- 1School of Geography, Planning and Spatial Sciences, University of Tasmania, Hobart, TAS, Australia
- 2Central Science Laboratory, University of Tasmania, Hobart, TAS, Australia
Global crop production rate has exceeded the availability of pollination services provided by managed honeybees, and habitat loss remains a key factor in the loss of wild pollinators. Revegetation of agricultural land and wild pollination may provide a solution; however, the collection of floral trait data that are correlated to pollinator preferences remains an under studied and complex process. Here, we demonstrate a method for scent analysis, ordination [non-metric dimensional scaling (NMDS)], and clustering outputs that provides a fast and reproducible procedure for a broad grouping of flora based on scent and unlocking characteristic inter-floral patterns. We report the floral profiles of 15 unstudied native Australian plant species and the extent to which they match the commonly cultivated seed crops of Daucus carota L and Brassica rapa L. Through solid-phase microextraction (SPME) paired with gas chromatography–mass spectrometry (GC-MS), we identify a set of inter-family shared, common floral volatiles from these plant species as well as unique and characteristic patterns.
Introduction
Pollination is a vital ecosystem service in both natural and agricultural settings. Biotically pollinated plants account for 35% of global crop volume, including crucial high-value seeds, nuts, fruits, and vegetables (Klein et al., 2007). Insects contribute to an estimated value of USD170–215 billion via agricultural pollination (see Kearns et al., 1998; Gallai et al., 2009; Vanbergen and the Insect Pollinators Initiative, 2013). Biotically pollinated crop production has nearly quadrupled in the recent half-century (1961–2007) and concerningly, this growth has not been matched in the global population of the dominant commercial pollinator, the European honeybee Apis mellifera L. (Klein et al., 2007; Aizen and Harder, 2009). Compounding this dilemma, both wild and managed pollinators are in decline worldwide (Bailes et al., 2015; Goulson et al., 2015; Potts et al., 2016).
Wild pollinator populations are threatened by multiple and frequent synergistic threats, including deforestation and land-use changes, broadscale agricultural pesticide and herbicide use, and climate change (Goulson et al., 2015; Potts et al., 2016). Similarly, managed honeybees are at risk from colony collapse disorder, the parasitic mite Varroa destructor, monofloral diets resulting in poor nutrition and declining disease resilience, and pesticide use (Rosenkranz et al., 2010; Staveley et al., 2014; Watson and Stallins, 2016). Given these threats to pollinators, recent research has investigated the potential for revegetation of agricultural land to provide habitat for wild pollinators and the extent to which this may meet crop pollination demand through increased wild pollination.
Native vegetation in agricultural landscapes can provide a beneficial conduit of native pollinators (see Holzschuh et al., 2012; Nicholls and Altieri, 2013; Eeraerts et al., 2019), and several insect groups may provide a useful pollination service (Rader et al., 2016). A few studies have demonstrated that crop yield per unit area positively increases with an increasing abundance of crop-adjacent native vegetation supportive of wild insects (Arthur et al., 2010; Garibaldi et al., 2016). Wild bees often remain to be reliant on native vegetation resources despite crop visitation. For example, Brown et al. (2020) determined that above ground nesting bees preferred to nest in native forest habitats despite frequently foraging in and pollinating perennial, woody crops. In this context, it can be acknowledged that semi-natural or remnant habitats at the margins of cropping land remain fundamental for sustaining wild pollinators and harnessing their pollination potential (Nicholls and Altieri, 2013; Bailes et al., 2015; Bartual et al., 2019).
The Tasmanian Midlands is one of the first regions modified for agriculture following the colonial settlement of Australia, and has lost an estimation of 83% of its original native vegetation (Fensham and Kirkpatrick, 1989). Spanning approximately a distance of 7,746 km2, the Midlands contributes toward an estimated 16% of the state's agricultural production, including biotically pollinated poppy, clover seed, carrot seed, brassica seed, and fruiting crops (Department of Economic Development, Tourism and the Arts: DEDTA, 2012). Carrot seed is a valuable commodity chiefly produced across the Midlands region, and Tasmania generates an estimated 280–320 tons annually across 700–800 ha of crop sites (DEDTA Tasmanian Institute of Agriculture Freshlogic, 2014). However, the expansion of this industry is constrained by the limited availability of rental honeybee hives, and a recent, local study has identified that rented honeybees preferentially visit alternative pollen sources despite their placement in carrot fields (Gaffney et al., 2019).
From both ecological and economic perspectives, the demand for increased, sustainable pollination in Tasmania offers an opportunity to explore the question of how best to support agricultural pollination needs while improving biodiversity conservation. As identified from on-farm wildflower strip studies by Burkle et al. (2020), it remains important to select both core revegetation species, which maximize wild pollinator diversity as well as support species that may sustain rarer pollinators at broader spatiotemporal levels. Furthermore, it is important to carefully select native flora to minimize competition with crop pollination. To select suitable revegetation species, it is therefore first necessary to understand the plant traits that may be mediating pollinator interactions for both crop and native plant species.
It is widely accepted that insect attraction to flowers is mediated by the three traits, namely color, shape, and scent (Raguso, 2008a,b). Historically, however, research has biased the conceptualization of pollination as visually mediated through floral shape and color (Raguso, 2008a), without embracing the immense array of floral odors exhibited by plants (Wright and Schiestl, 2009). A growing body of literature has demonstrated that scent plays an important role in structuring sophisticated, community-level interactions through plant–pollinator signaling (Wright and Schiestl, 2009; Burkle and Runyon, 2019). Furthermore, evidence supports the evolution of floral volatiles as a response to insect selection (Schiestl, 2010).
Advances in analytical chemistry in recent decades have nurtured a new capacity in exploring the role played by floral scent as a cue for pollinators. Characterized for the first time, floral volatiles have been demonstrated to attract and direct pollinators, fluctuating to target maximum pollinator activity, signal when flowers are receptive, and advertise rewards such as nectar (Junker and Parachnowitsch, 2015; Kantsa et al., 2017; Bouwmeester et al., 2019). Although floral scent cues are believed to function best in tandem with morphological traits other than floral color (Junker and Parachnowitsch, 2015; Schiestl, 2015), they have been correlated to floral color at a community level in limited studies (see Kantsa et al., 2017). However, due to the greater aptitude of animals to decipher olfactory over color signals, it has been suggested that floral volatiles allow the encoding of complex signals that remain to be more powerful than color cues, which are rarely highly specific (Haverkamp et al., 2018 in Bouwmeester et al., 2019).
Amongst chromatographic methods, solid-phase microextraction (SPME) paired with gas chromatography–mass spectrometry (GC-MS) has gained popularity as a fast, reliable analytical technique for assessing floral and other volatiles (Vas and Vékey, 2004; Tholl et al., 2006). Merits of the SPME method include the ability to isolate floral parts and avoid sampling of undesired green leaf volatiles, portability and reliable storage of samples from fieldwork to lab analysis, and easy reuse of the sampling apparatus (Vas and Vékey, 2004; Tholl et al., 2006). This is useful as obtaining suitable data on floral and vegetation traits can otherwise be a time- and resource-intensive process (Bartual et al., 2019). Common morphological floral traits often measured include floral symmetry, restrictiveness of flowers, corolla and flower size, and the color or spectral reflectance of flowers (Dellinger, 2020; Wang et al., 2020). However, in addition to being time-intensive to measure, recent findings have suggested that not all floral traits are of equal importance and that consistent methods for the collection of informative trait data are lacking (Dellinger, 2020). For example, Wang et al. (2020) compared five morphological traits and found that they were only accounted for 22.5% of the preferences driving pollinator visits.
Integrating these ideas, we examine whether SPME profiling of scent volatiles provides a viable method for rapidly unlocking characteristic patterns between native flora and crop hybrids. In case of these patterns being reproducible and quickly obtainable, they may allow the filtering and sorting of flora for revegetation studies when applied alongside insect visitation data. To achieve this, we document detailed, floral scent profiles for a subset of 15 previously unstudied Australian plant species and develop a scent database. We identify total, common, unique, and dominant volatile emissions and compare them to determine if similarities are shared at the family or genus level within native flora. Furthermore, we compare our results with the results of the literature of two crop hybrids, oilseed rape Brassica rapa L. and carrot Daucus carota L., ascertaining an overlap with native species. Lastly, we investigate whether non-metric dimensional scaling (NMDS) or hierarchical clustering of SPME results provides a reliable method for rapid species comparison.
Methods
Study Region and Flora Selection
A shortlist of 15 candidate native species within the Tasmanian Midlands was derived from, “A Field Guide to Native Flora Used by Honeybees in Tasmania” [Leech and Rural Industries Research and Development Corporation (Australia), 2009]. As the foraging habits of native bees remain largely unknown (Batley and Hogendoorn, 2009), and even more so of other pollinators, we considered the level of honeybee attraction as a substitute. Flora species were selected from four locally abundant families: Fabaceae, Myrtaceae, Pittosporaceae, and Proteaceae, and were limited to those extant to the Tasmanian Midlands region (Figure 1), as identified from cross-comparison to Fensham and Kirkpatrick (1989) and the Natural Values Atlas (Department of Primary Industries, Parks, Water and Environment, 2019). Ground-truthing of geographical distributions and traveling time constraints resulted in the refinement of the final list (Table 1). We also chose the two dominant crop species in the Midlands, oilseed rape B. rapa L. and carrot D. carota L., to characterize their literature-derived floral scent traits as a contrast with native species. This resulted in a total of 17 species in our study.
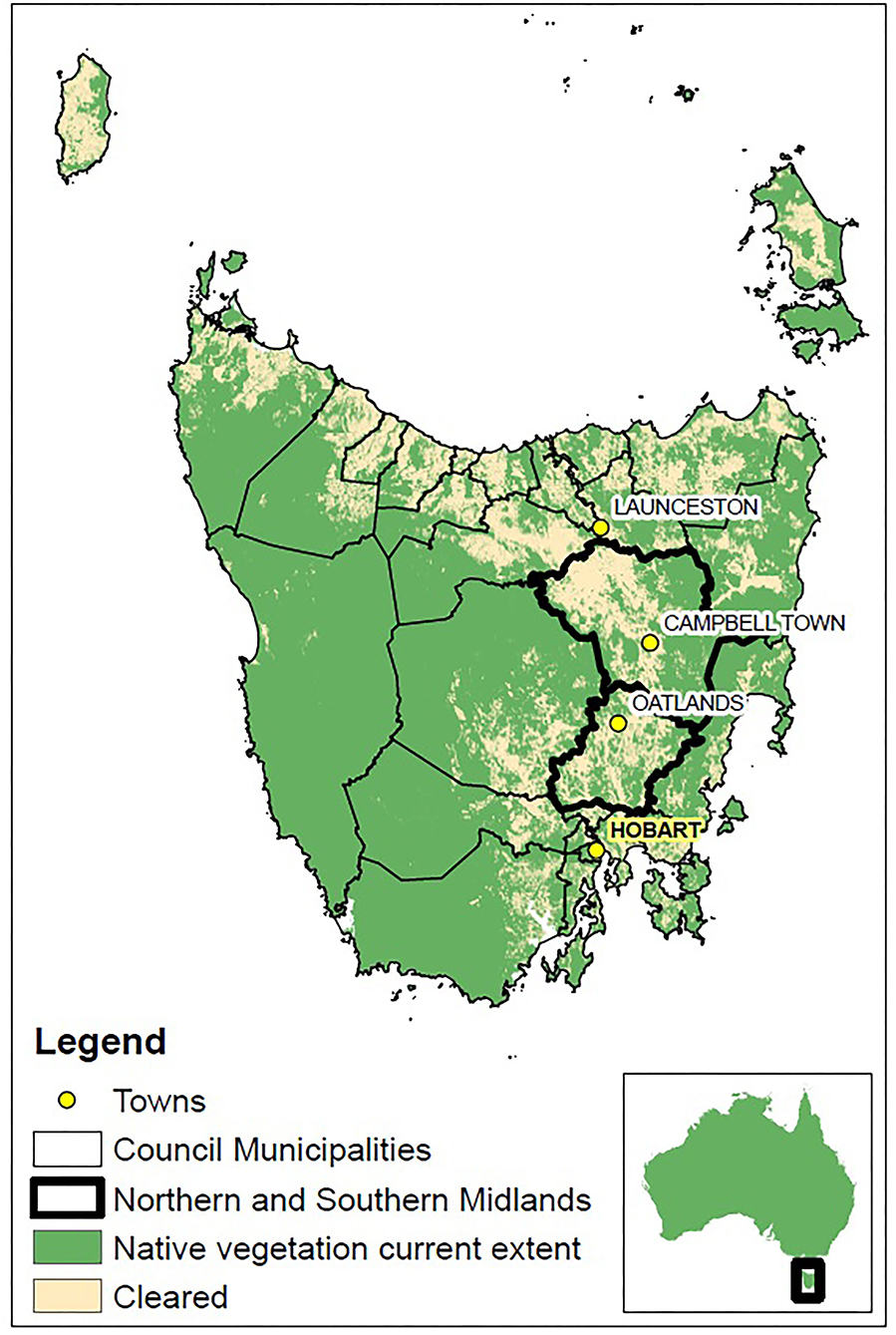
Figure 1. Map of Tasmanian state council municipality borders, depicting the study area of the Northern and Southern Midlands highlighted in bold. Key towns in the state and study region are given, the capital city Hobart is given in bold and highlighted [Map data sources: National Vegetation Information System (NVIS)—Department of Agriculture, Water, and the Environment (2018); LISTdata—Department of Primary Industries, Parks, Water and Environment (2012)].
Floral Scent Sampling and Analysis
Study sites for the scent sample selection were randomized across the Tasmanian Midlands (Figure 1). This allowed the capture of genetic as well as physiological variations in floral emissions, lowering the likelihood of data skewing by localized clonality or uniform pollination pressures. A total of 57 scent samples were collected in situ from plants across the study region over a single spring-autumn flowering period (August 2018–May 2019), with three geographically randomized scent replicates collected per plant species to account for a sample variation and reproducibility. Sampling time and budget limitations prohibited the study of a greater number of replicates.
Two opportunistic samples were collected for the Proteaceae species: Hakea microcarpa and Persoonia juniperina, several individuals of which were found co-occurring at a revegetation site. Due to the outbreak of bushfire and associated smoke haze in January to February 2019, only partial analyses were obtained for a commercial carrot seed hybrid crop. Literature scent profiles for D. carota (Species ID: Dau.car) and B. rapa (Species ID: Bra.rap) were sourced from the work of Vuts et al. (2018) (Kobayashi et al., 2012; Knauer and Schiestl, 2015; Vuts et al., 2018) to facilitate comparative statistical analyses between Midlands native flora and these two high-value crops.
Floral volatiles were sampled via solid-phase micro-extraction (SPME) during the warmest part of the day (12:00–15:00), targeting peak diurnal emissions. An oven bag (glad, 25 cm × 38 cm) headspace was created around inflorescences visually determined to be in peak flower and the volatiles accumulated for 30 min. The extraction of floral volatiles was performed with a Supelco 50/30 μm DVB/CAR/PDMS Stableflex (24 Ga) SPME fiber for 30 min per headspace. The samples were returned in Supelco Fiber Assembly Storage Devices for an immediate GC-MS analysis, conducted on a Varian CP-3800 GC—Bruker 300-MS TQ MS system, fitted with an Agilent DB-5 (30 m length × 0.25 mm internal diameter × 0.25 μm film thickness) GC column and utilizing helium as the carrier gas (flow rate 1.2 ml/min). Samples were injected in a splitless mode, with an injection port temperature of 270°C. Column temperature was programmed to increase from an initial 40°C (held for 4.0 min) to 90°C at a rate of 15.0°C/min, then to 250°C at 4.0°C/min, finishing with a column bakeout temperature of 290°C at a ramp rate of 25.0°C/min. The MS was set to full-scan mode and across the range of (m/z) 35–350. Following the analysis, SPME fibers were conditioned in preparation for subsequent sample collection.
Floral Scent Characterization
Volatiles observed through GC-MS were identified based on matching of their semi-standard, non-polar retention index values and mass spectra, against the National Institute of Standards and Technology (NIST17) Mass Spectral Library. Reference samples of selected compounds were analyzed to verify the validity of the identification procedure as it was unfeasible to procure or synthesize the total number of compounds observed in this study. Testing with standard solutions supported the identification of limonene in this study, rather than closely related sylvestrene, despite an unusual mass spectral profile obtained for both field and stock samples in comparison to the NIST17 Mass Spectral Library. A sample of sylvestrene could not be procured or isolated for additional confirmation.
Samples were tracked by the name of the sampling fiber used, and chromatograms were superimposed in the collection sequence of each fiber to check for any carryover from the previous sample. A sample was determined to contain carryover if the following two criteria were both met: (i) the target peak was lower in height to a matching peak in the previous sample acquired by the same fiber and (ii) the compound matching to the target peak was absent from the other two samples for that plant species.
Scent Profiling Database
Scent volatiles were tabulated for each plant species as separate spreadsheets within a master database, then collated in a master page (Appendix 1.1 in Supplementary Material). Species profile tables contained total scent volatiles (rows) against experimental and library retention indices (columns).
Based on the scent profiles, subsequent comparative analyses used two methods for comparing chemical profiles. For each species, the two methods of constructing chemical profiles were as follows.
Complete profile: All volatiles found in the samples were included into a single comprehensive profile. The presence of a volatile was indicated with the value of 1 and its absence was indicated with the value of 0.
Average profile: Volatiles were included in the profile if they were consistently found in samples. To test the sensitivity of this method, we considered two thresholds: (1) volatiles were included if they were present in 2/3 samples per species (2) or in 3/3 samples. The presence of a volatile was indicated with the value of 1 and its absence with the value of 0.
Data analyses were completed and compared for each of the profiles to test the robustness of the results. The results for the complete profile are presented in the main text and the master page of Appendix 1.1 in Supplementary Material (average profile results can be found in Appendices 1.2–1.3 in Supplementary Material).
Dominant Volatiles
Dominant scent volatiles were defined as those emitted in the highest volume at the time of sampling. For each scent sample, peak area integration allowed sorting from the highest to lowest emitted volatiles. The six highest area volatiles per sample were extracted and tabulated for each species, these were narrowed further to identify the “Top 2” most abundant peaks per species. The results are presented in the main text.
Exploring the Relationship Between Volatiles and Taxonomy: Common and Unique Volatiles
Using complete and average scent profiles for both full and significant volatile analyses, the patterns in volatile distribution at the species, genus, and family level were assessed by arranging species (rows) based on taxonomic relationships (sorted alphabetically by Family–Genus–Species) against volatiles (columns) by compound class and frequency (sorting by most common to least common volatiles per class). Colors were assigned by genera to aid visual assessment of data patterns. A subset of common volatiles was derived from the complete profile matrix, these were defined as volatiles, which are present in two-thirds of or greater than all 17 study species. Unique compounds were defined as those occurring only within a single study species.
Non-metric Multidimensional Scaling
To better understand dissimilarity, NMDS was performed on the scent profiles in R (RStudio Team, 2016). Bray–Curtis distances were calculated for species utilizing the Vegan Package “metaMDS” function, and NMDS repetition ensured that the results were not trapped in local minima.
Hierarchical Clustering
Species groupings for the matrices were investigated through agglomerative clustering dendrograms, applying the function “hclust” in R. The calculation of the Cophenetic Correlation Coefficient for the three different dendrogram linkage methods (single, complete, and average) supported average linkage for the data set. Dendrograms, based on the average linkage method, were cut to form 1, 2, 3, and 4, groups, respectively. Clusters were compared across scent profile methods to test the robustness of clusters toward the method of constructing a scent profile.
Results and Discussion
An Overview of Floral Profiles
A total of 192 unique floral volatiles were obtained and characterized (Table 2; please refer to Appendix 1.1 in Supplementary Material for full database results and SPME retention indices). Both shared and unique compounds were noted between native and crop species. Evidence of shared volatiles was also documented at the family, genus, and species level, within native flora. In the following sections, we discuss our floral profile findings in detail, contrast common vs. dominant volatiles, and consider the suitability of NMDS or hierarchical clustering as a fast method of mapping similarity based on floral scent information alone.
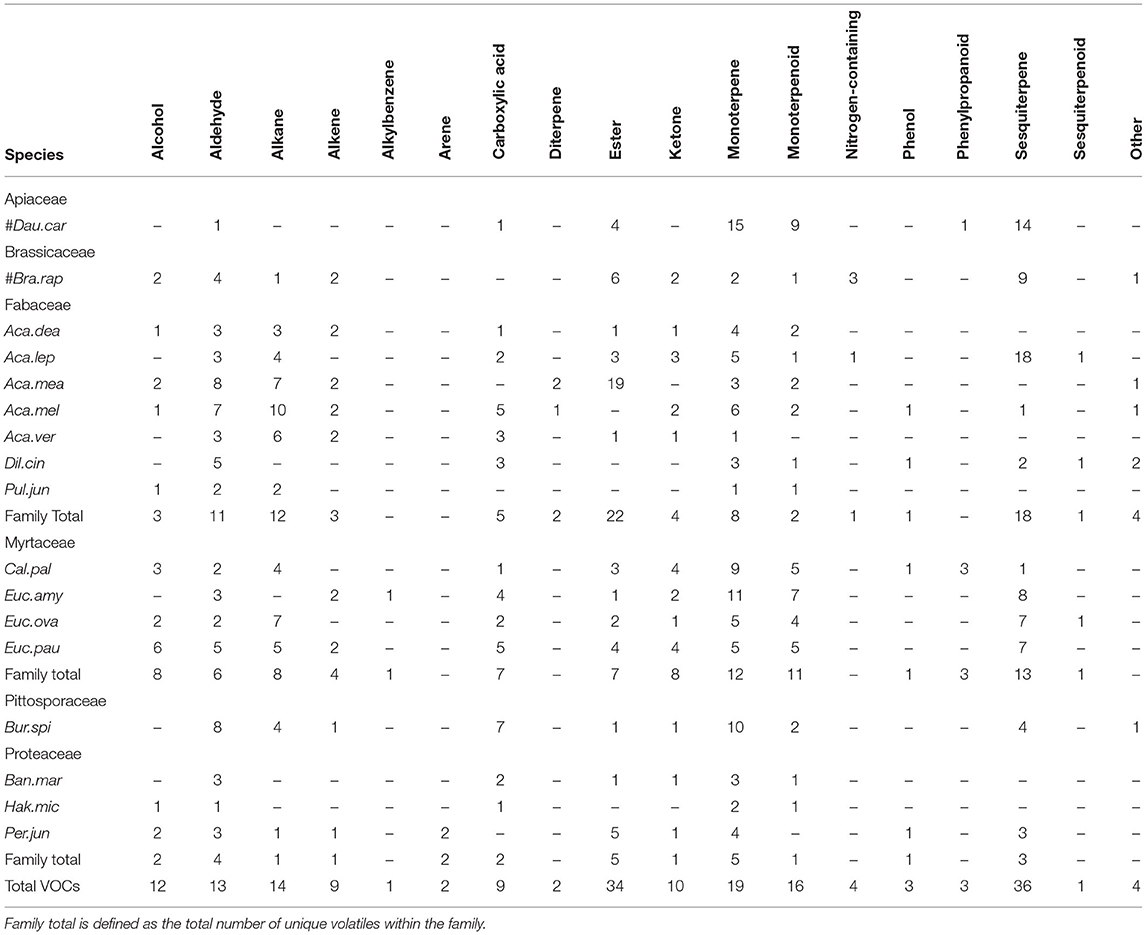
Table 2. Number of unique floral volatiles ordered against plant species, arranged by chemical class.
Floral Scent Volatiles in the Literature
Some 1,719 floral volatiles have been isolated from 991 angiosperm species (Knudsen et al., 2006). Due to their nature as secondary metabolites, scent volatiles consist of low molecular weight organic compounds from a limited number of groups: terpenes, terpenoids, nitrogen- or sulfur-containing compounds, benzenoids, phenylpropanoids, and fatty- or amino-acid derivatives (Tholl et al., 2006; Dudareva et al., 2013). Terpenoids form the largest class of volatiles, with ~556 identified, followed by the benzenoids and phenylpropanoids (Muhlemann et al., 2014). Historically, herbivore selection has largely influenced the evolution of monoterpenes as defense compounds in both gymnosperms and subsequently in angiosperms (Schiestl, 2010). However, some of these volatiles also signal to mutualists such as pollinators rather than antagonists (Pichersky and Raguso, 2018). The second largest class, the benzenoids and phenylpropanoids, is suggested to have evolved primarily for pollinator signaling (Schiestl, 2010).
From a meta-analysis of 268 papers, Knudsen et al. (2006) identified that the most common volatiles across 54–71% of the angiosperms studied included the monoterpenes: limonene, (E)-β-ocimene, myrcene, linalool, α-pinene, β-pinene; and the benzenoids/phenylpropanoids: benzaldehyde, methyl salicylate, benzyl alcohol, and phenylethyl alcohol. Also, the irregular terpene 6-methyl-5-hepten-2-one and sesquiterpene caryophyllene were present in 50% of the angiosperm families studied (Knudsen et al., 2006). In contrast to the global literature, floral scent in native Australian flora remains significantly understudied. To date, Australian studies have predominantly reported scent profiles for specialized pollination interactions in a small subset of native orchid species, or applied the technique to food quality and aroma improvement in commercial honey. However, recent studies by Sayers et al. (2020, 2021) into beetle- and fly-attracting species of Typhonium sp. (Araceae) document floral volatiles applicable to a wider suite of Australian pollinators and encouragingly progress the national floral scent literature.
Local and international studies focusing on the extraction or distillation of essential oils and non-floral material of Australian native species have identified a high terpene content for the members of the Myrtaceae such as Eucalyptus and Callistemon (see Brophy et al., 1998; Külheim et al., 2015; Sacchetti et al., 2015; Bustos-Segura et al., 2017). Monoterpene emissions have also been corroborated through foliar headspace studies of Eucalyptus (Steinbauer et al., 2004; Farnier et al., 2018). Similarly, for the members of the Fabaceae genus Acacia, aliphatic, terpenoid, and aromatic components have been described (see Brophy et al., 2007; Perriot et al., 2010; Avoseh et al., 2015; Souza-Alonso et al., 2018). One Australian study of the two species of Pittosporaceae: Pittosporum recorded differences in the essential oils, with one profile dominated by terpenes and the other by straight chain, cyclic alkanes, and related esters (Sadgrove and Jones, 2013). Variations in volatile emissions in closely related species are likely to be defined by differences in transcription factors that affect gene expression, and enzyme activity (see Dudareva et al., 2013). Information for the potential chemistry of Australian Proteaceae could not be identified.
Obtaining Common Floral Scent Volatiles
Overall, common volatiles shared across genera in this study were aligned with compounds known to be common across the majority of extant angiosperms (see Knudsen et al., 2006). These included the monoterpenes limonene (present in 16/17 plant species), alpha-pinene (12/17), trans-beta-ocimene (12/17), and para-cymene (11/17), the monoterpenoid eucalyptol (12/17) and the aliphatic aldehyde nonanal (11/17) and decanal (11/17) (Figure 2). Robustness testing against the average method (Appendix 1.2 in Supplementary Material) confirmed that the nine most common volatiles remained consistent across species, with only benzaldehyde replacing nonanal on some occasions.
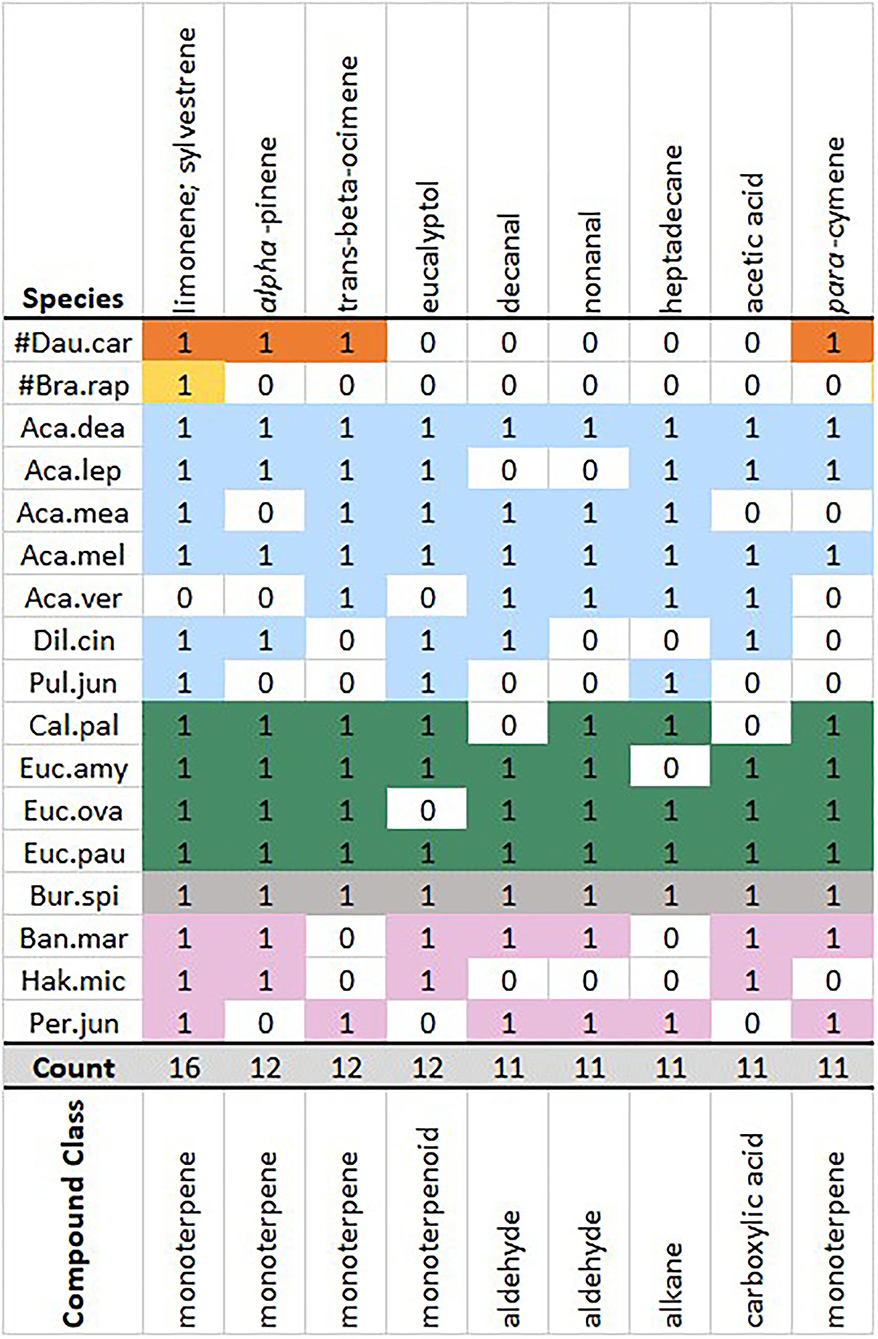
Figure 2. Complete method matrix common floral volatile excerpt. Common volatiles were defined as present in two-thirds or greater of the 17 study species. Species grouped and colored by genera, presence of a volatile = 1, absence = 0. Volatiles ordered from the most to least common. For species abbreviation keys, refer to Table 1 and Section Floral Scent Sampling and Analysis.
An Overview of Dominant Floral Scent Volatiles
We define Dominant volatiles (Figures 3A–C) as those exhibiting the largest abundance in samples, and cross-compare them to Common (shared across genera) volatiles (Figure 2). Whilst it should be acknowledged that the SPME provides a sampling snapshot per replicate and does not account for diurnal changes in emissions, we attempted to standardize collection to the middle part of the day (11:00–14:00) and sampled flowers actively attracting floral visitors. From the quantification of Dominant volatiles, some overlaps were identified with those known to be Common, chiefly the compounds limonene, eucalyptol, and benzaldehyde. However, it was not always the case that common volatiles were directly related to Dominant. Plant species exhibited abundant emissions of other floral volatiles, of which some were also unique to single species. It is, therefore, possible that these abundant, less common volatiles may play an important role in insect signaling.
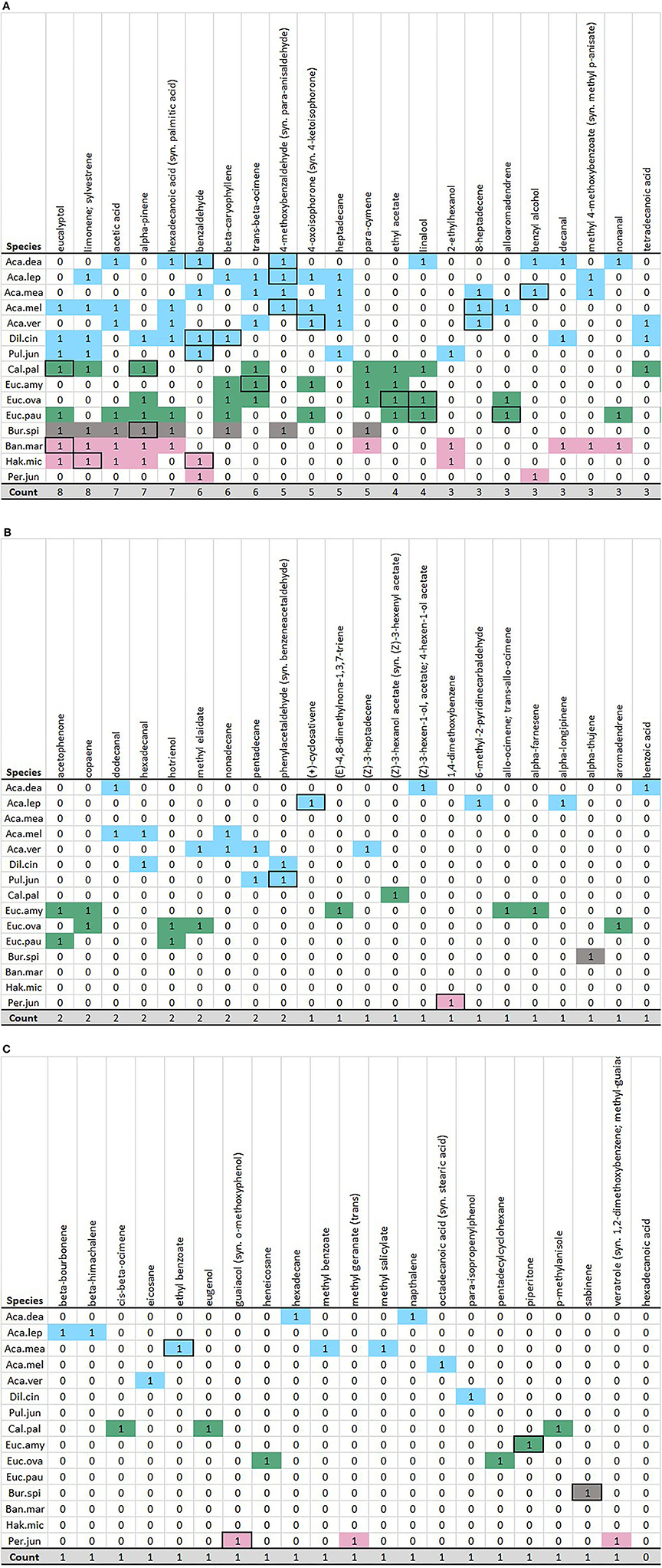
Figure 3. (A) Color matrix of dominant floral peaks, Part A. Rows are colored by family, columns sorted by most to least commonly occurring (left to right), and alphabetically. Black box outlines indicate the “Top 2” (highest emission) peaks per species. (B) Color matrix of dominant floral peaks, Part B. Rows are colored by family, columns sorted by most to least commonly occurring (left to right) and alphabetically. Black box outlines indicate the “Top 2” (highest emission) peaks per species. (C) Color matrix of dominant floral peaks, Part C. Rows are colored by family, columns sorted by most to least commonly occurring (left to right) and alphabetically. Black box outlines indicate the “Top 2” (highest emission) peaks per species.
Benzaldehyde was one of the most common Dominant volatiles—being a dominant volatile and “Top 2” peak for the four species of Fabaceae (Acacia dealbata, Dillwynia cinerascens, and Pul. juniperina) and Proteaceae (H. microcarpa). Four out of five wattles shared the related compound 4-methoxybenzaldehyde (exception: Acacia verticillata), and this formed a “Top 2” compound for three of these. Heptadecane was also observed in 5/7 Fabaceae, whilst not a Dominant volatile in other families. Ethyl acetate was the only Dominant volatile present in all Myrtaceae and a “Top 2” peak for Eucalyptus ovata; not dominant for other families. Linalool was both a Dominant volatile for the three species of Myrtaceae (absent from Eucalyptus amygdalina) and a “Top 2” peak for E. ovata and E. amygdalina. Trans-beta-ocimene was Dominant for 3/4 Myrtaceae; however, only a “Top 2” peak for E. amygdalina. For the Proteaceae: eucalyptol was a Dominant and “Top 2” peak for Banksia marginata and limonene for H. microcarpa. Other “Top 2” peaks were unique to species, such as 1,4-dimethoxybenzene and guaiacol in P. juniperina or sabinene in Bursaria spinosa.
Comparison and Summary of Native-Native Floral Profiles
Overall, the floral odors of A. verticillata and H. microcarpa contained more alternative or unique volatiles, sharing fewer common volatiles with other species. Cross-family similarities were observed between the Fabaceae Acacia leprosa var. graveolens and Myrtaceae E. amygdalina. Pittosporaceae B. spinosa exhibited some similarity to both Myrtaceae and Fabaceae. Aliphatic and aromatic alcohols were especially common in Eucalyptus pauciflora in comparison to all other genera. Straight-chain alkanes were particularly prominent in the Fabaceae followed by the Myrtaceae, if absent from the species D. cinerascens and E. amygdalina, and largely absent from all other families. Carboxylic acids were numerous in the Fabaceae, Myrtaceae, and Pittosporaceae, with a few exceptions at a species level. Aromatic aldehydes were absent in the Myrtaceae (Eucalyptus and Callistemon), whilst present in all other families. The aromatic aldehyde benzaldehyde appeared to be particularly prevalent in other genera, and its absence in the Myrtaceae may be linked to historically driven selection by herbivores favoring monoterpene production over benzenoids and phenylpropanoids (Schiestl, 2010). A larger number of aliphatic and aromatic ketones were also observed for the Myrtaceae. Although sesquiterpene and ester compounds were recorded more frequently than any other chemical class, it should be noted that this observation is inflated by their high diversity in the two species of Fabaceae.
Scent Profiles at Family and Genus Levels: Shared and Unique Volatiles
Strikingly, the family level scent results obtained from this study largely mirrored the non-floral literature, supporting a link between plant metabolism and floral emissions. The results are summarized below and explored from the family to genus and species level.
Fabaceae
A high number of esters (19) and sesquiterpenes (18) were detected in the wattles Acacia mearnsii and A. leprosa var. graveolens, respectively (Table 2). Floral profiles were otherwise dominated by aldehydes and monoterpenes; the only chemical classes present across all species and genera studied. Of aldehydes, decanal, hexadecanal, and benzaldehyde were common in ≥70% of species, across both wattle (Acacia) and pea (Dillwynia and Pultenaea) genera. In pea genera, benzaldehyde was observed to co-occur with phenylacetaldehyde (syn. benzeneacetaldehyde), whereas across wattle genera, benzaldehyde co-occurred with 4-methoxybenzaldehyde.
This is of interest as phenylacetaldehyde is produced from the shikimate pathway, whereas both benzaldehyde and 4-methoxybenzaldehyde are produced from the associated phenylpropanoid/benzenoid pathway. Phenylacetaldehyde is produced directly from the precursor amino acid phenylalanine, whereas benzaldehyde requires phenylalanine to first undergo conversion to t-cinnamic acid (Sheehan et al., 2012). These results may suggest that, unlike peas, wattle genera appear to forego the production of phenylacetaldehyde in the shikimic pathway and are driven toward benzenoid production. Given that a flowering overlap occurs between wattle and pea genera, whether this could be evidence of diversification due to a selection pressure warrants further examination. Furthermore, scent manipulation experiments have demonstrated that a difference in a single volatile can affect pollination outcomes. For example, Waelti et al. (2008) observed that addition of phenylacetaldehyde increased the transfer of a pollen-analog (dye) between two closely related Silene species (Caryophyllaceae).
It should also be noted that, whilst benzaldehyde was absent from A. dealbata sampled in this study, it has been recorded previously from cut flower emissions (Latinovic, 2015). Factors influencing this observation could be the timing of field sampling as floral volatiles are both developmentally and physiologically regulated and can alter following successful pollination (Dudareva et al., 2013; Muhlemann et al., 2014). Alternatively, changes in odor composition of cut flower samples may have occurred in a previous study (see Mookherjee et al., 1990; Theis, 2006).
Monoterpenes present in ≥70% of Fabaceae consisted of limonene and trans-beta-ocimene. Limonene was identified across all species, with the exception of A. verticillata. Trans-beta-ocimene was recorded across all wattle species and absent from pea species. All wattle species contained heptadecane, and a majority (4/5 species) contained pentadecane and non-adecane. Heptadecane and pentadecane were also present in the pea P. juniperina, whilst alkanes were notably absent from the profile D. cinerea.
Genus Acacia Unique VOCs
Characteristic, paired signals for 8-heptadecene and a related, unidentified heptadecene isomer were observed for the three wattle species: A. dealbata, Acacia melanoxylon, and A. verticillata. These alkene compounds were not recorded within any other family. Similarly, the diterpenes kaur-15-ene and kaur-16-ene only occurred in A. mearnsii and A. melanoxylon (kaur-16-ene only). Compounds unique to A. verticillata included the esters methyl-10-octadecenoate and para-methoxybenzyl acetate, and a tentative identification of 6-methyl-2-pyridinecarbaldehyde. It is noteworthy that the Acacia spp. studied flower sequentially, thereby minimizing pollinator competition, and belonging to four different clades: Botrycephalae (A. dealbata and A. mearnsii), Juliflorae (A. verticillata), Phyllodinae (A. leprosa var. graveolens), and Plurinerves (A. melanoxylon). Despite this, their characteristic floral signals converged in patterns unrelated to their taxonomic groupings. Further work would be required to determine whether these results were obtained from geographically dependent or independent pollinator pressures, in preference to a genetic origin. It has been suggested that the emission of plant volatiles exhibits both phenotypic plasticity and genotypic variation (Maffei, 2010), and phenotype selection can force rapid evolution (Zu et al., 2016).
Pea Genera Unique VOCs
The pea D. cinerascens contained heterocyclic compounds, which are not observed in any other native study species: indole and 5,5-dimethyl-2(5H)-furanone.
Myrtaceae
For the Myrtaceae, the aldehydes, nonanal, and decanal were present in ≥70% of species; nonanal across all Eucalyptus and Callistemon species; decanal occurring only in Eucalyptus. No single ketone occurred across ≥70% of species; however, a pattern was noted between pairs of species. E. amygdalina and E. pauciflora contained 4-oxoisophorone (syn. 4-ketoisophorone) and acetophenone whereas E. ovata and Callistemon pallidus contained the ketone acetoin. This is noteworthy as E. amygdalina and E. pauciflora are taxonomically placed in the same subgenus and do not overlap in flowering time in our study region.
All myrtaceous species exhibited the monoterpenes limonene, alpha-pinene, trans-beta-ocimene, and para-cymene. Despite of its presence in ≥70% of species, beta-pinene was notably absent from E. pauciflora. All species contained the monoterpenoids linalool and hotrienol; eucalyptol was present in all except E. ovata. Alpha-copaene was the only sesquiterpene present across all species and identified in the floral profile of C. pallidus. Three additional sesquiterpenes were confined to Eucalyptus: beta-caryophyllene, beta-bourbonene, and alloaromadendrene.
Genera-Specific VOCs
Interestingly, the monoterpenoid hotrienol was shared across all studied Myrtaceae whilst absent from all other native or crop families. In contrast to Eucalyptus, volatiles characteristic to the profile of C. pallidus included isobutyl butyrate (ester), 2-hydroxy-3-pentanone (alpha-hydroxy ketone), the aromatic ketones para-acetylanisole and para-methylanisole, and para-cresol (phenol). Methyleugenol, also recorded in the published profile of D. carota (Vuts et al., 2018), eugenol, and isoeugenol were the three phenylpropanoids unique to C. pallidus scent.
Compounds isolated from E. amygdalina scent alone included: the monoterpenes para-cymenene and allo-ocimene; the monoterpenoids alpha-terpinyl acetate, isothymol/thymol, and piperitone. Volatiles unique to E. ovata consisted of: pentadecylcyclohexane (alkane), trans-linalool oxide (pyranoid) (monoterpenoid), and the sesquiterpenes alpha-cubebene and calamenene. In contrast to other Myrtaceae, E. pauciflora contained the highest number of alcohols, of which 2-heptanol, 2-undecanol, alpha-methyl-benzenemethanol (syn. 1-phenylethanol; styrallyl alcohol), and 2,6-dimethyl-3,7-octadiene-2,6-diol were not recorded for any other study species. E. pauciflora contained both cis and trans isomers of linalool oxide (furanoid), whereas C. pallidus and E. ovata only contained the cis or trans isomer, respectively.
Pittosporaceae
Dominant chemical types in B. spinosa scent consisted of monoterpenes, aldehydes, and carboxylic acids. Monoterpenes mirrored the Myrtaceae in number and diversity although more than half were common to all study genera. The monoterpene terpinolene was unique to this species. Whilst B. spinosa contained aldehydes commonly distributed across all genera, other areas of the aldehyde profile exhibited similarity to the Fabaceae with the emission of benzenoid and phenylpropanoid compounds: benzaldehyde, 4-methoxybenzaldhyde and phenylacetaldehyde. Carboxylic acids overlapped with Fabaceae and Myrtaceae.
It is plausible that, as Bursaria flowers later in the flowering season and generally only overlaps with a small number of Eucalyptus or Leptospermum (tea tree) spp. (Myrtaceae) in the study region, it has evolved a more varied scent for maximizing pollinator attraction. Phenylacetaldehyde and benzaldehyde are often noted in butterfly- or moth-pollinated flowers (Schiestl, 2010), and both these groups of insects attend B. spinosa flowers (pers. obs.). As B. spinosa flowers are also rich in nectar [Leech and Rural Industries Research and Development Corporation (Australia), 2009], this may also support this explanation.
Proteaceae
Overall, floral profiles of the Proteaceae contained fewer volatiles than those identified for other species. This observation could be attributed to the opportunistic sampling and fewer replicates collected for H. microcarpa and P. juniperina, observed co-flowering with the target species. However, despite a full set of replicates for B. marginata and the selection of strongly scented inflorescences, the floral profile obtained was sparse in comparison to other study species. Previous studies have collated evidence for both simplistic and complex floral odors in basal angiosperms, to whom the Proteaceae belong; however, their scent chemistry remains understudied (Jürgens, 2009) and requires further interpretation.
Only the two chemical classes, aldehydes and monoterpenes, were present across all Proteaceae taxa in this study. Decanal and nonanal, common aldehydes of other families, were observed in B. marginata and P. juniperina but absent from H. microcarpa. Benzaldehyde was present in H. microcarpa and P. juniperina but not in B. marginata. Among monoterpenes, all three species contained limonene, alpha-pinene was identified in B. marginata and H. microcarpa, and para-cymene in B. marginata and P. juniperina. The floral profile of P. juniperina was of particular interest, exhibiting unusual, related compounds not found in any other species studied. The compounds included the arenes 1,2,4-trimethoxybenzene, 1,4-dimethoxybenzene, aromatic ester veratrole (syn. 1,2-dimethoxybenzene; methyl-guaiacol), and the phenol guaiacol (syn. o-methoxyphenol).
The diversification and perhaps specialization of P. juniperina scent in relation to other Proteaceae may reflect an association with a specific subset of native bees. Investigation of 20 species of eastern Australian Persoonia and two hybrids by Bernhardt and Weston (1996) identified Leioproctus (Colletidae) and Exoneura (Apidae) spp. as the most consistent pollen carriers. Leioproctus bees constituted almost half (47%) of the collected specimens (Bernhardt and Weston, 1996). The family Colletidae is phylogenetically close to the non-Australian Andrenidae (Hedtke et al., 2013; Peters et al., 2017). Significantly, the volatile 1,4-dimethoxybenze, which was observed in notable quantity in P. juniperina (Figure 3B), has been demonstrated to elicit a powerful response in the antennae of the andrenid bee Andrena vaga (Dötterl et al., 2005). Whether this compound is common to other Persoonia spp. and is also correlated to colletid bees warrants further investigation.
Native Flora Similarities to Crop Hybrids: D. carota L.
The literature profile compiled for D. carota (Vuts et al., 2018) contained a greater number of monoterpenes (15) than it is recorded for any native plant species (Table 2; Figure 4), leading to an overlap with all native species in relation to monoterpene emission. In particular, B. spinosa (9 shared compounds), E. amygdalina (9), and C. pallidus (8) matched most closely to the monoterpene profile of D. carota. Following these, the members of the wattle group A. leprosa var. graveolens (5), A. melanoxylon (5), and A. dealbata (4) overlapped with terpenes common to all families; however, A. verticillata (1) and A. mearnsii (2) and pea genera exhibited a notable dissimilarity. Similarly, Proteaceae exhibited a minor overlap of 2–3 monoterpenes (Figure 4). In contrast, sesquiterpenes, the second most dominant group of volatiles in the carrot scent profile (Vuts et al., 2018), exhibited a minimal overlap with native study species: beta-bourbonene in A. leprosa var. graveolens, B. spinosa, and all Eucalypt species; alpha-humulene (syn. alpha-caryophyllene) in A. leprosa var. graveolens and B. spinosa; and spathulenol in P. juniperina.
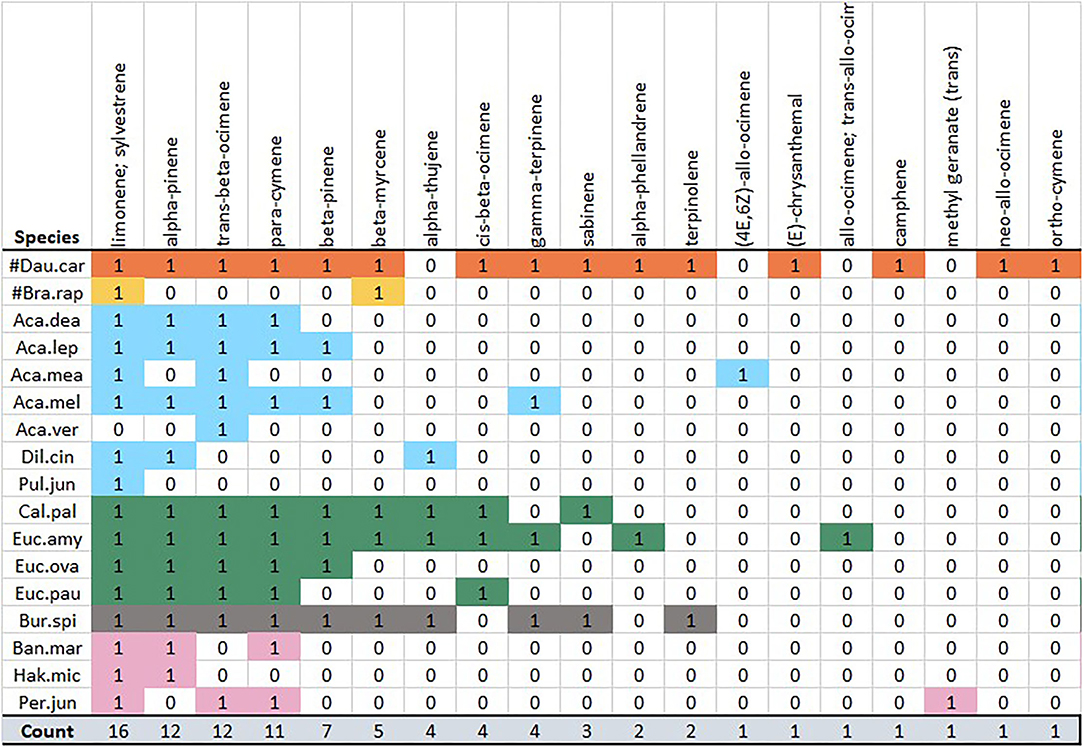
Figure 4. Complete method matrix: monoterpene subsection. Monoterpene presence–absence is shown against study species. Species grouped and colored by genera, presence of a volatile = 1, absence = 0. Volatiles ordered from most to least common.
Native Flora Similarities to Crop Hybrids: B. rapa
Sesquiterpenes, esters, and aldehydes occurred more frequently in the literature profile of B. rapa (Kobayashi et al., 2012; Knauer and Schiestl, 2015) (Table 2). Two sesquiterpenes were identified to overlap with native species: beta-caryophyllene and alpha-farnesene. A noteworthy ester overlap involved: methyl salicylate, (Z)-3-hexanol acetate [syn. (Z)-3-hexenyl acetate], benzyl ethanoate (syn. benzyl acetate) and methyl benzoate. Aldehydes shared with native flora included benzaldehyde,4-methoxybenzaldehyde (syn. para-anisaldehyde), 6-methyl-5-hepten-2-one, and acetophenone. Lastly, B. rapa and D. cinerascens were the only other species to contain indole (aromatic heterocycle); whilst B. rapa also contained several other nitrogen-containing volatiles, which are not observed in the native study species.
Native Floral Volatiles Common to Both Crop Taxa
Certain volatiles recorded for native species matched to known compounds of both D. carota and B. rapa scent. These included aldehyde phenylacetaldehyde (syn. benzeneacetaldehyde),; monoterpenes limonene (present in all species except A. verticillata) and beta-myrcene, and the monoterpenoid linalool. Sesquiterpenes shared with native flora included alpha-copaene, delta-cadinene, and germacrene D.
Crop-Native Plant Summary and Implications
Although the common volatile findings (Figure 2) found a very little overlap between native flora and B. rapa than D. carota, it is not the case that beneficial pollinator attraction is unlikely to exist. As demonstrated in Sections: Native Flora Similarities to Crop Hybrids: D. carota L., Native Flora Similarities to Crop Hybrids: B. rapa, Native Floral Volatiles Common to Both Crop Taxa, native flora shared a wide array of compounds with the two-crop species. For this reason, it is important to consider the potential significance of all volatiles and not only common volatiles. Overall, it was ascertained from a matrix interpretation (Appendix 1.1 in Supplementary Material) that the scent profile of D. carota matched most closely to those of the Myrtaceae: E. amygdalina, C. pallidus; Fabaceae: A. leprosa var. graveolens; and Pittosporaceae: B. spinosa. By comparison, the profile of B. rapa appeared more closely related to Fabaceae and Proteaceae species than to Myrtaceae and Pittosporaceae. These results have an interesting implication for revegetation projects targeting the margins of cropping land where carrots or oilseed rape are cultivated. It appears that certain species of the native flora studied may have a revegetation potential based on their floral scent traits that are similar to those of the crop species. Then, the question is whether revegetation of this landscape using these species with shared floral scent profiles could increase crop pollination reservoirs and thus provide both an agricultural and ecological benefit. This research gap could be addressed through experimental revegetation with the selected species and monitoring of pollination patterns within revegetated patches and crop fields.
Ordination and Clustering as Methods of Rapid Species Grouping
Non-metric multidimensional scaling of floral volatiles broadly grouped native species, as distanced from the crop species D. carota and B. rapa. Robustness testing against the alternative matrix input methods (Appendix 1.2 in Supplementary Material) identified sensitivity in Acacia, with species shifting from the range 0.0 to 0.5, to the range −1.5 to −0.5 in the first axis (NMDS1, Figure 5). Myrtaceae and Proteaceae groups exhibited higher cohesion in the second axis (NMDS2), whilst shifting in axis one from the range −0.5 to 0.0, to the range 0.0–0.75. Plot stress increased with robustness testing due to increasing data constraint through averaging to smaller sample sizes.
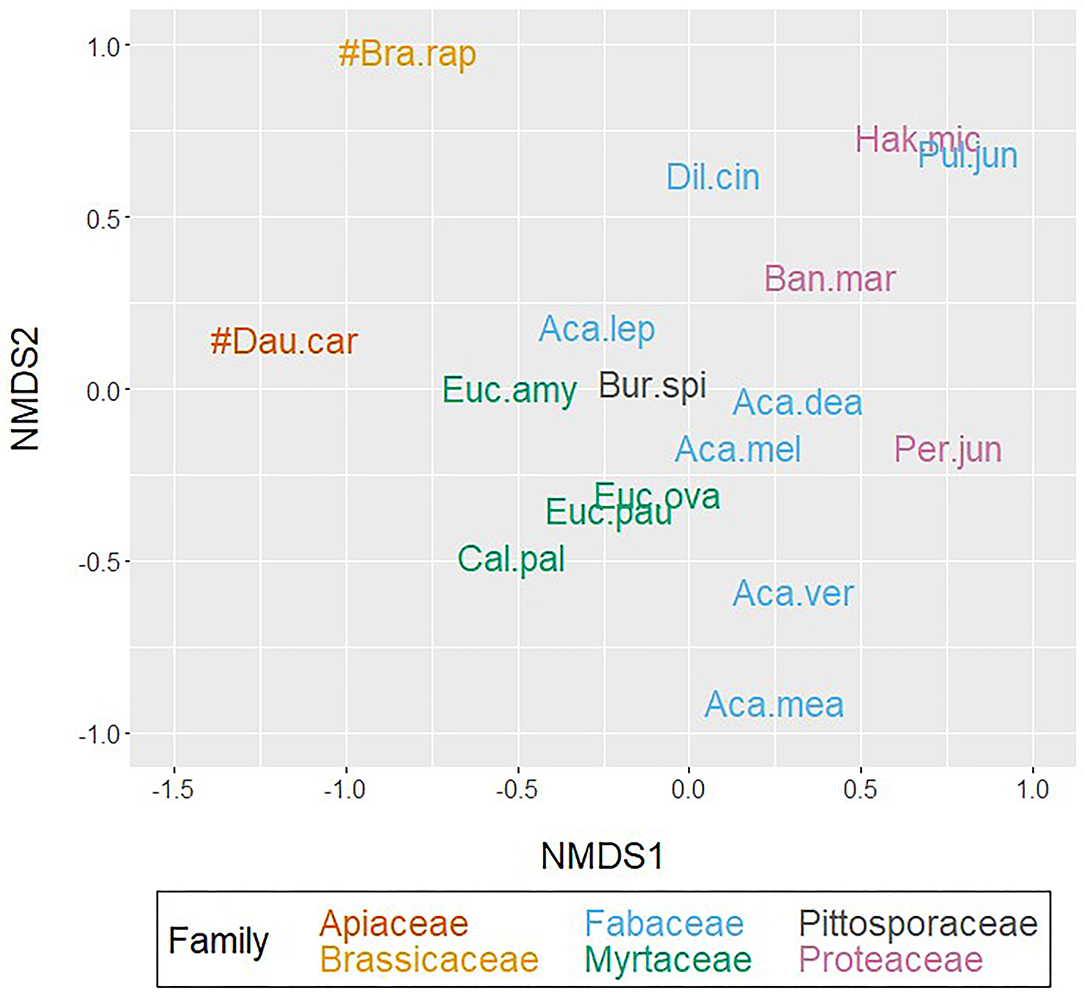
Figure 5. Two-dimensional, non-metric multidimensional scaling (NMDS) of species-level floral volatile presence-absence data, applying Bray–Curtis dissimilarity matrices (stress = 0.161). Total floral volatiles included: any compound present in any sample per species. Colored by the taxonomic rank of Family. For species abbreviation keys, refer to Table 1 and Section Floral Scent Sampling and Analysis.
Agglomerative hierarchical clustering of floral scent profiles identified two major clustering groups (Figure 6). Overall, the crop species were observed to cluster separately to the native flora, consistent with the observations from NMDS ordination (Figure 5). Whilst broadly clustering together, the native species could be split into two subclusters: “2a” containing the genus Acacia of the Fabaceae, all Myrtaceae, and Pittosporaceae, as well the Proteaceae: P. juniperina; and “2b” containing Fabaceae pea species: D. cinerascens, Pul. juniperina and the remaining Proteaceae: B. marginata and H. microcarpa. Robustness testing against the average method reaffirmed that this overall clustering pattern remained unchanged (Appendix 1.3 in Supplementary Material). However, it also highlighted that the two different methods produced different clustering results for a small number of species. The complete method split A. mearnsii and P. juniperina into separate clusters to the other native species, whereas the Average split A. mearnsii, A. leprosa var. graveolens, and P. juniperina. Interestingly H. microcarpa—Pul. juniperina, and E. ovata—E. pauciflora were observed to pair together irrespective of the data input method.
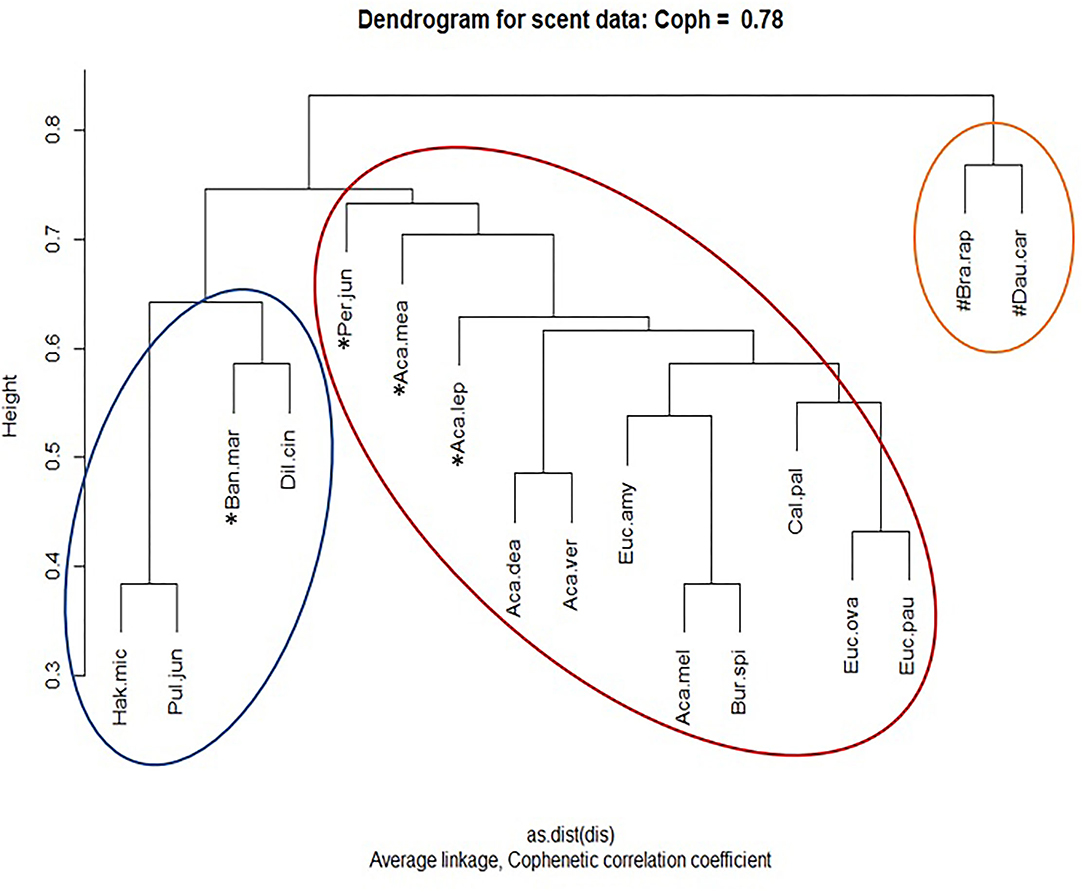
Figure 6. Average-linked hierarchical clustering dendrogram of species-level floral volatile presence-absence data. Total floral volatiles included: any compound present in any sample per species. Species that are clustered differently depending on the scent profile method are marked with an asterisk. Three broad clustering groups were observed and are circled. For species abbreviation keys, refer to Table 1 and Section Floral Scent Sampling and Analysis.
Overall, both the NMDS and clustering outputs grouped Fabaceae wattle genera, Myrtaceae, and Pittosporaceae separately from Fabaceae pea genera and the Proteaceae. Implications for the scent that can be raised largely reflect those observed from manual matrix interpretation. For example, this native-native split in grouping could indicate a primitive scent profile for Proteaceae and pea genera (fewer compounds recorded), highlighting their expected, bee-dominated pollination syndrome. However, it could also be a result of limited sampling. Further research with larger sampling is required. Similarly, the observed overall diversity of scent volatiles in Myrtaceae, Fabaceae, and Pittosporaceae could signal that they act as generalists attracting a wider range of pollinators. This could be tested through further scent volatile profile sampling, as well as field observations that confirm whether these are in fact attracting generalist pollinators.
Both outputs also identify that crops are highly different in their scent profile to the native flora as may be expected due to their exotic origin and high hybridization. Whilst this correlates to the result from manual matrix interpretation, neither the ordination nor clustering pick-up information regarding shared volatiles that may otherwise be subtly driving insect interactions. Whilst emphasis is usually placed on dominant volatiles as the drivers of pollinator or broader insect selection for flowers, minor constituents have been demonstrated to significantly influence selection among related flora species (Clavijo Mccormick et al., 2014). For both crop scent profiles, the NMDS correctly distinguishes that these hybrids are overall different to native flora and do not share many similar volatiles. However, as demonstrated through the results, manual matrix interpretation highlighted that crop and native species overlap in both more commonly found volatiles such as phenylacetaldehyde, limonene, and beta-myrcene, and rarer components such as indole. Therefore, although a large presence or absence of shared volatiles can influence broader grouping as observed in the NMDS, interpretation of Common, Dominant (abundant), and Unique (whether minor or abundant) volatiles must be considered before drawing conclusions about the similarity or dissimilarity between floral profiles.
Scent Analysis as the Method of Selecting Plants for Revegetation
Plant–pollinator networks have been demonstrated to exhibit high temporal variations—where the preferences of pollinators shift to match the available floral resources between seasons and years (Menz et al., 2011). As a result, it has been recommended that revegetation should aim to restore the maximum diversity of pollinating insects possible, so that insect species may replace each other when functional gaps occur (Menz et al., 2011). Plants play differing core roles in the plant–pollinator network that should be accounted for: framework species act as major pollen or nectar sources, bridging species provide resources in times of limitation, and magnet species help draw pollinators to less conspicuous flowers (Dixon, 2009). Identifying which plants play these core roles in the network is imperative for conservation and revegetation attempts, however, empirically assessing plant traits is time-consuming and expensive (Bartual et al., 2019; Hinton and Peters, 2021).
Scent analysis, as described in this work, may allow us to infer the possible roles played by these plants and highlight species of interest. For example, generalist plant species are likely to contain a greater abundance of common volatiles, common either to the study region or globally between all angiosperms (as defined by Knudsen et al., 2006). They may also contain common volatiles as their Dominant (abundant) emissions. Such observations were made for the members of the Myrtaceae, Fabaceae, and the sole Pittosporaceae studied, as mentioned in Sections: Obtaining Common Floral Scent Volatiles, An Overview of Dominant Floral Scent Volatiles, and Ordination and Clustering as Methods of Rapid Species Grouping. Generalist plant species play a vital ecological role by providing resources to a broad suite of pollinators (Biella et al., 2019), therefore these are likely to act as a framework or bridging species in the plant-pollinator network.
Similarly, scent analysis may also allow us to identify plant species with unique or characteristic volatile emissions, which may act as magnet species, drawing pollinators to a location, and allowing less conspicuous plants to be pollinated. For example, the unique volatiles recorded in P juniperina scent likely to play a vital role in attracting bee species of the genera Leioproctus and Exoneura known to visit the Persoonia genus (Bernhardt and Weston, 1996) (Section Proteaceae). This may have flow-on effects to other species flowering nearby.
However, as highlighted in Section: Ordination and Clustering as Methods of Rapid Species Grouping, despite the simplicity and relative accuracy of grouping potential revegetation species via ordination and clustering as a direct output of the tabulated SPME data, this method may be better applied in complement to other modes of data interpretation. Whether modeling could be extended and fine-tuned, however, through the collection of a higher number of replicates and variables remains a valid consideration. Although meticulous human interpretation of SPME data may be time-consuming, this is a worthwhile trade-off given the level of detail on compound assignations that can be obtained. However, it is important to investigate and define suitable cutoff points as increasing the level of detail may limit the ability to gauge key phylogenetic patterns rather than improving the analysis. The inclusion of pollinator visitation data would allow the confirmation of the accuracy of assumptions generated from the pure scent analysis, such as whether plant species truly act as generalists and framework species. If appropriate modeling could be developed, the interpretation of floral scent as well as shape, color, reward, value, and pollinator visitation could be united into a structured, comprehensive procedure for enabling a better comparison of species.
Conclusion and Future Directions
In conclusion, we have demonstrated that SPME profiling of floral volatiles is able to facilitate a rapid analysis of patterns present between diverse flora and collect much needed baseline data related to pollinator attraction. In addition, this method may be applied to the interpretation of inter-family- and intra-family-, generic-, or species-level comparisons, and the assessment of native flora against cropping hybrids. Patterns elucidated for shared and unique compounds document evidence of past and ongoing evolution toward selection pressures as well as volatile inheritance. Ordination (NMDS) and clustering outputs provide a fast and reproducible method for a broad grouping of flora based on scent and may be harnessed to facilitate better decision-making for revegetation projects. We propose that scent as a floral trait constitutes a demonstrable and testable variable, that is straightforward to collect. We advocate its continued adoption in revegetation studies, where it can be integrated with other floral traits and insect visitation data.
Data Availability Statement
The original contributions presented in the study are included in the article/Supplementary Material, further inquiries can be directed to the corresponding author.
Author Contributions
AL and DN conceived and designed the experiment. AL performed experiments, collected field data, processed samples, and analyzed raw data. DN contributed reagents/materials/analysis tools. AL (80%), DN, VA, and PM wrote and refined the manuscript. All authors contributed to the article and approved the submitted version.
Funding
This research was made possible by the Holsworth Wildlife Research Endowment—Equity Trustees Charitable Foundation and the Ecological Society of Australia.
Conflict of Interest
The authors declare that the research was conducted in the absence of any commercial or financial relationships that could be construed as a potential conflict of interest.
Publisher's Note
All claims expressed in this article are solely those of the authors and do not necessarily represent those of their affiliated organizations, or those of the publisher, the editors and the reviewers. Any product that may be evaluated in this article, or claim that may be made by its manufacturer, is not guaranteed or endorsed by the publisher.
Acknowledgments
We would like to acknowledge and express our gratitude to Dr. Ross Corkrey (Senior Research Fellow in Biometrics, Tasmanian Institute of Agriculture, Hobart) for his valued insights and feedback on the statistical aspects of this study. Furthermore, our appreciation to Seed Purity for their generous collaboration in the provision of field sites and insights into the Tasmanian carrot seed industry. Essential equipment purchases for this project were funded by the Holsworth Wildlife Research Endowment—Equity Trustees Charitable Foundation and the Ecological Society of Australia.
Supplementary Material
The Supplementary Material for this article can be found online at: https://www.frontiersin.org/articles/10.3389/fevo.2022.795122/full#supplementary-material
References
Aizen, M. A., and Harder, L. D. (2009). The global stock of domesticated honey bees is growing slower than agricultural demand for pollination. Curr. Biol. 19, 915–918. doi: 10.1016/j.cub.2009.03.071
Arthur, A. D., Li, J., Henry, S., and Cunningham, S. A. (2010). Influence of woody vegetation on pollinator densities in oilseed Brassica fields in an Australian temperate landscape. Basic Appl. Ecol. 11, 406–414. doi: 10.1016/j.baae.2010.05.001
Avoseh, O. N., Oyedeji, O. O., Aremu, K., Nkeh-Chungag, B. N., Songca, S. P., Oluwafemi, S. O., et al. (2015). Chemical composition and anti-inflammatory activities of the essential oils from Acacia mearnsii de Wild. Nat. Prod. Res. 29, 1184–1188. doi: 10.1080/14786419.2014.983504
Bailes, E. J., Ollerton, J., Pattrick, J. G., and Glover, B. J. (2015). How can an understanding of plant–pollinator interactions contribute to global food security? Curr. Opin. Plant Biol. 26, 72–79. doi: 10.1016/j.pbi.2015.06.002
Bartual, A. M., Sutter, L., Bocci, G., Moonen, A.-C., Cresswell, J., Entling, M., et al. (2019). The potential of different semi-natural habitats to sustain pollinators and natural enemies in European agricultural landscapes. Agric. Ecosyst. Environ. 279, 43–52. doi: 10.1016/j.agee.2019.04.009
Batley, M., and Hogendoorn, K. (2009). Diversity and conservation status of native Australian bees. Apidologie 40, 347–354. doi: 10.1051/apido/2009018
Bernhardt, P., and Weston, P. (1996). The pollination ecology of Persoonia (Proteaceae) in eastern Australia. Telopea 6, 775–804. doi: 10.7751/telopea19963035
Biella, P., Akter, A., Ollerton, J., Tarrant, S., Janeček, Š., Jersákov,á, J., et al. (2019). Experimental loss of generalist plants reveals alterations in plant-pollinator interactions and a constrained flexibility of foraging. Sci. Rep. 9, 7376. doi: 10.1038/s41598-019-43553-4
Bouwmeester, H., Schuurink, R. C., Bleeker, P. M., and Schiestl, F. (2019). The role of volatiles in plant communication. Plant J. 100, 892–907. doi: 10.1111/tpj.14496
Brophy, J. J., Goldsack, R. J., and Fookes, C. J. R. (2007). The volatiles of Acacia howittii F. Muell. J. Essent. Oil Res. 19, 457–459. doi: 10.1080/10412905.2007.9699951
Brophy, J. J., Goldsack, R. J., Forster, P. I., Craven, L. A., and Lepschi, B. J. (1998). The leaf essential oils of the australian members of the genus Callistemon (Myrtaceae). J. Essent. Oil Res. 10, 595–606. doi: 10.1080/10412905.1998.9700986
Brown, J., Barton, P. S., and Cunningham, S. A. (2020). Flower visitation and land cover associations of above ground- and below ground-nesting native bees in an agricultural region of south-east Australia. Agric. Ecosyst. Environ. 295, 106895. doi: 10.1016/j.agee.2020.106895
Burkle, L. A., Delphia, C. M., and O'Neill, K. M. (2020). Redundancy in wildflower strip species helps support spatiotemporal variation in wild bee communities on diversified farms. Basic Appl. Ecol. 44, 1–13. doi: 10.1016/j.baae.2020.02.005
Burkle, L. A., and Runyon, J. B. (2019). Floral volatiles structure plant–pollinator interactions in a diverse community across the growing season. Funct. Ecol. 33, 2116–2129. doi: 10.1111/1365-2435.13424
Bustos-Segura, C., Dillon, S., Keszei, A., Foley, W. J., and Külheim, C. (2017). Intraspecific diversity of terpenes of Eucalyptus camaldulensis (Myrtaceae) at a continental scale. Aust. J. Bot. 65, 2571. doi: 10.1071/BT16183
Clavijo Mccormick, A., Gershenzon, J., and Unsicker, S. B. (2014). Little peaks with big effects: establishing the role of minor plant volatiles in plant-insect interactions: minor plant volatiles. Plant Cell Environ. 37, 1836–1844. doi: 10.1111/pce.12357
DEDTA (2012). An Agricultural Profile of the Midlands Region, Tasmania. Tasmania, Australia: Department of Economic Development, Tourism and the Arts.
DEDTA Tasmanian Institute of Agriculture Freshlogic (2014). Carrot Seed Market Profile, Wealth for Water. Tasmania, Australia: DEDTA.
Dellinger, A. S.. (2020). Pollination syndromes in the 21st century: Where do we stand and where may we go? New Phytol. 228, 1193–1213. doi: 10.1111/nph.16793
Department of Agriculture Water the Environment. (2018). National Vegetation Information System (NVIS). Dep. Agric. Water Environ. Available online at: http://www.environment.gov.au/ (accessed August 1, 2020).
Department of Primary Industries Parks Water Environment. (2019). Natural Values Atlas. Available online at: https://www.naturalvaluesatlas.tas.gov.au/#HomePage (accessed August 4, 2019).
Department of Primary Industries Parks Water Environment (DPIPWE). (2012). LISTdata. Available online at: https://www.thelist.tas.gov.au/app/content/data/index (accessed August 6, 2020).
Dixon, K. W.. (2009). Pollination and restoration. Science 325, 571–573. doi: 10.1126/science.1176295
Dötterl, S., Füssel, U., Jürgens, A., and Aas, G. (2005). 1,4-dimethoxybenzene, a floral scent compound in willows that attracts an oligolectic bee. J. Chem. Ecol. 31, 2993–2998. doi: 10.1007/s10886-005-9152-y
Dudareva, N., Klempien, A., Muhlemann, J. K., and Kaplan, I. (2013). Biosynthesis, function and metabolic engineering of plant volatile organic compounds. New Phytol. 198, 16–32. doi: 10.1111/nph.12145
Eeraerts, M., Smagghe, G., and Meeus, I. (2019). Pollinator diversity, floral resources and semi-natural habitat, instead of honey bees and intensive agriculture, enhance pollination service to sweet cherry. Agric. Ecosyst. Environ. 284, 106586. doi: 10.1016/j.agee.2019.106586
Farnier, K., Davies, N., and Steinbauer, M. (2018). Not led by the nose: Volatiles from undamaged eucalyptus hosts do not influence psyllid orientation. Insects 9, 166. doi: 10.3390/insects9040166
Fensham, R. J., and Kirkpatrick, J. B. (1989). “The conservation of original vegetation remnants in the midlands, Tasmania in southern Tasmania,” in Papers and Proceedings of the Royal Society of Tasmania, 229–246.
Gaffney, A., Bohman, B., Quarrell, S. R., Brown, P. H., and Allen, G. R. (2019). Limited cross plant movement and non-crop preferences reduce the efficiency of honey bees as pollinators of hybrid carrot seed crops. Insects 10:3. doi: 10.3390/insects10020034
Gallai, N., Salles, J.-M., Settele, J., and Vaissière, B. E. (2009). Economic valuation of the vulnerability of world agriculture confronted with pollinator decline. Ecol. Econ. 68, 810–821. doi: 10.1016/j.ecolecon.2008.06.014
Garibaldi, L. A., Carvalheiro, L. G., Vaissière, B. E., Gemmill-Herren, B., Hipólito, J., Freitas, et al. (2016). Mutually beneficial pollinator diversity and crop yield outcomes in small and large farms. Science 351, 388–391. doi: 10.1126/science.aac7287
Goulson, D., Nicholls, E., Botias, C., and Rotheray, E. L. (2015). Bee declines driven by combined stress from parasites, pesticides, and lack of flowers. Science 347, 1255957–1255957. doi: 10.1126/science.1255957
Haverkamp, A., Hansson, B.S., and Knaden, M. (2018). Combinatorial codes and labeled lines: How insects use olfactory cues to find and judge food, mates, and oviposition sites in complex environments. Front. Physiol. 9, 49. doi: 10.3389/fphys.2018.00049
Hedtke, S. M., Patiny, S., and Danforth, B. N. (2013). The bee tree of life: a supermatrix approach to apoid phylogeny and biogeography. BMC Evol. Biol. 13, 138. doi: 10.1186/1471-2148-13-138
Hinton, C. R., and Peters, V. E. (2021). Plant species with the trait of continuous flowering do not hold core roles in a Neotropical lowland plant-pollinating insect network. Ecol. Evol. 11, 2346–2359. doi: 10.1002/ece3.7203
Holzschuh, A., Dudenhöffer, J.-H., and Tscharntke, T. (2012). Landscapes with wild bee habitats enhance pollination, fruit set and yield of sweet cherry. Biol. Conserv. 153, 101–107. doi: 10.1016/j.biocon.2012.04.032
Junker, R. R., and Parachnowitsch, A. L. (2015). Working towards a holistic view on flower traits— how floral scents mediate plant–animal interactions in concert with other floral characters. Ind. Instit. Sci. 95, 43–67. doi: 10.1663/0006-8101(2006)72[1:DADOFS]2.0.CO;2
Jürgens, A.. (2009). The hidden language of flowering plants: floral odours as a key for understanding angiosperm evolution? Commentary. New Phytol. 183, 240–243. doi: 10.1111/j.1469-8137.2009.02932.x
Kantsa, A., Raguso, R. A., Dyer, A. G., Sgardelis, S. P., Olesen, J. M., and Petanidou, T. (2017). Community-wide integration of floral colour and scent in a Mediterranean scrubland. Nat. Ecol. Evol. 1, 1502–1510. doi: 10.1038/s41559-017-0298-0
Kearns, C. A., Inouye, D. W., and Waser, N. M. (1998). Endangered mutualisms: the conservation of plant-pollinator interactions. Annu. Rev. Ecol. Syst. 29, 83–112.
Klein, A.-M., Vaissiere, B. E., Cane, J. H., Steffan-Dewenter, I., Cunningham, S. A., Kremen, C., et al. (2007). Importance of pollinators in changing landscapes for world crops. Proc. R. Soc. B Biol. Sci. 274, 303–313. doi: 10.1098/rspb.2006.3721
Knauer, A. C., and Schiestl, F. P. (2015). Bees use honest floral signals as indicators of reward when visiting flowers. Ecol. Lett. 18, 135–143.
Knudsen, J. T., Eriksson, R., Gershenzon, J., and Staahl, B. (2006). Diversity and distribution of floral scent. Bot. Rev. 72, 1–120.
Kobayashi, K., Arai, M., Tanaka, A., Matsuyama, S., Honda, H., and Ohsawa, R. (2012). Variation in floral scent compounds recognized by honeybees in Brassicaceae crop species. Breed. Sci. 62, 293–302. doi: 10.1270/jsbbs.62.293
Külheim, C., Padovan, A., Hefer, C., Krause, S. T., Köllner, T. G., Myburg, A. A., et al. (2015). The Eucalyptus terpene synthase gene family. BMC Genom. 16, 450. doi: 10.1186/s12864-015-1598-x
Latinovic, A. (2015). The Ecological Chemistry of Pollination in Tasmanian Plants and Its Conservation Implications (Honours thesis). University of Tasmania, Hobart.
Leech, M., and Rural Industries Research Development Corporation (Australia) (2009). A Field Guide to Native Flora used by Honeybees in Tasmania. Barton, ACT: RIRCD.
Maffei, M. E.. (2010). Sites of synthesis, biochemistry and functional role of plant volatiles. S. Afr. J. Bot. 76, 612–631. doi: 10.1016/j.sajb.2010.03.003
Menz, M. H. M., Phillips, R. D., Winfree, R., Kremen, C., Aizen, M. A., Johnson, S. D., et al. (2011). Reconnecting plants and pollinators: challenges in the restoration of pollination mutualisms. Trends Plant Sci. 16, 4–12. doi: 10.1016/j.tplants.2010.09.006
Mookherjee, B. D., Trenkle, R. W., and Wilson, R. A. (1990). The chemistry of flowers, fruits and spices: live vs. dead—a new dimension in fragrance research. Pure Appl. Chem. 62, 1357–1364. doi: 10.1351/pac199062071357
Muhlemann, J. K., Klempien, A., and Dudareva, N. (2014). Floral volatiles: from biosynthesis to function: Floral volatiles. Plant Cell Environ. 37, 1936–1949. doi: 10.1111/pce.12314
Nicholls, C. I., and Altieri, M. A. (2013). Plant biodiversity enhances bees and other insect pollinators in agroecosystems. A review. Agron. Sustain. Dev. 33, 257–274. doi: 10.1007/s13593-012-0092-y
Perriot, R., Breme, K., Meierhenrich, U. J., Carenini, E., Ferrando, G., and Baldovini, N. (2010). Chemical composition of French mimosa absolute oil. J. Agric. Food Chem. 58, 1844–1849. doi: 10.1021/jf903264n
Peters, R. S., Krogmann, L., Mayer, C., Donath, A., Gunkel, S., Meusemann, K., et al. (2017). Evolutionary history of the Hymenoptera. Curr. Biol. 27, 1013–1018. doi: 10.1016/j.cub.2017.01.027
Pichersky, E., and Raguso, R. A. (2018). Why do plants produce so many terpenoid compounds? New Phytol. 220, 692–702. doi: 10.1111/nph.14178
Potts, S. G., Imperatriz-Fonseca, V., Ngo, H. T., Aizen, M. A., Biesmeijer, J. C., Breeze, T. D., et al. (2016). Safeguarding pollinators and their values to human well-being. Nature 540, 220–229. doi: 10.1038/nature20588
Rader, R., Bartomeus, I., Garibaldi, L. A., Garratt, M. P. D., Howlett, B. G., Winfree, R., et al. (2016). Non-bee insects are important contributors to global crop pollination. Proc. Natl. Acad. Sci. 113, 146–151. doi: 10.1073/pnas.1517092112
Raguso, R. A.. (2008a). Start making scents: the challenge of integrating chemistry into pollination ecology. Entomol. Exp. Appl. 128, 196–207. doi: 10.1111/j.1570-7458.2008.00683.x
Raguso, R. A.. (2008b). Wake up and smell the roses: the ecology and evolution of floral scent. Annu. Rev. Ecol. Evol. Syst. 39, 549–569. doi: 10.1146/annurev.ecolsys.38.091206.095601
Rosenkranz, P., Aumeier, P., and Ziegelmann, B. (2010). Biology and control of Varroa destructor. J. Invertebr. Pathol. 103, S96–S119. doi: 10.1016/j.jip.2009.07.016
Sacchetti, P., Rossi, E., Bellini, L., Vernieri, P., Cioni, P. L., and Flamini, G. (2015). Volatile organic compounds emitted by bottlebrush species affect the behaviour of the sweet potato whitefly. Arthropod-Plant Interact. 9, 393–403. doi: 10.1007/s11829-015-9382-z
Sadgrove, N. J., and Jones, G. L. (2013). Chemical and biological characterisation of solvent extracts and essential oils from leaves and fruit of two Australian species of Pittosporum (Pittosporaceae) used in aboriginal medicinal practice. J. Ethnopharmacol. 145, 813–821. doi: 10.1016/j.jep.2012.12.019
Sayers, T. D. J., Johnson, K. L., Steinbauer, M. J., Farnier, K., and Miller, R. E. (2021). Divergence in floral scent and morphology, but not thermogenic traits, associated with pollinator shift in two brood-site-mimicking Typhonium (Araceae) species. Ann. Bot. 128, 261–280. doi: 10.1093/aob/mcab044
Sayers, T. D. J., Steinbauer, M. J., Farnier, K., and Miller, R. E. (2020). Dung mimicry in Typhonium (Araceae): explaining floral trait and pollinator divergence in a widespread species complex and a rare sister species. Bot. J. Linn. Soc. 193, 375–401. doi: 10.1093/botlinnean/boaa021
Schiestl, F. P.. (2010). The evolution of floral scent and insect chemical communication: evolution of floral scent. Ecol. Lett. 13, 643–656. doi: 10.1111/j.1461-0248.2010.01451.x
Schiestl, F. P.. (2015). Ecology and evolution of floral volatile-mediated information transfer in plants. New Phytol. 206, 571–577. doi: 10.1111/nph.13243
Sheehan, H., Hermann, K., and Kuhlemeier, C. (2012). Color and scent: how single genes influence pollinator attraction. Cold Spring Harb. Symp. Quant. Biol. 77, 117–133. doi: 10.1101/sqb.2013.77.014712
Souza-Alonso, P., González, L., López-Nogueira, A., Cavaleiro, C., and Pedrol, N. (2018). Volatile organic compounds of Acacia longifolia and their effects on germination and early growth of species from invaded habitats. Chem. Ecol. 34, 126–145. doi: 10.1080/02757540.2017.1404584
Staveley, J. P., Law, S. A., Fairbrother, A., and Menzie, C. A. (2014). A causal analysis of observed declines in managed honey bees (Apis mellifera). Hum. Ecol. Risk Assess. Int. J. 20, 566–591. doi: 10.1080/10807039.2013.831263
Steinbauer, M. J., Schiestl, F. P., and Davies, N. W. (2004). Monoterpenes and epicuticular waxes help female autumn gum moth differentiate between waxy and glossy eucalyptus and leaves of different ages. J. Chem. Ecol. 30, 1117–1142. doi: 10.1023/B10.1023/B:JOEC.0000030267.75347.c1
Theis, N.. (2006). Fragrance of Canada thistle (Cirsium arvense) attracts both floral herbivores and pollinators. J. Chem. Ecol. 32, 917–927. doi: 10.1007/s10886-006-9051-x
Tholl, D., Boland, W., Hansel, A., Loreto, F., Röse, U. S. R., and Schnitzler, J.-P. (2006). Practical approaches to plant volatile analysis. Plant J. 45, 540–560. doi: 10.1111/j.1365-313X.2005.02612.x
Vanbergen, A. J., and the Insect Pollinators Initiative (2013). Threats to an ecosystem service: pressures on pollinators. Front. Ecol. Environ. 11, 251–259. doi: 10.1890/120126
Vas, G., and Vékey, K. (2004). Solid-phase microextraction: a powerful sample preparation tool prior to mass spectrometric analysis. J. Mass Spectrom. 39, 233–254. doi: 10.1002/jms.606
Vuts, J., Woodcock, C. M., Caulfield, J. C., Powers, S. J., Pickett, J. A., and Birkett, M. A. (2018). Isolation and identification of floral attractants from a nectar plant for the dried bean beetle, Acanthoscelides obtectus (Coleoptera: Chrysomelidae, Bruchinae). Pest Manag. Sci. 74, 2069–2075.
Waelti, M. O., Muhlemann, J. K., and Schiestl, F. P. (2008). Floral odour and reproductive isolation in two species of Silene. J. Evol. Biol. 21. doi: 10.1111/j.1420-9101.2007.01461.x
Wang, X., Wen, M., Qian, X., Pei, N., and Zhang, D. (2020). Plants are visited by more pollinator species than pollination syndromes predicted in an oceanic island community. Sci. Rep. 10, 13918. doi: 10.1038/s41598-020-70954-7
Watson, K., and Stallins, J. A. (2016). Honey bees and colony collapse disorder: a pluralistic reframing: honey bees and colony collapse disorder. Geogr. Compass 10, 222–236. doi: 10.1111/gec3.12266
Wright, G. A., and Schiestl, F. P. (2009). The evolution of floral scent: the influence of olfactory learning by insect pollinators on the honest signalling of floral rewards. Funct. Ecol. 23, 841–851. doi: 10.1111/j.1365-2435.2009.01627.x
Keywords: solid-phase microextraction, crop pollination, floral traits, wild pollinators, native vegetation, agriculture
Citation: Latinovic A, Nichols DS, Adams VM and McQuillan PB (2022) Grouped SPME Comparison of Floral Scent as a Method of Unlocking Phylogenetic Patterns in Volatiles. Front. Ecol. Evol. 10:795122. doi: 10.3389/fevo.2022.795122
Received: 14 October 2021; Accepted: 14 January 2022;
Published: 11 February 2022.
Edited by:
Juergen Gross, Julius Kühn-Institut, GermanyReviewed by:
Martin James Steinbauer, La Trobe University, AustraliaJohannes Stökl, University of Bayreuth, Germany
Copyright © 2022 Latinovic, Nichols, Adams and McQuillan. This is an open-access article distributed under the terms of the Creative Commons Attribution License (CC BY). The use, distribution or reproduction in other forums is permitted, provided the original author(s) and the copyright owner(s) are credited and that the original publication in this journal is cited, in accordance with accepted academic practice. No use, distribution or reproduction is permitted which does not comply with these terms.
*Correspondence: Adelina Latinovic, YWRlbGluYS5sYXRpbm92aWNAdXRhcy5lZHUuYXU=