- 1Centre d’Écologie Fonctionnelle et Évolutive (CEFE), Université de Montpellier, CNRS, EPHE, IRD, Montpellier, France
- 2Université de Lyon, UJM-Saint-Etienne, CNRS, LBVpam UMR 5079, Saint-Etienne, France
Among air pollutants, tropospheric ozone (O3) is one of the most stressful for organisms due to its strong oxidative potential. For instance, high ozone concentration ([O3]) has the potential to affect (i) the emission of volatile organic compounds (VOCs) by plants and (ii) the lifetime of these VOCs in the atmosphere, and consequently disturb crucial signals in the interactions between plants and other organisms. However, despite the determinant role of VOCs emitted by flowers for pollinator attraction, a very limited number of studies have investigated the impact of O3 on floral VOCs. In this study, we investigated the effect of high [O3] episodes on the VOCs emitted by a flowering Mediterranean plant: the true lavender (Lavandula angustifolia Mill., Lamiaceae). To do so, in controlled conditions, we exposed (i) the entire plant to high but realistic [O3] (200 ppb for 5 h) and (ii) only the VOCs emitted by lavender to increasing [O3] (0, 40, 80, 120, and 200 ppb). We sampled VOCs of lavender in both conditions and analyzed them by Gas Chromatography-Mass Spectrometry in order to qualify and quantify the flowering lavender’s emissions and the reaction of VOCs with O3 in the atmosphere. Our results showed that exposure to high [O3] during a short period (5 h) did not affect the emission of VOCs by flowering lavender. Incidentally, we also showed that the chemical signal varied in quantities and proportions over the day. Moreover, we showed that after their emission by the plant, composition of the VOCs changed quantitatively and qualitatively in an atmosphere containing [O3] naturally observed nowadays. Quantities of several of the major terpenes emitted by lavender decreased drastically during O3 exposure, whereas concentrations of some VOCs increased, such as carbonyls and carboxylic acids, which are probably reaction products of terpenes with O3. Exposure to high [O3] thus directly affected the proportions of VOCs in the atmosphere. Because pollinators generally use a blend of VOCs in particular proportions as a signal to localize flowers, the numerous pollinators of lavender may experience difficulty in recognizing specific floral odors during frequent and moderate [O3] episodes in the Mediterranean region.
Introduction
Since the beginning of the industrial era, anthropic activities have impacted ecosystems and their functioning (Barnosky et al., 2012). The main consequences are habitat fragmentation, climate change and the pollution of soils, water, and the atmosphere. Atmospheric pollution consists in increased concentrations of primary pollutants, such as carbon dioxide or nitrogen oxides (NOx), released mainly by industry and agriculture. These pollutants are involved in complex chemical reactions in the troposphere that lead to the formation of secondary pollutants (Jenkin and Clemitshaw, 2000). Among these, tropospheric ozone (O3) is one of the most important pollutants in terms of its contribution to global warming (Sicard et al., 2017; Yeung et al., 2019). Ozone is formed by the reaction between UV light and NOx, the latter generally released by human activities in cities, but O3 tends to accumulate in the countryside, because of complex processes involving wind, altitude and NOx concentration. Thus, ozone concentration ([O3]) varies in space and, as its formation is correlated with UV light, it also varies in time, with [O3] being generally highest during mid-day in summer (Cape, 2008; Sicard et al., 2017; Young et al., 2018). Currently, in many Northern-hemisphere countries, the mean daily [O3] reaches a minimum of 40 parts per billion (ppb) with numerous O3 pollution episodes during summer. Between 1900 and 2000, the mean [O3] already increased by 30 ppb in the Northern hemisphere (Vingarzan, 2004; Ashmore, 2005) and some atmospheric chemistry models predict a further increase of about 5 ppb on average at the horizon of 2050 considering changes in climate with no control on the precursors source (Fowler et al., 2008). Due to its strong oxidative potential, O3 is very stressful for organisms and causes damage to human health, notably respiratory problems (Gryparis et al., 2004; Nuvolone et al., 2018). In plants, physiological injuries have been reported, such as leaf lesions (Long and Naidu, 2002), but also decreased vegetative growth and reproductive output (Black et al., 2007), or the inhibition of activities of PEPc and Rubisco carboxylases and of photosynthetic pigments (chlorophylls a and b) (Leitao et al., 2007). As a consequence, European directives (Directive 2008/50/EC, 2008 of the European parliament and of the council of 21 May 2008 on ambient air quality and cleaner air for Europe) have set an alert threshold of 120 ppb of exposure to O3 over 1 h for human health, beyond which emergency sanitary measures must be taken. For plants, the target value is set to 18,000 μg m–3 h–1 (900 ppb) of AOT40 (Accumulated Ozone over a Threshold of 40 ppb between 8:00 and 20:00 from April to August) in order to prevent physical and physiological injuries. Surprisingly, these thresholds do not take into account the effect of O3 on other characteristics of plants, such as the emission of volatile organic compounds (VOCs). However, VOCs are the foundation of plants’ chemical communication with their environment. For instance, plants produce them to communicate with other plants (Ueda et al., 2012), to protect themselves against pathogens, to repel herbivores and also to attract pollinators, which are crucial for plant reproduction (Dudareva et al., 2013; Abbas et al., 2017). Several studies have investigated the effect of O3 on the emission of VOCs by vegetative parts of plants (see reviews by Yuan et al., 2009; Holopainen and Gershenzon, 2010; Loreto and Schnitzler, 2010). These studies hypothesize that O3 exposure induces emission of terpenes by leaves (to an extent varying among species) because their high antioxidant potential could protect the plants from oxidative stress (Peñuelas and Staudt, 2010; Pinto et al., 2010; Bourtsoukidis et al., 2012). In addition, studies have been conducted on the effect of O3 on the lifetime of VOCs in the atmosphere (Atkinson and Arey, 2003a,b) and on the attraction of associated herbivorous insects (Fuentes et al., 2013; Li et al., 2016). Compared to plant-herbivore interactions, studies of the effect of O3 on signal recognition in plant-pollinator interactions are limited.
Only in the last 10 years have investigations been conducted on the impact of O3 on the emission of floral VOCs and on their lifetime in the atmosphere. However, flowers are well-known to emit VOCs to attract pollinators and ensure the plant’s reproduction, and their VOCs play a major role in structuring plant-pollinator interactions (Raguso, 2008b; Dudareva et al., 2013; Burkle and Runyon, 2019; Kantsa et al., 2019). In fact, as for the vegetative parts of the plant, exposure to increased O3 levels could modify the emission of some floral VOCs (Saunier and Blande, 2019) and/or reduce the lifetime of VOCs in the atmosphere, including those attractive to pollinators (McFrederick et al., 2009; Farré-Armengol et al., 2016). Because reaction time of the different VOCs with O3 is extremely variable (Atkinson and Arey, 2003a), during periods with elevated O3 levels significant changes in the proportions of VOCs are expected as soon as VOCs are emitted in the atmosphere. As the proportions of VOCs are crucial for the specific recognition of the floral chemical signal by pollinators (Raguso, 2008a; Proffit et al., 2020), these changes can reduce pollinator attraction to their host plants (Farré-Armengol et al., 2016). McFrederick et al. (2008) and Fuentes et al. (2016) showed by modeling under different scenarios of O3 pollution that the scent plume downwind of floral sources could be degraded and as a result increase honeybee foraging time. More recently, an experimental study conducted in the field reported a reduction in the number of pollinating insects of several families and their rate of flower visits in an environment enriched in O3 (90 ppb < [O3] < 120 ppb) (Ryalls et al., 2022). Authors of this study concluded that these changes were caused by the reactions of floral VOCs with O3 resulting in an alteration of the pollinators’ perceptions of the chemical signal emitted by the plants.
In the Mediterranean region, background [O3] is high and O3 episodes, i.e., short periods with high [O3], are frequent in summer due to high levels of both sunlight and human activities. For instance, in summer 2003 in the French Mediterranean region, a peak of 200 ppb O3 was registered (Vautard et al., 2005). Several Mediterranean flowering plants bloom during this same period. For instance, the true lavender (Lavandula angustifolia Mill.) is omnipresent in Mediterranean landscapes and flowers from the beginning of June to the end of July, depending on the altitude. It is a nectariferous plant that attracts a large number of pollinators and associated insects (e.g., predators) (Benachour, 2017). The censuses that we carried out on various fields of cultivated lavender show up to 140 different species of arthropods, including numerous species of bumblebees, solitary bees, butterflies, honeybees and Diptera (Nicolè, pers. comm.) (Li et al., 2019). It grows as a wild species but is also widely cultivated for its essential oil and for the production of honey by beekeepers (Barbier, 1963), creating a real olfactory landscape in Provence (McGregor, 1976). Moreover, behavioral experiments in controlled conditions, showed that essential oil extracts of L. angustifolia can act as plant signals, for example by significantly attracting honeybees (Apis mellifera) or repelling other insects (Li et al., 2019; Ganassi et al., 2020). The VOCs emitted by L. angustifolia inflorescences are well-known and include 50–90 molecules, mostly terpenes, dominated by linalyl acetate, linalool, 1,8-cineole, camphor, and borneol (Li et al., 2019; Stierlin et al., 2020; Héral et al., 2021). The reaction constants of some of these VOCs with O3 have already been described, e.g., linalool 1,8-cineole or camphor (Atkinson and Arey, 2003b). The results of these studies strongly suggest that during O3 episodes, the VOCs emitted from flowering lavender should react at different speed with O3, reducing differently their concentration, thereby affecting their relative proportions.
In this study we aimed to test to what extent exposure to high but realistic O3 levels can alter (i) lavender emissions of VOCs and (ii) the concentration and proportions of lavender VOCs once they are emitted in the atmosphere. To do so, in order to simulate a high [O3] episode in controlled conditions, we exposed flowering lavenders to 200 ppb for 5 h, collected VOCs surrounding the plant by dynamic headspace, and analyzed them by Gas Chromatography-Mass Spectrometry (GC-MS). In order to characterize the effect of O3 on the VOCs themselves, we isolated lavender VOCs in a reactor, exposed them to different [O3] (0, 40, 80, 120, and 200 ppb) for 30 min, collected the blend of VOCs in the reactor and analyzed them by GC-MS.
Materials and Methods
Study System
The true lavender, Lavandula angustifolia Miller (Lamiaceae), is a plant from the Mediterranean region (Upson et al., 2004; Passalacqua et al., 2017). Here we used clones of L. angustifolia cv. “Diva” from the Centre Régionalisé Interprofessionnel d’Expérimentation en Plantes à Parfum, Aromatiques et Médicinales (CRIEPPAM, Manosque, France). One-year-old plants were grown outdoors in individual pots in an NPK (nitrogen, phosphorus, potassium) 15 :15 :15 soil, from July 2018 at the Terrain d’Expériences, a technical platform of the Laboratoire d’Expertises Centre Méditerranéen Environnement et Biodiversité (LabEx CeMEB) located at the Centre d’Écologie Fonctionnelle et Évolutive (CEFE, Montpellier, France). In June 2020, we exposed 3-year-old plants to high O3 levels (experiment 1) and in June 2021, we used 4-year-old plants to expose only the VOCs to different O3 levels (experiment 2). The emissions of controlled plants were compared and proved to be similar between 3− and 4-year-old plants. In each experiment we used plants at the same flowering stage, when at least half of the flowers in the inflorescences were open.
Experiment 1: Volatile Organic Compounds Sampling of Flowering Lavender Exposed to High O3 Level
Lavender plants were exposed to O3 in greenhouses at the Terrain d’Expériences in which the temperature was controlled and fixed at 25°C in the day and 15°C during the night under natural light conditions (15 h/9 h day/night periods).
In order to expose the entire flowering plant to O3 and monitor the VOCs, a dynamic enclosure system was designed. Plants were individually enclosed in a 42 L inert and odorless PTFE (polytetrafluoroethylene) frame closed by a 50 μm thick PTFE film (Figure 1A). Air was pushed using a PTFE pump (KNF, N816.1.2K.18®, Germany) through a charcoal filter into the chambers at 6 L min–1. A PTFE fan ensured air mixing in the chambers. Air flow rates were controlled by flowmeters and all tubing lines were made of PTFE. Plants were bagged at least 12 h before the treatment phase to allow plants to acclimate to the enclosure. Exposure was always conducted on pairs of plants: the first plant was exposed to 0 ppb O3 and represented the control and the other plant was maintained at 200 ppb O3 using an O3 generator-analyzer (UV photometric Ozone Analyser with a generator option, Model 49i, Thermo Fisher Scientific, Franklin, MA, United States) for 5 h between 10:00 and 15:00, corresponding to the time of greatest pollinator activity in the fields (Nicolè, pers. comm.). For both plants, VOCs contained in the chambers were collected four times: (t0) before starting to ozonate the treated plant (0 ppb of O3 in both chambers); (t1) 3 h after the beginning of generation of O3 for the ozonated plant; (t2) 5 h after the beginning of generation of O3 for the ozonated plant; and (t3) between 30 min and 1 h after O3 generation was stopped (Figure 1B). VOCs were collected by pushing the air out of the chambers at 200 mL min–1 through an adsorbent steel cartridge acting as VOCs trap filled with 40 mg of Carbotrap and 80 mg of Tenax phases (Sigma-Aldrich, Munich, Germany). We collected VOCs of each plant of the pair for 10 min following the same protocol. In addition, before introducing the plant into the chambers, to assess background scent level in the chambers, we collected, using the same method, blank samples, i.e., samples of air within empty chambers. Indeed, in semi-controlled experiments with whole plants, despite the fact that the air injected into the sampling chambers is purified with activated charcoal, exogenous compounds, which are considered as contaminants, are always present in the samples, and therefore must be removed from the plant samples (see methods in Palmqvist et al., 2021; Rupp et al., 2021). At the end of each O3 exposure in the first experiment, inflorescences and leaves were cut from the plant and placed for 4 days at 40°C in an oven to measure the dry biomass. Ten pairs of plants were used, representing 20 individuals in total for this first experiment.
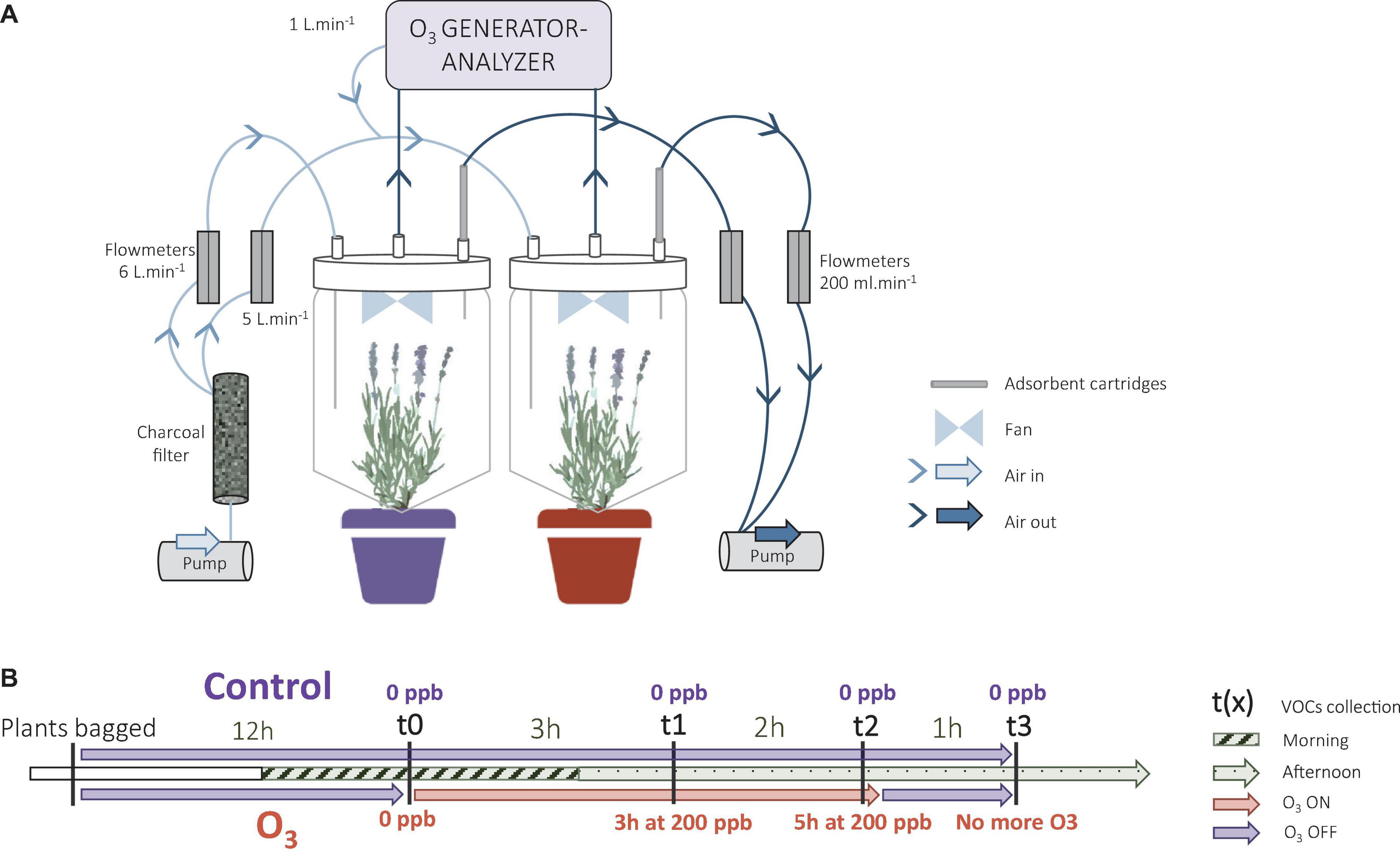
Figure 1. Schematic diagram of (A) the experimental set-up and (B) timeline used for flowering lavender exposure to high O3 level in experiment 1. Control lavender plants, represented by the purple color in the figure, were exposed to 0 ppb O3 during the entire experiment. Treated lavender plants, represented by the red color in the figure, were exposed to 200 ppb of O3 from t1 to t2 (red pot). After t2, O3 generation was stopped and 30 min to 1 h later the VOCs were collected in the chamber. t times correspond to collections of VOCs through adsorbent cartridges during 10 min (gray bars in panel A) in the two chambers.
Experiment 2: Sampling of Flowering Lavender Volatile Organic Compounds Exposed to Different [O3]
In order to evaluate the effect of O3 exposure on VOCs only, after their emission by the plant, we sequentially but randomly exposed lavender VOCs to 40, 80, 120, or 200 ppb of O3 (Figure 2A). Based on the results of experiment 1, experiments were conducted between 11:00 and 18:00 because variations of VOC estimated concentrations and proportions over time were limited during this period (see results section “Experiment 1: Effects of Exposure to High O3 Level on the Emission of VOCs by Flowering Lavender”). The same device described in experiment 1 was used to enclose the flowering lavender plant. This time, the set-up was installed in a room where the temperature (26.36 ± 0.041°C) and light were controlled (artificial light 400–700 nm SpectraBulb, LED E27, FloraLed, France) to better characterize the effect of O3 on lavender VOCs and avoid temperature- and light-related odor variations. Air purified by a charcoal filter was pushed into the chamber at 2 L min–1. The air charged with VOCs present in the chamber was sent into a glass reactor of 2 L (Hei-Vap Core model, Heidolph Instruments GmbH & Co. KG, Regensburg, Germany) at a flow rate of 400 mL min–1 using the same O3 generator/analyzer as in the experiment 1 that analyzed at 1.4 L min–1 the [O3] at the outlet of the reactor (Figure 2A). This very same device generated 40, 80, 120, and 200 ppb at a flow rate of 1.8 L min–1 at the inlet of the reactor. Respectively, 20, 50, 80, and 110 ppb were measured at the outlet of the reactor by a second O3 analyser (Model 106-L Ozone monitor, 2B Technologies, Boulder, CO, United States) at 850 mL min–1 (Figure 2A). The difference in [O3] between the inlet and the outlet of the reactor confirmed that O3 was reacting with VOCs in the reactor (loss of ∼50% of the [O3], but a loss of only ∼25% when only O3 was present in the reactor).
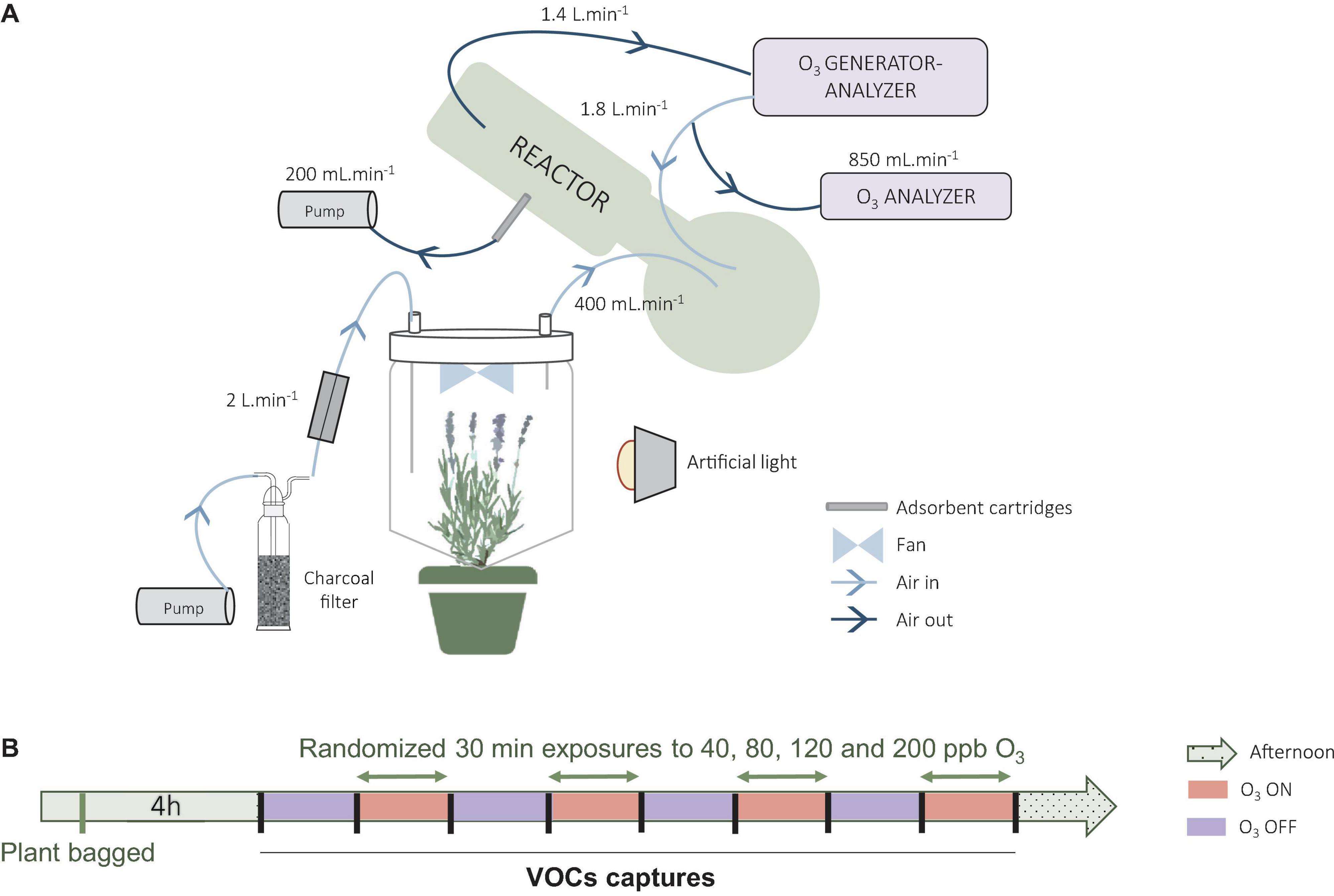
Figure 2. Schematic diagram of (A) the experimental set-up presenting the exposure of flowering lavender volatile organic compounds (VOCs) to different [O3] and (B) timeline of the exposure for experiment 2. We present the temporal scheme showing the 4-hour control period at the beginning followed by alternating ozone periods (red) and control periods (purple). Each period was 30 min and VOC samples were collected through adsorbent cartridges (gray bars in panel A) at the end of each period during 10 min (including controls and ozone exposure).
Each O3 phase lasted 30 min and was always preceded by a control exposure phase of 30 min (0 ppb of O3 in the reactor, Figure 2B). Plants were bagged at least 4 h before the treatment phase. VOCs in the reactor were collected for 10 min using the adsorbent traps containing Carbotrap/Tenax and analyzed by GC-MS for control (20 samples) and O3 conditions (20 samples: N = 5 per [O3] > 0 ppb). This experiment was conducted over 5 days with a different lavender plant every day (N = 5 plants for this second experiment). Each day, plant VOCs were exposed to the five [O3] and the order of the different concentrations used was randomized among the days.
Chemical Analyses
All chemical analyses were performed at the Plateforme d’Analyses Chimiques en Ecologie (PACE technical platform of the Labex CeMEB).
Analyses by GC-MS were conducted using the method detailed in Proffit et al. (2020). A gas chromatograph (GC, Trace 1310, Thermo Fisher Scientific, Milan, Italy) coupled to a mass spectrometer (ISQ QD Single Quadrupole, Thermo Fisher Scientific, Milan, Italy) was used with an Optima 5-MS capillary column (30 m, 0.25 mm ID, 0.25 μm film thickness, Machery-Nagel, Düren, Germany). Adsorbent traps were handled with a Multi Purpose Sampler (Gerstell, Mülheim, Germany) and desorbed with a double stage desorption system, composed of a Thermal Desorption Unit (TDU) and a Cold Injection System (CIS) (Gerstell, Mülheim, Germany). First, the injector was splitless with a temperature of 250°C on the CIS trap cooled at −80°C by liquid nitrogen. Then, the CIS trap was heated to 250°C with a 1:4 split ratio to inject the compounds into the column. We used helium at 1 mL min–1 as a carrier gas. Oven temperature was held at 40°C for 3 min, increased from 40 to 220°C at a rate of 5°C min–1 and from 220 to 250°C at 10°C min–1, and finally held for 2 min at 250°C. The temperatures of the transfer line and the ion source of the mass spectrometer were 250 and 200°C, respectively. The acquisition was at 70 eV ionization energy, from 38 to 350 m/z. Xcalibur™ 266 software (Thermo Fisher Scientific, Milan, Italy) was used for data processing. Retention times of a series of n-alkanes (Alkanes standard solution, Sigma Aldrich, Munich, Germany) were used to convert retention times into retention indices. Compounds were then identified based on matching of the mass spectra using Total Ion Current mass chromatogram (TIC) with a database (NIST 2007 MS library, Wiley 9th edition), and on confirmation by comparison of calculated retention index (RI) with the Adams (2007) library and, for some VOCs, with matching of their mass spectra and retention time with those of synthetic compounds using the same analytic method. Volatiles detected only in the empty chamber or reactor (blank controls) were considered contaminants and were removed from the samples.
In order to evaluate the concentrations of the different VOCs, peak areas of VOCs were integrated using TIC and calibration factors were calculated with the same spectral method. To do so, we prepared a stock solution in dichloromethane (Pestinorm, >99.8%) of four major VOCs of lavender, each of them at a mean concentration of 9.66 ± 0.8 μg/μL: linalyl acetate (Sigma-Aldrich, Analytical standard), camphor (Fluka, >99%), 1,8-cineole (Sigma-Aldrich, ∼99%) and linalool (Fluka, >99%). In order to have calibration in the concentration range of the VOCs detected in lavender samples, dilutions of 1:1,000, 1:400, and 1:200 of this stock solution were prepared in dichloromethane. For each of the solutions, 1 μL was injected in the adsorbent trap, with three replicates per solution, and analyzed by GC-MS using the same method as for the lavender samples. Calibration factors were obtained for each VOC using the slope of the regression between their peak area (integrated from TIC) and their concentration in the three replicates of the four dilutions, setting the intercept to zero. Because we do not have a reference standard for all VOCs of the lavender samples and we do not have clear expectations about which ones represent a signal for interacting species, we used the mean calibration factor of these four compounds to estimate the concentrations of all compounds present in our samples.
For experiment 1, estimated concentration (ng/min/g of dry biomass) of each VOC was calculated. For experiment 2, in order to evaluate the quantitative variation of the different VOCs after O3 exposure compared to the corresponding control (0 ppb) we calculated a relative index of variation following the formula:
“area of VOC[O3]i” stands for the peak area of a given compound at a given [O3] i (i: from 0 to 200 ppb), “area of VOC[O3]0” stands for the peak area of a given compound in the sample of the corresponding control (0 ppb of O3). The variation index varied between −1 and 1.
Statistical Analyses
All the data visualization and analyses were performed in R (v. 3.6.2; R Development Core Team)1 and required the following packages: ade4 (Thioulouse et al., 2018), car (Fox and Weisberg, 2019), vegan (Oksanen et al., 2020), mixOmics (Rohart et al., 2017), Hotelling (Curran, 2018), RVAideMemoire (Hervé, 2021), nlme (Pinheiro et al., 2021), contrast (O’Callaghan et al., 2020), and corrplot (Wei and Simko, 2021).
Experiment 1: Effects of Exposure to High O3 Level on the Emission of Volatile Organic Compounds by Flowering Lavender
Estimated concentrations (ng/min/g of dry biomass) and relative proportions in the total bouquet of each compound were calculated and set into two distinct data tables.
To assess the effect of O3 on the concentration of each compound, but also on the total estimated concentration of all VOCs, we fitted linear mixed models on their log-transformed quantity with treatment (control or ozone), time (t0, t1, t2, and t3) and their interaction as fixed factors and the pair of plants as random factor. Analyses of deviance allowed testing the significance of effects of these factors on concentration of each VOC. Then, pairwise comparisons were made between modalities of the factors using contrasts.
If significant variations of VOC estimated concentrations were detected between the control and treated plants, we also evaluated the effect of O3 on the total estimated concentration of VOCs of lavender plants with a linear model with treatment, time and their interaction as fixed factors and the pair of plants as random factor. Data were log-transformed prior to the analysis to normalize the distribution of the residuals. An analysis of deviance was used in order to test the significance of effects of these factors on the plants’ total emission. Then, contrasts were conducted between modalities of the factors to reveal true differences.
In addition, variations in the relative proportions of VOCs in the overall odor bouquet were tested using partial redundancy analyses (pRDA) (Hervé et al., 2018). This multivariate analysis consisted of two steps. Firstly, a multivariate linear regression on the relative proportions of VOCs with treatment, time and their interaction, was performed with pair of plants as a random factor. Secondly, a constrained Principal Component Analysis (PCA) on the fitted values of the regression model was conducted in order to evaluate the variation in the relative proportions of VOCs that was explained by the two factors and their interaction. In addition, a permutation test was performed to assess the significance of the effects of the fixed factors. Finally, pairwise comparisons were conducted between the modalities of the factors and p-values were adjusted with the “false discovery rate” (fdr) method (Benjamini and Hochberg, 1995).
Experiment 2: Effects of Different [O3] on Flowering Lavender Volatile Organic Compounds
To assess the effect of [O3] (0, 40, 80, 120, and 200 ppb) on the variation index of each compound, we fitted linear models with [O3] as fixed factor and the plant as random factor. An analysis of deviance was conducted to test the significance of the effect of [O3] on the variation index. Then, pairwise comparisons were made between modalities of this factor using contrast.
In order to highlight possible relationships between the different VOCs in terms of degradation-formation with O3, we performed a correlation matrix between each pair of compounds based on Pearson’s correlation test with scaled peak areas of VOCs ozonated at all [O3].
The effect of [O3] on the relative proportions of VOCs in the reactor was tested using a pRDA, with plants as a random factor. A permutation test was performed to assess the global effect of [O3] but also pairwise comparisons between modalities of the factor; p-values were adjusted with the “fdr” method (Benjamini and Hochberg, 1995).
Results
Experiment 1: Effects of Exposure to High O3 Level on the Emission of Volatile Organic Compounds by Flowering Lavender
In the volatile emission of flowering lavender, we detected a total of 34 VOCs, seven of which were present only as traces (see Table 1 and Supplementary Figure 1). As expected, monoterpenes were the family of compounds most represented in terms of concentrations and number of compounds.
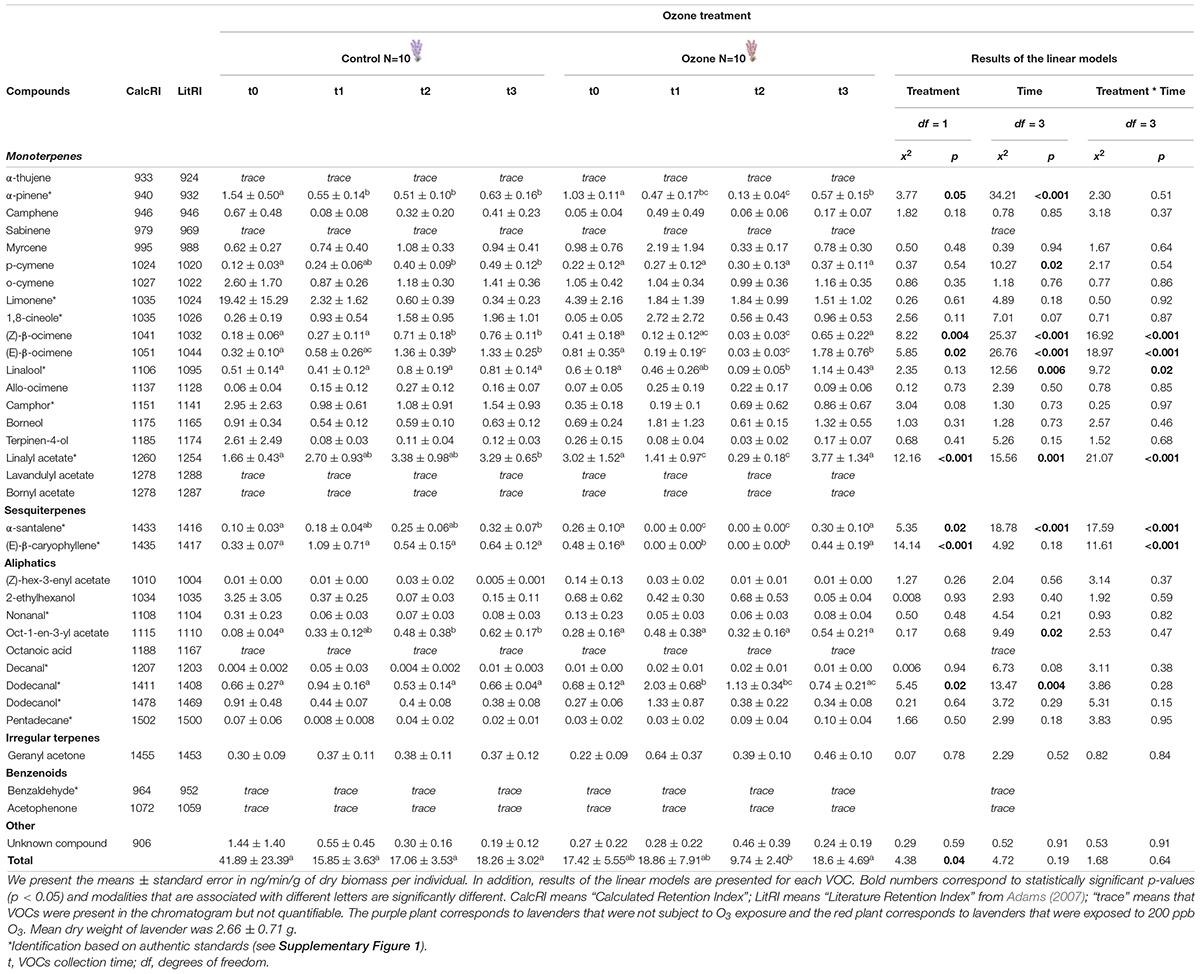
Table 1. Effects of the O3 treatment and time on the estimated concentrations of the different VOCs detected in experiment 1. For each modality of the different factors, see Figure 1.
Volatile Organic Compounds Estimated Concentrations and Proportions in Control Conditions
The control conditions (0 ppb) provided information about the expected chemical profile of L. angustifolia in normal conditions without ozone exposure. For all control samples (N = 40), limonene was the major compound (control sample mean ± SE: 24.4 ± 15.49% of the total of VOCs emitted and 22.68 ± 6.88 ng/min/g of dry biomass), owing to its high estimated concentration at t0 (19.42 ± 15.29 ng/min/g of dry biomass), followed by linalyl acetate (11.9 ± 6.7% of the total VOCs emitted by control plants and 2.76 ± 0.58 ng/min/g of dry biomass), which dominated the odor bouquet of all the control samples except at t0. Camphor (7.05 ± 0.74%), o-cymene (6.53 ± 0.74%) and 1,8-cineole (5.1 ± 3.38%) were the other most abundant compounds, each of them being present at around 1.45 ± 0.18 ng/min/g of dry biomass on average (Table 1). Each of the other VOCs were present at less than 6% on average of the total blend.
Effects of Ozone Treatment and Time of Day on Volatile Organic Compounds Estimated Concentrations
Both O3 treatment and time of day affected the estimated concentrations of emitted VOCs. Of the 34 VOCs in the chamber, concentrations of 10 VOCs were significantly affected by one or both of these factors (linear models, at least one significant p-value, i.e., p < 0.02, per VOC, Table 1). Significant decreases in the estimated concentrations of monoterpenes [α-pinene, (Z) and (E)-β-ocimene, linalool, linalyl acetate, and p-cymene] and of two sesquiterpenes [α-santalene and (E)-β-caryophyllene] were observed when the plants were exposed to 200 ppb O3 for 3 h or 5 h (p ≤ 0.02) (Table 1). The concentration of only one compound, dodecanal, increased significantly when plants were exposed to 200 ppb O3 for 3 h (“ozone:t1”) compared to the corresponding control (“control:t1”) (p = 0.02). There were no significant differences between the estimated concentrations of different VOCs of lavender plants that after a 5 h exposure were no longer ozonated (“ozone:t3”), and the corresponding control plants (“control:t3”).
Incidentally, this experiment also showed, apparently for the first time, that emission of VOCs by flowering lavender varied over the course of the day. Concentration of α-pinene decreased over the day, while estimated concentrations of p-cymene, 1,8-cineole, (Z) and (E)-β-ocimene, linalyl acetate, oct-1-en-3-yl acetate and α-santalene increased (Table 1). The total concentration of VOCs emitted by flowering lavender was affected only by the O3 treatment (p = 0.04) and not by the time of the day or the interaction of these two factors (p = 0.19 and p = 0.64, respectively, Table 1). Pairwise comparisons revealed only one significant difference: the total VOCs concentration of lavenders ozonated for 5 h (“ozone:t2”) was significantly lower than in the corresponding control (“control:t2”).
Effects of Ozone Treatment and Time of Day on the Relative Proportions of Volatile Organic Compounds
Because estimated concentrations of different VOCs were affected both by O3 exposure and by time of day, their relative proportions also changed during O3 exposure. The O3 treatment (0 vs. 200 ppb ozone), time of day (t0, t1, t2, and t3) and their interaction together explained 20% of this variability in relative proportions of VOCs, as shown by the multivariate linear regression analysis (Figure 3). The random factor “pair of plants” explained 4.9% of the variation. The relative proportions of VOCs varied significantly between O3 treatments (permutation test: F1, 71 = 4.53, p = 0.001) and among times of day (permutation test: F3, 71 = 3.04, p = 0.001), and the interaction of the two factors was also significant (permutation test: F3, 71 = 1.74, p = 0.008). Regarding diurnal variation, pairwise comparisons detected significant differences in relative proportions of VOCs emitted by lavender plants from the morning to the afternoon (from t0 to t3) between the control groups (Figure 3C). Moreover, the relative proportions of VOCs in chambers with plants exposed to O3 for 3 h (“ozone:t1”) and 5 h (“ozone:t2”) were significantly different from those in all other groups (respectively p < 0.012 and p < 0.002). The pRDA score plot from the constrained PCA (Figure 3A) shows the significant effects of ozone treatment, time of day and their interaction on the relative proportions of VOCs. A total of 82% of the constrained variance is explained by the first and the second components of the PCA (67.50 and 14.44%, respectively). As previously shown by the pairwise comparisons, three groups appear on this PCA. First, the effect of time of day can be observed on the second component, where samples collected in the morning and mid-day (t0 and t1) were completely separated from those collected in the afternoon (t2 and t3). Second, the effect of the O3 treatment is clearly observed in the first component. Samples collected when lavender plants were ozonated for 3 and 5 h (“ozone:t1” and “ozone:t2”) were positively correlated with the first component, in contrast to those collected in control chambers and with plants that were no longer ozonated (“ozone:t3”), which were negatively correlated with the first component.
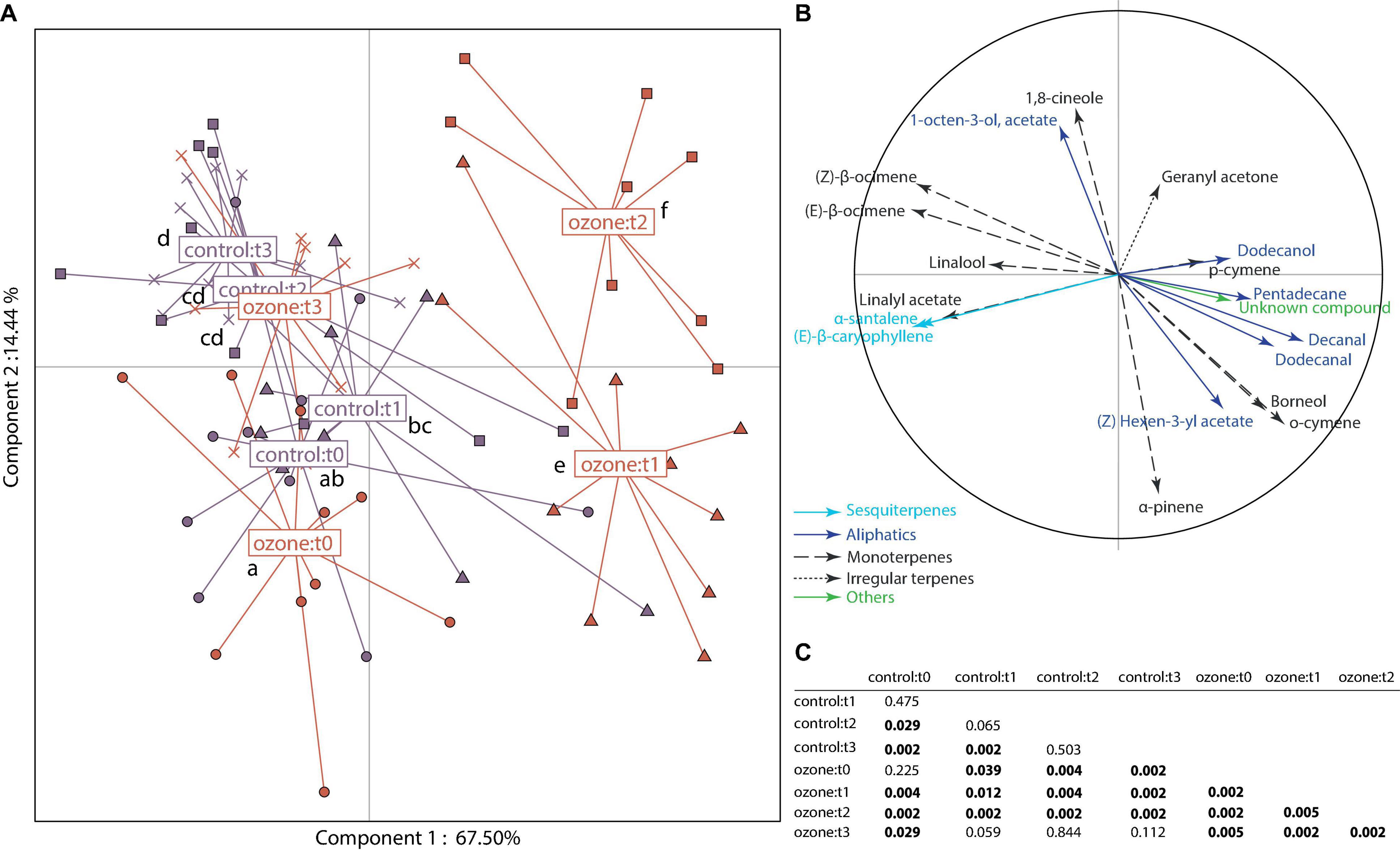
Figure 3. Partial Redundancy Analysis (pRDA) score plot from the constrained Principal Component Analysis of the relative proportions of flowering lavender volatile organic compounds (VOCs) in chambers as a function of treatment and time of day for experiment 1 (A) and the associated correlation circle (B). Red symbols on the score plot correspond to the odor bouquet of “ozone” lavenders, purple symbols correspond to that of “control” lavenders; and symbols indicate the time of the VOCs collection in the chambers (circle = t0, triangle = t1, square = t2, cross = t3). Groups with different letters next to the barycenters show statistically significant different chemical profiles, as detailed in table (C), presenting the p-values of the permutation test assessing pairwise comparisons for different modalities of the treatment and time. Significant differences (p < 0.05) are indicated in bold.
The correlation circle of the constrained PCA (Figure 3B) highlights the VOCs correlated individually with the first or the second component at the threshold of 30% and shows which compounds were affected by the different experimental conditions. Linalool, (Z)- and (E)-β-ocimene, (E)-β-caryophyllene, α-santalene, and linalyl acetate were associated with control plants and with previously exposed plants (“ozone:t3”). In contrast, dodecanol, p-cymene, decanal, dodecanal, pentadecane, an unknown compound and 2-ethylhexanol were positively correlated with the first component, suggesting that their proportions were higher in plants that sustained exposure to 200 ppb of O3 for 3 and 5 h (“ozone:t1” and “ozone:t2”). In fact, compared to control plants, in plants ozonated at 200 ppb for 5 h (“ozone:t2”), linalyl acetate was no longer the major compound in the scent bouquet, being replaced by dodecanal and limonene (Figure 4). Regarding diurnal variation, geranyl acetone, 1,8-cineole and oct-1-en-3-yl acetate were positively correlated with the second component, indicating that their proportions were higher in odor bouquets of plants sampled in the afternoon, whereas α-pinene was present in higher proportion in odor bouquets of plants sampled in the morning.
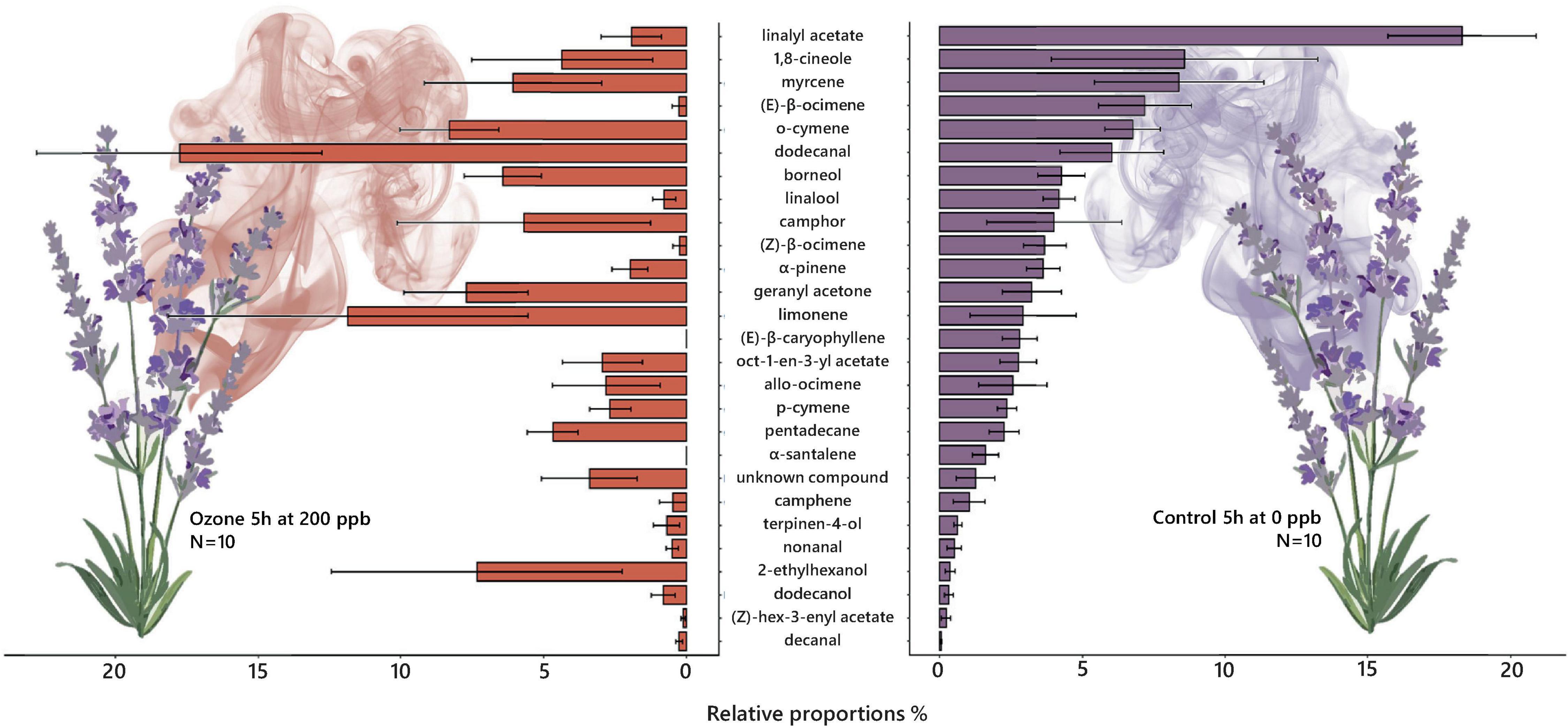
Figure 4. Relative proportions of volatile organic compounds (VOCs) emitted by flowering lavenders in experiment 1 exposed to 0 ppb O3 for 5 h (right) vs. proportions of VOCs emitted by flowering lavenders exposed to 200 ppb O3 for 5 h (left). Mean ± standard error per individual of the relative proportions of VOCs emitted by 10 plants without O3 in the chamber and by 10 plants exposed to 200 ppb O3 for 5 h.
Experiment 2: Effects of Different [O3] on Flowering Lavender Volatile Organic Compounds
In this experiment, plants were not ozonated. Only the VOCs, after their emission by the plant, were exposed to O3. We detected 35 VOCs in the reactor chamber, of which 23 could be quantified and 12 were present only as traces.
Volatile Organic Compounds Proportions in Control Conditions
In control conditions (0 ppb in the reactor), among the VOCs detected in the reactor chamber, terpenes were still the most represented family (43.94% of the total blend), with linalyl acetate being the main monoterpene, comprising 21.04 ± 15.22% of the total blend (Supplementary Table 1).
Effects of [O3] on the Quantities of Volatile Organic Compounds in the Reactor
The [O3] in the reactor significantly affected the variation index of 16 VOCs among the 23 VOCs quantified in the chemical blend (Figure 5). The remaining seven VOCs did not vary significantly with increasing [O3]: borneol (x2 = 4.92, p = 0.3), camphene (x2 = 2.48, p = 0.65), camphor (x2 = 1, p = 0.91), o-cymene (x2 = 5.88, p = 0.21), p-cymene (x2 = 8.41, p = 0.08), an unknown compound (x2 = 4.8, p = 0.31) and undecanal (x2 = 6.09, p = 0.19). Linear models revealed a significant effect of the [O3] on the variation index of VOCs. Pairwise comparisons highlighted once again significant decreases in the quantities of monoterpenes [(Z)- and (E)-β-ocimene, linalool, linalyl acetate, limonene, myrcene, α-pinene, 1,8-cineole, and bornyl acetate], of one aliphatic compound (1-octen-3-ol acetate) and of some sesquiterpenes [(E)-β-caryophyllene, α-santalene, and caryophyllene oxide] when VOCs were ozonated (Figure 5A), while estimated concentrations of dodecanal and decanal increased significantly (Figure 5B).
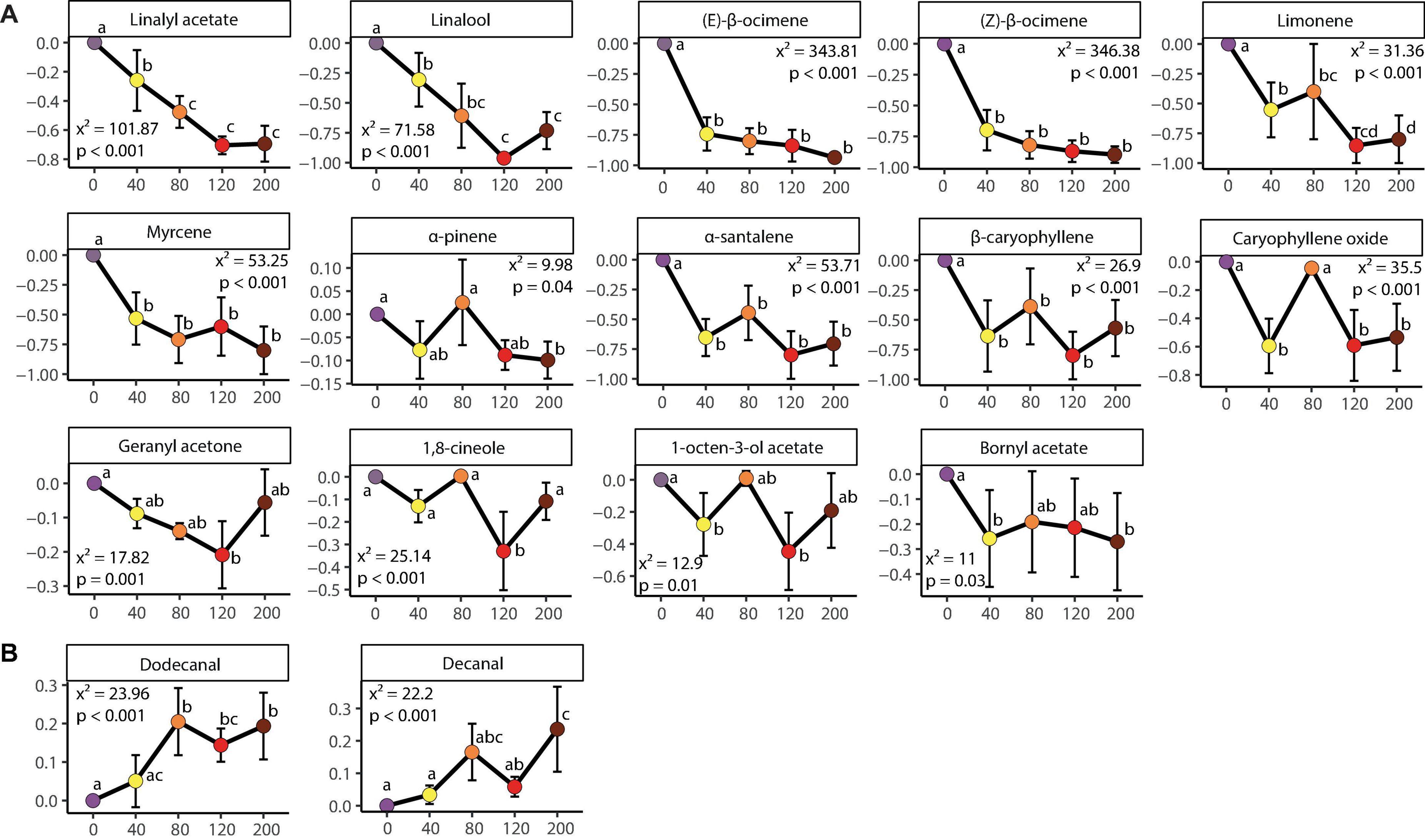
Figure 5. Effects of exposure to increasing [O3] in experiment 2 on the volatile organic compounds (VOCs) variation index (mean ± standard error). Panel (A) lists VOCs whose variation index significantly decreased with [O3], (B) panel lists VOCs whose variation index increased significantly with increasing [O3]. Purple, yellow, orange, red, and brown colors correspond, respectively to an [O3] exposure of 0, 40, 80, 120, and 200 ppb. For each VOC, results of the linear models are presented and values of the variation index with different letters indicate statistically significant difference in pairwise comparisons (p < 0.05).
Quantities of decanal, dodecanal, the unknown compound and geranyl acetone were significantly negatively correlated with quantities of some monoterpenes and sesquiterpenes of the bouquets [mostly linalyl acetate, bornyl acetate, borneol, 1-octen-3-ol acetate, (Z) and (E)-β-ocimene] (Figure 6; r < 0.4, p < 0.05). These results confirm the assumption that different VOCs emitted by plants react differentially with O3 and may form new VOCs in the odor bouquet.
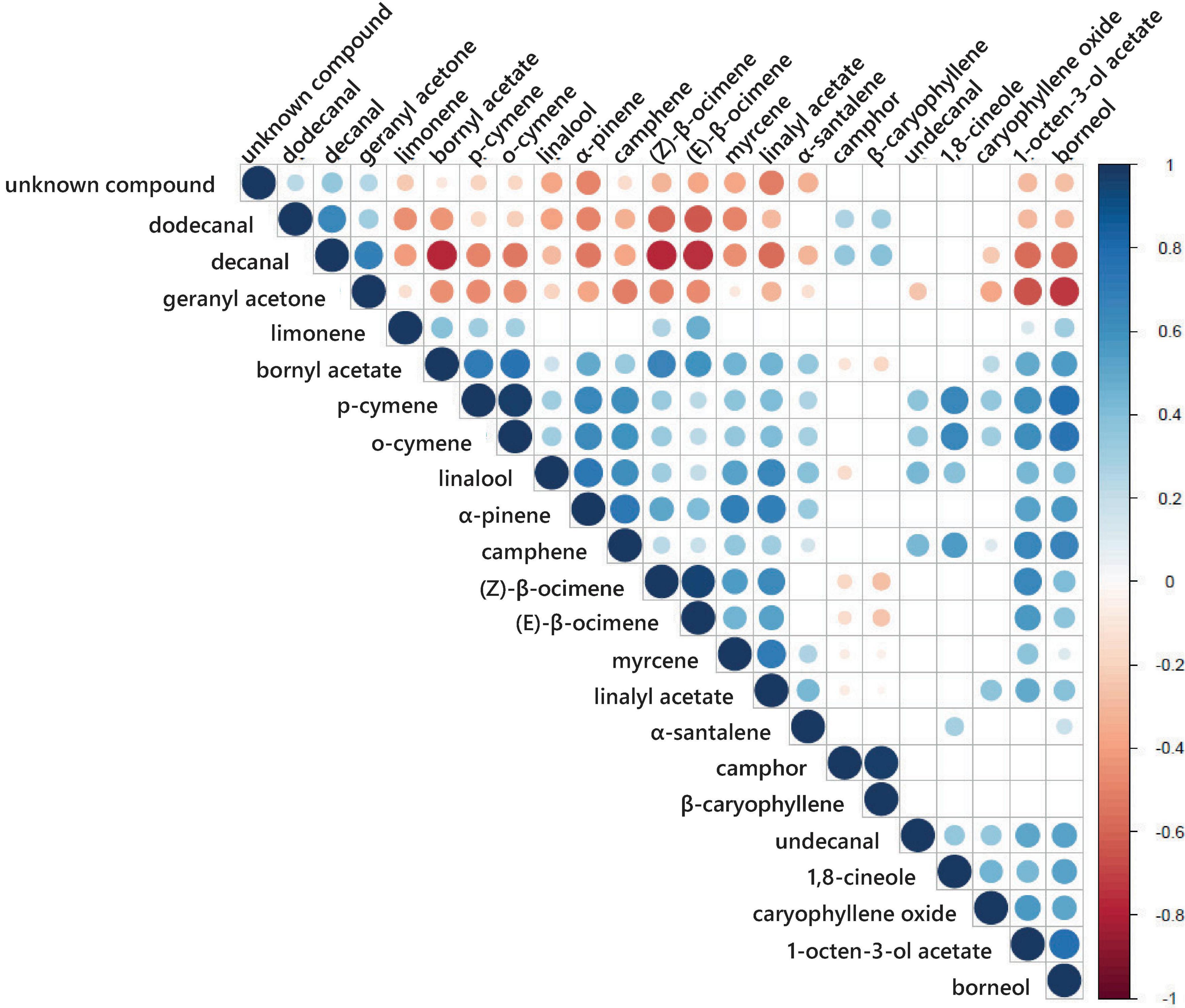
Figure 6. Visualization of the pairwise correlation matrix of the concentrations-like (peak areas) of all flowering lavender compounds during volatile organic compounds (VOCs) O3 exposure in experiment 2. Red symbols correspond to negative correlation coefficients, blue symbols correspond to positive correlation coefficients. The size of circles represents the magnitude of the value of the correlation coefficient (r). No circles means that the correlation coefficient was not significant at the threshold of 95% based on Pearson’s test.
Effects of [O3] on the Proportions of Volatile Organic Compounds in the Reactor
The [O3] in the reactor had a significant effect on variation of the relative proportions of VOCs (permutation test: F4, 16 = 5.03, p = 0.001). Twenty-nine percent of the total variation of relative proportions of VOCs was explained by the [O3] (0, 40, 80, 120, and 200 ppb) in the redundancy analysis. Forty-eight percent of this variation was explained by the random factor (plants). Pairwise comparisons showed that the relative proportions of VOCs were significantly different between the non-ozonated VOCs (0 ppb) and all the ozonated VOCs, irrespective of the [O3] applied (p < 0.04). Among the ozonated samples, the proportions of different VOCs were not significantly different among treatments (p > 0.08), except between the 40 and 200 ppb treatments (p = 0.04).
The significant effect of [O3] on the relative proportions of VOCs in the reactor is clearly illustrated in the pRDA score plot from the constrained PCA (Figure 7A). A total of 96% of the constrained variance was explained by the first and second components of the PCA (92 and 4%, respectively). The effect of [O3] can be observed on the first component, where ozonated samples were positively correlated with this component, in contrast to non-ozonated controls, which were negatively correlated. On the correlation circle of the constrained PCA, camphor, decanal, dodecanal, o-cymene, p-cymene, camphene, borneol, 1,8-cineole, geranyl acetone, α-pinene, and unknown compound were positively correlated with the first component (Figure 7B): their relative proportions were higher in the ozonated odor bouquets than in control ones (Supplementary Table 1). On the contrary, (Z)- and (E)-β-ocimene, α-santalene, (E)-β-caryophyllene, myrcene, linalool, and caryophyllene oxide were negatively correlated with the first component; their proportions were higher in the control odor bouquet than in ozonated ones (Supplementary Table 1). The second component of the pRDA clearly separates the chemical profiles as a function of the value of increasing [O3]. There was a gradient from VOCs exposed to 40 ppb with a positive score on the axis to VOCs exposed to 200 ppb with a negative score on the axis. Undecanal and 1-octen-3-ol acetate were negatively correlated with the second component and were associated with samples ozonated at high [O3] (at 80 ppb and higher values) (Figure 7B).
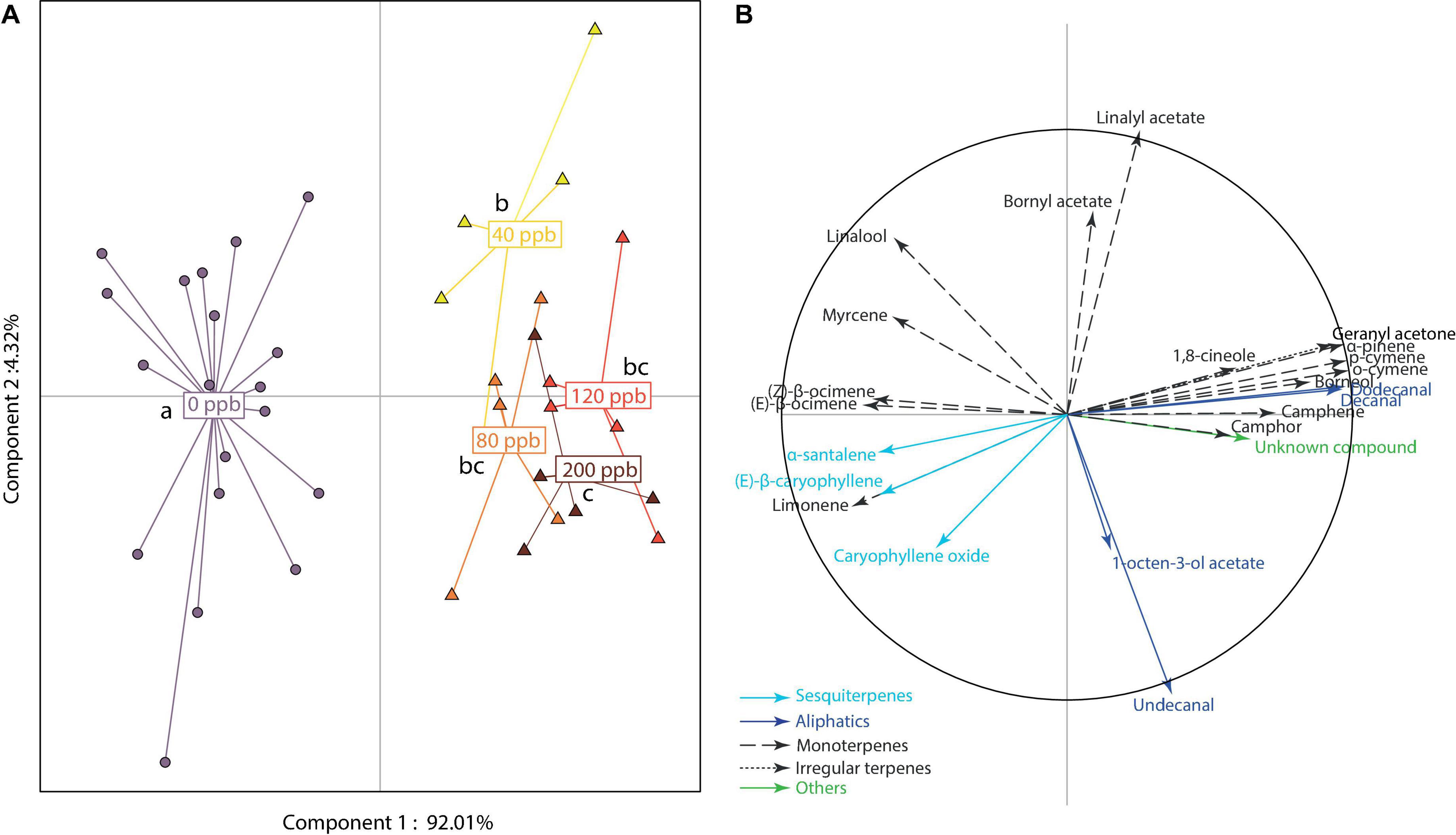
Figure 7. Partial Redundancy Analysis (pRDA) score plot from the constrained Principal Component Analysis of the relative proportions of flowering lavender volatile organic compounds (VOCs) sampled in the reactor as a function of the [O3] during experiment 2 (A) and the associated correlation circle (B). Purple, yellow, orange, red, and brown colors correspond, respectively, to an exposure of 0, 40, 80, 120, and 200 ppb O3 and symbols indicate whether the VOCs in the reactor were exposed to 0 ppb O3 (circles) or increasing [O3] (triangles). Groups with different letters next to the barycenters show statistically significant differences based on the permutation test assessing the pairwise comparisons at the threshold of 95%.
Discussion
This study expands our knowledge of VOCs emission in Lavandula angustifolia and also provides important insights on the impact of high [O3] episodes on chemical signals emitted by flowers.
In this study, we created a new experimental device allowing plants to be exposed to different [O3] during several hours under controlled conditions (light, ventilation, temperature and humidity). Our results on the control emissions attest to the validity of our method. In our study, the chemical composition of L. angustifolia cv. “Diva” included more than 30 VOCs, dominated by oxygenated monoterpenes (linalyl acetate, camphor, 1,8-cineole), other monoterpenes (myrcene, limonene, linalool, and o- and p-cymene) and sesquiterpenes [α-santalene and (E)-β-caryophyllene]. The chemical composition of our plants was similar to what has been reported in a recent review of the compounds already identified in other studies of L. angustifolia cv. “Diva” (Héral et al., 2021), except that we found a smaller number of compounds. This might be explained by the fact that in all these studies headspace collection of flowering lavender VOCs was performed immediately, or at least shortly after enclosure of the inflorescences, or VOCs were sampled in liquid extractions, thereby including some other compounds stored in the plant but not emitted by flowers (Ormeño et al., 2011). In contrast, in our study, in order to avoid measuring a handling effect (pressure on the glandular trichomes) in the chemical data coming from the release of VOCs stored in the handled tissues (Li et al., 2018), we chose to wait for 12 h after manipulating the plants to start the collection of VOCs. Even if VOCs concentrations were not calculated with full precisions, interestingly, this study highlights, to our knowledge for the first time, a variation in lavender emissions over the course of the day. Proportions and estimated concentrations of monoterpenes changed significantly from morning to afternoon: α-pinene, p-cymene, 1,8-cineole, (Z) and (E)-β-ocimene, linalyl acetate, and α-santalene increased significantly from morning to noon. A recent study documented diurnal variation in quantities of chemicals stored in different structures of L. angustifolia with the highest amount of compounds stored in flowers harvested at 15:00 (Yildirim et al., 2019). Daily emission rhythms in VOCs emission have already been shown in other plants (Zeng et al., 2017). This change in the emissions of plant VOCs could be caused by diurnal changes in abiotic conditions, e.g., light and temperature (Owen et al., 2002; Lerdau and Gray, 2003), or may be due to an internal circadian rhythm (Fenske and Imaizumi, 2016; Picazo-Aragonés et al., 2020). Since the temperature was controlled in our study, we can assume that VOCs that varied significantly in time in control conditions are compounds that are part of the daily emission rhythm or else compounds whose emissions are light-dependent. As the frequency of visitation of lavender pollinators varies over the course of the day (Nicolè, pers. comm.), it would be interesting to test if the rhythm of emission of lavender volatiles coincides with variations in pollinator foraging periods.
When we exposed the whole plant to 200 ppb O3 for 5 h, we detected a significant difference in proportions of VOCs in the chamber between the ozonated and the control lavenders. However, no difference was found between these plants when all the O3 was removed from the chamber (VOC collection ∼45 min after O3 exposure). These results, combined with the observed decrease in estimated concentrations of terpenes during O3 exposure, suggest that the changes detected are due to reaction of O3 with the VOCs in the chamber, rather than to changes in the emission of VOCs by lavenders. This suggests that either short-term O3 exposure had no effect on VOC emission by lavender, or our sampling time after O3 exposure was too short to detect a possible response in the emission of lavender VOCs. Indeed, specific organs such as trichomes can be a barrier to O3 exposure and could thus protect L. angustifolia from short but high [O3] episodes, as has been shown for other plants (Li et al., 2018). Because L. angustifolia stores VOCs in trichomes (Guitton et al., 2010) and the time between the production and the emission of stored compounds is longer than for non-stored VOCs, the effect of short-term exposure to O3 on VOCs emission from lavender might be observed after a longer periods of time than those studied here. For instance, tobacco plants exposed to 170 ppb O3 for 5 h showed a significant increase in emission of some monoterpenes by leaves 24 h after the exposure (Heiden et al., 1999). Compared to studies conducted on leaf VOCs, studies investigating the effect of O3 on floral VOCs are limited. Interestingly, it has been reported in some species of Brassicaceae that emission of some floral VOCs, such as indole and methyl salicylate, can be triggered after 5 days under high [O3] (Saunier and Blande, 2019). In our study we aimed to explore the immediate response of the plant to a short-term O3 exposure but it would thus be interesting to test the effect on L. angustifolia of a longer period of O3 exposure, for example from 24 h.
The direct effect of O3 on the VOCs was clearly confirmed by our experiment testing the result of exposure in the reactor chamber to different [O3] of the VOCs only, after their emission by the plant. Interestingly, in both experiments, when the whole plant was exposed to O3 (experiment 1) and when only VOCs were ozonated in the reactor (experiment 2), we detected a significant decrease in the estimated concentrations of terpenes such as linalool, linalyl acetate, α-pinene, and (Z)- and (E)-β-ocimene, (E)-β-caryophyllene and α-santalene, whereas other monoterpenes such as 1,8-cineole, camphor and borneol were relatively more stable during exposure to O3. In addition, when only the VOCs were ozonated there was a significant decrease in the estimated concentrations of myrcene, geranyl acetone, 1-octen-3-ol-acetate and bornyl acetate. For all these VOCs, estimated concentration decreased linearly with increasing [O3]. On the contrary, for caryophyllene oxide, the estimated concentration did not seem to follow a monotonous decrease with [O3]: it decreased significantly at 40, 120, and 200 ppb O3 but not at 80 ppb. This pattern of variation could be explained by the limited occurrence of this VOC in the blend emitted by flowering lavender and particularly the absence of this compound in most control samples and samples ozonated at 80 ppb. For the compounds detected in the volatile profile of lavender in this study, the detailed reaction kinetics with O3 have been described for only two, linalool (McFrederick et al., 2008; Bernard et al., 2012) and (E)-β-caryophyllene (Winterhalter et al., 2009; Jokinen et al., 2016). Because the oxidation of some terpenes by O3 can produce the same secondary compounds in some cases (McFrederick et al., 2008; Winterhalter et al., 2009; Bernard et al., 2012; Jokinen et al., 2016), accurate determination of the reaction kinetics of VOCs with O3 can only be approached by performing experimental oxidation studies with a single VOC. Therefore, we can only speculate on the possible degradation-formation of VOCs under O3 exposure. Besides, we found significant negative correlations between the estimated concentrations of decanal, dodecanal and the unknown compound, on the one hand, and estimated concentrations of monoterpenes [mainly (Z)- and (E)-ocimene, bornyl acetate, borneol, 1-octen-3-ol-acetate, linalyl acetate, and α-pinene], on the other under O3 exposure (even at [O3] as low as 40 ppb). These results are in accordance with chemical kinetics studies on the gas-phase terpene oxidation with O3 (Calogirou et al., 1999; Atkinson and Arey, 2003b), which report carbonyls and carboxylic acids (and sometimes alcohols, epoxides, esters, nitrates, or peroxynitrates) to be the most prevalent products resulting from these reactions.
As expected, this study highlights different reactivities of some specific lavender VOCs with O3, suggesting that O3 exposure not only alters concentrations, but also changes the proportions of compounds in the odor bouquet. Similar results were found in a study investigating the degradation of floral scent volatiles from Brassica nigra by reaction with O3 (at 80 and 120 ppb) over a distance gradient (Farré-Armengol et al., 2016). This latter study showed that supplementing air with O3 led to a reduction of the concentrations of floral volatiles in air and a change in the proportions with increasing distance from the volatile source, at [O3] as low as 80 ppb. In that study, attraction tests with Bombus terrestris revealed that attractiveness of floral scent to pollinators was reduced after exposure to 120 ppb O3. In the present study, we found an alteration in the reactor of the VOCs emitted by flowering lavender at even lower [O3] (40 ppb).
As in most studies exploring the effect of ozone exposure on VOCs and their consequences for plant-insect chemical communication (Fuentes et al., 2013; Farré-Armengol et al., 2016; Li et al., 2016), some conditions of our experiments do not represent the realistic probability of encounter of O3 with VOCs in normal atmosphere (although the [O3] we tested were within a realistic range). Nevertheless, our results are alarming. In fact, some carbonyls and carboxylic acids —decanal, dodecanol and dodecanal—appeared to be in higher concentration and/or proportion when the VOCs of lavender were ozonated. These compounds could be detected by pollinators’ antennae and could also affect their behavior. For instance, electroantennographic tests showed that bees responded less to high quantities of decanal than to lower quantities (Mas et al., 2018). This study suggested that high concentration of decanal has a repellent effect on bees but behavioral tests are now needed to address this direct effect on this pollinator attraction.
Our findings warn of the strong impact of such modifications of floral chemical signals on the communication of plants with their biotic environment, particularly pollinating insects. Pollination is a fundamental service to natural and agricultural ecosystems (Klein et al., 2007; Winfree et al., 2011). Unfortunately, limited research has been conducted on the impact of O3 on the chemical communication of plants in the context of plant-pollinator interactions, particularly in the field. Because L. angustifolia attracts different groups of insects (Li et al., 2019), which use different signals to find their host plant olfactory cues being of prime importance (Junker and Parachnowitsch, 2015), it would be of great interest to compare the recognition of non-ozonated and ozonated lavender plants by different pollinators. Differences in pollinator sensitivity to variation in plant VOCs induced by high [O3] could consequently affect the composition of insects visiting lavender plants.
Conclusion
We successfully demonstrate that the reactivity with O3 of major and ubiquitous compounds emitted by lavender plants leads to the formation of new compounds, including carbonyls and carboxylic acids, even at [O3] as low as 40 ppb, an [O3] frequently observed in summer. These modifications of individual compounds lead to variation in the proportions of VOCs within the overall bouquet. In contrast, we do not show here an immediate plant response to an O3 pollution episode affecting the emission of floral VOCs. A general issue to be addressed is whether pollinators might thereby be unable to recognize the O3-degraded scent. Here we underline the importance of studying the effects of O3 pollution on plant-insect and especially plant-pollinator interactions in the Mediterranean region. With an increasing number of studies investigating this fundamental issue, thresholds set by European directives on chronic and episodic pollution levels may need to be reviewed and revised to values that take into account scientific studies of the impact of ozone on plant-pollinator interactions.
Data Availability Statement
The raw data supporting the conclusions of this article will be made available by the authors, without undue reservation.
Author Contributions
CD, MP, and MH-M designed the experiment. CD performed the experiments and analyzed the data. CD and BB identified the chemical compounds. CD, FN, and MP wrote the first version of the article. All authors read and approved the final version.
Funding
This work was partly funded by the Montpellier Université d’Excellence (MUSE, ANR under the Investissements d’avenir program, reference ANR-16-IDEX-0006), International Research Project (IRP)-CNRS-MOST, and the French National Research Program for Environmental and Occupational Health of ANSES (reference 2018/1/138).
Conflict of Interest
The authors declare that the research was conducted in the absence of any commercial or financial relationships that could be construed as a potential conflict of interest.
Publisher’s Note
All claims expressed in this article are solely those of the authors and do not necessarily represent those of their affiliated organizations, or those of the publisher, the editors and the reviewers. Any product that may be evaluated in this article, or claim that may be made by its manufacturer, is not guaranteed or endorsed by the publisher.
Acknowledgments
We thank the PACE and the TE (LabEx CeMEB, the French National Research Agency under the Investissements d’avenir) for hosting our experiments. We thank the CRIEPPAM for providing the lavender plants and Doyle McKey (a native speaker) for English proofreading and scientific advices on the manuscript. We also thank two reviewers for their comments on the manuscript, and Michael Staudt, Elena Ormeño-Lafuente, Henri Wortham for their valuable technical advices.
Supplementary Material
The Supplementary Material for this article can be found online at: https://www.frontiersin.org/articles/10.3389/fevo.2022.795588/full#supplementary-material
Footnotes
References
Abbas, F., Ke, Y., Yu, R., Yue, Y., Amanullah, S., Jahangir, M. M., et al. (2017). Volatile terpenoids: multiple functions, biosynthesis, modulation and manipulation by genetic engineering. Planta 246, 803–816. doi: 10.1007/s00425-017-2749-x
Adams, R. P. (2007). Identification of Essential Oil Components by Gas Chromatography/Mass Spectroscopy, 4th Edn. Carol Stream, IL: Allured publishing corporation.
Ashmore, M. R. (2005). Assessing the future global impacts of ozone on vegetation. Plant Cell Environ. 28, 949–964. doi: 10.1111/j.1365-3040.2005.01341.x
Atkinson, R., and Arey, J. (2003a). Atmospheric degradation of volatile organic compounds. Chem. Rev. 103, 4605–4638. doi: 10.1021/cr0206420
Atkinson, R., and Arey, J. (2003b). Gas-phase tropospheric chemistry of biogenic volatile organic compounds: a review. Atmos. Environ. 37, 197–219. doi: 10.1016/S1352-2310(03)00391-1
Barbier, E. (1963). Les lavandes et l’apiculture dans le sud-est de la France. Ann. Abeille 6, 85–159. doi: 10.1051/apido:19630201
Barnosky, A. D., Hadly, E. A., Bascompte, J., Berlow, E. L., Brown, J. H., Fortelius, M., et al. (2012). Approaching a state shift in Earth’s biosphere. Nature 486, 52–58. doi: 10.1038/nature11018
Benachour, K. (2017). Insect visitors of lavender (Lavandula officinalis L.): comparison of quantitative and qualitative interactions of the plant with its main pollinators. Afr. Entomol. 25, 435–444. doi: 10.4001/003.025.0435
Benjamini, Y., and Hochberg, Y. (1995). Controlling the false discovery rate: a practical and powerful approach to multiple testing. J. R. Stat. Soc. Ser. B 57, 289–300. doi: 10.1111/j.2517-6161.1995.tb02031.x
Bernard, F., Daële, V., and Mellouki, A. (2012). Studies of the gas phase reactions of linalool, 6-methyl-5-hepten-2-ol and 3-methyl-1-penten-3-ol with O3 and OH radicals. J. Phys. Chem. A 116, 6113–6126. doi: 10.1021/jp211355d
Black, V. J., Stewart, C. A., Roberts, J. A., and Black, C. R. (2007). Ozone affects gas exchange, growth and reproductive development in Brassica campestris (Wisconsin Fast Plants). New Phytol. 176, 150–163. doi: 10.1111/j.1469-8137.2007.02163.x
Bourtsoukidis, E., Bonn, B., Dittmann, A., Hakola, H., Hellén, H., and Jacobi, S. (2012). Ozone stress as a driving force of sesquiterpene emissions: a suggested parameterisation. Biogeosciences 9, 4337–4352. doi: 10.5194/bg-9-4337-2012
Burkle, L. A., and Runyon, J. B. (2019). Floral volatiles structure plant–pollinator interactions in a diverse community across the growing season. Funct. Ecol. 33, 2116–2129. doi: 10.1111/1365-2435.13424
Calogirou, A., Larsen, B. R., and Kotzias, D. (1999). Gas-phase terpene oxidation products: a review. Atmos. Environ. 33, 1423–1439. doi: 10.1016/S1352-2310(98)00277-5
Cape, J. N. (2008). Surface ozone concentrations and ecosystem health: past trends and a guide to future projections. Sci. Total Environ. 400, 257–269. doi: 10.1016/j.scitotenv.2008.06.025
Curran, J. (2018). Hotelling: Hotelling’s T∧2 Test and Variants. Available online at: https://cran.r-project.org/package=Hotelling (accessed December 22, 2021).
Directive 2008/50/EC (2008). Directive 2008/50/EC of the European Parliament and of the Council of 21May 2008 on Ambient Air Quality and Cleaner Air for Europe. Luxembourg: Publications office of the European Union.
Dudareva, N., Klempien, A., Muhlemann, J. K., and Kaplan, I. (2013). Biosynthesis, function and metabolic engineering of plant volatile organic compounds. New Phytol. 198, 16–32. doi: 10.1111/nph.12145
Farré-Armengol, G., Peñuelas, J., Li, T., Yli-Pirilä, P., Filella, I., Llusia, J., et al. (2016). Ozone degrades floral scent and reduces pollinator attraction to flowers. New Phytol. 209, 152–160. doi: 10.1111/nph.13620
Fenske, M. P., and Imaizumi, T. (2016). Circadian rhythms in floral scent emission. Front. Plant Sci. 7:462. doi: 10.3389/fpls.2016.00462
Fowler, D., Amann, M., Anderson, R., Ashmore, M., Cox, P., Depledge, M., et al. (2008). Ground-Level Ozone in the 21st Century: Future Trends, Impacts and Policy Implications. London: The Royal Society.
Fox, J., and Weisberg, S. (2019). An {R} Companion to Applied Regression, 3rd Edn. Thousand Oaks CA: Sage.
Fuentes, J. D., Chamecki, M., Roulston, T., Chen, B., and Pratt, K. R. (2016). Air pollutants degrade floral scents and increase insect foraging times. Atmos. Environ. 141, 361–374. doi: 10.1016/j.atmosenv.2016.07.002
Fuentes, J. D., Roulston, T. H., and Zenker, J. (2013). Ozone impedes the ability of a herbivore to find its host. Environ. Res. Lett. 8:014048. doi: 10.1088/1748-9326/8/1/014048
Ganassi, S., Cascone, P., Di Domenico, C., Pistillo, M., Formisano, G., Giorgini, M., et al. (2020). Electrophysiological and behavioural response of Philaenus spumarius to essential oils and aromatic plants. Sci. Rep. 10:3114. doi: 10.1038/s41598-020-59835-1
Gryparis, A., Forsberg, B., Katsouyanni, K., Analitis, A., Touloumi, G., Schwartz, J., et al. (2004). Acute effects of ozone on mortality from the “Air pollution and health: a European approach” project. ATS J. 170, 1080–1087. doi: 10.1164/rccm.200403-333oc
Guitton, Y., Nicolè, F., Moja, S., Valot, N., Legrand, S., Jullien, F., et al. (2010). Differential accumulation of volatile terpene and terpene synthase mRNAs during lavender (Lavandula angustifolia and L. x intermedia) inflorescence development. Physiol. Plant. 138, 150–163. doi: 10.1111/j.1399-3054.2009.01315.x
Heiden, A. C., Hoffmann, T., Kahl, J., Kley, D., Klockow, D., Langebartels, C., et al. (1999). Emission of volatile organic compounds from ozone-exposed plants. Ecol. Appl. 9, 1160–1167. doi: 10.1890/1051-0761(1999)009[1160:eovocf]2.0.co;2
Héral, B., Stierlin, É, Fernandez, X., and Michel, T. (2021). Phytochemicals from the genus Lavandula: a review. Phytochem. Rev. 20, 751–771. doi: 10.1007/s11101-020-09719-z
Hervé, M. (2021). RVAideMemoire: Testing and Plotting Procedures for Biostatistics. Available online at: https://cran.r-project.org/package=RVAideMemoire (accessed December 22, 2021).
Hervé, M. R., Nicolè, F., and Lê Cao, K. A. (2018). Multivariate analysis of multiple datasets: a practical guide for chemical ecology. J. Chem. Ecol. 44, 215–234. doi: 10.1007/s10886-018-0932-6
Holopainen, J. K., and Gershenzon, J. (2010). Multiple stress factors and the emission of plant VOCs. Trends Plant Sci. 15, 176–184. doi: 10.1016/j.tplants.2010.01.006
Jenkin, M. E., and Clemitshaw, K. C. (2000). Ozone and other secondary photochemical pollutants: chemical processes governing their formation in the planetary boundary layer. Atmos. Environ. 34, 2499–2527. doi: 10.1016/S1474-8177(02)80014-6
Jokinen, T., Kausiala, O., Garmash, O., Peräkylä, O., Junninen, H., Schobesberger, S., et al. (2016). Production of highly oxidized organic compounds from ozonolysis of β-caryophyllene: laboratory and field measurements. Boreal Environ. Res. 21, 262–273.
Junker, R. R., and Parachnowitsch, A. L. (2015). Working towards a holistic view on flower traits-how floral scents mediate plant-animal interactions in concert with other floral characters. J. Indian Inst. Sci. 95, 43–67.
Kantsa, A., Raguso, R. A., Lekkas, T., Kalantzi, O.-I., and Petanidou, T. (2019). Floral volatiles and visitors: a meta-network of associations in a natural community. J. Ecol. 107, 2574–2586. doi: 10.1111/1365-2745.13197
Klein, A.-M., Vaissire, B. E., Cane, J. H., Steffan-Dewenter, I., Cunningham, S. A., Kremen, C., et al. (2007). Importance of pollinators in changing landscapes for world crops. Proc. R. Soc. Biol. Sci. 274, 303–313. doi: 10.1098/rspb.2006.3721
Leitao, L., Bethenod, O., and Biolley, J. P. (2007). The impact of ozone on juvenile maize (Zea mays L.) plant photosynthesis: effects on vegetative biomass, pigmentation, and carboxylases (PEPc and Rubisco). Plant Biol. 9, 478–488. doi: 10.1055/s-2007-964942
Lerdau, M., and Gray, D. (2003). Ecology and evolution of light-dependent and light-independent phytogenic volatile organic carbon. New Phytol. 157, 199–211. doi: 10.1046/j.1469-8137.2003.00673.x
Li, H., Li, J., Dong, Y., Hao, H., Ling, Z., Bai, H., et al. (2019). Time-series transcriptome provides insights into the gene regulation network involved in the volatile terpenoid metabolism during the flower development of lavender. BMC Plant Biol. 19:313. doi: 10.1186/s12870-019-1908-6
Li, S., Tosens, T., Harley, P. C., Jiang, Y., Kanagendran, A., Grosberg, M., et al. (2018). Glandular trichomes as a barrier against atmospheric oxidative stress: relationships with ozone uptake, leaf damage, and emission of LOX products across a diverse set of species. Plant Cell Environ. 41, 1263–1277. doi: 10.1111/pce.13128
Li, T., Blande, J. D., and Holopainen, J. K. (2016). Atmospheric transformation of plant volatiles disrupts host plant finding. Sci. Rep. 6:33851. doi: 10.1038/srep33851
Long, S. P., and Naidu, S. L. (2002). “Effects of oxidants at the biochemical, cell and physiological levels, with particular reference to ozone,” in Air Pollution and Plant Life, eds J. N. B. Bell and M. Treshow (West Sussex: John Wiley & Sons, Ltd), 69–88.
Loreto, F., and Schnitzler, J. P. (2010). Abiotic stresses and induced BVOCs. Trends Plant Sci. 15, 154–166. doi: 10.1016/j.tplants.2009.12.006
Mas, F., Harper, A., Horner, R., Welsh, T., Jaksons, P., and Suckling, D. M. (2018). The importance of key floral bioactive compounds to honey bees for the detection and attraction of hybrid vegetable crops and increased seed yield. J. Sci. Food Agric. 98, 4445–4453. doi: 10.1002/jsfa.8967
McFrederick, Q. S., Fuentes, J. D., Roulston, T., Kathilankal, J. C., and Lerdau, M. (2009). Effects of air pollution on biogenic volatiles and ecological interactions. Oecologia 160, 411–420. doi: 10.1007/s00442-009-1318-9
McFrederick, Q. S., Kathilankal, J. C., and Fuentes, J. D. (2008). Air pollution modifies floral scent trails. Atmos. Environ. 42, 2336–2348. doi: 10.1016/j.atmosenv.2007.12.033
McGregor, E. S. (1976). “Crops dependent upon or benefited by insect pollination,” in Insect Pollination of Cultivated Crop Plants (Washington, DC: Agricultural Research Service United States Department of Agriculture), 238. doi: 10.5860/choice.37-2165
Nuvolone, D., Petri, D., and Voller, F. (2018). The effects of ozone on human health. Environ. Sci. Pollut. Res. 25, 8074–8088. doi: 10.1007/s11356-017-9239-3
O’Callaghan, A., Kuhn, M., Weston, S., Wing, J., Forester, J., and Thaler, T. (2020). contrast: A Collection of Contrast Methods. Available online at: https://cran.rproject.org/package=contrast (accessed December 22, 2021).
Oksanen, J., Blanchet, F. G., Friendly, M., Kindt, R., Legendre, P., McGlinn, D., et al. (2020). vegan: Community Ecology Package. R Package Version 2.5-7. Available online at: https://CRAN.R-project.org/package=vegan (accessed March 01, 2022).
Ormeño, E., Goldstein, A., and Niinemets, Ü. (2011). Extracting and trapping biogenic volatile organic compounds stored in plant species. Trends Anal. Chem. 30, 978–989. doi: 10.1016/j.trac.2011.04.006
Owen, S. M., Harley, P., Guenther, A., and Hewitt, C. N. (2002). Light dependency of VOC emissions from selected Mediterranean plant species. Atmos. Environ. 36, 3147–3159. doi: 10.1016/s1352-2310(02)00235-2
Palmqvist, B., Brazeau, H. A., and Parachnowitsch, A. L. (2021). Differences in floral scent and petal reflectance between diploid and tetraploid Chamerion angustifolium. Front. Ecol. Evol. 9:734128. doi: 10.3389/fevo.2021.734128
Passalacqua, N. G., Tundis, R., and Upson, T. M. (2017). A new species of Lavandula sect. Lavandula (Lamiaceae) and review of species boundaries in Lavandula angustifolia. Phytotaxa 292, 161–170. doi: 10.11646/phytotaxa.292.2.3
Peñuelas, J., and Staudt, M. (2010). BVOCs and global change. Trends Plant Sci. 15, 133–144. doi: 10.1016/j.tplants.2009.12.005
Picazo-Aragonés, J., Terrab, A., and Balao, F. (2020). Plant volatile organic compounds evolution: transcriptional regulation, epigenetics and polyploidy. Int. J. Mol. Sci. 21:8956. doi: 10.3390/ijms21238956
Pinheiro, J., Bates, D., DebRoy, S., Sarkar, D., and R Core Team (2021). nlme: Linear and Nonlinear Mixed Effects Models. Available online at: https://cran.r-project.org/package=nlme
Pinto, D. M., Blande, J. D., Souza, S. R., Nerg, A. M., and Holopainen, J. K. (2010). Plant volatile organic compounds (VOCs) in ozone (O3) polluted atmospheres: the ecological effects. J. Chem. Ecol. 36, 22–34. doi: 10.1007/s10886-009-9732-3
Proffit, M., Lapeyre, B., Buatois, B., Deng, X. X., Arnal, P., Gouzerh, F., et al. (2020). Chemical signal is in the blend: bases of plant-pollinator encounter in a highly specialized interaction. Sci. Rep. 10:10071. doi: 10.1038/s41598-020-66655-w
Raguso, R. A. (2008b). Wake up and smell the roses: the ecology and evolution of floral scent. Annu. Rev. Ecol. Evol. Syst. 39, 549–569. doi: 10.1146/annurev.ecolsys.38.091206.095601
Raguso, R. A. (2008a). Start making scents: the challenge of integrating chemistry into pollination ecology. Entomol. Exp. Appl. 128, 196–207. doi: 10.1111/j.1570-7458.2008.00683.x
Rohart, F., Gautier, B., Singh, A., and Lê Cao, K. A. (2017). mixOmics: an R package for ‘omics feature selection and multiple data integration. PLoS Comput. Biol. 13:e1005752. doi: 10.1371/journal.pcbi.1005752
Rupp, T., Oelschlägel, B., Rabitsch, K., Mahfoud, H., Wenke, T., Disney, R. H. L., et al. (2021). Flowers of deceptive Aristolochia microstoma are pollinated by phorid flies and emit volatiles known from invertebrate carrion. Front. Ecol. Evol. 9:658441. doi: 10.3389/fevo.2021.658441
Ryalls, J. M. W., Langford, B., Mullinger, N. J., Bromfield, L. M., Nemitz, E., Pfrang, C., et al. (2022). Anthropogenic air pollutants reduce insect-mediated pollination services. Environ. Pollut. 297:118847. doi: 10.1016/j.envpol.2022.118847
Saunier, A., and Blande, J. D. (2019). The effect of elevated ozone on floral chemistry of Brassicaceae species. Environ. Pollut. 255:113257. doi: 10.1016/j.envpol.2019.113257
Sicard, P., Anav, A., De Marco, A., and Paoletti, E. (2017). Projected global ground-level ozone impacts on vegetation under different emission and climate scenarios. Atmos. Chem. Phys. 17, 12177–12196. doi: 10.5194/acp-17-12177-2017
Stierlin, É, Nicolè, F., Costes, T., Fernandez, X., and Michel, T. (2020). Metabolomic study of volatile compounds emitted by lavender grown under open-field conditions: a potential approach to investigate the yellow decline disease. Metabolomics 16:31. doi: 10.1007/s11306-020-01654-6
Thioulouse, J., Dray, S., Dufour, A., Siberchicot, A., Jombart, T., and Pavoine, S. (2018). Multivariate Analysis of Ecological Data with ade4. New York, NY: Springer. doi: 10.1007/978-1-4939-8850-1
Ueda, H., Kikuta, Y., and Matsuda, K. (2012). Plant communication. Mediated by individual or blended VOCs? Plant Signal. Behav. 7, 222–226. doi: 10.4161/psb.18765
Upson, T. M., Andrews, S., and Harriott, G. (2004). The Genus Lavandula. Portland, OR: Timber Press.
Vautard, R., Honoré, C., Beekmann, M., and Rouil, L. (2005). Simulation of ozone during the August 2003 heat wave and emission control scenarios. Atmos. Environ. 39, 2957–2967. doi: 10.1016/j.atmosenv.2005.01.039
Vingarzan, R. (2004). A review of surface ozone background levels and trends. Atmos. Environ. 38, 3431–3442. doi: 10.1016/j.atmosenv.2004.03.030
Wei, T., and Simko, V. (2021). R Package “corrplot”: Visualization of a Correlation Matrix. Available online at: https://github.com/taiyun/corrplot (accessed December 22, 2021).
Winfree, R., Gross, B. J., and Kremen, C. (2011). Valuing pollination services to agriculture. Ecol. Econ. 71, 80–88. doi: 10.1016/j.ecolecon.2011.08.001
Winterhalter, R., Herrmann, F., Kanawati, B., Nguyen, T. L., Peeters, J., Vereecken, L., et al. (2009). The gas-phase ozonolysis of β-caryophyllene (C15H 24). Part I: an experimental study. Phys. Chem. Chem. Phys. 11, 4152–4172. doi: 10.1039/b817824k
Yeung, L. Y., Murray, L. T., Martinerie, P., Witrant, E., Hu, H., Banerjee, A., et al. (2019). Isotopic constraint on the twentieth-century increase in tropospheric ozone. Nature 570, 224–227. doi: 10.1038/s41586-019-1277-1
Yildirim, M., Sarihan, E., Kil, H., and Khawar, K. (2019). Diurnal and nocturnal variability of essential oil content and components of Lavandula angustifolia Mill. (Lavender). Mustafa Kemal Univ. J. Agric. Sci. 24, 268–278.
Young, P. J., Naik, V., Fiore, A. M., Gaudel, A., Guo, J., Lin, M. Y., et al. (2018). Tropospheric ozone assessment report: assessment of global-scale model performance for global and regional ozone distributions, variability, and trends. Elementa 6:10. doi: 10.1525/elementa.265
Yuan, J. S., Himanen, S. J., Holopainen, J. K., Chen, F., and Stewart, C. N. (2009). Smelling global climate change: mitigation of function for plant volatile organic compounds. Trends Ecol. Evol. 24, 323–331. doi: 10.1016/j.tree.2009.01.012
Keywords: VOCs, flowers, ozone, pollution, lavender, Mediterranean ecosystems
Citation: Dubuisson C, Nicolè F, Buatois B, Hossaert-McKey M and Proffit M (2022) Tropospheric Ozone Alters the Chemical Signal Emitted by an Emblematic Plant of the Mediterranean Region: The True Lavender (Lavandula angustifolia Mill.). Front. Ecol. Evol. 10:795588. doi: 10.3389/fevo.2022.795588
Received: 15 October 2021; Accepted: 15 February 2022;
Published: 15 March 2022.
Edited by:
Robbie Girling, University of Reading, United KingdomCopyright © 2022 Dubuisson, Nicolè, Buatois, Hossaert-McKey and Proffit. This is an open-access article distributed under the terms of the Creative Commons Attribution License (CC BY). The use, distribution or reproduction in other forums is permitted, provided the original author(s) and the copyright owner(s) are credited and that the original publication in this journal is cited, in accordance with accepted academic practice. No use, distribution or reproduction is permitted which does not comply with these terms.
*Correspondence: Candice Dubuisson, Y2FuZGljZS5kdWJ1aXNzb25AY2VmZS5jbnJzLmZy