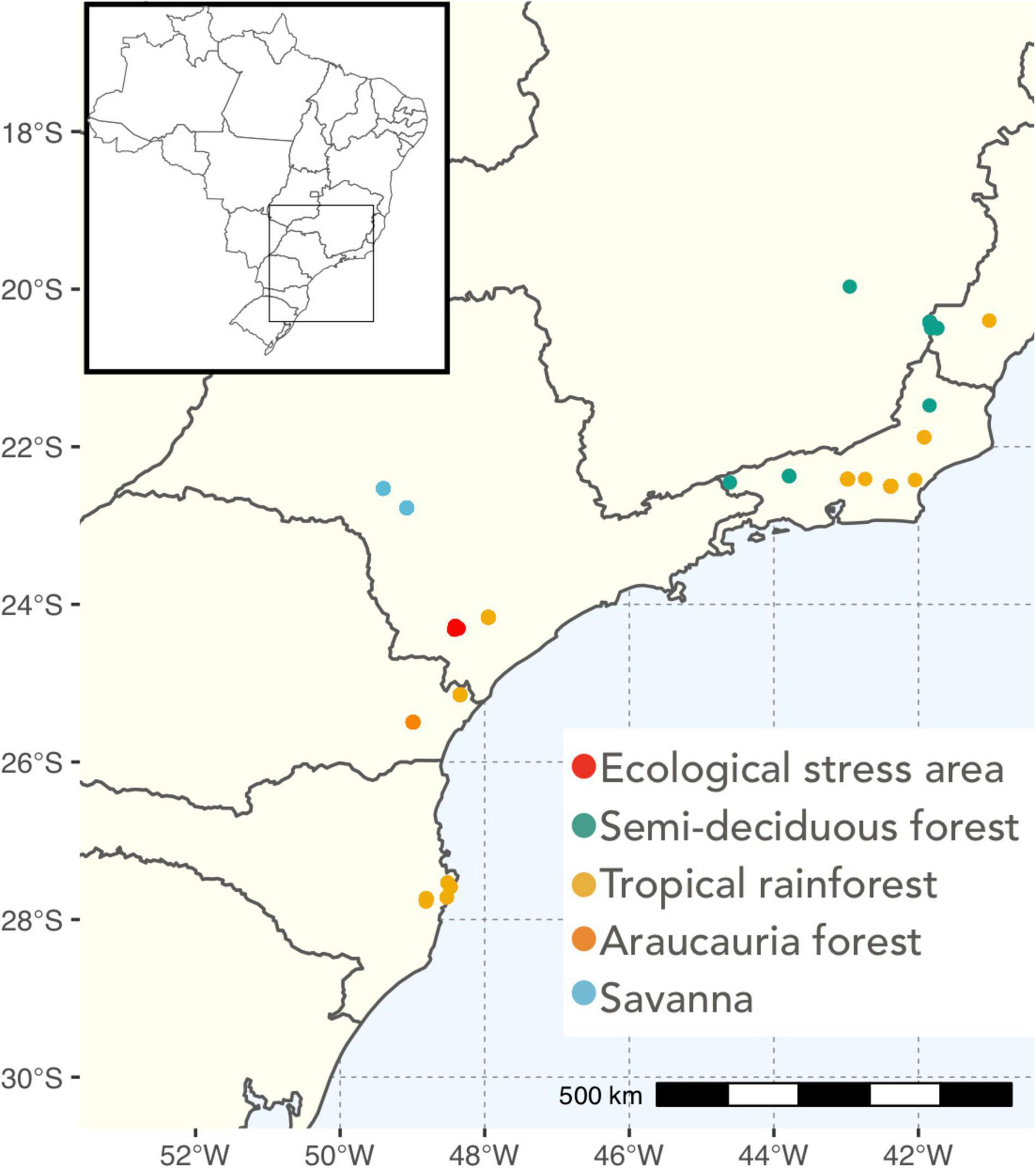
Figure 2. Map displaying the localities from sampled Chiroxiphia caudata individuals used in this study, different colors represent different forest vegetation types.
Vegetation types
We divided the specimens according to the vegetation type where the specimen was sampled, using categories defined in Ribeiro et al. (2009). C. caudata is present in six different vegetation types: (1) ecological stress area, which are areas under ecological stress such as fragmentation (2) deciduous forest; (3) semi-deciduous forest, (4) tropical rainforest, (5) Araucaria Forest, and (6) savanna (Cerrado). Ecological stress areas are areas under strong fragmentation pressure and ecotones between two different vegetation types (Ribeiro et al., 2009). It is important to note that even when the specimen was collected in savanna it was sampled in the associate forest patch, since C. caudata is a strict forest specialist (Barbosa et al., 2017). Due to the small sample size in deciduous forest (2 specimens), we removed these specimens and vegetation type from subsequent analyses (total number of individuals for subsequent analyses 237). We then compared the mean morphological traits across vegetation types to understand whether and how habitat and morphology are associated. This comparison was made using a multivariate One-Way-Manova, and subsequent differences were investigated using Tukey post hoc test to disentangle which groups significantly differed. Also, we used a principal component analysis to compare overall morphology across vegetation types. To do so, we first standardized traits in z-scores to remove scaling effects, and plotted individuals in a two principal component analysis graph. We also separated the populations in each vegetation types according to geographic distance for all analyses to investigate whether the patterns we observed comparing vegetation types was also reproduced in smaller geographical scales. Because C. caudata is an understory species, that does not disperse very far (Francisco et al., 2007), we assigned to a given population individuals that were collected less than 20 km from each other (see Supplementary material) and analyzed populations that contained more than 10 individuals for the comparisons among means (ESA = 1 population, SDF = 1 population, TR = 6 populations, AF = 1 population, Sav = 2 populations) and more than 15 individuals for the analyses that required the estimation of covariance matrices (ESA = 1 population, SDF = 1 population, TR = 3 populations, AF = 1 population, Sav = 1 population) (Supplementary Figures 3, 4).
Estimation of covariance matrices
All evolutionary potential metrics are dependent upon the estimation of covariance matrices. Therefore, after comparing the mean traits in each vegetation type, we estimated covariance matrices among traits for each vegetation type with the aim to investigate whether habitat type is associated with evolutionary potential in this species. In a covariance matrix each row and column represent a trait, in the diagonal we have the variance of each trait and in the off-diagonal the covariance among the two traits. While ideally one should have the estimation of G-matrices (i.e., additive genetic covariance matrices) when studying the potential response to selection, estimation of G-matrices in the wild are hampered by the difficulty in obtaining pedigreed samples, as they are necessary to estimate the genetic parameters (Wilson et al., 2010). Therefore, we used P-matrices, i.e., the phenotypic variance/covariance matrices, as surrogates (Marroig and Cheverud, 2001; de Oliveira et al., 2009; Shirai and Marroig, 2010; Rossoni et al., 2017). P-matrices are a good approximation of G-matrices in multiple systems, especially for vertebrate morphological traits, as G and P matrices tend to be proportional (Marroig and Cheverud, 2001; de Oliveira et al., 2009; Rossoni et al., 2019). For the estimation of the phenotypic covariance matrices we calculated the cubic root of the body size, to make body size into the same scale as the other morphological measurements. We then estimated the covariance matrices for the whole populations for each habitat, resulting in five different matrices. We performed a MonteCarlo resampling to estimate samples of covariance matrices, considering the sample size of 30 individuals to estimate the dispersion in the estimation of all evolutionary potential metrics.
Evolutionary potential metrics
In order to compare the evolutionary potential in each vegetation type we estimated five different metrics. First, we compared in a pairwise way each covariance matrix using Random Skewers to gauge how structurally similar they are. This metric uses Lande’s response to selection equation to calculate how similar (in terms of direction) would a response to selection be when comparing two populations. We also estimated evolvability and conditional evolvability (Hansen and Houle, 2008) which are metrics that measure the amount of the response to selection that would be in the direction being selected, considering only a directional selection scenario (evolvability) or with the other traits under stabilizing selection (conditional evolvability). Finally, we also measured the mean squared correlation (r2) which calculates the mean value of correlation observed for all traits and flexibility that measures the angle between the selection vector and response to selection to gauge how biased the response to selection would be (Table 1).
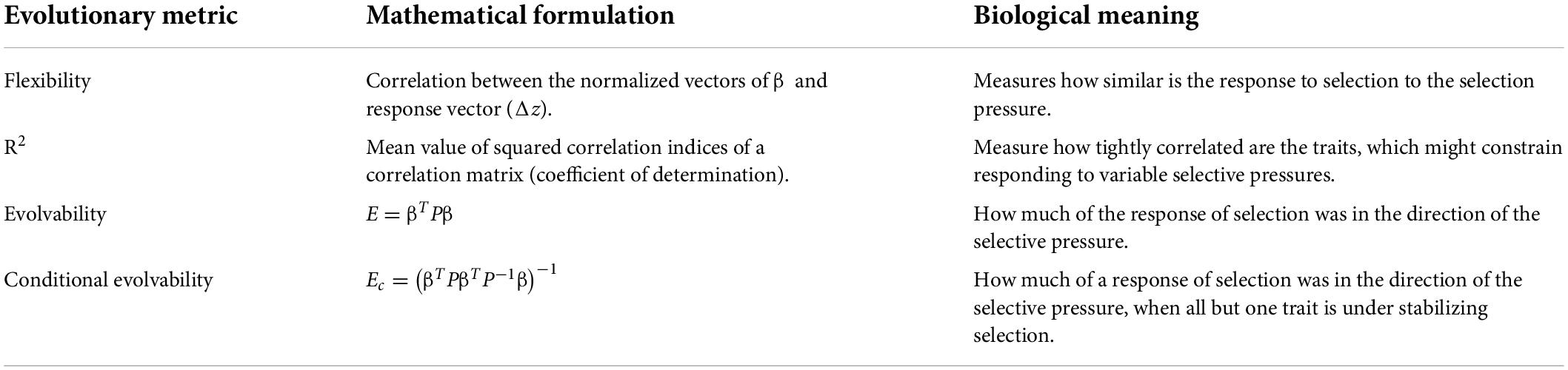
Table 1. Evolutionary potential metrics, their respective mathematical formulation and biological meaning.
Random skewers
First we estimated whether the covariance matrices in different vegetation types were structurally similar. To do this, we used the metric Random Skewers which is based in the Lande’s multivariate response to selection equation (Lande, 1979). This metric is calculated by applying 10,000 selection gradients randomly sampled from a normal distribution (simulating selection in random directions of the morphospace) to different covariance matrices. Thus, it is a pairwise comparison to compare the response to selection to the same set of selection vectors from one population to the other. This comparison is made by calculating the angle of the response to selection from one population and the other population in a pairwise way (Marroig and Cheverud, 2001). The mean value of the 10,000 angles between response vectors describes how similarly two populations respond to selection and is an indication of the overall similarity between the two covariance matrices. The smaller the angle, the higher is the similarity in the potential evolutionary response between both populations. Because matrices are estimated with error due to sampling, we calculated the matrix repeatability, to adjust the similarity between two matrices given the repeatability of a matrix, as done in Cheverud and Marroig (2007). Matrix repeatability is a form of correcting for sample size biases, as it is known that covariance matrices estimation are impacted by small sample sizes. This correction is done by estimating the similarity between matrices from the same population estimated using resampling (which should theoretically be equal 1, as they derive from the same population). Therefore, adjusted by repeatability matrices can have values greater than one.
Evolvability
Next, we estimated the mean evolvability, a measure of the amount of the response to selection that was in the direction of selection (Hansen and Houle, 2008; Assis et al., 2016a). Evolvability, E, is estimated as the projection of the response vector on the selection vector. The equation used to estimate evolvability is E = βTPβ, where β is the selection gradient, T denotes transposition and P is the covariance matrix (the phenotypic covariance matrix in each habitat). This equation gives us the value of evolvability respective to a given selection gradient (β) (Hansen and Houle, 2008). Therefore, to have an estimate of mean evolvability, we calculated the mean evolvability by simulating 1,000 different selection gradients for each MonteCarlo resampled matrix and habitats. These selection gradients were sampled from a normal distribution, with mean zero and standard deviation one. Then the selection vectors were normalized to one before being multiplied by the covariance matrices (equation above), estimating evolvability to each habitat.
Conditional evolvability
In addition to a measure of evolvability we also estimated a measure of conditional evolvability. This metric computes the length of the response to selection in a given direction considering the other directions in morphospace to be under stabilizing selection, i.e., how free to change in the direction of selection is the trait when the traits not directly under directional selection are under stabilizing selection. This was measured by calculating Ec = (βTPβTP−1β)−1 for a given selection gradient (β), in which T denotes transposition and −1 the inverse of a given matrix (Hansen and Houle, 2008). As with the evolvability measure we sampled the selection gradient from a normal distribution and the vector was normalized to one, and estimated the conditional evolvability for each habitat and resampled MonteCarlo matrices.
Mean squared correlation R2
This metric measures the mean correlation of a given correlation matrix, and therefore gives us a measurement of how tight the correlations between traits are, and in this sense how much of a given selection gradient in one trait would result in change in other traits. A correlation matrix is a normalized covariance matrix in which the diagonal is equal to one (as by definition a trait is perfectly correlated with itself), and the off diagonal presents the correlation between two traits ranging from −1 to 1. The higher the value of the R2 the tighter is the correlations in the matrix (Marroig and Cheverud, 2005; Marroig et al., 2009; Shirai and Marroig, 2010; Assis et al., 2016a). To calculate this, we transformed the MonteCarlo resample covariance matrices into correlation matrices and estimated the average of the square of the correlations in the correlation matrix.
Flexibility
Flexibility measures how aligned to selection a given population can respond, suggesting how flexible a population is to respond to selection (Marroig et al., 2009). We calculated it by estimating the average correlation between 1,000 simulated selection gradient vectors and the respective predicted response to selection. The selection gradient was drawn from a normal distribution and normalized to norm 1.
All evolutionary potential metrics might be influenced by sample size, as sample size impacts the estimation of the covariance and correlation matrices. Therefore, to evaluate the impact of sample sizes in our metric estimations we performed a rarefaction analysis for each metric, in each habitat (Supplementary Figures 5–8).
Results
We analyzed 237 specimens, 30 from Ecological stress areas, 33 from semi-deciduous forests, 122 from tropical rainforests, 27 from Araucaria forests, and 39 from savanna-associated forests (Supplementary Table 1). Araucaria forest blue manakins presented a smaller beak morphology in all three beak traits (Table 2 and Supplementary Figure 2), when compared with the other habitats (Figure 3 and Table 2). Savanna’s blue manakins present a smaller body size than the rest of the habitats (Figure 3). The one-way-MANOVA analysis showed that the different vegetation types differed in their mean values significantly (Pillai’s trace = 0.77, F16,928 = 13.84, p < 0.001). The post hoc Tukey test showed several pairwise differences in each trait and vegetation types (Figure 3). When considered together in a principal component analysis the different habitats overlap in the space of the two first principal components (Figure 4), with their centroid slightly shifted between the different populations, especially the Araucaria forest. The first principal component represents an allometric size component (with all traits loadings in the same direction) and explains 46.3% of the total variance (with loadings equal beak depth = 0.49, length = 0.60, width = 0.61, and body size = 0.07), and the second principal component is mainly associated with body size (with loadings equal beak depth = 0.05, length = 0.02, width = 0.05, body size = −0.99), and explains 24.8% of the total variance).
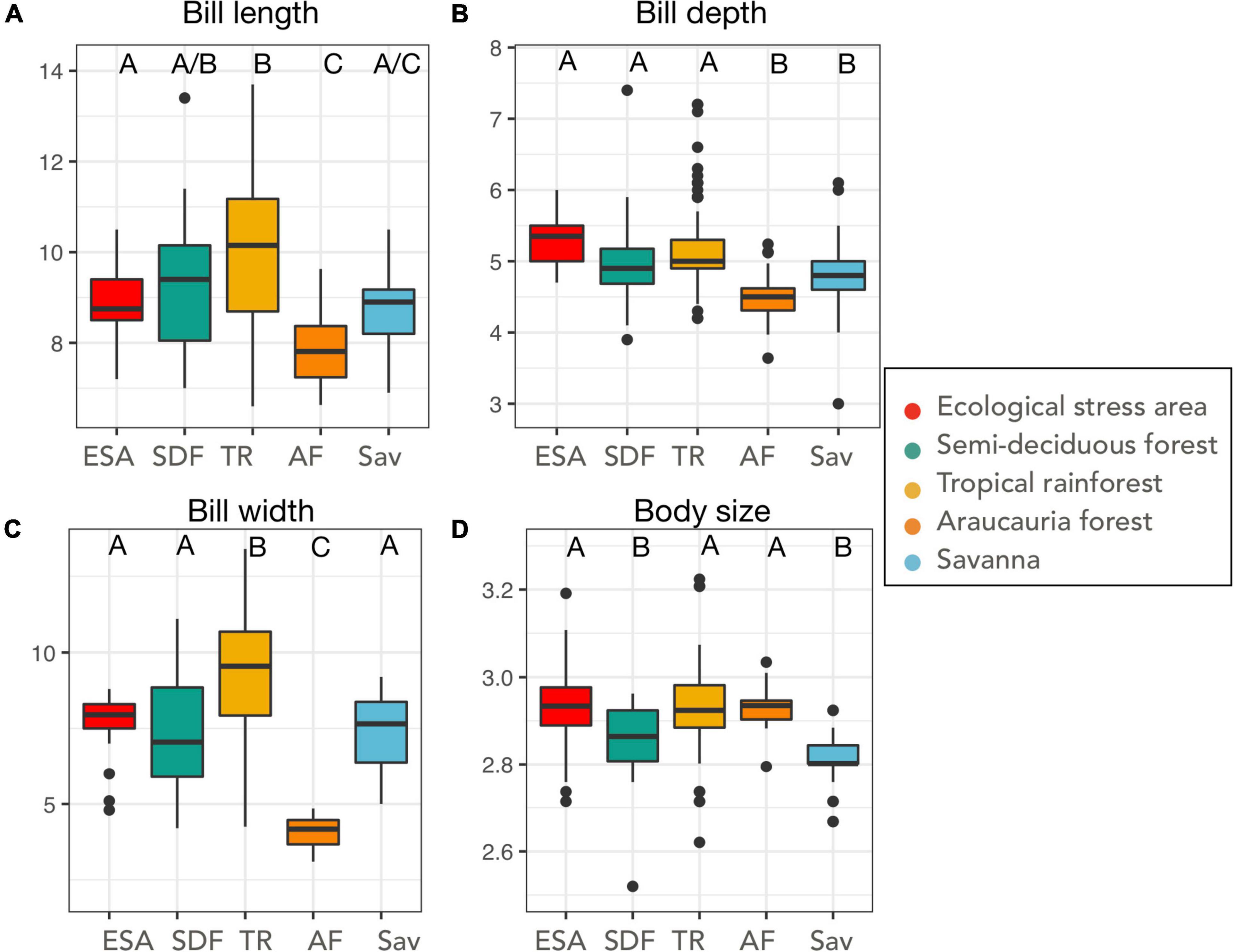
Figure 3. Boxplots depicting traits distribution for each habitat analyzed. (A) Bill length, (B) bill depth, (C) bill width, (D) body size. The boxplot represents the median values, boxes are 50% distribution, whiskers are 75% distribution and points are outliers. Letters on top of each graph represent the groups formed by Tukey pairwise tests. ESA, ecological stress areas; SDF, semi-deciduous forest; TR, tropical forest; AF, Araucaria forest; Sav, savanna.
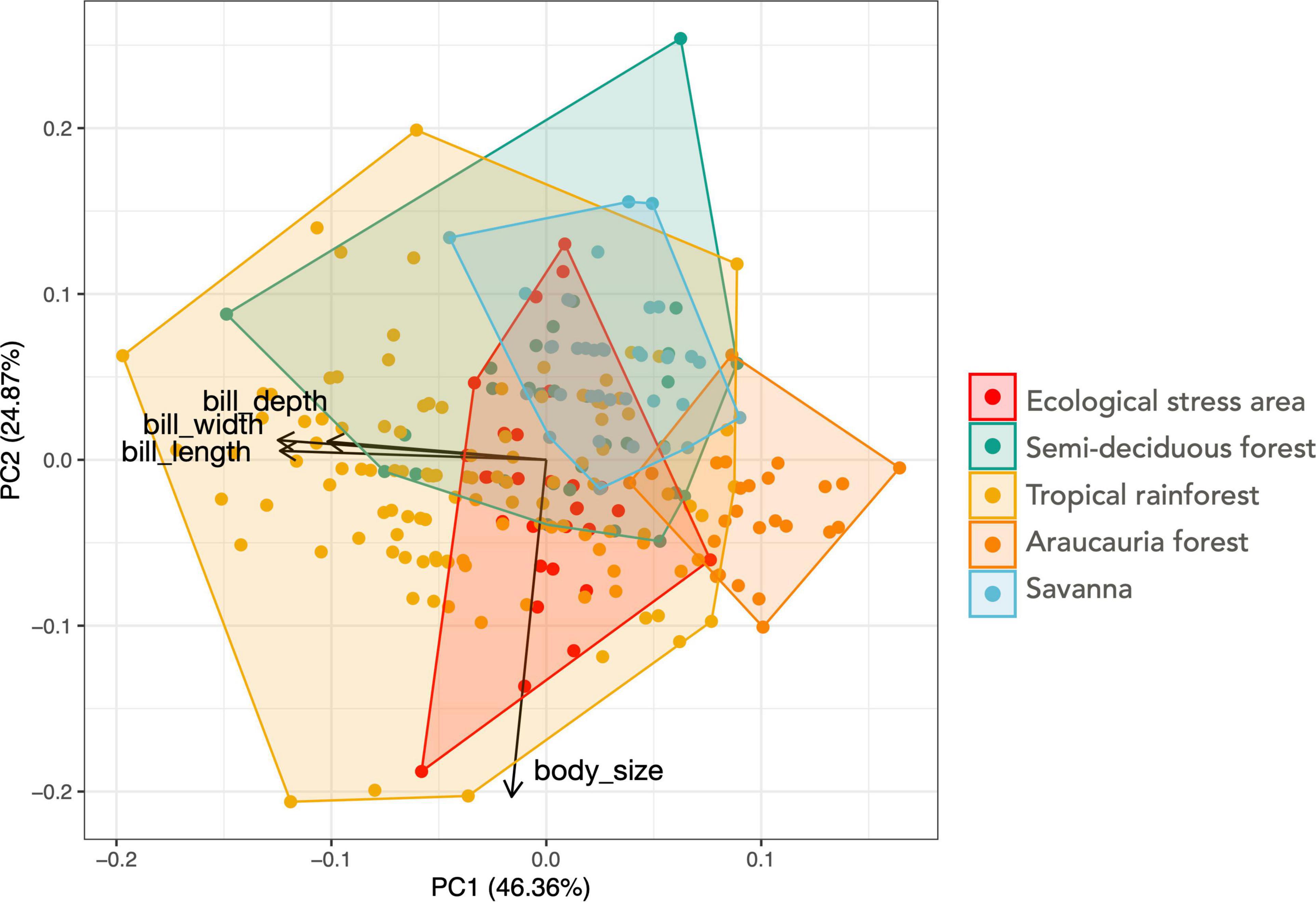
Figure 4. PCA plot of the morphological traits used. The first principal component is an allometric size component, while the second principal component is mainly a body size component. Each dot represents a projection of one individual in this principle component space. Polygons represent the total morphospace occupation of populations from each vegetation type and arrows show the loadings of the different traits.
The matrices comparisons using Random Skewers showed that the matrices derived from all habitats were highly similar, with a mean value of 0.90 among all comparisons for the adjusted by repeatability matrices, and the lowest similarities were observed among the comparisons including the Araucaria forest habitat (Table 3). All comparisons were significant indicating that the similarity among the matrices was higher than expected by chance. These high values of matrix similarity indicate that the populations in each habitat tend to respond to selection in a similar way, and their matrix structures are similar. Despite their structural similarities, our next results show that populations from different habitats present important differences in their evolutionary potential.

Table 3. Random Skewers results, where below the diagonal is the mean of the response to selection vectors correlations in the raw matrices and above diagonal we estimated the correlation between pairwise covariance matrices taking into account the repeatability of the matrix.
Evolvability, was higher for the populations from tropical rainforests, with a mean value of 2.19 and 95% confidence interval from the resampled matrices ranging from 1.35 to 3.11 (Figure 5A). Semi-deciduous forests populations also presented a high mean value of evolvability of 1.60 (95% CI from 1.12–2.22). The other habitats presented much lower values of evolvability, with the mean value for savanna being 0.56 (95% CI: 0.38–0.78), mean value for ecological stress areas being 0.37 (95% CI: 0.25–0.52) and mean value for Araucaria forest being 0.22 (95% CI: 0.15–0.32), indicating that populations from these habitats present a lower ability to respond in the direction of selection. The same pattern was observed for conditional evolvability (Figure 5B), with Araucaria forest population presenting the lowest values of conditional evolvability (mean = 0.03, 95% CI = 0.02–0.04), followed by savanna (mean = 0.07, 95% CI: 0.04–0.09), and ecological stress areas (mean = 0.08, 95% CI: 0.05–0.10).
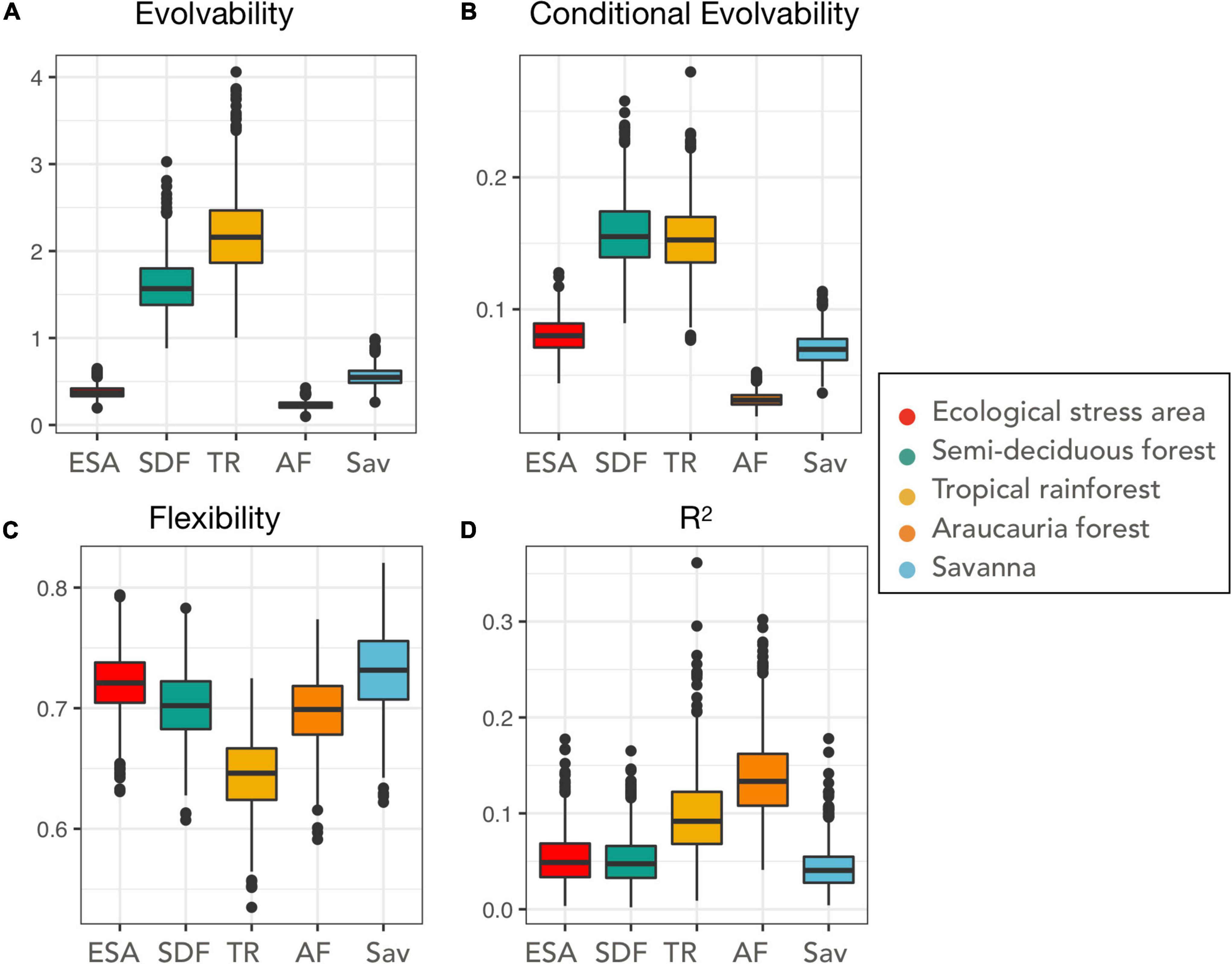
Figure 5. Evolutionary potential results. Each box represents a given metric for a given habitat, estimated from 100 resampled covariance matrices using MonteCarlo resampling. (A) Evolvability, (B) conditional evolvability, (C) flexibility, (D) R2. The bar represents the median of the metric estimate, boxes represent 50% distribution, whiskers 75% and points are outliers estimates. ESA, ecological stress areas; SDF, semi-deciduous forest; TR, tropical forest; AF, Araucaria forest, Sav, savanna.
The values for the other two metrics, flexibility and r2 (Figures 5C,D), were similar across populations. Populations from all vegetation types presented similar patterns of flexibility to respond to selection. Ecological stress areas (mean = 0.72, 95% CI: 0.66–0.77), semi-deciduous forests (mean = 0.70, 95% CI: 0.63–0.75), Araucaria forests (mean = 0.69, 95% CI: 0.64–0.75), savanna populations (mean = 0.73 95% CI: 0.65–0.79), and tropical rainforests (mean = 0.64, 95% CI: 0.58–0.70) had similar flexibility indexes, as their confidence intervals of populations from different habitats overlap. Finally, populations from different vegetation types had similar r2 values, with populations from tropical rainforests and Araucaria forests having higher overall correlation among traits (rainforest: mean = 0.09, 95% CI: 0.03–0.18; Araucaria: mean = 0.13, 95% CI: 0.06–0.22), while populations from ecological stress areas (mean = 0.05, 95% CI: 0.01–0.12), semi-deciduous forests (mean = 0.05, 95% CI: 0.01–0.11) and savannas (mean = 0.04, 95% CI: 0.01–0.09) presented lower overall correlation among traits. The r2 index for all populations overlapped in their confidence intervals.
Discussion
The Brazilian Atlantic Forest is a biodiversity hotspot, holding high levels of endemism in its reduced and fragmented area (Myers et al., 2000). Thus, understanding the distribution in this fragmented landscape of morphological diversity and the evolutionary potential of a key seed disperser has important implications: it helps us to elucidate a mechanism through which habitat loss and fragmentation erodes diversity, it can shed light on the conservation status of this species, and it will help us to predict the ability of the forest to both resist and regenerate in future environmental scenarios. Specifically, we investigated differences in morphological attributes of a widely distributed frugivorous bird species from the Brazilian Atlantic Forest, the blue manakin, which occurs in different habitat types. We also investigated how the relationship – variance and covariance – among these morphological traits might influences the potential of this species’ populations, occurring in different habitats types, to respond to selection. We found that blue manakins from different vegetation types have only slightly different average morphologies, while occupying different portions of the morphospace. Despite the relatively similar morphology, we found that populations at different vegetation types present strikingly different patterns of evolutionary potential, which might impact their ability to respond to selection in a changing environment.
Our results show that the most different population in mean morphological attributes is the population of Araucaria forests. Araucaria forest is a high elevation formation from the southern part of the Atlantic Forest (500–1,600 m above sea-level) (Ribeiro et al., 2011), characterized by mixed vegetation of conifers and broad-leaved trees. It has been shown that fruit diameter decreases toward higher elevation in the Atlantic Forest (Almeida-Neto et al., 2008), which coincides with our observation that blue manakins in the Araucaria forest are smaller in all beak traits – the ones directly associated with fruit consumption (Wheelwright, 1985) – but not in body size. Even if we have not tested whether our observed pattern of smaller beaks in Araucaria forests is due to local adaptation or simply generated by drift or plasticity, the association of smaller beaks with smaller fruits indicates the potential role of local adaptation, reinforcing the importance of understanding the potential of these traits for seed dispersal and their potential to continue to respond to further selection. It is important to note that because morphological functional traits tend to be shaped more strongly by selection over genetic drift, and because they present a complex genetic basis, the effective population size of these populations tend to have a small effect in the overall phenotypic variance (as long as there is no genetic inbreeding, which is the case here Francisco et al., 2007). Geographical morphological variation is a well-documented pattern in different kinds of organisms (Schluter and Grant, 1984; Hereford, 2009) and disentangling the specific mechanisms responsible for this morphological variation, either plasticity, genetic drift and/or local adaptation, is a hard task beyond the scope of our work. But more importantly, the documented pattern, irrespective of the specific mechanism that generated it, show that there are conspicuous morphological differences among different vegetation types, which might influence how those different populations interact with other species. This observed pattern has consequences to persistence and ecosystem services. Moving forward, it would be important to investigate at a finer geographical scale resolution whether the reported patterns are replicated in different sites, especially, the patterns observed for Araucaria Forest, since our sampling for this particular habitat all come from the same site.
Intra-populational variation has important consequences at the community level, since the higher the population-level variance, the higher its potential to interact with other species and with the ecosystem in different ways (Bolnick et al., 2011). Inter-individual differences in how seed dispersers interact with plants can be associated with individuals’ sex, body size, behavior or individual specialization, all of which with pervasive consequences for plant communities as individuals may prefer specific plant traits, and disperse different quantities of seeds to different distances and locations (Zwolak, 2018). In fact, seemingly generalist populations can be composed of specialist individuals feeding on different resources (Pires and de Melo, 2020). Because morphology is an important factor shaping frugivory at the species level (Dehling et al., 2016), and species are aggregates of individuals - the true interacting unit, morphology could also be an important factor shaping interactions within populations (Jung, 1992; Guimarães, 2020). Therefore, despite the similar manakin trait averages observed across vegetation types, the distinct levels of morphological variation across populations (Figures 4, 5A) could lead to conspicuous differences in the patterns of frugivory and seed dispersal across manakin populations. Therefore, if morphology shapes individual-level interactions, we expect the manakin populations in savanna, Araucaria forests and ecological stress areas (smallest occupation of the morphospace, Figure 4) to be able to disperse a narrower diversity of plant morphologies within and across species than those in areas of rainforest.
The potential to respond to selection is essential for populations occurring in ecosystems and natural environments under change (Milot et al., 2020). Understanding how adaptive potential is distributed across populations of a given species will enable us to predict their resilience to ongoing and future threats. We used several metrics intended to gauge different aspects of the evolutionary potential in blue manakins. First, we investigated total evolvability – a measure of the amount of phenotypic (co)variance (Hansen and Houle, 2008) – in the four traits analyzed. Populations from Araucaria forests, ecological stress areas and savannas presented a much lower evolvability when compared to the other habitats. The same pattern was observed for conditional evolvability with populations from these three areas (ecological stress area, Araucaria forests and savanna) presenting lower values than other habitat types. The rationale behind conditional evolvability is that if a population is at equilibrium most traits will be adapted to current conditions, so that a correlated trait under selection will have a constrained response due to the stabilizing selection in the other traits (Hansen et al., 2019). Therefore, evolvability and conditional evolvability capture the generation-to-generation potential of populations to respond to selection. Response to any selective pressure is contingent on genetic variation, and the lower phenotypic variance presented by manakins in these three habitats may indicate lower resilience to sustained selective pressures, increasing extinction risk (Bürger and Lynch, 1995).
The second set of evolutionary potential metrics – flexibility and r2 – measures how tight are the correlations among different traits (Figure 1C vs. Figure 1D) (Olson and Miller, 1958). Tighter correlations imply a biased response to selection, as this response will be deflected in the direction of the correlation, which is not necessarily the direction of selection (Schluter, 1996). These biases can lead to maladaptation in a generation-to-generation response, which can further hamper the potential of a given population to track evolutionary optima, and have profound impacts on the macroevolutionary patterns of a clade (Marroig and Cheverud, 2005; Machado, 2020). The higher overall integration (r2) – i.e., the tighter correlation among traits – was observed for birds in the Araucaria and tropical rainforests. Even if the differences were not as extreme as the observed for evolvability metrics, an interesting pattern emerges. The lower evolvability combined with the high overall trait correlation (r2 index) observed for Araucaria forest populations, suggests a highly constrained population in its evolutionary potential, reinforcing its inability to respond to selection. We can therefore expect the lowest resilience and the higher maladaptation rates to fluctuating selection for this Araucaria population of C. caudata. Contrastingly, ecological stress areas and savanna which also presented low levels of evolvability, presented looser correlations among traits (low r2). This pattern suggests the population of individuals inhabiting savannas and ecological stress areas lacks overall variance in all traits (low evolvability), irrespective of the correlation among traits. The response to selection is therefore constrained in all directions due to lack of trait variance, and not constrained to a given morphospace direction [the line of least evolutionary resistance, (Schluter, 1996)]. Finally, flexibility, the alignment to a given selection gradient, was similar for all populations.
All the evolutionary potential metrics were estimated using random selective pressures instead of a known direction of selection. We chose this approach in order to mimic two potential processes that might threaten these different populations. First, climate change is increasing the frequency and intensity of extreme events (IPCC, 2021), which indicates that populations will experience a wide variation of different selective pressures more frequently through time. There is evidence that selection gradients in nature tend to vary spatially (Siepielski et al., 2013) and temporally (Morrissey and Hadfield, 2012), indicating that fluctuating selective pressures might be common. Moreover, even if these populations are subjected to selection in a consistent direction in the next generations, it is impossible to know which specific direction of selective pressure these populations will have to endure. Also importantly, we used phenotypic variation in our analyses and, as in any complex quantitative trait, the morphological traits used in this study are influenced both by genetic factors and environmental variation (Walsh and Lynch, 2018). Therefore, in order to understand how organisms will evolutionarily respond, it will be important to disentangle genetic from environmental sources of trait variation in future studies.
A general pattern in tropical rainforest land cover, reproduced in the Atlantic Forest, is that older-growth forests are being replaced by fragmented secondary forests under ecological stress conditions (Laurance, 2013; Rosa et al., 2021). As a result, we can expect the evolvability in blue manakins – low in ecological stress areas – to diminish and, with that, their potential to respond to novel selective pressures. As evolvability captures the amount of phenotypic (co)variance, its decrease may also affect the ecological role of manakins, by decreasing the diversity of seeds blue manakins can disperse, leading to an even faster degradation of the environment (Zwolak, 2018). Our study, therefore, brings to light the effects of habitat loss and fragmentation on biodiversity which could not be understood by simply looking at species occurrences in a given area.
Conclusion
Our study highlights the importance of investigating morphological variation across a given species distribution, focusing not only on the mean trait but also in the patterns of variance and covariance. This has conservation implications since the potential to respond to selection affects not only the species being studied but also its ability to perform ecosystem services. Future work should try to disentangle the plastic and genetic components of the differences in covariance and quantify the cascading effects that changes in evolutionary potential can have on community dynamics.
Data availability statement
The original contributions presented in this study are included in the article/Supplementary material, further inquiries can be directed to the corresponding author.
Ethics statement
Ethical review and approval was not required for the animal study because this study used publicly available data on the bird species analyzed.
Author contributions
AA, MG, and PG designed the study. AA and PG performed the analyses. AA and KM wrote the first draft. All authors contributed to the writing.
Funding
AA was supported by FAPESP (2016/14277-2) and CAPES; KM was supported by FAPESP (2019/21732-6); PG was supported by CNPq (307134/2017-2) and FAPESP (2018/14809-0); and the Royal Society, London (CHL R1 180156). MG was supported by CNPq and FAPESP fellowships.
Acknowledgments
The authors would like to thank Pamela Santana, Irina Birskis-Barros, and Carolina Dracxler for comments in previous drafts of this manuscript, and members of the Guimarães lab for thoughtful discussions.
Conflict of interest
The authors declare that the research was conducted in the absence of any commercial or financial relationships that could be construed as a potential conflict of interest.
Publisher’s note
All claims expressed in this article are solely those of the authors and do not necessarily represent those of their affiliated organizations, or those of the publisher, the editors and the reviewers. Any product that may be evaluated in this article, or claim that may be made by its manufacturer, is not guaranteed or endorsed by the publisher.
Supplementary material
The Supplementary Material for this article can be found online at: https://www.frontiersin.org/articles/10.3389/fevo.2022.804138/full#supplementary-material
References
Almeida-Neto, M., Campassi, F., Galetti, M., Jordano, P., and Oliveira-Filho, A. (2008). Vertebrate dispersal syndromes along the Atlantic forest: broad-scale patterns and macroecological correlates. Glob. Ecol. Biogeogr. 17, 503–513. doi: 10.1111/j.1466-8238.2008.00386.x
Assis, A. P. A., Rossoni, D. M., Costa, B. M. A., Melo, D., and Marroig, G. (2016b). “Modularity and Integration,” in Encyclopedia of Evolutionary Biology, ed. R. M. Kliman (Amsterdam: Elsevier), 34–40.
Assis, A. P. A., Patton, J. L., Hubbe, A., and Marroig, G. (2016a). Directional selection effects on patterns of phenotypic (co)variation in wild populations. Proc. R. Soc. B Biol. Sci. 283:20161615. doi: 10.1098/rspb.2016.1615
Barbosa, K. V., de, C., Knogge, C., Develey, P. F., Jenkins, C. N., and Uezu, A. (2017). Use of small Atlantic Forest fragments by birds in Southeast Brazil. Perspect. Ecol. Conserv. 15, 42–46. doi: 10.1016/j.pecon.2016.11.001
Bolnick, D. I., Amarasekare, P., Araújo, M. S., Bürger, R., Levine, J. M., Novak, M., et al. (2011). Why intraspecific trait variation matters in community ecology. Trends Ecol. Evol. 26, 183–192. doi: 10.1016/j.tree.2011.01.009
Bürger, R., and Lynch, M. (1995). Evolution and extinction in a changing environment: a quantitative-genetic analysis. Evolution 49, 151–163. doi: 10.1111/j.1558-5646.1995.tb05967.x
Cheverud, J. M., and Marroig, G. (2007). Comparing covariance matrices: random skewers method compared to the common principal components model. Genet. Mol. Biol. 30, 461–469. doi: 10.1590/S1415-47572007000300027
Crestani, A. C., Mello, M. A. R., and Cazetta, E. (2019). Interindividual variations in plant and fruit traits affect the structure of a plant-frugivore network. Acta Oecol. 95, 120–127. doi: 10.1016/j.actao.2018.11.003
de Oliveira, F. B., Porto, A., and Marroig, G. (2009). Covariance structure in the skull of Catarrhini: a case of pattern stasis and magnitude evolution. J. Hum. Evol. 56, 417–430. doi: 10.1016/j.jhevol.2009.01.010
Dehling, D. M., Jordano, P., Schaefer, H. M., Böhning-Gaese, K., and Schleuning, M. (2016). Morphology predicts species’ functional roles and their degree of specialization in plant–frugivore interactions. Proc. R. Soc. B 283:20152444. doi: 10.1098/rspb.2015.2444
Eklöf, A., Jacob, U., Kopp, J., Bosch, J., Castro-Urgal, R., Chacoff, N. P., et al. (2013). The dimensionality of ecological networks. Ecol. Lett. 16, 577–583. doi: 10.1111/ele.12081
Fitzpatrick, S. W., Gerberich, J. C., Kronenberger, J. A., Angeloni, L. M., and Funk, W. C. (2015). Locally adapted traits maintained in the face of high gene flow. Ecol. Lett. 18, 37–47. doi: 10.1111/ele.12388
Francisco, M. R., Gibbs, H. L., Galetti, M., Lunardi, V. O., and Junior, P. M. G. (2007). Genetic structure in a tropical lek-breeding bird, the blue manakin (Chiroxiphia caudata) in the Brazilian Atlantic Forest. Mol. Ecol. 16, 4908–4918. doi: 10.1111/j.1365-294X.2007.03519.x
Fricke, E. C., and Svenning, J.-C. (2020). Accelerating homogenization of the global plant–frugivore meta-network. Nature 585, 74–78. doi: 10.1038/s41586-020-2640-y
Galetti, M., Guevara, R., Côrtes, M. C., Fadini, R., Von Matter, S., Leite, A. B., et al. (2013). Functional extinction of birds drives rapid evolutionary changes in seed size. Science 340, 1086–1090. doi: 10.1126/science.1233774
Guimarães, P. R. (2020). The Structure of Ecological Networks Across Levels of Organization. Annu. Rev. Ecol. Evol. Syst. 51, 433–460. doi: 10.1146/annurev-ecolsys-012220-120819
Hansbauer, M. M., Storch, I., Leu, S., Nieto-Holguin, J.-P., Pimentel, R. G., Knauer, F., et al. (2008). Movements of neotropical understory passerines affected by anthropogenic forest edges in the Brazilian Atlantic rainforest. Biol. Conserv. 141, 782–791. doi: 10.1016/j.biocon.2008.01.002
Hansen, T. F., and Houle, D. (2008). Measuring and comparing evolvability and constraint in multivariate characters. J. Evol. Biol. 21, 1201–1219. doi: 10.1111/j.1420-9101.2008.01573.x
Hansen, T. F., Solvin, T. M., and Pavlicev, M. (2019). Predicting evolutionary potential: a numerical test of evolvability measures. Evolution 73, 689–703. doi: 10.1111/evo.13705
Hasui, É, da Gomes, V. S. M., Kiefer, M. C., Tamashiro, J., and Silva, W. R. (2009). Spatial and seasonal variation in niche partitioning between blue manakin (Chiroxiphia caudata) and greenish schiffornis (Schiffornis virescens) in southeastern Brazil. Stud. Neotrop. Fauna Environ. 44, 149–159. doi: 10.1080/01650520903381729
Hereford, J. (2009). A Quantitative Survey of Local Adaptation and Fitness Trade-Offs. Am. Nat. 173, 579–588. doi: 10.1086/597611
IPCC (2021). “Summary for Policymakers,” in Climate Change 2021: The Physical Science Basis. Contribution of Working Group I to the Sixth Assessment Report of the Intergovernmental Panel on Climate Change, eds V. P. Masson-Delmotte, A. Zhai, S. L. Pirani, C. Connors, S. Peìan, N. Berger, et al. (Cambridge: Cambridge University Press).
Jordano, P. (2000). “Fruits and Furgivory,” in Seeds: The Ecology Of Regeneration In Plant Communities, ed. M. Fenner (Wallingford: CABI Publ), 125–166. doi: 10.1371/journal.pone.0119609
Jung, R. E. (1992). Individual Variation in Fruit Choice by American Robins (Turdus migratorius). Auk 109, 98–111. doi: 10.2307/4088270
Lande, R. (1979). Quantitative genetic analysis of multivariate evolution, applied to brain:body size allometry. Evolution 33, 402–416. doi: 10.1111/j.1558-5646.1979.tb04694.x
Laurance, W. F. (2013). “Emerging Threats to Tropical Forests,” in Treetops at Risk: Challenges of Global Canopy Ecology and Conservation, eds M. Lowman, S. Devy, and T. Ganesh (New York, NY: Springer), 71–79. doi: 10.1007/978-1-4614-7161-5_5
Levey, D. J. (1987). Seed Size and Fruit-Handling Techniques of Avian Frugivores. Am. Nat. 129, 471–485. doi: 10.1086/284652
Machado, F. A. (2020). Selection and Constraints in the Ecomorphological Adaptive Evolution of the Skull of Living Canidae (Carnivora, Mammalia). Am. Nat. 196, 197–215. doi: 10.1086/709610
Marroig, G., and Cheverud, J. M. (2001). A Comparison of Phenotypic Variation and Covariation Patterns and the Role of Phylogeny, Ecology, and Ontogeny During Cranial Evolution of New World Monkeys. Evolution 55, 2576–2600. doi: 10.1111/j.0014-3820.2001.tb00770.x
Marroig, G., and Cheverud, J. M. (2005). Size as a line of least evolutionary resistance: diet and adaptive morphological radiation in New World monkeys. Evolution 59, 1128–1142.
Marroig, G., Shirai, L. T., Porto, A., de Oliveira, F. B., and De Conto, V. (2009). The Evolution of modularity in the mammalian skull II: evolutionary consequences. Evol. Biol. 36, 136–148. doi: 10.1007/s11692-009-9051-1
Milot, E., Béchet, A., and Maris, V. (2020). The dimensions of evolutionary potential in biological conservation. Evol. Appl. 13, 1363–1379. doi: 10.1111/eva.12995
Morrissey, M. B., and Hadfield, J. D. (2012). Directional Selection in Temporally Replicated Studies Is Remarkably Consistent. Evolution 66, 435–442. doi: 10.1111/j.1558-5646.2011.01444.x
Myers, N., Mittermeier, R. A., Mittermeier, C. G., da Fonseca, G. A. B., and Kent, J. (2000). Biodiversity hotspots for conservation priorities. Nature 403, 853–858. doi: 10.1038/35002501
Olson, E. C., and Miller, R. L. (1958). Morphological Integration. Chicago: The University Of Chicago Press.
Pigot, A. L., Bregman, T., Sheard, C., Daly, B., Etienne, R. S., and Tobias, J. A. (2016). Quantifying species contributions to ecosystem processes: a global assessment of functional trait and phylogenetic metrics across avian seed-dispersal networks. Proc. R. Soc. B. 283:20161597. doi: 10.1098/rspb.2016.1597
Pires, L. P., and de Melo, C. (2020). Individual–resource networks reveal distinct fruit preferences of selective individuals from a generalist population of the Helmeted Manakin. Ibis 162, 713–722. doi: 10.1111/ibi.12794
Rezende, C. L., Scarano, F. R., Assad, E. D., Joly, C. A., Metzger, J. P., Strassburg, B. B. N., et al. (2018). From hotspot to hopespot: an opportunity for the Brazilian Atlantic Forest. Perspect. Ecol. Conserv. 16, 208–214. doi: 10.1016/j.pecon.2018.10.002
Ribeiro, M. C., Martensen, A. C., Metzger, J. P., Tabarelli, M., Scarano, F., and Fortin, M.-J. (2011). “The Brazilian Atlantic Forest: A Shrinking Biodiversity Hotspot,” in Biodiversity Hotspots: Distribution and Protection of Conservation Priority Areas, eds F. E. Zachos and J. C. Habel (Berlin: Springer), 405–434. doi: 10.1007/978-3-642-20992-5_21
Ribeiro, M. C., Metzger, J. P., Martensen, A. C., Ponzoni, F. J., and Hirota, M. M. (2009). The Brazilian Atlantic Forest: how much is left, and how is the remaining forest distributed? Implications for conservation. Biol. Conserv. 142, 1141–1153. doi: 10.1016/j.biocon.2009.02.021
Rodrigues, R. C., Hasui, É, Assis, J. C., Pena, J. C. C., Muylaert, R. L., Tonetti, V. R., et al. (2019). ATLANTIC BIRD TRAITS: a data set of bird morphological traits from the Atlantic forests of South America. Ecology 100:e02647. doi: 10.1002/ecy.2647
Rosa, M. R., Brancalion, P. H. S., Crouzeilles, R., Tambosi, L. R., Piffer, P. R., Lenti, F. E. B., et al. (2021). Hidden destruction of older forests threatens Brazil’s Atlantic Forest and challenges restoration programs. Sci. Adv. 7:eabc4547. doi: 10.1126/sciadv.abc4547
Rossoni, D. M., Assis, A. P. A., Giannini, N. P., and Marroig, G. (2017). Intense natural selection preceded the invasion of new adaptive zones during the radiation of New World leaf-nosed bats. Sci. Rep. 7, 1–11. doi: 10.1038/s41598-017-08989-6
Rossoni, D. M., Costa, B. M. A., Giannini, N. P., and Marroig, G. (2019). A multiple peak adaptive landscape based on feeding strategies and roosting ecology shaped the evolution of cranial covariance structure and morphological differentiation in phyllostomid bats. Evolution 73, 961–981. doi: 10.1111/evo.13715
Schluter, D. (1996). Adaptive Radiation Along Genetic Lines of Least Resistance. Evolution 50, 1766–1774. doi: 10.2307/2410734
Schluter, D., and Grant, P. R. (1984). Ecological Correlates of Morphological Evolution in a Darwin’s Finch, Geospiza difficilis. Evolution 38, 856–869. doi: 10.2307/2408396
Shirai, L. T., and Marroig, G. (2010). Skull modularity in neotropical marsupials and monkeys: size variation and evolutionary constraint and flexibility. J. Exp. Zool. Part B Mol. Dev. Evol. 314, 663–683. doi: 10.1002/jez.b.21367
Siepielski, A. M., Gotanda, K. M., Morrissey, M. B., Diamond, S. E., DiBattista, J. D., and Carlson, S. M. (2013). The spatial patterns of directional phenotypic selection. Ecol. Lett. 16, 1382–1392. doi: 10.1111/ele.12174
Teplitsky, C., Tarka, M., Møller, A. P., Nakagawa, S., Balbontín, J., Burke, T. A., et al. (2014). Assessing Multivariate Constraints to Evolution across Ten Long-Term Avian Studies. PLoS One 9:e90444. doi: 10.1371/journal.pone.0090444
Terborgh, J., Robinson, S. K., Parker, T. A., Munn, C. A., and Pierpont, N. (1990). Structure and Organization of an Amazonian Forest Bird Community. Ecol. Monogr. 60, 213–238. doi: 10.2307/1943045
Tuff, K. T., Glidden, C. K., Melbourne, B. A., Meyers, J. A., Nix, H. A., Sarre, S. D., et al. (2019). Shrinking skinks: lizard body size declines in a long-term forest fragmentation experiment. Landsc. Ecol. 34, 1395–1409. doi: 10.1007/s10980-019-00853-4
Walsh, B., and Lynch, M. (2018). Evolution and Selection of Quantitative Traits. New York, NY: Oxford University Press.
Warzecha, D., Diekötter, T., Wolters, V., and Jauker, F. (2016). Intraspecific body size increases with habitat fragmentation in wild bee pollinators. Landsc. Ecol. 31, 1449–1455. doi: 10.1007/s10980-016-0349-y
Wheelwright, N. T. (1985). Fruit-Size, Gape Width, and the Diets of Fruit-Eating Birds. Ecology 66, 808–818. doi: 10.2307/1940542
Wilson, A. J., Réale, D., Clements, M. N., Morrissey, M. M., Postma, E., Walling, C. A., et al. (2010). An ecologist’s guide to the animal model. J. Anim. Ecol. 79, 13–26. doi: 10.1111/j.1365-2656.2009.01639.x
Keywords: seed dispersal, evolutionary potential, functional morphology, Atlantic Forest, Chiroxiphia caudata, individual variation
Citation: Assis APA, Galetti M, Maia KP and Guimarães PR Jr (2022) Reduced evolutionary potential of a frugivorous bird species in fragmented forests. Front. Ecol. Evol. 10:804138. doi: 10.3389/fevo.2022.804138
Received: 28 October 2021; Accepted: 06 July 2022;
Published: 16 August 2022.
Edited by:
Eliana Cazetta, Universidade Estadual de Santa Cruz, BrazilReviewed by:
Etsuko Nonaka, University of Jyväskylä, FinlandHernani Oliveira, Federal University of Paraná, Brazil
Copyright © 2022 Assis, Galetti, Maia and Guimarães. This is an open-access article distributed under the terms of the Creative Commons Attribution License (CC BY). The use, distribution or reproduction in other forums is permitted, provided the original author(s) and the copyright owner(s) are credited and that the original publication in this journal is cited, in accordance with accepted academic practice. No use, distribution or reproduction is permitted which does not comply with these terms.
*Correspondence: Ana Paula A. Assis, YW5hcGF1bGEuYXByaWdpb2Fzc2lzQHVtb250YW5hLmVkdQ==