- 1Muhlenberg College, Allentown, PA, United States
- 2Retired, Wilmington, DE, United States
Background and Aims: Bumblebees provide vital pollination services to both natural and agricultural ecosystems. Consequently their declines in species-diversity and population size over the last five decades is alarming. Direct contributors to these declines include pesticides, habitat loss, and disease. However, given that colony fitness is linked to foraging success, successful conservation requires mitigation of any anthropogenic practices that negatively impact foraging. Previous work has shown that agrochemical odor-pollution, including that of fungicides, can modulate bumblebee foraging behavior. This study investigates how odor pollution from three common fungicides (Safer® Brand Garden Fungicide II, Scotts® Lawn Fungus Control, and Reliant® Systemic Fungicide) affects Bombus impatiens’ floral-odor learning and recognition using an associative learning paradigm.
Methods: The effects of fungicide-odor pollution were tested in three ways: (1) background pollution during floral-odor learning; (2) background pollution during floral-odor recognition; and (3) point (localized) pollution during floral-odor recognition. Electroantennogram (EAG) recordings from B. impatiens confirmed the salience of all odor-stimuli and examined impacts of background fungicide-odor on antennal responses to floral-odor. To better understand how fungicide-odor structure related to behavioral data, scents were sampled (Solid Phase Microextraction) and analyzed using gas chromatography–mass spectrometry. Odors were then characterized using the Compounds Without Borders (CWB) vectorization method.
Conclusion: All fungicides tested disrupted floral-odor learning and recognition for at least one concentration tested, and Scotts® was universally disruptive at all tested concentrations. All fungicides induced EAG responses, indicating they provide perceivable odor stimuli. Interestingly, two of three tested fungicides (Scotts® and Reliant®) inhibit antennal responses to Monarda fistulosa odor. Odor characterization supports previous findings that sulfurous scents could be disruptive to odor-driven foraging behaviors. Inability for foraging bumblebees to associate to rewarding floral odors in the presence of fungicidal odor pollution could have negative large-scale implications for colony health and reproductive fitness.
Introduction
Bumblebees Are Critical Pollinators Facing Concerning Declines
The production of pollinator dependent crops has increased 300% worldwide over the last five decades, increasing human reliance on pollinator populations (ISPBES, 2016). Bombus spp. are integral to agricultural systems, as their larger sized hairy bodies and buzz-pollination behavior makes them efficient pollinators for multi-billion dollar crops such as tomatoes, peppers, squash, cucumbers, and berries (Cameron et al., 2011; Goulson et al., 2015). Unfortunately, an estimated half of over 4000 wild bee species in North America, including bumblebees, are in decline: showing both loss of absolute numbers and species diversity (Cameron et al., 2011; Goulson et al., 2015; Mathiasson and Rehan, 2019). Climate change, habitat loss, pathogen infections, and agrochemicals are all contributing and synergistic stressors (Cameron et al., 2011; Goulson et al., 2015; ISPBES, 2016). The loss of wild pollinators cannot be fully offset with use of managed honeybee and bumblebee colonies (Greenleaf and Kremen, 2006; Brittain et al., 2010; Potts et al., 2016; Winfree et al., 2018; Mathiasson and Rehan, 2019; Eeraerts et al., 2020). Declining bumblebee populations are not only concerning from an agricultural perspective, as they are critical pollinators in natural ecosystems as well (Corbet et al., 1981; Motten, 1986; Mänd et al., 2002; Hegland and Totland, 2008; Klein et al., 2008; van Heemert et al., 2015). Therefore, bumblebee conservation is an important component of sustaining pollination services.
Non-insecticidal Agrochemicals Are Pervasive and Problematic
A wide range of agrochemical compounds and their metabolites have been found in flower pollen and nectar, as well as on the body surfaces and colony wax of multiple bee species (Pettis et al., 2013; Bernauer et al., 2015; Goulson et al., 2015; David et al., 2016; Sgolastra et al., 2018). Agricultural chemicals are not only found on crops, but also commonly appear in bordering foliage where bees forage (Botías et al., 2017; Sgolastra et al., 2018). Sublethal effects of neonicotinoid insecticides are a rigorous area of study [as reviewed by Lundin et al. (2015)]. However, effects from other classes of agrochemicals, such as herbicides and fungicides, are less examined despite the fact that these chemicals are commonly used in both agricultural and urban settings; often in conjunction with neonicotinoids (Bernauer et al., 2015; Muratet and Fontaine, 2015; van de Merwe et al., 2018; Cullen et al., 2019; Meftaul et al., 2019). Indeed, some data indicate that fungicides are the most common agrochemicals found in the pollen loads of foraging honey bees and bumblebees in the United States (David et al., 2016; Christen et al., 2019). Despite the high LD50 values of many fungicides, there is evidence that chemical interactions between active ingredients and/or additives may synergistically increase insecticide toxicity to honeybees, solitary bee, and bumblebee species (Goulson et al., 2015; Tomé et al., 2016; Sgolastra et al., 2017, 2018). Moreover, direct negative effects of fungicides have also been found; i.e., chlorothalonil-induced decreases in bumblebee worker lifespan and queen reproductive fitness, and Cerconil (active ingredients: chlorothalonil and thiophanate-methyl) significantly increased mortality of honeybees and stingless bees (Bernauer et al., 2015; Tomé et al., 2016). Fungicides are frequently used in urban, suburban, and rural contexts, resulting in contamination of bordering natural flora. Further insight into the impacts of fungicides on bumblebees is a necessary component of conservation efforts.
Indirect Effects of Odor Pollution Are Facing Increasing Scrutiny
Bumblebees are capable of using odor information alone to locate resources (Spaethe et al., 2007; Sprayberry et al., 2013). Incomplete odor plumes, or complete blends of compounds at unnatural ratios, may not evoke the same natural behavior in insect pollinators (Hosler and Smith, 2000; Carde and Willis, 2008; Ceuppens et al., 2015). However, floral scent plumes and the distances at which they can be detected by insect foragers are rapidly degraded by increasing levels of common oxidizing air pollutants (McFrederick et al., 2008; Girling et al., 2013; Farré-Armengol et al., 2015; Lusebrink et al., 2015; Fuentes et al., 2016; Leonard et al., 2018). In addition to degradative odor-modulation, additive odor-pollution is also problematic. Previous work has shown that the presence of agrochemical odors can negatively impact bumblebee foraging behavior (Sprayberry et al., 2013). As a logical next step, this study tests the effects of agrochemical odor pollution on floral-odor learning and recognition in bumblebees, using Bombus impatiens as our study species. Specifically, we are asking: (1) What is the effect of background fungicide-odor pollution on floral-odor learning? (2) What is the effect of background fungicide-odor pollution on the ability to recognize learned floral-odors? (3) What is the effect of fungicide-odor point pollution on floral-odor recognition? (4) How does fungicide odor impact antennal-responses to floral odor? and (5) What are the structural attributes of fungicide-odors associated with changes in learning behavior?
Materials and Methods
Animals
Bombus impatiens colonies consisting of 100–125 bees, supplied by Koppert Biological Systems, were maintained in a lab-environment with ad libitum access to pollen and 30% sucrose solution. Each colony is wrapped in a seedling heat mat (IPower Propogate) via thermostat to ensure a consistent temperature range of 75–85°F in the hive. These experiments used six colonies from November 2019 to July 2021.
Free Moving Proboscis Extension Reflex Protocol
Associative odor (AO)-learning was measured using a modified Free Moving Proboscis Extension Reflex (FMPER) (Muth et al., 2017; Sprayberry, 2020; Figure 1A). Healthy-, active-individual B. impatiens (from Koppert Biological Systems) were selected from lab colonies, placed in screen backed vials, acclimated for 2 h, and placed into the odor stimulation apparatus. The ventilating testing array drew air in through two small holes in the lid and out the back, with flow rates ranging from 0.1 to 0.3 m/s (VWR-21800-024 hot wire anemometer). During conditioning, bees were offered a single drop of 50% sucrose on a yellow strip inserted through one of the two lid holes (hole-selection was randomized). These strips were cut from plastic folders and had absorbent adhesive bandage tape (Cover Roll) placed on the back to hold AO stimuli. The plastic prevented the odor-solution from diffusing into the sugar solution on the top of the strip, therefore the primary sensory encounter with odorants was through the olfactory rather than the gustatory system. Bumblebees would undergo four association trials at 5-min intertrial intervals, where they were presented an AO paired with sucrose reward via the yellow plastic strip. Bumblebees that successfully completed four association trials would then undergo a test trial after an additional 5 min wait, where they were presented with two unrewarding yellow plastic strips, one containing the AO and one containing unscented mineral oil (MO) (Figure 1B). Proboscis extension on the AO strip is considered a “correct” choice, on the control strip is considered an “incorrect” choice, and individuals that approached the strips three times without exhibiting PER or didn’t react to odor presentation within 30–45 s are classified as “no-choice.” All tested bees were tagged after experiments to prevent re-testing, ensuring statistical independence of data points. If all bees tested on a given day for a given stimulus (i.e., an experimental session) responded “no choice,” that session’s data was excluded from the final dataset to protect against potential experimenter error disrupting the dataset. This was a rare event: across the 623 bees tested from six colonies, 37 data points (6%) fell into this exclusion category. An additional 48 data points (8%) were excluded due to experimenter error or drastically atypical bumblebee behavior (Supplementary Dataset 1).
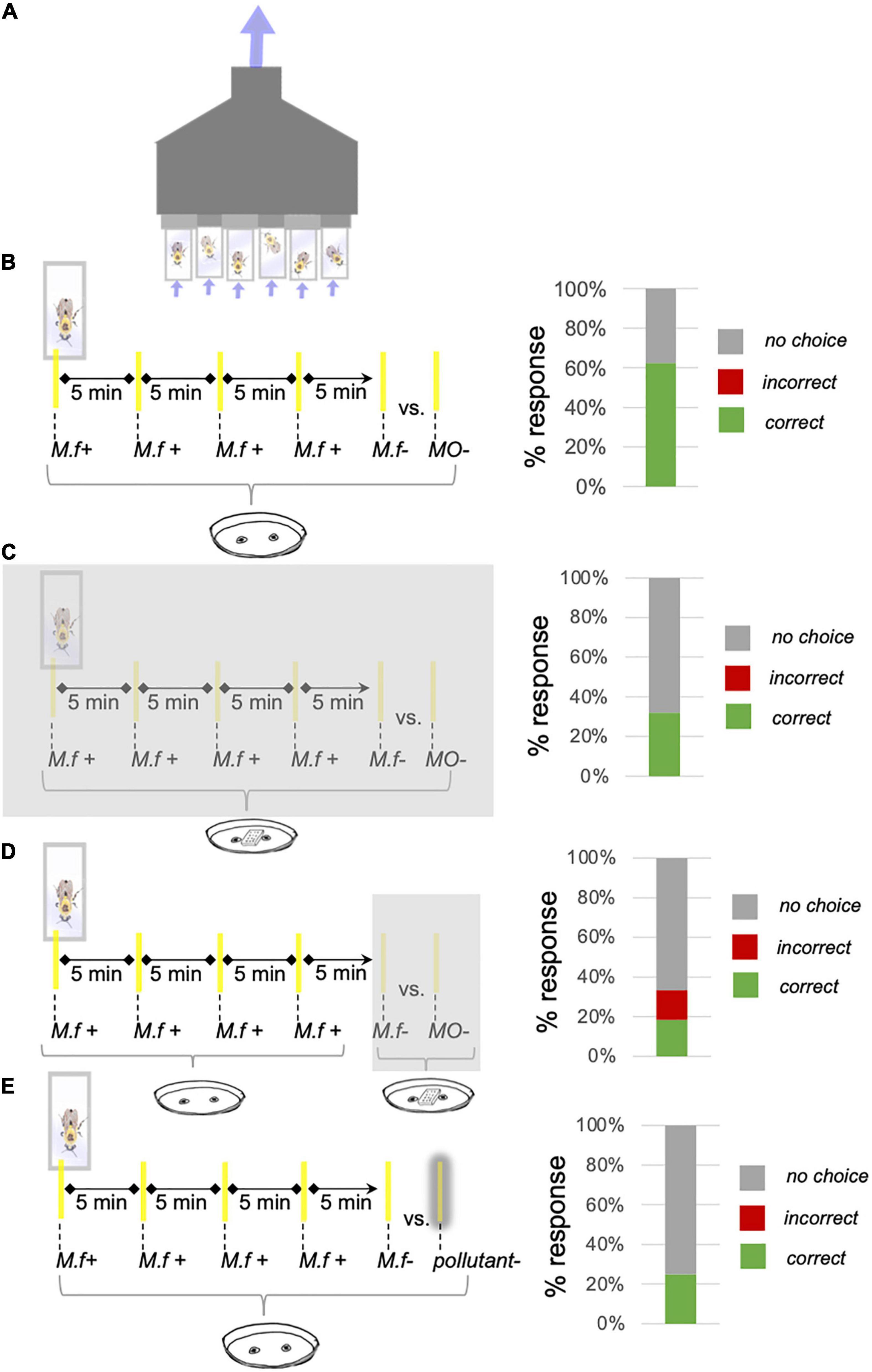
Figure 1. Free Moving Proboscis Extension Reflex (FMPER) tests bumblebee associative odor learning and recognition in different pollution paradigms. (A) The FMPER apparatus. This rig holds six bees in individual tubes with screen backs and lids with two small holes. Tubes are attached to a venting fan, which creates airflow through the tubes and prevents odor buildup. Diagram adapted from Sprayberry (2020). (B) The control FMPER experiment for associative odor learning. Bees were presented with a sucrose-rewarding strip scented with the M. fistulosa extract (M.f+) four times at 5-min interstimulus intervals. Recognition of M.f extract was tested by simultaneously presenting the bees with two unrewarding strips: one scented with M.f extract (M.f–) and one with unscented mineral oil (MO–). Proboscis extension onto the M.f– strip is scored as a correct response and onto the MO– strip was scored as incorrect. Bees that completed the association trials but did not pick between the M.f– and MO– were scored as “no-choice.” The distribution of correct/incorrect/and no-choice responses for these experiments are plotted next to the protocol schematic. Background odor-pollution introduced during association tested the effects of pollution on learning (C) and recognition (D) of M.f extract. To create background odor-pollution we added a 3-D printed box with perforated top, containing filter paper with the pollutant of interest, to the inside of vial lids; permeating the vial with pollutant odor. As indicated by the placement of the translucent gray box, the pollutant was either present throughout training and testing (C) or just testing (D) for these experiments. Results for a single representative experiment are plotted for both pollution during learning (0.1 μL of Safer® fungicide, n = 25) and recognition (1 μL of Scotts® fungicide, n = 27) are plotted to the right of their respective schematics. (E) Replacement of the unscented mineral oil strip (MO–) during testing with a pollutant-scented strip, as indicated by the gray shadow around the pollutant-strip, tested the effect of point-pollution odor on bumblebees’ ability/willingness to recognize M.f extract. Responses for a representative point-pollution experiment (1 μL of Safer® fungicide, n = 20) is plotted to the right of this schematic. Note the decrease in % correct responses and the increase in % no choice in all pollution conditions.
Control trials, which tested the ability of bumblebees to learn Monarda fistulosa extract (see details in “Odor Selection and Preparation”), used unscented MO as the contrasting odor in test trials with no pollution present (Figure 1B). To mimic the effects of fungicide being applied to an entire plant, creating a background of polluting odor, we added a 3-D printed box with perforated top to the inside of vial lids. The box held a 14 × 6 mm piece of filter (Fisher Brand P4) with the pollutant of interest, permeating the vial with pollutant odor. Spraying fungicide to an entire garden or agricultural field should introduce fungicide odor as a background condition. Fungicide application could occur before bloom, in which case floral-odor learning is occurring within this background pollution. To test effects of background odor-pollution on odor-learning, the box would be placed before association trials began and stay throughout the experiment (Figure 1C). Fungicides can also be applied for the first time after bloom, in which case odor-recognition is occurring against the background. To test the effects of background odor-pollution on odor-recognition, the box would be placed at the start of the 5-min wait before testing (Figure 1D). Given that localized fungicide applications (i.e., foliar spray on diseased leaves) may be encountered as point pollution, rather than as an odor-background, we also ran FMPER tests using fungicides as the contrasting odor in test trials (Figure 1E).
Odor Selection and Preparation
One microliter of mechanically extracted M. fistulosa (wild-bergamot/bee-balm) odor was used as the AO throughout all FMPER experiments (hereon referred to as “M.f extract”). The mechanical extract was made by adding 100 cm3 of floral material to a mortar, coating them generously with approximately 5–10 mL of unscented MO (Sigma Aldrich), and macerating the flowers with a pestle. The resultant mixture was filtered through a stainless steel tea strainer to isolate the oil-based liquid extract. This method of odor-stimulus preparation provides an ecologically relevant odor stimulus that has been found to stimulate indistinguishable behavioral responses from natural plant material (Edwards and Sprayberry, in preparation). The same batch of M.f extract, kept in cold storage, was used throughout experiments for consistency. The contrasting odors for these experiments varied across trial types as outlined below, but all trials needing an unscented stimulus used 1 μL of odorless mineral-oil.
These experiments tested three different environmentally relevant fungicides across learning and discrimination paradigms: Safer® Brand Garden Fungicide II (active ingredient: sulfur, 0.4%), Scotts® Lawn Fungus Control (active ingredient: thiophanate-methyl, 2.3%), and Reliant® Systemic Fungicide (Agri-Fos/Garden-Phos) (active ingredient: mono and dipotassium salts, 45.8%). All three fungicides were selected because their active ingredients overlap with those used at local farms. Safer® fungicide is applied topically to the plant, and both Reliant® and Scotts® are systemic action formulations which are absorbed by plant roots into nectar and pollen.
Two of the fungicides, Safer® Brand Garden Fungicide II, and Reliant® Systemic Fungicide (Agri-Fos/Garden-Phos), are water-soluble and came in liquid formulations. Safer® was used undiluted, and Reliant® was prepared at a 1:100 dilution to match the concentration of active ingredient found in the Safer® fungicide (0.4%). The Scotts® Lawn Fungus Control came in solid (granule) formulation and requires water to activate (“Lawn Fungus Control,” “Safer® Brand Garden Fungicide II”), as per the product label. To prepare a liquid solution, granules were mixed with deionized water in a 1:6 volumetric ratio of fungicide:deionized water and allowed to sit overnight. Fungicide solutions were disposed of after 72 h.
Odor Sampling
All experimental odors (M.f extract, Safer®, Reliant®, and Scotts®) were sampled using Solid-Phase Microextraction (SPME) fibers (Supelco 57359-U with PDMS/DVB coating) and analyzed with gas chromatography–mass spectrometry (GC-MS). Odors were placed in a 6 mL glass sampling jar fitted with a septum cap. The headspace within the jar was allowed to equilibrate for 30–45 min, then the SPME fiber was injected and allowed to adsorb volatiles for 30 min. Fibers were processed on a Shimadzu GC-QP5050A. Samples were injected into a splitless-programmed temperature-injector to a Zebron ZB-5MS (5% phenyl-arylene, 95% dimethylpolysiloxane) of 30 m × 0.2 mm i.d. × 0.25 μm film thickness analytical column from Phenomenex. The GC temperature was programmed to 5 min at 50°C, followed by a 10 C/min ramp to 320, then held for 15 min at 320°C (total time 47 min). All peaks on the GC spectra higher than 10× noise (Harris, 2016) were tentatively identified via their mass-spectra (MS) with the connected chemical library. Known contaminants and peaks with <80% similarity to the top library match were removed from analysis. Peaks with high similarity (>92%) that could be verified with MS were assigned the top match. For peaks with lower similarity (80–92%) or with unclear MS differentiation, the top 4–6 matches were examined for structural similarity. If multiple potential matches shared structural characteristics, indicating which characteristics were represented in the compound’s MS-spectra, a representative structure was assigned. All peak assignments were verified with retention indices where possible. Peak verification status (MS verified, RI verified, or representative) can be found in Supplementary Table 1. RI indices for all RI-verified peak-assignments are provided in Supplementary Table 2. Peaks that did not meet any of the assignment criteria listed above were labeled as unidentified. This process was repeated for three runs for each odor we were able to quantify.
Statistical Analysis
The first question our statistical analysis asks of the FMPER data is: are B. impatiens capable of learning M.f extract? For this we analyzed the control data set (AO = M.f extract, CO = mineral oil, no pollution) as described in Sprayberry (2020). First, the distribution of “correct” (C), “incorrect” (I), and “no choice” (NC) for FMPER experiments with no background contamination and an unscented CO were compared to a random distribution (1:1:1) with an exact goodness of fit test using a log likelihood ratio method for calculating p-values. Rejection of the null-hypothesis in this test would indicate a non-random distribution of responses. Next, we tested the control data against an expected response distribution for successful odor learning and recognition in this FMPER paradigm. This distribution (C = 61.6%, I = 8.5%, NC = 29.9%) is based on FMPER data from 177 bees using 7 different AO stimuli – representing normal associative-odor learning behavior in this paradigm; full details are available in Sprayberry (2020). Large p-values, or acceptance of the null hypothesis, in this test would indicate that B. impatiens’ ability to learn and recognize M.f extract is similar to their ability to learn other odors in an unpolluted FMPER paradigm. Following the recommendations of Amrhein et al. (2019), we are reporting exact p-values and not classifying data into binary categories of “significant” versus “insignificant.” However, readers wanting to assess traditional significance of p-values for this particular data set will want to use the Bonferroni-corrected alpha value of 0.025 for the control data, and an alpha value of 0.05 for the remaining data sets.
Datasets from pollution-FMPER experiments were analyzed against the expected response distribution using the exact goodness of fit test to determine if learning of M.f extract is impaired by background fungicide odor. Rejection of the null hypothesis would indicate disruption of learning. Datasets with p-values less than 0.15 were subjected to a binomial post hoc comparison to theoretical distribution for the “correct,” “incorrect,” and “no choice” data.
Odor Vectorization and Angular Distance Calculations
The sensory attributes of sampled odors were characterized using the Compounds Without Borders (CWB) vectorization method (Sprayberry, 2020). This is a novel neuroethological method of odor characterization that calculates the amount of sensory energy (power) present in a complex odor. The dimensional signature of each odor-blend was calculated by describing the molecular structure of each component odorant (see “Odor Sampling” above) based upon their respective carbon chain length (CCL), cyclic carbon count (CCC), and functional group (FG) characteristics (Figure 2 and Supplementary Movie 1). From a dimensional perspective, the power for each dimension was calculated as the summed normalized area under the curve of all peaks from molecules with that attribute (CCL, CCC, or FG). If no molecules within a given odor blend have that attribute, that dimension has a power of zero. From a peak/molecule perspective, each odorant’s normalized area will be assigned to multiple dimensions; with a minimum of three (CCC, CCL, and at least one FG) and no maximum. This dimensional representation aligns with known odorant-attributes that are coded for in insect olfactory systems (Lei and Vickers, 2008; Sandoz, 2011; Mertes et al., 2021). While the selection of dimensions was motivated by neurophysiological studies, these vectors do not represent a physiologically filtered sensory space of bumblebees, rather they are a representation of the sensory energy available to a bee; much as a spectrogram of a flower is not the same as where that floral color resides in bumblebee color space (Chittka, 1992). In addition to allowing comparison of specific sensory composition, these CWB vectors allow the difference between odors to be calculated as the angular distance between their vectors with the following equation:
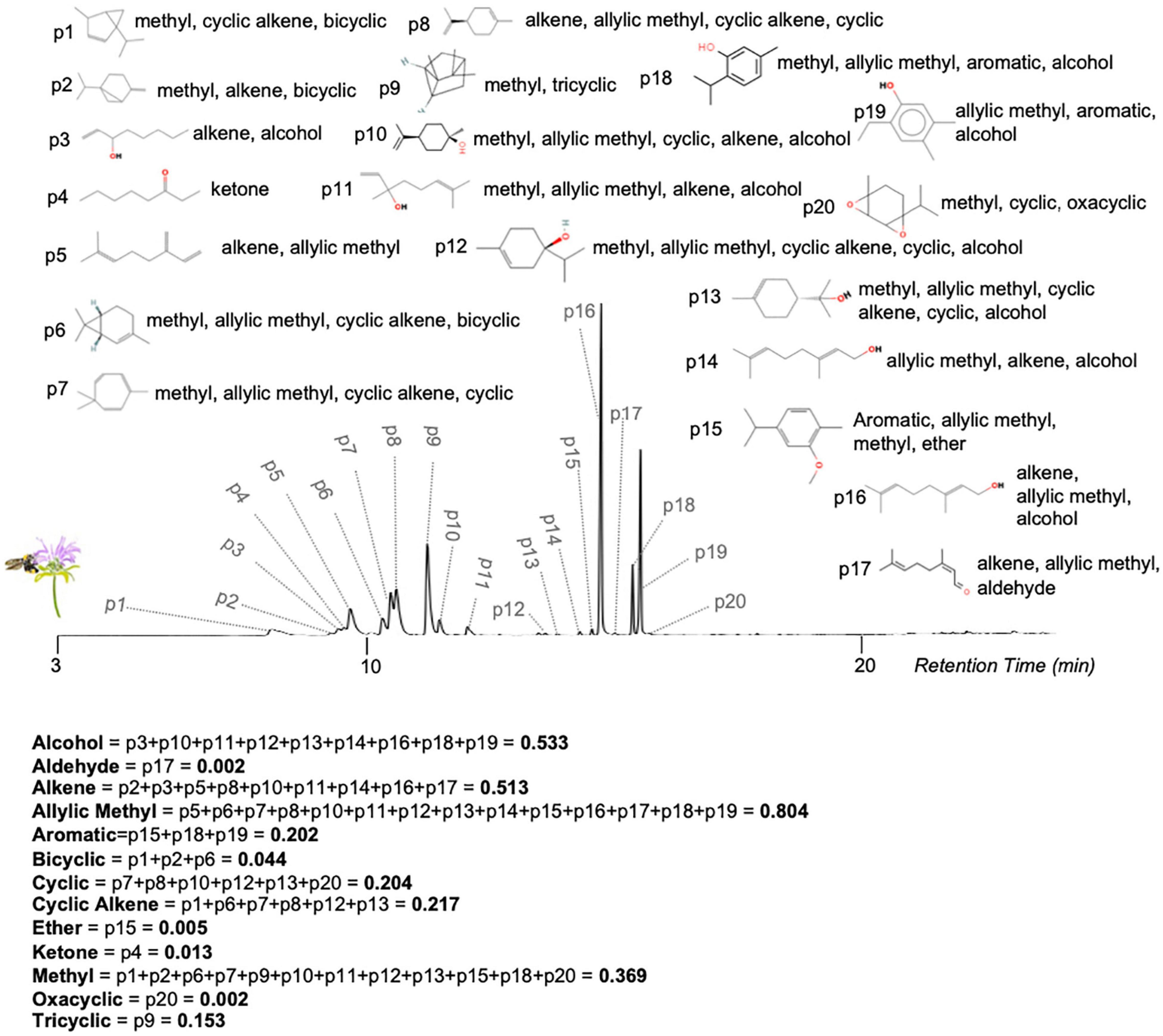
Figure 2. Gas chromatogram for Monarda fistulosa extract showing how Compounds Without Borders (CWB) vectors are calculated. CWB vectors provide a quantification of an odor-blend’s power in physiologically relevant dimensions of molecular structure. For a complete description see the methods section. The spectrum for M.f extract (relative intensity versus time) is plotted above. All peaks above noise are numbered, and the corresponding representative-molecule for the peak is diagrammed. The dimensional attributes based on functional groups of the representative structures for each peak are listed, as well as the total resulting power for that dimension. An animated version of this figure is available in Supplementary Movie 1. Peak assignments are: 1 = bicyclo[3.1.0]hex-2-ene, 4-methyl-1-(1-methylethyl); 2 = bicyclo[3.1.0]hexane, 4-methylene-1-(1-methylethyl); 3 = 1-octen-3-ol; 4 = 3-octanone; 5 = myrcene; 6 = 2-carene; 7 = 1,3,5-cycloheptatriene, 3,7,7-trimethyl-; 8 = limonene; 9 = tricyclo[2.2.1.0(2,6)]heptane, 1,7,7-trimethyl-; 10 = cyclohexanol, 1-methyl-4-(1-methylethenyl)-; 11 = linalool; 12 = 3-cyclohexen-1-ol, 4-methyl-1-(1-methylethyl); 13 = terpineol; 14 = geraniol; 15 = benzene, 2-methoxy-1-methyl-4-(1-methylethyl); 16 = geraniol; 17 = neral; 18 = thymol; 19 = phenol, 2-ethyl-4,5-dimethyl; 20 = (1S,2R,4R,7R)-4-isopropyl-7-methyl-3,8-dioxatricyclo[5.1.0.02,4]octane.
For full details on this method see Sprayberry (2020).
Electroantennogram Recordings
Electroantennograms (EAGs) were used to answer two questions: (1) Do B. impatiens transduce fungicide scent? and (2) Does fungicide odor-background modulate antennal-responses of B. impatiens to M.f extract?
Recording Preparation
A single antenna was removed from a cold anesthetized B. impatiens and the basal end placed into a borosilicate glass capillary tube (0.4 mm ID) filled with conductive gel (Parker Signal Gel). The proximal segment of the antenna was then clipped and placed in a second gel-filled capillary tube. These tubes were connected to the headstage for an AM-Systems 1200 extracellular amplifier (Seattle, WA, United States) via lead wires. Data were acquired with DataWave SciWorks V11 acquisition system.
Odor Stimuli
Air-flow for these experiments was provided by compressed breathing-quality air (80% nitrogen, 20% oxygen) delivered at 20 psi to controlling flow-meters (Dakota, model # PMRI-018083). A steady stream of humidified-air was positioned to flow over the antenna at a rate of 4373 mL/min. A second air stream (952 mL/min) fed into a solenoid with two outputs. These outputs connected to two syringes injected into the constant air stream. At zero voltage the solenoid directed air into the background syringe, while a 5V pulse caused the solenoid to direct air into the stimulus syringe for the duration the pulse, allowing computer-control over timing of stimulus delivery. Odor syringes were prepared by soaking 3cc plastic syringes in deionized water overnight, then letting them dry in the hood for 24 h. Syringes were labeled and only used for one type of odor stimulus. Prior to experiments, 2 μL of odorant were pipetted onto filter paper (Fisher Brand P4) placed in the syringe. The odor stimuli used in EAG experiments were the same as in FMPER: M.f extract, Safer®, Reliant®, and Scotts®.
Regardless of which fungicide was being tested, the temporal structure of experiments stayed the same. Each stimulus set delivered 3 × 0.5 s odor pulses with 5 s interpulse-intervals. The first stimulus-set used was a blank syringe, to assess the mechanosensitive component of EAG responses. The second stimulus set was M.f extract. The third-set delivered a fungicide odor. After measuring EAG response to fungicide the blank background stream was replaced with the fungicide syringe, resulting in a continuous stimulation of the antenna with fungicide odor. This was left in place for 1 min before recording the responses to a stimulus set of M.f extract over the fungicide background.
Analysis
Raw voltage responses were exported as text files from SciWorks and analyzed in Python. Individual EAG traces from all three trials for each stimulus were convolved with a Gaussian window to smooth out noise in the data, then all traces were averaged prior to amplitude calculations to create a single representative trace. The amplitude of the responses was calculated as the delta-V from the peak of the response to the hyperpolarization from slowly adapting units.
Results
Bombus impatiens Readily Learn Monarda fistulosa Extract Scent
Prior to testing impacts of fungicide pollution we confirmed that B. impatiens are capable of learning the ecologically relevant odor provided by M.f extract. The FMPER response distribution (62.5% correct, 37.5% no choice) was markedly different from random (p < 0.001) but did not differ from the expected response distribution (p = 0.14, Table 1), indicating that bumblebees readily learn this odor signal (Figure 1B).
Background Odor Pollution Disrupts Learning and Recognition of Monarda fistulosa Extract
Background fungicide-odor pollution introduced during association-trials tested impacts on B. impatiens’ ability to learn M.f extract, while pollution introduced during testing-trials investigated effects on recognition. In both paradigms, at least one tested headspace-concentration of each type of fungicide disrupted predicted behavior (Table 1 and Figures 1, 3), as indicated by low p-values (<0.05) when comparing measured response distributions against expected (exact test of goodness of fit). Disruption of odor learning and/or recognition typically manifested as a decrease in % correct and an increase in % no-choice responses (Figures 1C,D), as indicated by post hoc binomial comparisons (Table 1). Plotting these responses over volume reveals differences in the disruption patterns of the three tested fungicides. Scotts® showed decreased % correct and increased % no-choice at all treatment volumes in both learning and recognition paradigms (Table 1, Figure 3, and Supplementary Figure 1). Safer® and Reliant® both showed variability across treatment volumes, with the lowest impacts at the highest volumes (10 μL, Table 1, Figure 3, and Supplementary Figure 1). Safer® was the least impactful fungicide, only showing disruption of learning and/or recognition at the lowest tested volume (0.1 μL, Table 1, Figure 3, and Supplementary Figure 1). Reliant® effects were less consistent, with the intermediary test volume (1 μL) being the most disruptive in the recognition paradigm (Table 1, Figure 3, and Supplementary Figure 1).
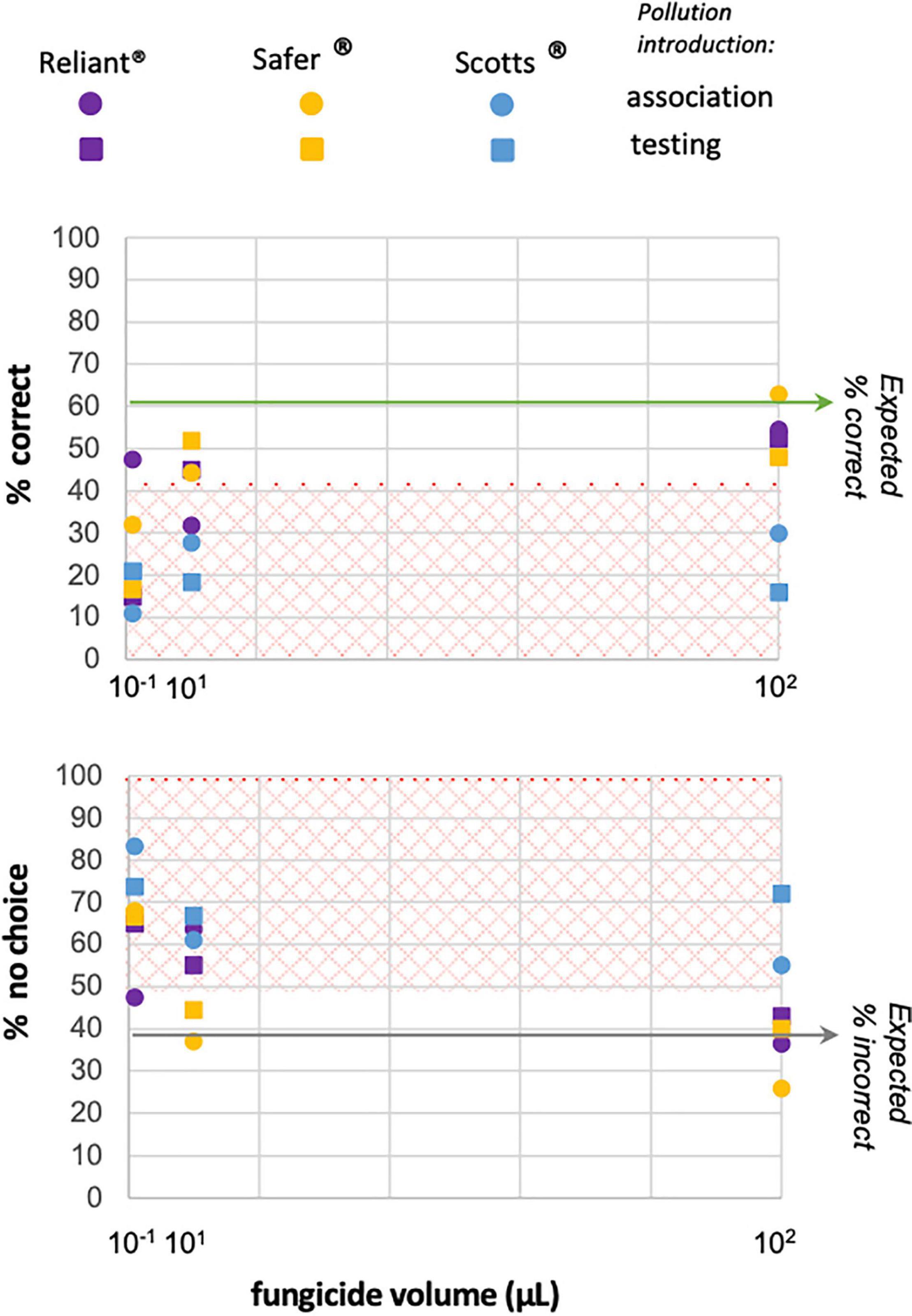
Figure 3. Background odor-pollution treatments are disruptive across all types of fungicides tested. Disruption of normal associative odor learning responses in this FMPER paradigm principally manifests as decreases in % correct response and increases in % no-choice response, as evidenced by the post hoc binomial comparisons in Table 1. To look at impacts of fungicides across treatment volumes and pollution paradigms, the percent correct (top graph) and the percent no-choice (bottom graph) responses are plotted above. Background pollution introduced during the association phase is plotted with circles, while pollution introduced during the testing phase is represented with squares. Fungicide treatments are demarcated by color: purple is Reliant®, yellow is Safer®, and blue is Scotts®. The green horizontal-line on the top panel indicates the % correct in control trials, while the gray line on the bottom panel indicates the % no-choice in control trials. For both plots the boxes with orange-diamond hashing encompass points that statistically differ from expected in post hoc comparisons (p ≤ 0.03, a = 0.05, Table 1). The sample size for all plotted-treatments is greater than or equal to 18, with exact values listed in Table 1.
Point Pollution Disrupts Monarda fistulosa Extract Recognition
Again, all three tested fungicides disrupted recognition of M.f extract at at-least one treatment volume. In contrast with the background pollution trials, the lowest volume of fungicide did not disrupt odor recognition for Safer® (Table 1 and Figure 4).
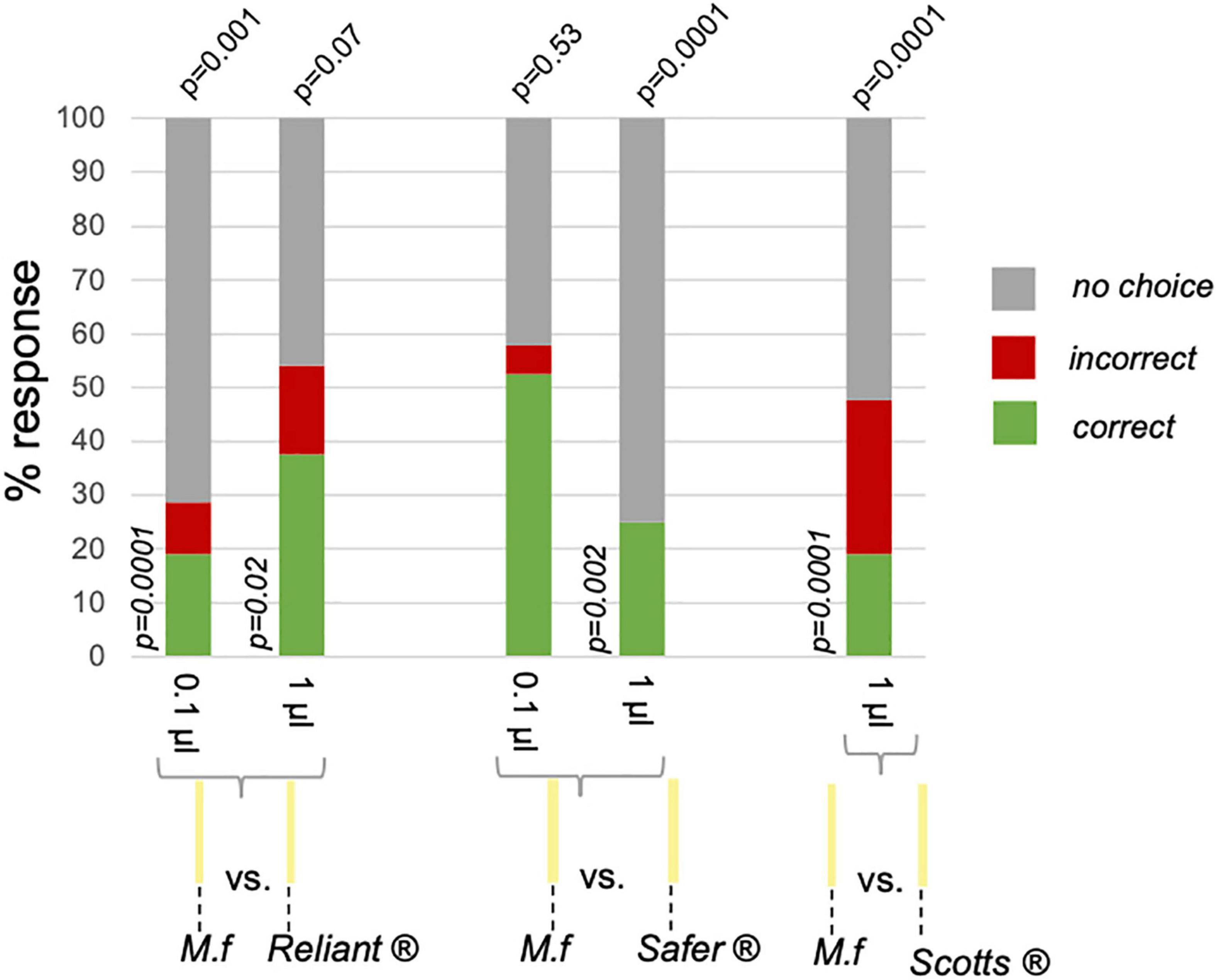
Figure 4. Fungicide odors are consistently disruptive in the point pollution paradigm. Results of FMPER experiments are represented in stacked bar plots, with the bottom section showing percent correct (in green), the middle section showing incorrect (in red), and the top section showing no-choice (in gray). Responses are clustered according to fungicide treatment, with volume of treatment indicated below each bar. The p-values for the exact goodness of fit test against expected response distribution is indicated on the top of each bar, while the p-value from post hoc comparisons with expected % correct is noted next to the bar when relevant (post hoc comparisons were run when p < 0.15 for exact goodness of fit tests). The sample sizes are: reliant 0.1 μL n = 21, 1 μL n = 24; Safer® 0.1 μL n = 19, 1 μL n = 20; Scotts® 1 μL n = 21.
All Tested Fungicides Elicit Electroantennogram Responses
While all three fungicides elicited measurable EAG responses, both Reliant® and Scotts® had significantly lower amplitude responses than M.f extract (p = 0.0001 and p < 0.0001, respectively, Figures 5A,B). Given the inability to measure headspace concentrations of delivered stimuli it is important to note that these differences could be driven by differences in stimulus intensity. These experiments consider responses to equal volumes of odor stimulus; therefore, they are most relevant in relationship to FMPER data for 1 μL fungicide-treatments, when equal volumes of M.f extract and fungicide were employed. Interestingly, when testing for potential inhibitory effects of fungicide background-odor on antennal responses to M.f extract both Reliant® and Scotts® reduced the amplitude of M.f extract responses (p = 0.03 and p = 0.005, respectively, Figure 5C). Safer® odor elicited similar magnitude responses as M.f extract when presented as a discrete stimulus and did not inhibit M.f extract responses when present as background (Figure 5).
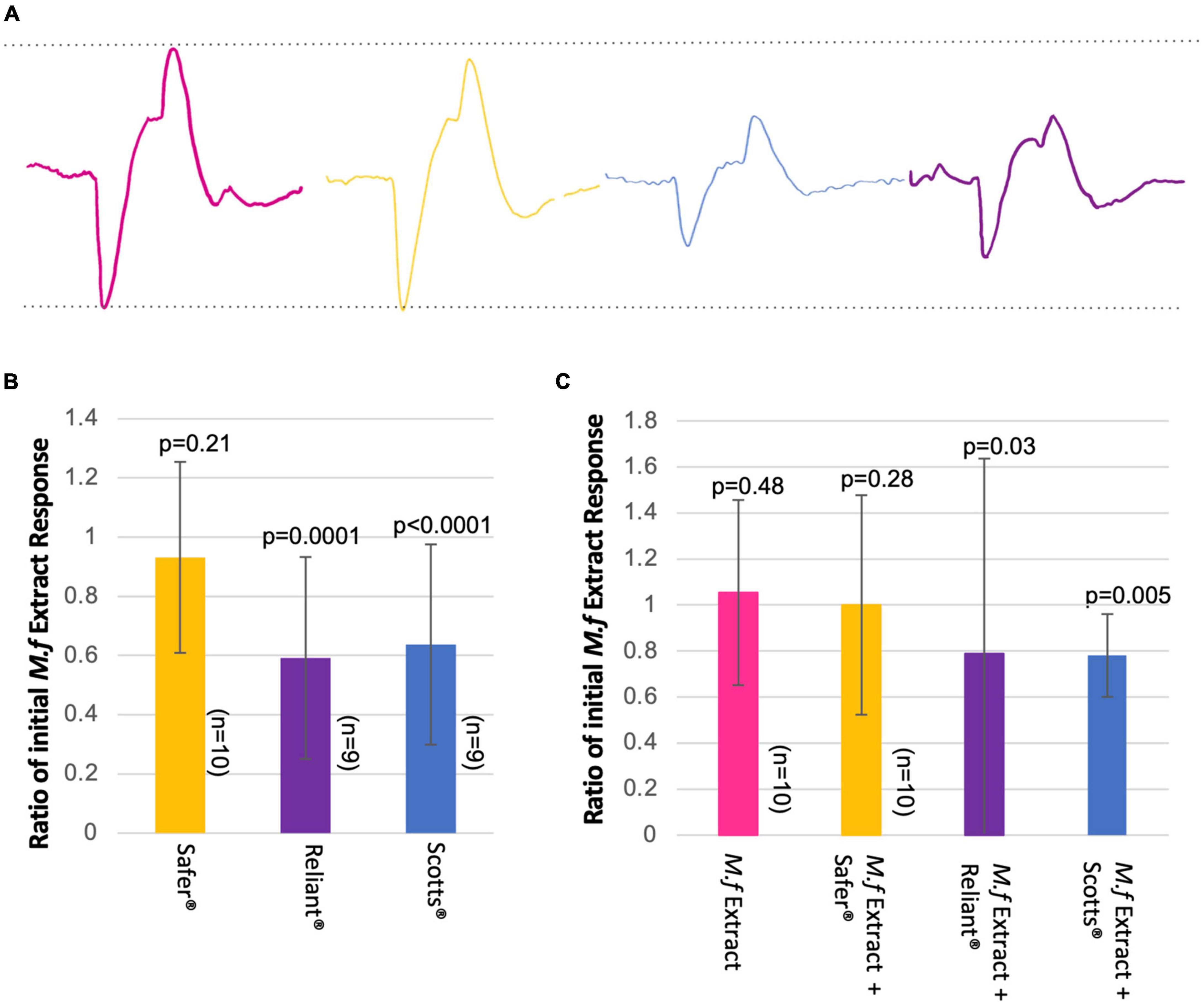
Figure 5. Electroantennogram recordings indicate the potential for fungicide-induced inhibition of responses to M.f extract. (A) Representative mean-EAG traces (smoothed- and averaged- over three trials) for each odor tested. M.f extract is shown in pink, Reliant® in purple, Safer® in yellow, and Scotts® in blue. (B) Average ratio of fungicide-odor antennal response relative to the initial M.f extract response. The p-values for EAG responses to fungicide odor relative to M.f extract (paired t-test) are printed on each bar. Sample sizes for each treatment are listed alongside each bar. Fungicide treatments are labeled and demarcated by color. Error bars are ±SD. (C) After stimulation with M.f extract, antenna received 1 min of continuous background stimulation with fungicide odor. At the end of this minute, the response to a second M.f extract stimulus was recorded. For control conditions antennae experienced 60 s of unscented air (no pollution). Responses to M.f extract stimulation are plotted as a ratio of the response to the first stimulus – this is why all initial data points are a value of 1. Individual trials of fungicide treatments are plotted as points, while the solid lines represent the mean response. Fungicide treatments are demarcated by color, with pink representing no pollution, yellow for Safer®, purple for Reliant®, and blue for Scotts®. The p-values for EAG responses to M.f extract before and after fungicide exposure (paired t-test) are printed next to each data label, along with the relevant sample size.
Fungicide Odor Vectors Differ From Monarda fistulosa Extract
Odors were characterized based on the CCL, CCC, and FG attributes of their constituent odorants (Sprayberry, 2020). A quantitative CWB-vector (Figure 2) could be calculated for M.f extract, Safer®, and Reliant® (Figure 6 and Supplementary Tables 1, 3). Unfortunately, excessive exclusion of peaks because of low percent similarity to top match for the Scotts® samples meant that we could not produce a quantitative vector for this fungicide odor (Supplementary Table 1). However, the majority of identified representative structures are reasonable breakdown products of thiophanate-methyl, the active ingredient in Scotts®. Tentatively analyzed runs of Scotts® demonstrated the presence of many sulfur containing compounds; a finding markedly different from M.f extract (Figure 6 and Supplementary Table 3). Thus our scent-analysis was able to provide some qualitative information on this odor structure. Using the M.f extract as a reference-vector, Reliant® and Safer® fungicides each had an angular distance well above the 30° threshold for odor-discrimination that has been previously found for B. impatiens at 57.4° and 63.2°, respectively (Figure 6), and thus are unlikely to be mistaken for M.f extract (Sprayberry, 2020). When comparing M.f extract to fungicide odors, we see that it has higher power in the alcohol, alkene, methyl, and allylic methyl functional-group dimensions, as well energy in larger cyclic structure and shorter CCL dimensions (typically 2 and 3 carbon chains often found branching off of cyclic structures). Safer® scent has more structural overlap with M.f extract than the other two fungicides, specifically in the alcohol, CCL8 and CCL2 dimensions (Figure 6). Non-zero dimensions that are unique to either Reliant® or Scotts® include long carbon chains (>CCL10), oxime, and sulfite (Figure 6).
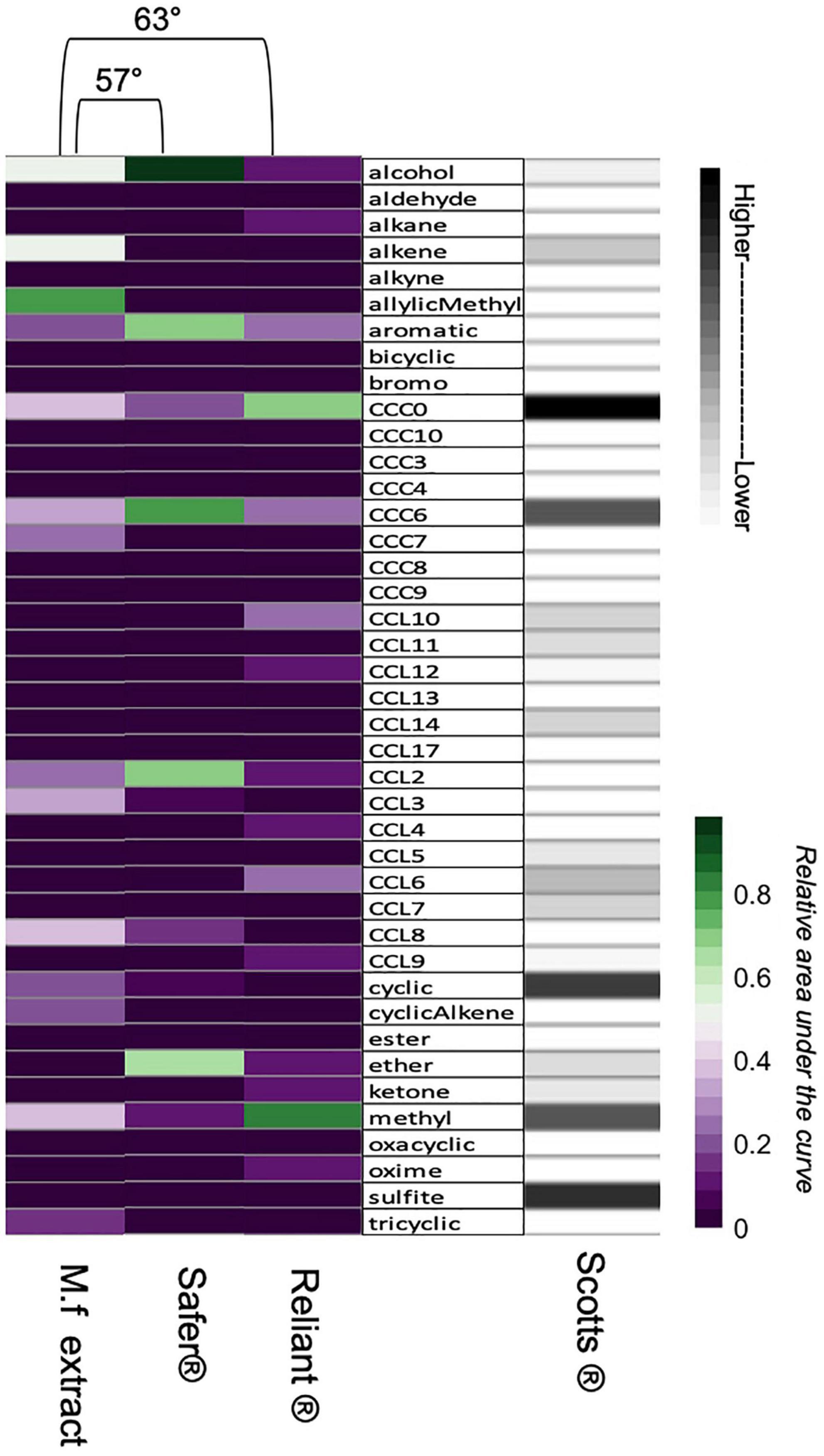
Figure 6. Dimensional distribution of sampled odors show differences in scent structure. The measured power in all 41 non-zero CWB-dimensions across the odors used in these experiments are represented with heatmaps above. A colored heatmap with numerical key is used for M.f extract, Safer® and Reliant® as we were able to quantify those three odors via CWB-vectors. Power is measured as summed relative area under the curve from relevant peaks and is therefore unitless. A corresponding grayscale heatmap is provided for the qualitative analysis of Scotts®, which was calculated from the peaks for which representative structures could reasonably be assigned (accounting for 68% of relative area under the curve). Given the qualitative nature of this analysis the heatmap has not been assigned quantitative values. Angular distances between M.f extract and the two quantifiable fungicide odors are indicated with the brackets connecting heatmap columns.
Discussion
Foraging Deficits From Fungicide Odor-Pollution May Be Due to Impairment in Odor Learning and Recognition
Previous work in this laboratory demonstrated that fungicide odor alone negatively impacted bumblebee foraging behavior in both maze and free-choice experiments (Sprayberry et al., 2013). However, the underlying-mechanisms could not be resolved with those experimental paradigms. This study explicitly tests the effects of fungicide odor-pollution on AO learning and recognition in bumblebees. Given that at least one concentration in every pollution-paradigm was disruptive (Figure 6), our results strongly suggest that fungicide odors decrease bumblebee learning and recognition of floral scent. While our results do not indicate that pollution modality (present during learning, recognition, or as a point source) affects the likelihood of fungicide-induced odor-behavior disruption, the FMPER assay used in this study is laboratory based. A field assay may elucidate more nuances in odor-driven bumblebee behaviors.
Effects of Fungicide-Odors Are Not Universal
While disruption of floral-odor learning and/or recognition was demonstrated for all tested fungicides in each pollution paradigm, the patterns of disruption across treatment volumes were not uniform. Scotts® fungicide odor appeared to be the most impactful, as it was disruptive in every test. However, Reliant® and Safer® data indicated that not all encounters with these fungicide odors are problematic. For both background-pollution paradigms tested, Safer® was disruptive to M.f extract learning and recognition only at the lowest volume tested. While this finding is initially counterintuitive, it is less surprising in light of the EAG and scent-structure data. EAG data show equally robust antennal-responses to both M.f extract and Safer. Additionally, Safer® was the only fungicide that did not inhibit antennal-responses to M.f extract which has two implications: (1) receptors activated by Safer® do not have excessive overlap with M.f extract – if they did we would expect them to exhibit adaptation and result in a lower amplitude response to M.f and (2) receptors activated by Safer® do not have clear lateral inhibition pathways to those triggered by M.f extract. Given that Safer® odor has a 57.4° angular distance to M.f extract, well above the previously determine 30° discrimination threshold (Sprayberry, 2020), it is logical that at adequate concentrations bumblebees are readily able to distinguish between these two scents. Lack of inhibition and discrimination can explain the responses seen at treatment volumes of 1 and 10 μL (Figure 3). Given that the stimulus intensity of 0.1 μL treatments will be lower, concurrent responses to Safer® odor at the antennae and antennal lobes will also be reduced. However, the intensity of M.f stimuli remains consistent throughout experiments, so the relative ratio of responses to M.f and Safer® odor in the antennal lobe will have shifted as well. If the response to Safer® is low enough to prevent clear detection, the physiological responses to the low concentration of Safer® odor could be configurally blended with those of M.f extract (Martin et al., 2011; Clifford and Riffell, 2013). In other words, at moderate to high concentrations, Safer® could be clearly distinguishable as a separate odor object, but at low concentrations – where detection is liminal – it might get wrapped into neurophysiological responses to M.f extract, resulting in a perceptual shift of M.f’s odor identity. Previous work on honeybees has demonstrated a similar phenomenon, where more similar floral scents required a higher stimulus concentration for honeybees to be able to discriminate between them (Wright and Smith, 2004). Likewise, Riffell et al. (2014) showed that background odor-pollution can negatively affect hawkmoths’ ability to track floral volatiles. Together, these two studies support the idea that odor pollution experienced at a liminal level can disrupt insects’ ability to engage with floral odors normally. While this liminal-disruption hypothesis could explain why the lowest concentrations of Safer® odor background pollution were the most problematic, it is speculative at this point and requires direct neurophysiological testing. Initially, the Reliant® data seem to push back against this hypothesis. However, EAG recordings clearly indicate that Reliant® odor is a less salient stimulus than both M.f extract and Safer® (Figure 5), which means that a higher concentration will be needed to reach the threshold of detection. This may explain why the 0.1 μL data for tests where pollution is present throughout (Figure 3) resemble the control data. Given the extensive period of time for odor to dissipate, the low volume, and the low salience, the actual headspace concentration of Reliant® may quickly drop below the threshold of detection; whereas the 1 μL treatment could operate above the threshold of detection, but still at a very low concentration. As with the Safer® data, these interpretations are hypothetical without direct neurophysiological testing. Regardless, the behavior data presented here indicate that fungicide odors are readily capable of disrupting floral odor learning and recognition.
Fungicide Odors Could Have Aversive Components
Point pollution experiments showed a lower than expected ratio of percent correct for at least one concentration in all three fungicides (Figure 6). Given the large structural differences (both angles were greater than 30°) it is unlikely that this decrease is due to an inability to discriminate between M.f extract and fungicide odor. Moreover, previous work on odor discrimination with this FMPER paradigm indicates that introduction of novel odors alone does not disrupt bumblebee’s willingness to engage with a learned odor (Sprayberry, 2020). Therefore, it is likely that fungicide odors either triggered an aversive response causing bumblebees to disengage, and/or that fungicide-induced inhibition reduced bumblebee ability to perceive the M.f extract as reliably. Given that Safer® point pollution was disruptive to M.f recognition and the fact that Safer® did not inhibit EAG-responses, aversion is the more parsimonious hypothesis. The characteristics unique to the three fungicide-odors used in this study include alkynes, long carbon chains (>10), nitrogenous (e.g., oxime) and sulfurous conformations. Ethers were present in M.f extract, but at much lower power than found in Safer® and Reliant® (Figure 6 and Supplementary Table 3). Manzate odor, a fungicide-scent previously demonstrated to adversely impact odor-driven foraging behavior in bumblebees, is entirely composed of carbon disulfide (Sprayberry et al., 2013). Sulfur compounds are not typical in bee-pollinated flowers, but are found in bat-and fly-pollinated flowers (Bestmann et al., 1997; Formisano et al., 2009; Shuttleworth and Johnson, 2010). Indeed, the presence of sulfur-compounds is associated with shifts away from hymenopteran pollination and toward fly pollination (Shuttleworth and Johnson, 2010). This could explain why fungicide odors are not innately attractive to B. impatiens, but does not necessarily explain aversion. However, when Butler et al. (1943) explored the potential for deliberately using sulfur-sprays to keep honey bees away from recently sprayed apple trees, they found they could reduce visitation for up to 7 days! Additionally, honeybees are also known to avoid nectar containing sulfurous fungicide (Kang and Jung, 2017). The possibility that nitrogenous and sulfurous odor dimensions reliably induce aversion in bumblebees and other hymenopteran pollinators is certainly worth further study.
Implications and Future Directions
Odor-search and navigation is likely critical for foraging bumblebees to locate resources given that bumblebees have been found to travel up to 1.75 km from their nest (Hellwig and Frankl, 2000) and visual information from flowers is only available at smaller spatial scales (Sprayberry, 2018). Bumblebees utilize individual foraging experiences and floral sensory information to locate resources (Lunau et al., 1996; Cnaani et al., 2006; Molet et al., 2008; Leonard et al., 2011; Lawson et al., 2018). Associative learning forms the basis for bumblebee ability to relocate specific types of food sources, and can even overcome innate biases (Internicola et al., 2009; Sommerlandt et al., 2014; Zhang et al., 2020). Given the importance of odor-learning to bumblebee foraging, the data presented here should stimulate concern about how fungicides are used in both agricultural and residential settings. As reviewed by Cullen et al. (2019), our understanding of how pesticides affect bees is substantially larger than that of fungicides’ impacts. Given that the only other study examining the effect of fungicide-odor pollution also showed deficits in foraging behavior (Sprayberry et al., 2013), continuing to explore the consequences of fungicide use is extremely relevant to bumblebee conservation.
However, the consistency and longevity of fungicidal odor-pollution in a field context is still unknown. As Lihoreau et al. (2019) poignantly argued, solely relying on laboratory based experiments separated from the natural environment does not allow for the full understanding of insect behavior and cognition. Thus future field studies are a critical component of determining the real-world effects of fungicide treatment on bumblebee health and pollination services. In addition, as mentioned above, a better understanding of which odor attributes are aversive and how they impact neural processing of floral odors will help conservation scientists better assess which agrochemical threats are the most pressing.
Data Availability Statement
The original contributions presented in the study are included in the article/Supplementary Material, further inquiries can be directed to the corresponding author.
Author Contributions
ND collected the FMPER and SPME/GCMS data. JS collected the EAG data and done the EAG analysis. ND, TH, and JS done the FMPER, GCMS, and CWB analysis. ND and JS shared writing of the manuscript, with editorial input from TH. All authors contributed to the article and approved the submitted version.
Funding
All funding for research supplies came from JS’s research allowance provided by Muhlenberg College. Summer salary for ND was provided by a Muhlenberg College Dean’s Grant.
Conflict of Interest
TH was employed by E. I. du Pont de Nemours, Inc.
The remaining authors declare that the research was conducted in the absence of any commercial or financial relationships that could be construed as a potential conflict of interest.
Publisher’s Note
All claims expressed in this article are solely those of the authors and do not necessarily represent those of their affiliated organizations, or those of the publisher, the editors and the reviewers. Any product that may be evaluated in this article, or claim that may be made by its manufacturer, is not guaranteed or endorsed by the publisher.
Acknowledgments
We would like to thank Koppert Biological Systems for providing research hives. Katharine Chen, Vanessa Pham, Morgan Tietz, and Nour Yousry aided in FMPER data collection. Nour Yousry aided in SPME sampling and chemical analysis.
Supplementary Material
The Supplementary Material for this article can be found online at: https://www.frontiersin.org/articles/10.3389/fevo.2022.765388/full#supplementary-material
Supplementary Figure 1 | Comparative data for repeated odor samples are represented in this figure.
Supplementary Table 1 | Odor analysis of all characterized odors are found in this table.
Supplementary Table 2 | Means and ranges for retention indices used to confirm odorant identity are found in this table.
Supplementary Table 3 | All CWB-vectors for characterized odors are found in this table.
Supplementary Dataset 1 | All FMPER data are downloadable from this supplement.
Supplementary Movie 1 | This movie provides a video walkthrough on the construction and uses of CWB-vectors for analyzing and comparing complex odors.
References
Amrhein, V., Greenland, S., and McShane, B. (2019). Scientists rise up against statistical significance. Nature 567, 305–307. doi: 10.1038/d41586-019-00857-9
Bernauer, O., Gaines-Day, H., and Steffan, S. (2015). Colonies of bumble bees (Bombus impatiens) produce fewer workers, less bee biomass, and have smaller mother queens following fungicide exposure. Insects 6, 478–488. doi: 10.3390/insects6020478
Bestmann, H. J., Winkler, L., and von Helversen, O. (1997). Headspace analysis of volatile flower scent constituents of bat-pollinated plants. Phytochemistry 46, 1169–1172. doi: 10.1016/s0031-9422(97)80004-0
Botías, C., David, A., Hill, E. M., and Goulson, D. (2017). Quantifying exposure of wild bumblebees to mixtures of agrochemicals in agricultural and urban landscapes. Environ. Pollut. 222, 73–82. doi: 10.1016/j.envpol.2017.01.001
Brittain, C. A., Vighi, M., Bommarco, R., Settele, J., and Potts, S. G. (2010). Impacts of a pesticide on pollinator species richness at different spatial scales. Basic Appl. Ecol. 11, 106–115. doi: 10.1016/j.baae.2009.11.007
Butler, B. C. G., Finney, D. J., and Schiele, P. (1943). Experiments on the poisoning of honeybees by insecticidal and fungicidal sprays used in orchards. Ann. Appl. Biol. 30, 143–150. doi: 10.1111/j.1744-7348.1943.tb06173.x
Cameron, S. A., Lozier, J. D., Strange, J. P., Koch, J. B., Cordes, N., Solter, L. F., et al. (2011). Patterns of widespread decline in North American bumble bees. Proc. Natl. Acad. Sci. U.S.A. 108, 662–667. doi: 10.1073/pnas.1014743108/-/dcsupplemental/pnas.201014743si.pdf
Carde, R. T., and Willis, M. A. (2008). Navigational strategies used by insects to find distant, wind-borne sources of odor. J. Chem. Ecol. 34, 854–866. doi: 10.1007/s10886-008-9484-5
Ceuppens, B., Ameye, M., Langenhove, H., Roldan-Ruiz, I., and Smagghe, G. (2015). Characterization of volatiles in strawberry varieties ‘Elsanta’ and ‘Sonata’ and their effect on bumblebee flower visiting. Arthropod Plant Interact. 9, 281–287. doi: 10.1007/s11829-015-9375-y
Chittka, L. (1992). The colour hexagon: a chromaticity diagram based on photoreceptor excitations as a generalized representation of colour opponency. J. Comp. Physiol. A 170, 533–543.
Christen, V., Krebs, J., and Fent, K. (2019). Fungicides chlorothanolin, azoxystrobin and folpet induce transcriptional alterations in genes encoding enzymes involved in oxidative phosphorylation and metabolism in honey bees (Apis mellifera) at sublethal concentrations. J. Hazard. Mater. 377, 215–226. doi: 10.1016/j.jhazmat.2019.05.056
Clifford, M. R., and Riffell, J. A. (2013). Mixture and odorant processing in the olfactory systems of insects: a comparative perspective. J. Comp. Physiol. A 199, 911–928. doi: 10.1007/s00359-013-0818-6
Cnaani, J., Thomson, J. D., and Papaj, D. R. (2006). Flower choice and learning in foraging bumblebees: effects of variation in nectar volume and concentration. Ethology 112, 278–285. doi: 10.1111/j.1439-0310.2006.01174.x
Corbet, S. A., Cuthill, I., Fallows, M., Harrison, T., and Hartley, G. (1981). Why do nectar-foraging bees and wasps work upwards on inflorescences? Oecologia 51, 79–83. doi: 10.1007/bf00344656
Cullen, M. G., Thompson, L. J., Carolan, J. C., Stout, J. C., and Stanley, D. A. (2019). Fungicides, herbicides and bees: a systematic review of existing research and methods. PLoS One 14:e0225743. doi: 10.1371/journal.pone.0225743
David, A., Botías, C., Abdul-Sada, A., Nicholls, E., Rotheray, E. L., Hill, E. M., et al. (2016). Widespread contamination of wildflower and bee-collected pollen with complex mixtures of neonicotinoids and fungicides commonly applied to crops. Environ. Int. 88, 169–178. doi: 10.1016/j.envint.2015.12.011
Eeraerts, M., Smagghe, G., and Meeus, I. (2020). Bumble bee abundance and richness improves honey bee pollination behaviour in sweet cherry. Basic Appl. Ecol. 43, 27–33. doi: 10.1016/j.baae.2019.11.004
Farré-Armengol, G., Peñuelas, J., Li, T., Yli-Pirilä, P., Filella, I., Llusia, J., et al. (2015). Ozone degrades floral scent and reduces pollinator attraction to flowers. New Phytol. 209, 152–160. doi: 10.1111/nph.13620
Formisano, C., Senatore, F., Porta, G. D., Scognamiglio, M., Bruno, M., Maggio, A., et al. (2009). Headspace volatile composition of the flowers of Caralluma europaea N.E.Br. (Apocynaceae). Molecules 14, 4597–4613. doi: 10.3390/molecules14114597
Fuentes, J. D., Chamecki, M., Roulston, T., Chen, B., and Pratt, K. R. (2016). Air pollutants degrade floral scents and increase insect foraging times. Atmos. Environ. 141, 361–374. doi: 10.1016/j.atmosenv.2016.07.002
Girling, R. D., Lusebrink, I., Farthing, E., Newman, T. A., and Poppy, G. M. (2013). Diesel exhaust rapidly degrades floral odours used by honeybees. Sci. Rep. 3:2779. doi: 10.1038/srep02779
Goulson, D., Nicholls, E., Botías, C., and Rotheray, E. L. (2015). Bee declines driven by combined stress from parasites, pesticides, and lack of flowers. Science 347:1255957. doi: 10.1126/science.1255957
Greenleaf, S. S., and Kremen, C. (2006). Wild bee species increase tomato production and respond differently to surrounding land use in Northern California. Biol. Conserv. 133, 81–87. doi: 10.1016/j.biocon.2006.05.025
Hegland, S. J., and Totland, Ø. (2008). Is the magnitude of pollen limitation in a plant community affected by pollinator visitation and plant species specialisation levels? Oikos 117, 883–891. doi: 10.1111/j.2008.0030-1299.16561.x
Hellwig, K. W., and Frankl, R. (2000). Foraging habitats and foraging distances of bumblebees, Bombus spp. (Hym., Apidae), in an agricultural landscape. J. Appl. Entomol. 124, 299–306. doi: 10.1046/j.1439-0418.2000.00484.x
Hosler, J. S., and Smith, B. H. (2000). Blocking and the detection of odor components in blends. J. Exp. Biol. 203, 2797–2806. doi: 10.1242/jeb.203.18.2797
Internicola, A. I., Page, P. A., Bernasconi, G., and Gigord, L. D. B. (2009). Carry-over effects of bumblebee associative learning in changing plant communities leads to increased costs of foraging. Arthropod Plant Interact. 3, 17–26. doi: 10.1007/s11829-008-9051-6
ISPBES (2016). The Assessment Report of the Intergovernmental Science-Policy Platform on Biodiversity and Ecosystem Services on Pollinators, Pollination and Food Production, eds S. G. Potts, V. L. Imperatriz-Fonseca, and H. T. Ngo (Bonn: Intergovernmental Science-Policy Platform on Biodiversity and Ecosystem Services).
Kang, M., and Jung, C. (2017). Avoidance behavior of honey bee, Apis mellifera from commonly used fungicides, acaricides and insecticides in apple orchards. J. Apic. 32, 295–302. doi: 10.17519/apiculture.2017.11.32.4.295
Klein, A.-M., Cunningham, S. A., Bos, M., and Steffan-Dewenter, I. (2008). Advances in pollination ecology from tropical plantation crops. Ecology 89, 935–943. doi: 10.1890/07-0088.1
Lawson, D. A., Chittka, L., Whitney, H. M., and Rands, S. A. (2018). Bumblebees distinguish floral scent patterns, and can transfer these to corresponding visual patterns. Proc. R. Soc. B Biol. Sci. 285:20180661. doi: 10.1098/rspb.2018.0661
Lei, H., and Vickers, N. (2008). Central processing of natural odor mixtures in insects. J. Chem. Ecol. 34, 915–927. doi: 10.1007/s10886-008-9487-2
Leonard, A. S., Dornhaus, A., and Papaj, D. R. (2011). Flowers help bees cope with uncertainty: signal detection and the function of floral complexity. J. Exp. Biol. 214, 113–121. doi: 10.1242/jeb.047407
Leonard, R. J., Vergoz, V., Proschogo, N., McArthur, C., and Hochuli, D. F. (2018). Petrol exhaust pollution impairs honey bee learning and memory. Oikos 128, 264–273. doi: 10.1111/oik.05405
Lihoreau, M., Dubois, T., Gomez-Moracho, T., Kraus, S., Monchanin, C., and Pasquaretta, C. (2019). Putting the ecology back into insect cognition research. Adv. Insect. Physiol. 57, 1–25. doi: 10.1016/bs.aiip.2019.08.002
Lunau, K., Wacht, S., and Chittka, L. (1996). Colour choices of naive bumble bees and their implications for colour perception. J. Comp. Physiol. 178, 477–489. doi: 10.1007/bf00190178
Lundin, O., Rundlöf, M., Smith, H. G., Fries, I., and Bommarco, R. (2015). Neonicotinoid insecticides and their impacts on bees: a systematic review of research approaches and identification of knowledge gaps. PLoS One 10:e0136928. doi: 10.1371/journal.pone.0136928
Lusebrink, I., Girling, R. D., Farthing, E., Newman, T. A., Jackson, C. W., and Poppy, G. M. (2015). The effects of diesel exhaust pollution on floral volatiles and the consequences for honey bee olfaction. J. Chem. Ecol. 41, 904–912. doi: 10.1007/s10886-015-0624-4
Mänd, M., Mänd, R., and Williams, I. H. (2002). Bumblebees in the agricultural landscape of Estonia. Agric. Ecosyst. Environ. 89, 69–76. doi: 10.1016/s0167-8809(01)00319-x
Martin, J. P., Beyerlein, A., Dacks, A. M., Reisenman, C. E., Riffell, J. A., Lei, H., et al. (2011). The neurobiology of insect olfaction: sensory processing in a comparative context. Prog. Neurobiol. 95, 427–447. doi: 10.1016/j.pneurobio.2011.09.007
Mathiasson, M. E., and Rehan, S. M. (2019). Status changes in the wild bees of north-eastern North America over 125 years revealed through museum specimens. Insect Conserv. Diver. 12, 278–288. doi: 10.1111/icad.12347
McFrederick, Q. S., Kathilankal, J. C., and Fuentes, J. D. (2008). Air pollution modifies floral scent trails. Atmos. Environ. 42, 2336–2348. doi: 10.1016/j.atmosenv.2007.12.033
Meftaul, I. M., Venkateswarlu, K., Dharmarajan, R., Annamalai, P., and Megharaj, M. (2019). Pesticides in the urban environment: a potential threat that knocks at the door. Sci. Total Environ. 711:134612. doi: 10.1016/j.scitotenv.2019.134612
Mertes, M., Carcaud, J., and Sandoz, J.-C. (2021). Olfactory coding in the antennal lobe of the bumble bee Bombus terrestris. Sci. Rep. 11:10947. doi: 10.1038/s41598-021-90400-6
Molet, M., Chittka, L., and Raine, N. E. (2008). How floral odours are learned inside the bumblebee (Bombus terrestris) nest. Naturwissenschaften 96, 213–219. doi: 10.1007/s00114-008-0465-x
Motten, A. F. (1986). Pollination ecology of the spring wildflower community of a temperate deciduous forest. Ecol. Monogr. 56, 21–42. doi: 10.2307/2937269
Muratet, A., and Fontaine, B. (2015). Contrasting impacts of pesticides on butterflies and bumblebees in private gardens in France. Biol. Conserv. 182, 148–154. doi: 10.1016/j.biocon.2014.11.045
Muth, F., Carvalheiro, L., Cooper, T. R., Bonilla, R. F., and Leonard, A. S. (2017). A novel protocol for studying bee cognition in the wild. Methods Ecol. Evol. 9, 78–87. doi: 10.1111/2041-210x.12852
Pettis, J. S., Lichtenberg, E. M., Andree, M., Stitzinger, J., Rose, R., and vanEngelsdorp, D. (2013). Crop pollination exposes honey bees to pesticides which alters their susceptibility to the gut pathogen Nosema ceranae. PLoS One 8:e70182. doi: 10.1371/journal.pone.0070182
Potts, S. G., Imperatriz-Fonseca, V., Ngo, H. T., Aizen, M. A., Biesmeijer, J. C., Breeze, T. D., et al. (2016). Safeguarding pollinators and their values to human well-being. Nature 540, 220–229. doi: 10.1038/nature20588
Riffell, J. A., Shlizerman, E., Sanders, E., Abrell, L., Medina, B., Hinterwirth, A. J., et al. (2014). Sensory biology. Flower discrimination by pollinators in a dynamic chemical environment. Science 344, 1515–1518. doi: 10.1126/science.1251041
Sandoz, J.-C. (2011). Behavioral and neurophysiological study of olfactory perception and learning in honeybees. Front. Syst. Neurosci. 5:98. doi: 10.3389/fnsys.2011.00098
Sgolastra, F., Arnan, X., Cabbri, R., Isani, G., Medrzycki, P., Teper, D., et al. (2018). Combined exposure to sublethal concentrations of an insecticide and a fungicide affect feeding, ovary development and longevity in a solitary bee. Proc. R. Soc. B Biol. Sci. 285:20180887. doi: 10.1098/rspb.2018.0887
Sgolastra, F., Medrzycki, P., Bortolotti, L., Renzi, M. T., Tosi, S., Bogo, G., et al. (2017). Synergistic mortality between a neonicotinoid insecticide and an ergosterol-biosynthesis-inhibiting fungicide in three bee species. Pest Manag. Sci. 73, 1236–1243. doi: 10.1002/ps.4449
Shuttleworth, A., and Johnson, S. D. (2010). The missing stink: sulphur compounds can mediate a shift between fly and wasp pollination systems. Proc. R. Soc. B Biol. Sci. 277, 2811–2819. doi: 10.1098/rspb.2010.0491
Sommerlandt, F. M. J., Rössler, W., and Spaethe, J. (2014). Elemental and non-elemental olfactory learning using PER conditioning in the bumblebee, Bombus terrestris. Apidologie 45, 106–115. doi: 10.1007/s13592-013-0227-4
Spaethe, J., Brockmann, A., Halbig, C., and Tautz, J. (2007). Size determines antennal sensitivity and behavioral threshold to odors in bumblebee workers. Naturwissenschaften 94, 733–739. doi: 10.1007/s00114-007-0251-1
Sprayberry, J. D. H. (2018). The prevalence of olfactory- versus visual-signal encounter by searching bumblebees. Sci. Rep. 8:14590. doi: 10.1038/s41598-018-32897-y
Sprayberry, J. D. H. (2020). Compounds without borders: a mechanism for quantifying complex odors and responses to scent-pollution in bumblebees. PLoS Comput. Biol. 16:e1007765. doi: 10.1371/journal.pcbi.1007765
Sprayberry, J. D. H., Ritter, K. A., and Riffell, J. A. (2013). The effect of olfactory exposure to non-insecticidal agrochemicals on bumblebee foraging behavior. PLoS One 8:e76273. doi: 10.1371/journal.pone.0076273.s001
Tomé, H. V. V., Ramos, G. S., Araújo, M. F., Santana, W. C., Santos, G. R., Guedes, R. N. C., et al. (2016). Agrochemical synergism imposes higher risk to Neotropical bees than to honeybees. R. Soc. Open Sci. 4:160866. doi: 10.1098/rsos.160866
van de Merwe, J. P., Neale, P. A., Melvin, S. D., and Leusch, F. D. L. (2018). In vitro bioassays reveal that additives are significant contributors to the toxicity of commercial household pesticides. Aquat. Toxicol. 199, 263–268. doi: 10.1016/j.aquatox.2018.03.033
van Heemert, C., de Ruijter, A., van den Eijnde, J., and van der Steen, J. (2015). Year-round production of bumble bee colonies for crop pollination. Bee World 71, 54–56. doi: 10.1080/0005772x.1990.11099036
Winfree, R., Reilly, J. R., Bartomeus, I., Cariveau, D. P., Williams, N. M., and Gibbs, J. (2018). Species turnover promotes the importance of bee diversity for crop pollination at regional scales. Science 359, 791–793. doi: 10.1126/science.aao2117
Wright, G. A., and Smith, B. H. (2004). Different thresholds for detection and discrimination of odors in the honey bee (Apis mellifera). Chem. Senses 29, 127–135. doi: 10.1093/chemse/bjh016
Keywords: fungicide, pollution, foraging, olfaction, bumble bee
Citation: David NF, Henry TJ and Sprayberry JDH (2022) Odor-Pollution From Fungicides Disrupts Learning and Recognition of a Common Floral Scent in Bumblebees (Bombus impatiens). Front. Ecol. Evol. 10:765388. doi: 10.3389/fevo.2022.765388
Received: 27 August 2021; Accepted: 25 March 2022;
Published: 09 June 2022.
Edited by:
Robbie Girling, University of Reading, United KingdomCopyright © 2022 David, Henry and Sprayberry. This is an open-access article distributed under the terms of the Creative Commons Attribution License (CC BY). The use, distribution or reproduction in other forums is permitted, provided the original author(s) and the copyright owner(s) are credited and that the original publication in this journal is cited, in accordance with accepted academic practice. No use, distribution or reproduction is permitted which does not comply with these terms.
*Correspondence: Jordanna D. H. Sprayberry, am9yZGFubmFzcHJheWJlcnJ5QG11aGxlbmJlcmcuZWR1