- 1Naturalis Biodiversity Center, Leiden, Netherlands
- 2Institute of Biology, Leiden University, Leiden, Netherlands
- 3Department of Multiphase Chemistry, Max Planck Institute for Chemistry, Mainz, Germany
- 4Department of Botany, Instituto Nacional de Pesquisas da Amazônia, Manaus, Brazil
- 5Department of Environmental Engineering, Federal University of Parana, Curitiba, Brazil
- 6Department of Pharmacy and Biomedical Sciences, La Trobe University, Bendigo, VIC, Australia
- 7Division of Plant Sciences, Institute of Biology, University of Graz, Graz, Austria
Biological particles suspended in the atmosphere have a crucial role in the dynamics of the biosphere underneath. Although much attention is paid for the chemical and physical properties of these particles, their biological taxonomic identity, which is relevant for ecological research, remains little studied. We took air samples at 300 meters above the forest in central Amazonia, in seven periods of 7 days, and used high-throughput DNA sequencing techniques to taxonomically identify airborne fungal and plant material. The use of a molecular identification technique improved taxonomic resolution when compared to morphological identification. This first appraisal of airborne diversity showed that fungal composition was strikingly different from what has been recorded in anthropogenic regions. For instance, basidiospores reached 30% of the OTUs instead of 3–5% as found in the literature; and the orders Capnodiales and Eurotiales—to which many allergenic fungi and crop pathogens belong—were much less frequently recorded than Pleosporales, Polyporales, and Agaricales. Plant OTUs corresponded mainly to Amazonian taxa frequently present in pollen records such as the genera Helicostilys and Cecropia and/or very abundant in the region such as Pourouma and Pouteria. The origin of extra-Amazonian plant material is unknown, but they belong to genera of predominantly wind-pollinated angiosperm families such as Poaceae and Betulaceae. Finally, the detection of two bryophyte genera feeds the debate about the role of long distance dispersal in the distribution of these plants.
Introduction
Biosphere and atmosphere form an interconnected system, dynamically shaped by the exchange of gases and biological material. The formation of bioaerosols—airborne organic particles associated with, and emitted from, living and dead organisms (Després et al., 2012)—is part of this exchange. In the last two decades, much insight has been gained on concentration, size classes and emission patterns of bioaerosols in Amazonia, information that is deeply related to atmospheric chemical and physical processes (Martin et al., 2010), and crucial for the understanding of cloud cycling and rainfall regimes. Fungal spores, for instance, form an important fraction of small sized bioaerosols that contributes significantly to hydrological cycling (Huffman et al., 2012), while larger pollen grains act as the so-called “giant condensation cloud nuclei,” forming more cloud droplets at lower supersaturations than most other aerosol particles, thereby facilitating rain formation (Pöhlker et al., 2016).
It is, however, the largely unknown taxonomic identity of some of these airborne particles that will inform us about biological processes in the forest, such as spore dispersal, plant pollination and population dynamics. In a forest environment, turbulent vertical fluctuations in wind and the coherency in vertical eddy motion promote the uplift of plant and fungal diaspores (i.e., dispersal units), as well as pollen, much above the canopy. Their subsequent transportation by wind, as airborne, is part of a fundamental process in forest dynamics: Long Distance Dispersal, i.e., the movement of propagules both outside the stand geographic limits and outside the genetic neighborhood area of individuals (Robledo-Arnuncio et al., 2014). Long Distance Dispersal shapes ecological and evolutionary process, such as gene flow among populations and the geographical distribution of plants (Jordano, 2017), connecting local to regional scales in biogeographic theories (Hubbell, 2001). We therefore believe that knowledge on airborne taxonomic composition in Amazonia opens up multiple research possibilities on the role of dispersal in ecology and evolution of a major pristine ecosystem. Furthermore, from a societal point of view, the transport of allergenic microorganisms and plant pathogens has an impact on human health and the world economy (Genitsaris et al., 2011; Meyer et al., 2017).
The international project “Amazon Tall Tower Observatory” (ATTO, see Andreae et al., 2015) is an attempt to understand how regional dynamics affect local scale dynamics in Amazonia, with scientific questions that encompass several research fields. Described as the “laboratory in the sky [that] will sample some of the last unspoiled air on the planet” (Wade, 2015), the 325 m height tower of the project allowed us to capture biological material being transported into the atmosphere above a pristine forest canopy in Central Amazonia (Figure 1). Historical challenges associated with the study of airborne particles include the capture, and the determination of their taxonomic identity with the very limited discriminative power of morphological identification. Here, we addressed these challenges by using the top of the ATTO tower infrastructure for continuous sampling, combined with high-throughput DNA sequencing for the taxonomic identification. We aimed at revealing the diversity of fungal and plant material in the Amazonian atmosphere.
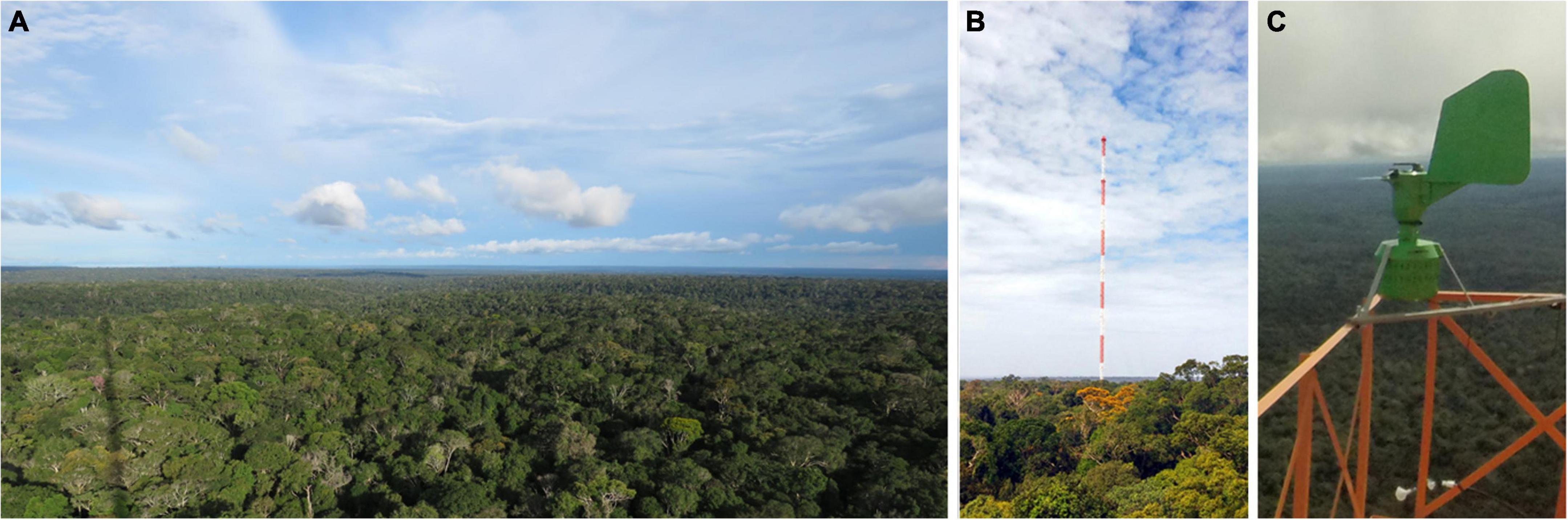
Figure 1. View of the Amazon forest from the ATTO tower at 100 m height (A). View of the ATTO tower (B). Spore sampler installed at the edge of the tower at 300 m height (C). Photos by SMO (A,C) and Jorge Saturno MPI-C (B).
Methods
Field sampling
The air sampling was carried out at 300 m height on the top of the ATTO tower (S 2° 8.752′ W 59° 0.335′).1 The tower is located in a biological reserve—Reserva Biológica do Uatumã—ca. 150 km distance from Manaus, Brazil, the largest city of Central Amazonia. A total of seven periods of 7 days of continuous sampling were carried out in April, September and October 2017; and in March 2018.
The equipment used was a Hirst-type spore sampler (Burkard 7 Day Recording Volumetric Spore Samplers, Burkard Scientific, Uxbridge, United Kingdom) that can run automatically 24 h per day, for 7 days. This equipment has a beak that sucks air from the environment directly against an adhesive tape. The tape is mounted on a clockwork-driven drum that turns on a regular speed of 2 mm per hour.
Laboratory—DNA extraction and sequencing
The seven sampling tapes, corresponding to 7 weeks of sampling, were cut in pieces that corresponded to 12 h sampling each, which amounted to 96 pieces—the last sampling week was 1 day (= 2 samples) shorter. All samples, and another 9 negative controls prepared regularly during the preparation of the samples, were placed into 2 ml Eppendorf tube each for DNA extraction.
The DNA of the particles captured by the spore sampler was extracted following specific methodology (Kraaijeveld et al., 2015). The ITS2 region was amplified with the forward primer ITS3 and the reverse primer ITS4 (White et al., 1990). These primers also contained unique Ion Xpress barcodes for individual sample identification. The PCR reactions were carried out in (25 μl) using 5 μl 5 × buffer, 0.5 μl 2.5 mM dNTP’s, 1.25 μl 10 mM of the respective forward primer and the reverse primer ITS4 coupled with Ion XpressTM barcodes for individual sample identification, 1 μl 25 mM MgCl2, 0.75 μl 100 mM BSA, 0.25 μl 100% DMSO, 0.5 μl Phire HS2 Taq polymerase (Thermo Fisher Scientific), and 4 μl of DNA template. The following PCR program was used: 3 min at 98°C, 35 cycles of 5 s at 98°C, 10 s at 50°C, and 30 s at 72°C, and 5 min at 72°C. An equimolar pool was made by measuring the amplified samples on the Qiaxcel using the DNA Screening Kit (QIAgen) followed by pooling equimolar with the QIAgility (Qiagen) pipetting robot. A cleanup of the pool was done with 0.9× (Macherey-Nagel). NucleoMag NGS clean-up and size select beads and a final quality and quantity check was performed with the Agilent 2,100 Bioanalyzer High Sensitivity DNA chip (Agilent Technologies). The final pool was diluted according to the calculated template dilution factor to target 10–30% of all positive ISPs. The template preparation of this pool was carried out on an Ion One Touch instrument with the Ion PGM Template OT2 400 kit (Life Technologies) according to manual 7,218 v3.0. The ion sphere quality control kit was used to check quality of the Ion One Touch2 400 ion sphere particles on a Life Qubit 2.0. The ion spheres were loaded on a 318 chip v2 and sequenced with the ion PGM sequencing 400 kit (Life Technologies) on an Ion-Torrent personal genome machine (Life Technologies, Thermo Fischer Scientific, United States). Template preparation and enrichment (Thermo Fisher Scientific) were according to the manufacturers protocol 7218Rev3.0.
Bioinformatics pipeline
The raw sequence reads were demultiplexed, filtered, clustered and blasted against a local ITS database using scripts on the Galaxy platform (Giardine et al., 2005). Because DNA extraction and sequencing was carried out in two different pools in the lab, the bioinformatics pipeline was also carried out twice, one per batch. Primers were first trimmed with Cutadapt (Martin, 2011), a tool that removes primers and discards reads that are below a given length threshold. In order to improve sequence analysis, we used PRINSEQ (Schmieder and Edwards, 2011) to graphically visualize sequence length distribution and base quality distribution. After establishing minimum and maximum sequence lengths, based on the visual analysis of the data, we kept all sequences from 270 to 440 bp—in order to avoid biases related to sequence size during the clustering procedure—and discarded sequences containing Phred value below 10. Although this was the threshold selected for the analysis, the visual inspection of PRINSEQ graphs showed that most of the read positions reached quality scores above 20 and just a few, mostly above 480 bp read length, had values between 10 and 20. Clustering was performed with USEARCH, using the default option of 98%. Obtained Operational Taxonomic Units (OTUs) were blasted against a local ITS database from NCBI (latest 20/11/2020), using the taxonomy provided by the database. OTUs resolved to NCBI accession numbers with unknown taxonomy or N/A were filtered out. Tag jumping analysis reduced the number of reads in ∼4.5–6% especially from lab contamination detected in negative controls.
Choices during data analysis
Frequency of OTUs in samples: the indefinite number of copies that can be produced during amplification might bias the abundance of a given OTU in a single sample. Therefore, per sample, only the presence or absence of a given OTU was recorded. As a consequence, in order to rank the taxa according to their frequency in the atmosphere and to present the results in the manuscript, we used the number of samples where a given OTU was found. Both groups showed that most of the OTUs recorded were present in less than 5 samples (Supplementary Figures 1A,B). Although OTU frequency and abundance (which takes into account the number of reads) were well correlated, the high variation of number of reads in a single sample (= abundance) does not reflect how frequently an OTU was captured through time (Supplementary Figures 1C,D).
Taxonomic level: we opted for a conservative approach concerning the blast hits, by choosing to report and discuss taxonomic composition at genus level. In an experimental approach for the identification of pollen samples with ITS2, 96% of taxa present in a controlled database were correctly identifiable at the genus level and 70% at the species level (Keller et al., 2015).
Best practices
Metabarcoding involves sample collection, DNA extraction, amplification and sequencing, and bioinformatics analysis. Potential biases in each of these steps were evaluated (see Table 1) in the light of the note of caution published by Zinger et al. (2019).
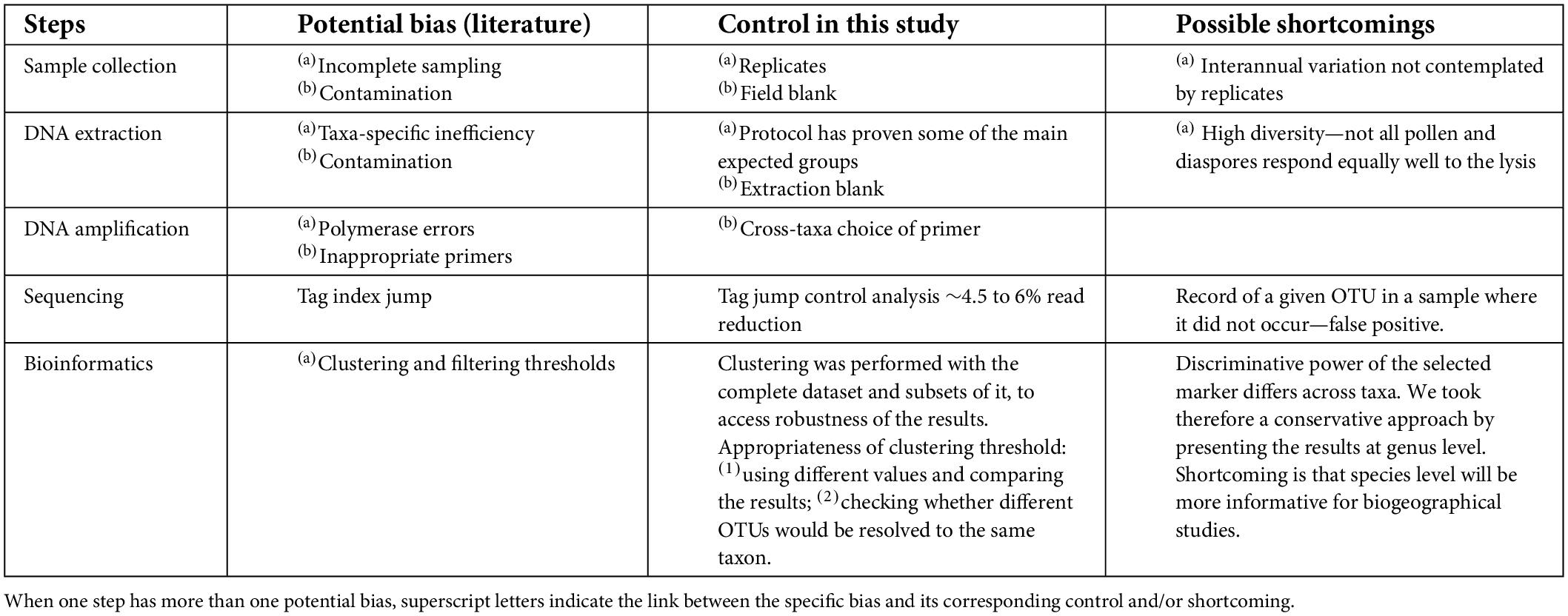
Table 1. Best practices summarized for each step of the data generation. Each step (first column) includes its own potential biases as mentioned in the literature (second column), the controls as applied in this study (third column), and the possible shortcomings (fourth column), which are also dealt with in the manuscript discussion section.
Results
The ITS2 DNA sequences obtained from sequencing of 96 samples, recorded in seven separated periods of seven continuous days of sampling, and clustered at 98% similarity before blasting procedure, were assigned to a total of 4,209 Operational Taxonomic Units (OTUs) that corresponded with > 97% identity to sequences deposited in the National Center for Biotechnology Information (NCBI). OTUs matching to NCBI sequence accessions without any taxonomic identification were not used here. This previous selection, together with tag jumping analysis, carried out to filter out sample contamination (see section “Methods”), reduced the number of OTUs analyzed in this study to 1,409.
The 1,409 OTUs corresponded to 1,202 Fungi and 207 Viridiplantae. Following the classification system used by NCBI, Viridiplantae (onward “Plants”) OTUs corresponded to 177 vascular plants (Streptophyta, classes Magnoliopsida, and Pinopsida), five bryophytes (Streptophyta, class Bryopsida), two green algae in Streptophyta (Streptophyta, class Klebsormidiophyceae), and 23 green algae in Chlorophyta. A total of 521 OTUs (36.7%) were detected in only one sample and the most frequent OTU was found in 59 samples.
The success rate of taxonomic identification of OTUs through blasting differed substantially between Fungi and Plants. Around 25% of the Fungi (317 OTUs) corresponded to NCBI sequences with unknown or unrecorded taxonomic identity at order level and below, and around 35% at genus level (417 OTUs in both), while only 6% (12 OTUs) of the Plants OTUs—mostly among the green algae—corresponded to NCBI sequences with unknown or unrecorded taxonomic identity at order level, and 11 OTUs and six OTUs at family and genus levels, respectively.
The fungal OTUs were resolved to 62 orders; the four richest orders—Pleosporales, Polyporales, Agaricales, and Xylariales—corresponded to 36% of the records, summing up to around 75% of the records with the next six orders (Figure 2A). Of the 395 genera identified (Supplementary Material), 105 were detected in only one sample and 49 in more than 15 samples. Most of the very frequent OTUs also belonged to the four richest orders (Figure 2C).
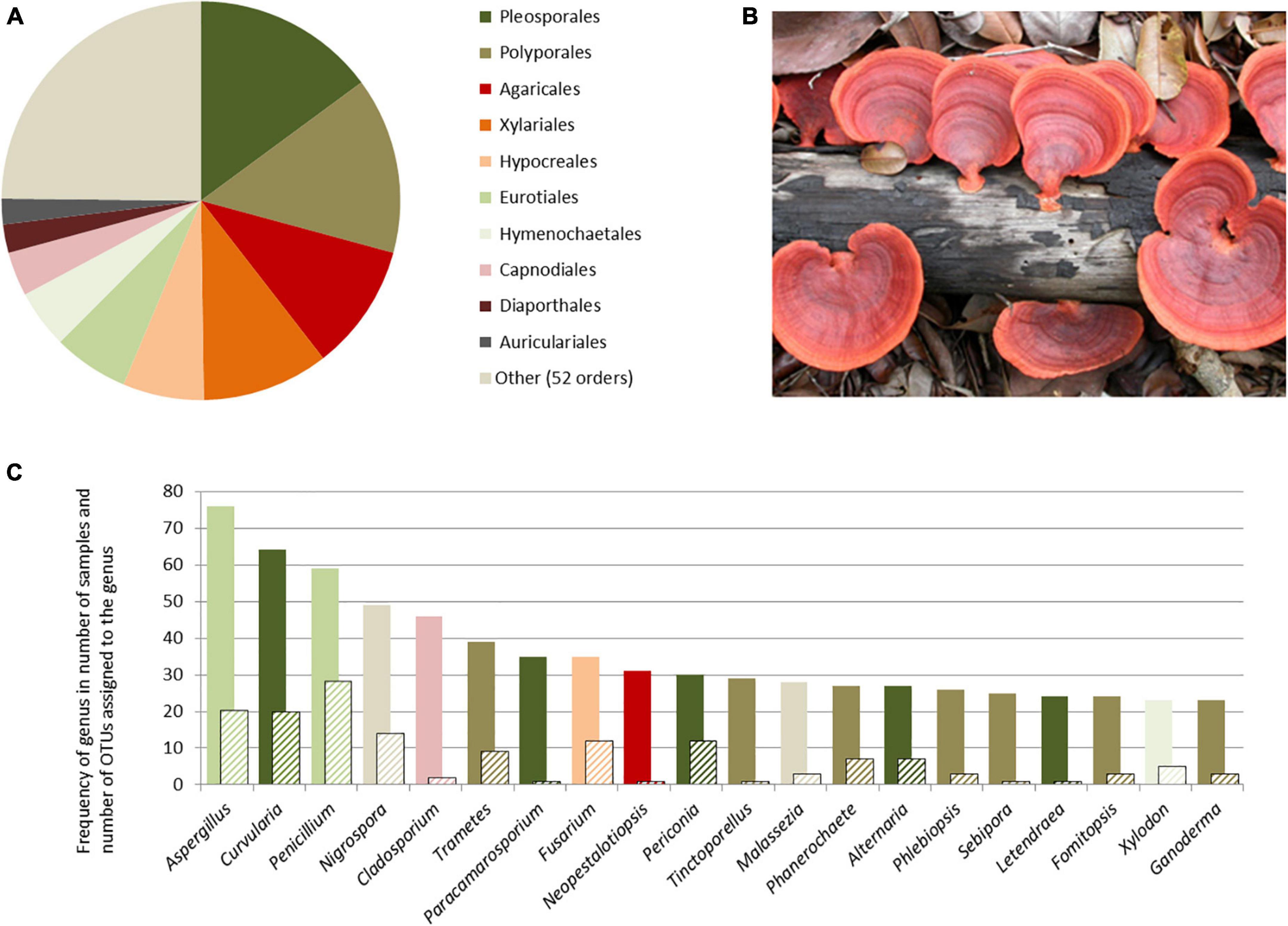
Figure 2. Overview of the taxonomic identification of fungal sequences extracted from air samples taken in Reserva Biológica do Uatumã, Central Amazonia, based on ITS2 target region. (A) Number of OTUs identified per fungi order; (B) Pycnoporus sanguineus (= Trametes sanguinea in NCBI) on a decaying log in Central Amazonia, a representative of one of the most frequently captured fungi genera; (C) frequency of genera in terms of number of samples (colored bars) and number of OTUs assigned to each genus (hatched bars)—column color refers to the order they belong, following (A).
The OTUs of vascular plants were resolved to 24 orders and 53 families. A total of 12 families included 55% of the OTUs (Figure 3A). The most frequently sampled genera are mostly represented by only one OTU, and five of them were not among the 12 OTU- richest families (Figure 3C). Poaceae, although the second OTU- richest family, had several genera detected only once or in very few samples. Out of 123 genera, 73 (59%) occur naturally in the Amazon Basin and another 20 (16%) in South America. Among the other genera detected, which do not naturally occur in South America, five are known to be cultivated in the region.
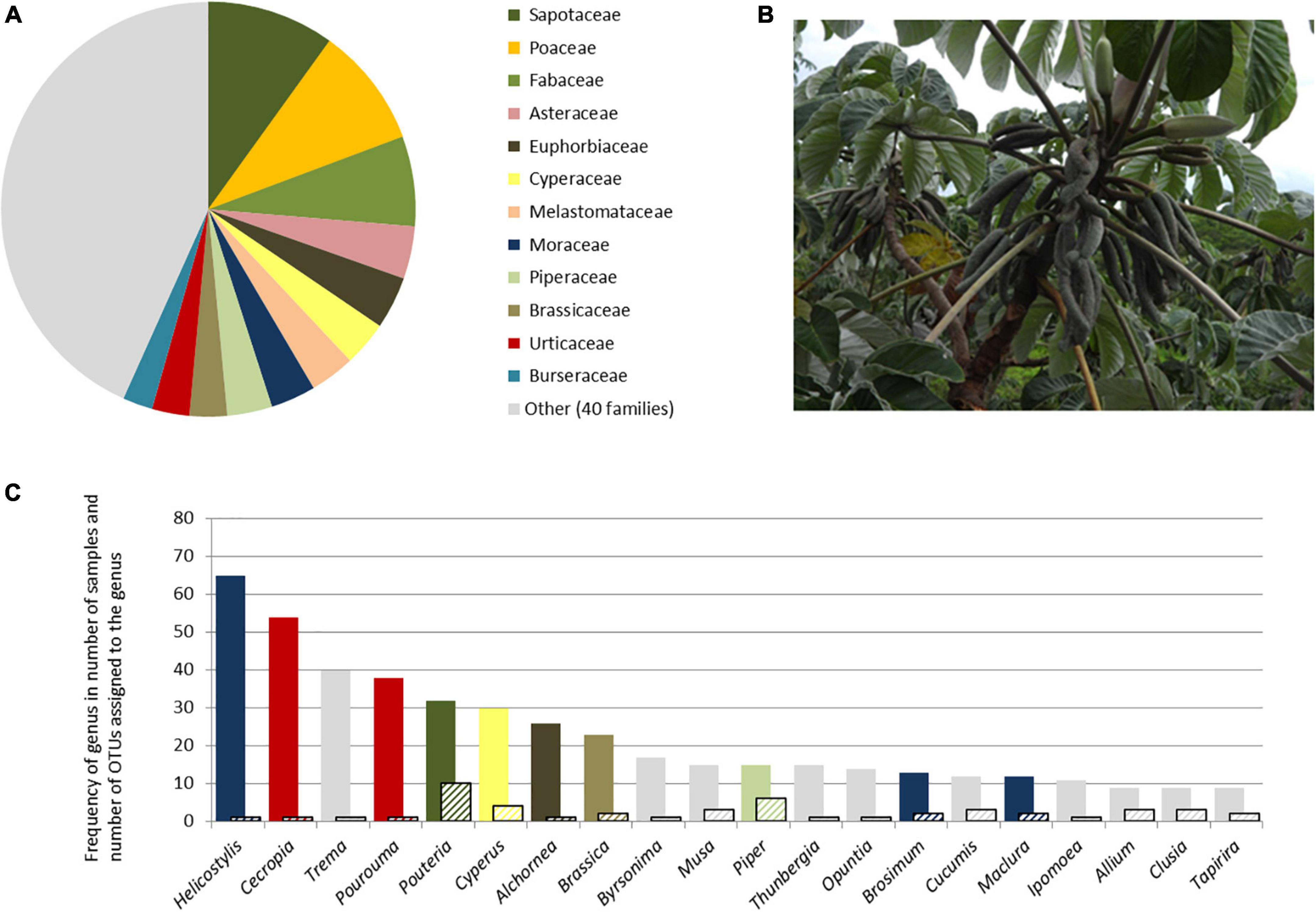
Figure 3. Overview of the taxonomic identification of plant sequences extracted from air samples taken in Reserva Biológica do Uatumã, Central Amazonia, based on ITS2 target region. (A) Number of OTUs identified per plant family; (B) Cecropia sp., one of the most frequently captured angiosperm genera; (C) frequency of genera in terms of number of samples (colored bars) and number of OTUs assigned to each genus (hatched bars)—column color refers to the family they belong, following (A).
The OTUs corresponding to bryophyte species belonged to the genera Octoblepharum and Pilosium. Among the 26 algae OTUs, the richest family was Trebouxiaceae (6 OTUs), the most widespread lichen-forming algal family, with genera such as Trebouxia and Myrmecia.
Discussion
Fungi diversity: Sign of a healthy environment
A number of the most frequent genera recorded in this study have wide geographical distribution, high abundance in a variety of environments—including indoor environment—and vast morphological documentation. Aspergillus, Nigrospora, Penicillium, Curvularia, and Cladosporium fall in this category and were also detected in the first morphological study of air samples taken from 300 m at the ATTO tower (Barbosa, 2018). The improved detection power given by molecular techniques became, however, clear in the frequent record of bracket fungi, such as Trametes and Fomitopsis. These taxa have been collected in Amazonia during inventories (Gibertoni et al., 2016), but not recorded in air samples when identification was solely based on spore morphology, probably due to the lack of enough morphological variation in some groups. The same has been documented in a study in Cuba: although the presence of these two polypore genera was known from ground inventories, the morphological identification of the airborne material only recorded the genus Ganoderma, which is frequently illustrated in the literature (Almaguer-Chávez et al., 2018). As anticipated, the use of a molecular technique is complementary to, and can fill some of the gaps of the morphological identification.
Genera containing endophytic fungi species were also among the most frequently recorded OTUs. Endophytic fungi live in association with plant tissues and are frequently investigated due to the potential discover of new molecules with antifungal activity. In our study, the frequently recorded genera Periconia, Tinctoporellus, Fusarium, Phanerochaete, and Alternaria have also been recorded across different Brazilian ecosystems in plant tissues, as well as the less frequent Phomopsis, Talaromyces, Xylaria, and Didymella (see review in Bezerra et al., 2019). Air samples offer an insight about the relative frequency of their dispersal in the environment. On the other hand, a deeper taxonomic resolution, including other loci, is imperative when defining the role of some taxa in the environment. Phoma is an example of a species rich genus of endophytes, but also of important plant-associated pathogens and lichenicolous anamorphic fungi, in which ITS regions alone are insufficient to distinguish closely related species, and ongoing phylogenetic research is still unraveling its diversity (Lawrey et al., 2012; Hou et al., 2020). Other genera recorded in this study of which an interpretation of their ecological role depends on better taxonomic resolution are Neopestalotiopsis, Pseudopestalotiopsis, and Pestalotiopsis. In this sense, the list presented here is a preliminary exploration of the taxonomic diversity expected.
Our dataset showed a much higher relative importance of polypore fungi, in comparison with studies in highly anthropogenic areas. The family Polyporaceae yielded the highest number of genera, a consequence of a vast diversity of taxa that are saprobes on dead wood and essential for nutrient cycling. In Northern Italy, the most frequent and widely distributed genera of fungi, detected by the same sampling and molecular identification technique used here, were those allergenic to humans or containing well-known plant and animal pathogens, for example, Botrytis, Periconia, Acremonium, Candida, Cryptococcus (Banchi et al., 2018). The order Capnodiales that includes many of these taxa was the best represented order in every site covered by the study, while it ranked 8th in OTU richness in Amazonia. Furthermore, while basidiospores do not exceed 2% in inventories from anthropogenic areas, it amounted to a staggering 30% of the OTUs in our dataset, using exactly the same target region. In previous studies of airborne composition in urban areas, the much lower percentages of basidiospores when compared to ascospores has been explained by the notion that the use of ITS2 as target region causes a bias toward ascospores (Bellemain et al., 2010). Here we argue that the difference is probably much more related to the natural environment. Future combination of short and long reads will certainly increase taxonomic accuracy, as reported by Ritter et al. (2020).
There is mounting evidence that natural environments have the potential to improve human health. The biodiversity hypothesis of von Hertzen et al. (2011) emphasizes the causal relationship between the increase in immune dysfunction and in inflammatory diseases with the decrease in diversity of the environmental microbiota. Under the light of this hypothesis, our results on airborne diversity offers clear evidence of the habitat quality given by the presence of the forest.
Airborne plant material: A biased sample of the forest
In a very general sense, two factors seem to influence the taxonomic composition of the airborne material: the high abundance of certain Amazonian taxa, such as the genera Pouteria and Pourouma (ter Steege et al., 2013), and the wind pollination syndrome. The massive presence of the genera Helicostylis and Cecropia in our samples, as well as Trema, Cyperus, and Alchornea is in line with previous results from palynological data from cores in the Amazon basin but also from present pollen records (Mendes et al., 2021). It has also been shown that, in the present Amazonian vegetation, the mean relative abundance of the families to which they belong correlate significantly with modern pollen assemblages (Gomes et al., 2020; Mendes et al., 2021). Wind pollination syndrome is apparently important for the presence of extra-Amazonian taxa. Among the extra-Amazonian taxa, we found well-known wind-pollinated groups such as the Asteraceae genus Ambrosia and several representatives of the families Poaceae, Betulaceae, and Fagaceae. In these taxa, spherical and small pollen grains, released from exposed anthers, promote their uptake and carriage by wind (Ackerman, 2000). Based on morphological features, wind pollination is assumed to occur in only 18–20% of the angiosperm families. From a community based approach, the estimated rate is on average 15%, but much lower in tropical forests, where ca. of 98% of the species are supposed to show animal-mediated pollination (Ollerton et al., 2011). As a matter of fact, botanists may assume animal-mediated pollination more frequently than it actually occurs, apparently due to the lack of experimental work. One of the most abundant species in Amazonia, Mauritia flexuosa L. (Khorsand and Koptur, 2013) is an example where pollinator exclusion experiments revealed an unexpected dependency of wind pollination. The recording of high amounts of airborne pollen of Amazonian taxa may therefore guide future species level experimental research.
The relationship between the current pollen assemblage and forest species composition is a powerful tool to reconstruct past vegetation. Palynological data from cores in the Amazon basin is used to understand the long-term effects of climate change on vegetation. But the comparison of pollen deposition and vegetation revealed that important taxa might be completely absent from the pollen record, while others are overrepresented (Gosling et al., 2009). The discrepancy between taxonomic richness per family in our records and in the documented forest composition is very clear. Apart from Fabaceae, Sapotaceae, and Melastomataceae, the most species rich families in Amazonia (Rubiaceae, Melastomataceae, Myrtaceae, Lauraceae, and Annonaceae, to cite a few) are not among the 12 most represented in the airborne material. The discrepancy is clearly related to the different mechanisms of pollen release and chances of the pollen grains to become airborne. As observed by Guimarães et al. (2014) in a study of pollen and spores from a lake in Southeastern Amazonia, species pollinated by insects, birds or bats do not give a regional signal, their pollen records being the result of local carriage of pollen by rainwater and catchments to lakes. A good way of reducing misinterpretation of palynological data is to identify these biases in present time studies. In this line, our list offers an independent line of evidence for current pollen assessments.
Finally, the recording of only two bryophyte genera has a much greater scientific importance than the numbers suggest. Laboratory experiments have shown how variation on wind speed and turbulence affect spore release (Johansson et al., 2014), and that settling velocity of spore dispersal is correlated to spore size (Zanatta et al., 2016). Long distance dispersal events, however, are a product of stochastic conditions that cannot be covered by experimental settings, and have been invoked to explain bryophyte species distributions based on ecological analysis (Barbé et al., 2016). Two out of the three species recorded in this study are among the 10 most common species in Amazonia (Mota de Oliveira and ter Steege, 2013), which would lend support to the hypothesis that bryophyte dispersal at large scale is stochastic, and consequently, species relative abundance in the metacommunity will strongly influence the chance of being dispersed far from the mother plant (Mota de Oliveira and ter Steege, 2015). Further sampling will allow testing this hypothesis.
False positives, false negatives, and the variable taxonomic accuracy
The applied technique records the presence of a DNA fragment, the interpretation of it into pollen or spore records is an assumption based on the fact that there is ample evidence of their transportation as viable cells, while the uplift of plant debris due to forest burning would hardly result in extractable DNA content. False positives can still occur, due to “probable contamination that cannot be discarded, such as the skin fungus Malassezia, and”, among others (Badotti et al., 2017). False negatives can occur at any stage of air sampling, namely collection, storage/transportation, processing/extraction, and analysis. Repeated use of the same technique, as well as experimental studies focusing on the lysis of spores and pollen and on the success rate of polymerase chain reaction in different taxa are desirable developments that can minimize the uncertainty given by false negatives (Nilsson et al., 2014). But perhaps the largest source of false negatives is given by the geographical and taxonomic biases in the molecular databases. As an example, the frequent occurrence of the endophytic fungi genus Letendraea in our records and the complete lack of the Amazonian Letendraeopsis raise the question whether the latter was not recorded due to the lack of molecular sequences deposited in NCBI. In our study, the staggering amount of 74% of the final OTU clusters could not be resolved with more than 97% similarity to any sequence deposited in NCBI. Accordingly, taxonomic accuracy varies with the taxonomic level and the extent of previous phylogenetic research done for a given taxon. The use of additional barcodes is also desirable in many cases where ITS1 and ITS2 alone are not enough for proper identification (Badotti et al., 2017).
Gained insights and future prospects
Our study showed, among others, that airborne species composition is intrinsically related to the environment underneath, but also receives input from extra-Amazonian taxa, especially those that are known to have an effective pollen spreading strategy. The most striking difference with previously reported airborne composition is the much lower relative presence of allergenic fungi and plant pathogens. The finding of three bryophyte species is remarkably important because it provides rare, yet robust evidence for the hypothesized role of long distance dispersal in the distribution patterns of these plants in the Amazon. The investigation of the taxonomic composition of airborne biological material opens up completely new opportunities to test hypothesis of biological processes in Amazonia.
Data availability statement
The molecular datasets generated for this study can be found in the NCBI under BioProject ID: PRJNA814506 (https://www.ncbi.nlm.nih.gov/bioproject/PRJNA814506).
Author contributions
SM: conceptualization, formal analysis, and writing—original draft preparation. SM, ED, MS, JR, and MP: methodology. SM and ED: investigation. SM, CB, GC, AN, RG, MS, and JK: resources. SM, JR, and MP: data curation. SM, RG, MS, SW, BW, and JK: funding acquisition. All authors writing—review and editing, project administration (in different aspects).
Funding
This work was supported by the Brazilian Ministério da Ciência, Tecnologia e Inovação (MCTI/FINEP contract 01.11.01248.00), German Federal Ministry of Education and Research (BMBF contract 01LB1001A and 01LK1602B), Amazon State University (UEA), FAPESP, CNPq, FAPEAM, LBA/INPA and SDS/CEUC/RDS-Uatumã, and Stichting Het Kronendak in the Netherlands for a pilot grant.
Acknowledgments
We acknowledge “Het Kronendak Stichting” for the support, without which this project would not start. We thank Dr. József Geml for discussions on the bioinformatic tools; Dr. Hanster Steege for comments on the manuscript and pictures in Figures 2, 3; the staff of the ATTO project field station; the staff of Bureau Voorlichting for including one of the fieldwork trips of this study in their Dutch documentary “De Toren,” about the natural history collection towers of Naturalis Biodiversity Center.
Conflict of interest
The authors declare that the research was conducted in the absence of any commercial or financial relationships that could be construed as a potential conflict of interest.
Publisher’s note
All claims expressed in this article are solely those of the authors and do not necessarily represent those of their affiliated organizations, or those of the publisher, the editors and the reviewers. Any product that may be evaluated in this article, or claim that may be made by its manufacturer, is not guaranteed or endorsed by the publisher.
Supplementary material
The Supplementary Material for this article can be found online at: https://www.frontiersin.org/articles/10.3389/fevo.2022.789791/full#supplementary-material
Footnotes
References
Ackerman, J. D. (2000). Abiotic pollen and pollination: Ecological, functional, and evolutionary perspectives. Plant Syst. Evol. 222, 167–185. doi: 10.1007/BF00984101
Almaguer-Chávez, M., Aira, M. J., Rojas, T.-I., Fernández-González, M., and Rodríguez-Rajo, F.-J. (2018). New findings of airborne fungal spores in the atmosphere of Havana, Cuba, using aerobiological non-viable methodology. Ann. Agric. Environ. Med. 25, 349–359. doi: 10.26444/aaem/89738
Andreae, M. O., Acevedo, O. C., Araùjo, A., Artaxo, P., Barbosa, C. G. G., Barbosa, H. M. J., et al. (2015). The Amazon Tall Tower Observatory (ATTO): Overview of pilot measurements on ecosystem ecology, meteorology, trace gases, and aerosols. Atmos. Chem. Phys. 15, 10723–10776. doi: 10.5194/acp-15-10723-2015
Badotti, F., de Oliveira, F. S., Garcia, C. F., Vaz, A. B. M., Fonseca, P. L. C., Nahum, L. A., et al. (2017). Effectiveness of ITS and sub-regions as DNA barcode markers for the identification of Basidiomycota (Fungi). BMC Microbiol. 17:42. doi: 10.1186/s12866-017-0958-x
Banchi, E., Ametrano, C. G., Stanković, D., Verardo, P., Moretti, O., Gabrielli, F., et al. (2018). DNA metabarcoding uncovers fungal diversity of mixed airborne samples in Italy. PLoS One 13:e0194489. doi: 10.1371/journal.pone.0194489
Barbé, M., Fenton, N. J., and Bergeron, Y. (2016). So close and yet so far away: Long distance dispersal events govern bryophyte metacommunity re-assembly. J. Ecol. 104, 1707–1719.
Barbosa, C. G. G. (2018). Primary biological aerosol particles at the amazon tall tower observatory: Inventory and vertical distribution. Ph.D. thesis. Curitiba: Universidade Federal do Paraná.
Bellemain, E., Carlsen, T., Brochmann, C., Coissac, E., Taberlet, P., and Kauserud, H. (2010). ITS as an environmental DNA barcode for fungi: An in silico approach reveals potential PCR biases. BMC Microbiol. 10:189. doi: 10.1186/1471-2180-10-189
Bezerra, J. D. P., da Silva, L. F., and de Souza-Motta, C. M. (2019). “The explosion of brazilian endophytic fungal diversity: Taxonomy and biotechnological potentials,” in Advancing frontiers in mycology & mycotechnology: Basic and applied aspects of fungi, eds T. Satyanarayana, S. K. Deshmukh, and M. V. Deshpande (Singapore: Springer), 405–433.
Després, V., Huffman, J. A., Burrows, S. M., Hoose, C., Safatov, A., Buryak, G., et al. (2012). Primary biological aerosol particles in the atmosphere: A review. Tellus B Chem. Phys. Meteorol. 64:15598. doi: 10.3402/tellusb.v64i0.15598
Genitsaris, S., Kormas, K. A., and Moustaka-Gouni, M. (2011). Airborne algae and cyanobacteria: Occurrence and related health effects. Front. Biosci. 3, 772–787. doi: 10.2741/e285
Giardine, B., Riemer, C., Hardison, R. C., Burhans, R., Elnitski, L., Shah, P., et al. (2005). Galaxy: A platform for interactive large-scale genome analysis. Genome Res. 15, 1451–1455. doi: 10.1101/gr.4086505
Gibertoni, T. B., Medeiros, P. S., Soares, A. M. S., Gomes-Silva, A. C., Santos, P. J. P., Sotão, H. M. P., et al. (2016). The distribution of polypore fungi in endemism centres in Brazilian Amazonia. Fungal Ecol. 20, 1–6. doi: 10.1016/j.funeco.2015.09.012
Gomes, V. H. F., Mayle, F. E., Gosling, W. D., Vieira, I. C. G., Salomão, R. P., and ter Steege, H. (2020). Modelling the distribution of Amazonian tree species in response to long-term climate change during the Mid-Late Holocene. J. Biogeogr. 47, 1530–1540. doi: 10.1111/jbi.13833
Gosling, W. D., Mayle, F. E., Tate, N. J., and Killeen, T. J. (2009). Differentiation between Neotropical rainforest, dry forest, and savannah ecosystems by their modern pollen spectra and implications for the fossil pollen record. Rev. Palaeobot. Palynol. 153, 70–85. doi: 10.1016/j.revpalbo.2008.06.007
Guimarães, J. T. F., Souza-Filho, P. W. M., Alves, R., de Souza, E. B., da Costa, F. R., Reis, L. S., et al. (2014). Source and distribution of pollen and spores in surface sediments of a plateau lake in southeastern Amazonia. Quat. Int. 352, 181–196. doi: 10.1016/j.quaint.2014.06.004
Hou, L. W., Groenewald, J. Z., Pfenning, L. H., Yarden, O., Crous, P. W., and Cai, L. (2020). The phoma-like dilemma. Stud. Mycol. 96, 309–396. doi: 10.1016/j.simyco.2020.05.001
Hubbell, S. P. (2001). The unified neutral theory of biodiversity and biogeography. Princeton, NJ: Princeton University Press.
Huffman, J. A., Sinha, B., Garland, R. M., Snee-Pollmann, A., Gunthe, S. S., Artaxo, P., et al. (2012). Size distributions and temporal variations of biological aerosol particles in the Amazon rainforest characterized by microscopy and real-time UV-APS fluorescence techniques during AMAZE-08. Atmos. Chem. Phys. 12, 11997–12019. doi: 10.5194/acp-12-11997-2012
Johansson, V., Lönnell, N., Sundberg, S., and Hylander, K. (2014). Release thresholds for moss spores: The importance of turbulence and sporophyte length. J. Ecol. 102, 721–729. doi: 10.1111/1365-2745.12245
Jordano, P. (2017). What is long-distance dispersal? And a taxonomy of dispersal events. J. Ecol. 105, 75–84. doi: 10.1111/1365-2745.12690
Keller, A., Danner, N., Grimmer, G., Ankenbrand, M., von der Ohe, K., von der Ohe, W., et al. (2015). Evaluating multiplexed next-generation sequencing as a method in palynology for mixed pollen samples. Plant Biol. 17, 558–566. doi: 10.1111/plb.12251
Khorsand, R., and Koptur, S. (2013). New findings on the pollination biology of Mauritia flexuosa (Arecaceae) in Roraima, Brazil: Linking dioecy, wind, and habitat. Am. J. Bot. 100, 613–621. doi: 10.3732/ajb.1200446
Kraaijeveld, K., de Weger, L. A., Ventayol García, M., Buermans, H., Frank, J., Hiemstra, P. S., et al. (2015). Efficient and sensitive identification and quantification of airborne pollen using next-generation DNA sequencing. Mol. Ecol. Resour. 15, 8–16. doi: 10.1111/1755-0998.12288
Lawrey, J. D., Diederich, P., Nelsen, M. P., Freebury, C., Van den Broeck, D., Sikaroodi, M., et al. (2012). Phylogenetic placement of lichenicolous Phoma species in the Phaeosphaeriaceae (Pleosporales, Dothideomycetes). Fungal Divers. 55, 195–213. doi: 10.1007/s13225-012-0166-9
Martin, M. (2011). Cutadapt removes adapter sequences from high-throughput sequencing reads. EMBnet. J. 17, 10–12. doi: 10.1089/cmb.2017.0096
Martin, S. T., Andreae, M. O., Artaxo, P., Baumgardner, D., Chen, Q., Goldstein, A. H., et al. (2010). Sources and properties of Amazonian aerosol particles. Rev. Geophys. 48:RG2002. doi: 10.1029/2008RG000280
Mendes, L. A. D. S., Meneses, M. E. N. D. S., Behling, H., and Costa, M. L. D. (2021). Modern and fossil pollen record from region of the middle Araguaia River floodplain, Tocantins State (Brazil). Pesqui. Geociências 48. doi: 10.22456/1807-9806.111397
Meyer, M., Cox, J. A., Hitchings, M. D. T., Burgin, L., Hort, M. C., Hodson, D. P., et al. (2017). Quantifying airborne dispersal routes of pathogens over continents to safeguard global wheat supply. Nat. Plants 3, 780–786. doi: 10.1038/s41477-017-0017-5
Mota de Oliveira, S., and ter Steege, H. (2013). Floristic overview of the epiphytic bryophytes of terra firme forests across the Amazon basin. Acta Bot. Brasilica 27, 347–363.
Mota de Oliveira, S., and ter Steege, H. (2015). Bryophyte communities in the Amazon forest are regulated by height on the host tree and site elevation. J. Ecol. 103, 441–450. doi: 10.1111/1365-2745.12359
Nilsson, R. H., Hyde, K. D., Pawłowska, J., Ryberg, M., Tedersoo, L., Aas, A. B., et al. (2014). Improving ITS sequence data for identification of plant pathogenic fungi. Fungal Divers. 67, 11–19. doi: 10.1007/s13225-014-0291-8
Ollerton, J., Winfree, R., and Tarrant, S. (2011). How many flowering plants are pollinated by animals? Oikos 120, 321–326. doi: 10.1111/j.1600-0706.2010.18644.x
Pöhlker, M. L., Pöhlker, C., Ditas, F., Klimach, T., Hrabe de Angelis, I., Araújo, A., et al. (2016). Long-term observations of cloud condensation nuclei in the Amazon rain forest – Part 1: Aerosol size distribution, hygroscopicity, and new model parametrizations for CCN prediction. Atmos. Chem. Phys. 16, 15709–15740. doi: 10.5194/acp-16-15709-2016
Ritter, C. D., Dunthorn, M., Anslan, S., de Lima, V. X., Tedersoo, L., Nilsson, R. H., et al. (2020). Advancing biodiversity assessments with environmental DNA: Long-read technologies help reveal the drivers of Amazonian fungal diversity. Ecol. Evol. 10, 7509–7524. doi: 10.1002/ece3.6477
Robledo-Arnuncio, J. J., Klein, E. K., Muller-Landau, H. C., and Santamaría, L. (2014). Space, time and complexity in plant dispersal ecology. Mov. Ecol. 2:16. doi: 10.1186/s40462-014-0016-3
Schmieder, R., and Edwards, R. (2011). Quality control and preprocessing of metagenomic datasets. Bioinformatics 27, 863–864. doi: 10.1093/bioinformatics/btr026
ter Steege, H., Pitman, N. C. A., Sabatier, D., Baraloto, C., Salomão, R. P., Guevara, J. E., et al. (2013). Hyperdominance in the Amazonian Tree Flora. Science 342:1243092. doi: 10.1126/science.1243092
von Hertzen, L., Hanski, I., and Haahtela, T. (2011). Natural immunity. Biodiversity loss and inflammatory diseases are two global megatrends that might be related. EMBO Rep. 12, 1089–1093. doi: 10.1038/embor.2011.195
Wade, L. (2015). A pristine Amazon’s last stand. Science 347, 1051–1052. doi: 10.1126/science.347.6226.1051
White, T. J., Bruns, T., Lee, S., and Taylor, J. (1990). “Amplification and direct sequencing of fungal ribosomal RNA genes for phylogenetics,” in PCR protocols, eds M. A. Innis, D. H. Gelfand, J. J. Sninsky, and T. J. White (San Diego, CA: Academic Press), 315–322.
Zanatta, F., Patiño, J., Lebeau, F., Massinon, M., Hylander, K., de Haan, M., et al. (2016). Measuring spore settling velocity for an improved assessment of dispersal rates in mosses. Ann. Bot. 118, 197–206. doi: 10.1093/aob/mcw092
Keywords: airborne, Amazonia, bioaerosol, dispersal, eDNA, pollen, spores
Citation: Mota de Oliveira S, Duijm E, Stech M, Ruijgrok J, Polling M, Barbosa CGG, Cerqueira GR, Nascimento AHM, Godoi RHM, Taylor PE, Wolff S, Weber B and Kesselmeier J (2022) Life is in the air: An expedition into the Amazonian atmosphere. Front. Ecol. Evol. 10:789791. doi: 10.3389/fevo.2022.789791
Received: 25 April 2022; Accepted: 02 August 2022;
Published: 02 September 2022.
Edited by:
Domingos Cardoso, Federal University of Bahia, BrazilReviewed by:
Aristóteles Góes-Neto, Federal University of Minas Gerais, BrazilSiu Mui Tsai, University of São Paulo, Brazil
Copyright © 2022 Mota de Oliveira, Duijm, Stech, Ruijgrok, Polling, Barbosa, Cerqueira, Nascimento, Godoi, Taylor, Wolff, Weber and Kesselmeier. This is an open-access article distributed under the terms of the Creative Commons Attribution License (CC BY). The use, distribution or reproduction in other forums is permitted, provided the original author(s) and the copyright owner(s) are credited and that the original publication in this journal is cited, in accordance with accepted academic practice. No use, distribution or reproduction is permitted which does not comply with these terms.
*Correspondence: Sylvia Mota de Oliveira, c3lsdmlhLm1vdGFkZW9saXZlaXJhQG5hdHVyYWxpcy5ubA==
†ORCID: Sylvia Mota de Oliveira, orcid.org/0000-0002-1440-9718; Elza Duijm, orcid.org/0000-0002-6383-3419; Michael Stech, orcid.org/0000-0001-9804-0120; Marcel Polling, orcid.org/0000-0002-4439-3681; Cybelli G. G. Barbosa, orcid.org/0000-0001-6156-8749; Gabriela R. Cerqueira, orcid.org/0000-0001-9829-2088; Ricardo H. M. Godoi, orcid.org/0000-0002-4774-4870; Stefan Wolff, orcid.org/0000-0003-0103-4889; Bettina Weber, orcid.org/0000-0002-5453-3967; Jürgen Kesselmeier, orcid.org/0000-0002-4446-534X