- 1Department of Ecology and Evolutionary Biology, Tulane University, New Orleans, LA, United States
- 2Fundación Para la Conservación de los Andes Tropicales, Quito, Ecuador
Understanding the factors that shape the diversity and composition of biotic communities in natural and human-modified landscapes remains a key issue in ecology. Here, we evaluate how functional traits, species diversity and community composition of palm species vary in relation to biogeographic variables and forest age in northwest Ecuador. Functional traits capture essential aspects of species’ ecological tradeoffs and roles within an ecosystem, making them useful in determining the ecological consequences of environmental change, but they have not been used as commonly as more traditional metrics of species diversity and community composition. We inventoried palm communities in 965 10 × 10 m plots arrayed in linear transects placed in forests of varying age. Adult palms in forests of younger regeneration stages were characterized by species with greater maximum stem height, greater maximum stem diameter, and solitary stems. The shift in functional features could indicate that shade tolerant palms are more common in old-growth forest. The shift could also reflect the legacy of leaving canopy palms as remnants in areas that were cleared and then allowed to regrow. Moreover, younger forest age was associated with decreased abundance and altered species composition in both juvenile and adult palms, and decreased species richness in adults. These results highlight the importance of retaining intact, old-growth forest to preserve functional and species diversity and highlight the importance of considering multiple aspects of diversity in studies of vegetation communities.
Introduction
Tropical forests harbor most of the planet’s terrestrial biodiversity, however, anthropogenic influences put these systems under increasing pressure (Laurance et al., 2006; Bradshaw et al., 2009; Gardner et al., 2010). Expanding human settlements, agriculture, and infrastructure development induce persistently high rates of deforestation (Achard et al., 2002). Deforested areas are often allowed to regenerate after exploitation (Rudel et al., 2009), and, consequently, over half of the planet’s contemporary forest cover is regenerating (Food and Agriculture Organization of the United Nations [FAO], 2010). As the proportion of regenerating forests continues to increase (Wright, 2010), the ability of these regenerating (i.e., secondary) forests to retain diverse biota has emerged as an important research topic over the past several decades.
The relative ability of secondary forests to support biodiversity is controversial (Barlow et al., 2007; Gardner et al., 2007; Norden et al., 2009; Gibson et al., 2011; Melo et al., 2013; Rozendaal et al., 2019). Some studies suggest that succession will occur predictably, indicating secondary forest species will converge with old-growth forests and retain significant proportions of the original diversity in the process (Finegan, 1996; Letcher and Chazdon, 2009; Norden et al., 2009). Alternatively, secondary forests may have disrupted ecological processes, with negative implications for biodiversity retention and conservation (Tabarelli et al., 2010, 2012; Gibson et al., 2011). A myriad of factors may influence the biodiversity value of secondary forests, including landscape context (Tabarelli et al., 2010; Araia et al., 2019), successional pathways (Arroyo-Rodríguez et al., 2017), and stochasticity (Chazdon, 2008), with many studies emphasizing the resilience of secondary forests occurring under ideal conditions (e.g., proximity to old-growth forest, high levels of seed dispersal) (Letcher and Chazdon, 2009; Norden et al., 2009). Moreover, outcomes differ markedly based on study taxa; while secondary forests may provide appropriate habitat for many species, others are disadvantaged or excluded (Barlow et al., 2007; Tabarelli et al., 2012; Martin et al., 2013).
Another important consideration concerns how biodiversity is measured. Species richness is a fundamental metric of biodiversity (Su et al., 2004), but it may fail to capture important components of a habitat’s value for conservation. For example, in a recent meta-analysis of longitudinal studies, Blowes et al. (2019) concluded that while local species richness is not changing on average worldwide, local change in community composition is pervasive. The decoupling of species richness and community composition may be due to differential speeds of recovery: in tropical tree communities, species richness is thought to recover more quickly, over the span of decades, while community composition may require centuries to recover (Finegan, 1996; Liebsch et al., 2008; Martin et al., 2013; Rozendaal et al., 2019).
Functional trait analyses may also provide important perspectives that complement those provided by species richness and community composition. Functional traits are a species’ morphological, physiological, or phenological characteristics that influence growth, reproduction, or survival (Violle et al., 2007). These traits mediate species’ responses to environmental changes, revealing how different ecological strategies are constrained in community assembly (Paine et al., 2011; Boukili and Chazdon, 2017). Moreover, changes within the functional composition of forest communities along environmental gradients may point to mechanisms behind alterations in ecosystem functioning and services (Dıìaz and Cabido, 2001; Díaz et al., 2013). Studies that combine richness, community composition, and functional trait analysis are rare but have the potential to provide a more holistic understanding of how habitat characteristics impact biodiversity.
Palms (Arecaceae) are among the most abundant and diverse neotropical plant taxa (Goulding and Smith, 2007; ter Steege et al., 2013; Muscarella et al., 2020). As fundamental components of forest structure and function, palms influence plant recruitment (Wang and Augspurger, 2004), nutrient turnover (Villar et al., 2020), and the movement of dispersers and pollinators (Galetti et al., 2006; Muñoz et al., 2019; Sardeshpande and Shackleton, 2019). Furthermore, they are a keystone food resource for many frugivorous species (Galetti et al., 2006; Muñoz et al., 2019; Sardeshpande and Shackleton, 2019). Additionally, from an anthropogenic perspective, neotropical palms often provide crucial economic and cultural services (Bernal et al., 2011; Macía et al., 2011). Previous work has suggested palms are sensitive to anthropic disturbances and environmental gradients (Pintaud, 2006; Arroyo-Rodríguez et al., 2007; Baez and Balslev, 2007; Eiserhardt et al., 2011; Montúfar et al., 2011; Benchimol et al., 2017), highlighting the importance of understanding impacts of human activities on this key group.
As many palms are long-lived (Tomlinson, 2006), the contemporary mix of adults on the landscape may represent the recruitment, establishment, and persistence processes that occurred over the past several decades, whereas juveniles are more likely to reflect more recent assembly processes (Norden et al., 2009; Green et al., 2014). Differences between the two life stages can indicate demographic changes occurring in the community, pointing to trends in population growth and impending shifts in diversity and composition related to abiotic (e.g., light, moisture) or biotic (e.g., dispersal mutualisms) factors. Yet, how palm communities vary between relatively undisturbed vs. regenerating forests has not been widely studied (Montúfar et al., 2011). Those studies available have highlighted increasing species richness of juveniles (Capers et al., 2005), converging composition of canopy palm communities to those in old-growth forest during succession (Norden et al., 2009), and increased susceptibility of juveniles to distance to forest edge (Browne and Karubian, 2016).
Our goal in this study was to identify how patterns of Neotropical palm abundance, species richness, community composition, and functional trait composition vary in relation to forest age, in juvenile and adult life stages. We predicted that, after controlling for effects of elevation, abundance and richness will decrease in younger forests and community composition and functional trait composition will differ in relation to forest age. We also predicted that responses to forest age will be stronger in juvenile palms due to recent habitat loss and stronger responses to diversifying processes.
Materials and Methods
Study Region
We conducted this study in the Chocó Biogeographic zone, a relatively poorly studied, increasingly modified conservation hotspot known for exceptional diversity of palms and other species (Gentry, 1986; Dodson and Gentry, 1991; Myers et al., 2000). Data were collected in and around the Mache-Chindul Ecological Reserve (REMACH, 0°47′N, 79°78′W), Esmeraldas Province, Ecuador, from June to August 2019. The most common forest types in REMACH are humid evergreen and sub-humid evergreen, with canopy height of primary forest typically ranging from 30–40 m. Dominant tree families include Arecaceae, Rubiaceae, Lauraceae, Moraceae, and Myristicaceae (Clark et al., 2006). REMACH is a mountainous area ranging from sea level to over 700 m asl. Previous work here by Browne and Karubian (2016) showed that elevation was an important predictor of abundance and community composition of palms, across both adult and seedling life stages.
We sampled habitats within two privately owned reserves – Fundación para la Conservación de los Andes Tropicales (FCAT, 00°23′28″N, 79°41′05″W) and Bilsa Biological Station (BBS, 00°21′33″N, 79°42′02″W) – and in adjacent areas on privately owned lands (Figure 1). The study region receives approximately 2,500-3,500 mm of precipitation annually and the average temperature is between 23°C and 25.5°C (Clark et al., 2006). We sampled representative habitat types (forest, pasture, agricultural crops) covering nearly the full gradient of elevations (198-686 m.a.s.l., Supplementary Table 1) and range of regeneration (primary (undisturbed) forest and secondary (previously disturbed) forest) found in REMACH (Supplementary Figure 1). This area has been extensively deforested, reflecting the deforestation that has occurred in all of western Ecuador (Dodson and Gentry, 1991). Deforestation in our study area is relatively recent, beginning ca. 40 years ago (Browne and Karubian, 2016) and continuing into the present (Van der Hoek, 2017; Kleemann et al., 2022). The annual mean net change in forested area is dynamic, with the periods between 1990 to 2000 and 2000 to 2008 exhibiting the highest amount of forest lost (Tamayo et al., 2020). The landscape at the time of data collection was a dynamic agro-mosaic consisting of many isolated forest fragments, pasture, and agricultural crops. As several members of our team (JO, DC) have lived in the area for over 30 years, we had a priori knowledge of forest chronosequence. We were unable to obtain exact data on forest age from remotely sensed images or aerial photograph due to low image resolution and the persistence of cloud cover in the study area.
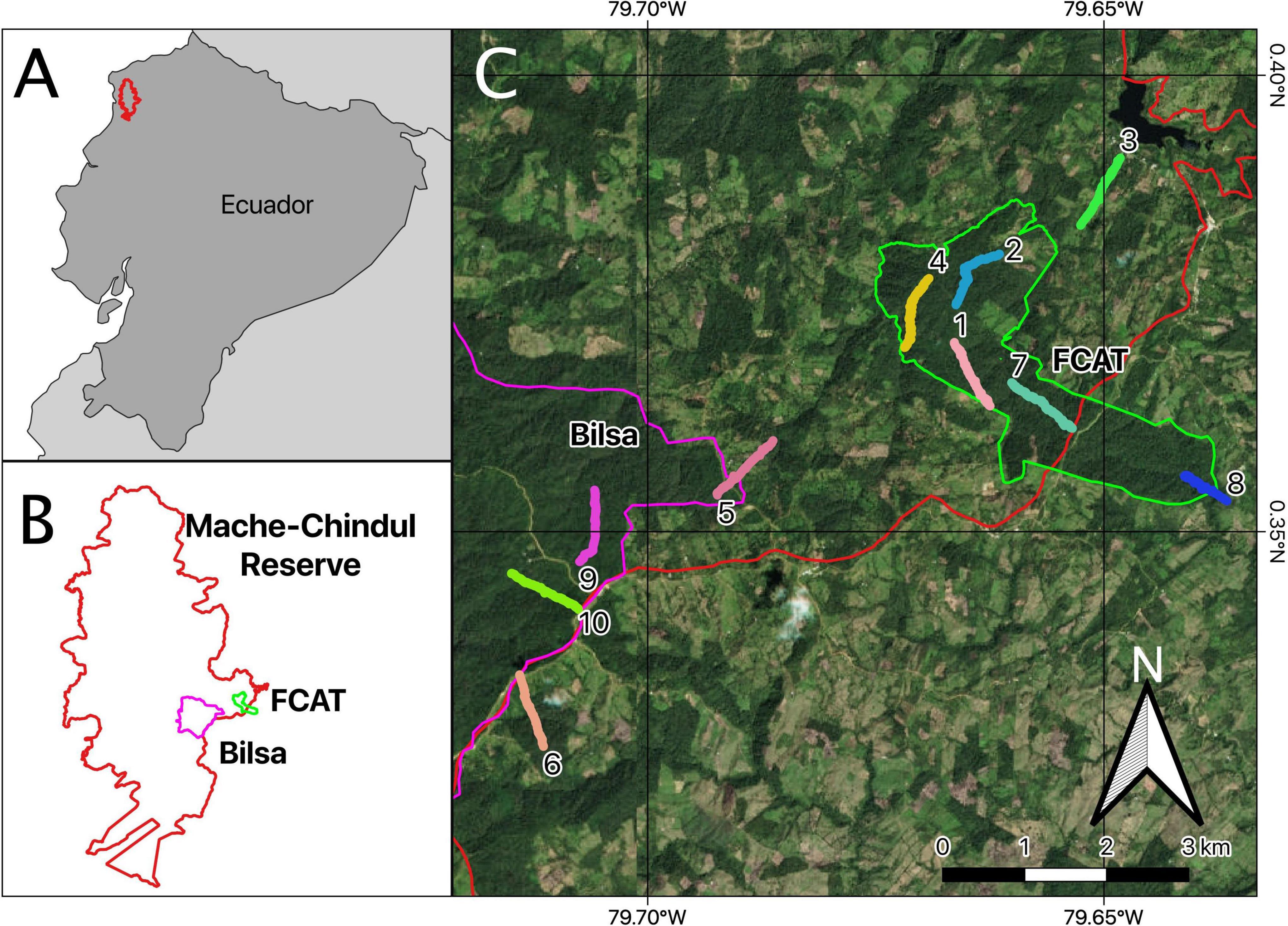
Figure 1. (A): Map of Ecuador with the Mache-Chindul Ecological Reserve (REMACH) outlined in red. (B): A close up of REMACH with the Fundación para la Conservación de los Andes Tropicales (FCAT, green) and the Bilsa Biological Station (Bilsa, purple) outlined. (C): The study site, the transect locations, and associated ecological reserves. Transects, made of continuous 10 × 10 m plots, are distinguished by color.
Palm Transect Sampling
To sample palm communities, we established nine, 10 × 1000-m long transects and one 10 × 650-m long transect in and around REMACH. Each transect was composed of a linear series of 10 × 10 m plots. Transect locations were selected to cover the full range of forest regeneration in the area, ranging from old-growth forest through those highly degraded, as well as agricultural and pasture lands (Supplementary Figure 1). At each plot, we classified the habitat as pasture, crop, or forest; the forest category represented forests of primary and secondary successional stages. Within each plot, we recorded all individuals of palms, assigning them to the lowest possible taxonomic level. We classified each individual into seedlings, juveniles, subadults, and adults following Browne and Karubian, 2016: seedlings have undivided leaves; juveniles, divided leaves but no signs of reproduction; subadults, a size near that of a reproductive adult but with no signs of reproductions; and adults, signs of current or past reproduction. We later collapsed seedlings and juveniles into a single ‘juvenile’ category and sub-adults and adults into a single ‘adult’ category. For clonal species (e.g., Prestoea decurrens), we classified each ramet as an individual.
We characterized the habitat of each 10 × 10 m plot in each transect. We recorded soil moisture (M0750 Soil Moisture Meter, Extech, Boston, MA, United States) and leaf litter at five points within the plot: once in the center and once in each cardinal direction at the plot boundaries. To record leaf litter, we inserted a sharp metal dowel (3 mm diameter) through the litter and into the humus layer. We then marked the dowel, pushed the litter aside, and measured between the two points to the nearest 0.1 cm (Kostel-Hughes et al., 1998). We measured canopy cover using a spherical crown densiometer (Concave – Mode C – Robert E. Lemon, Forest Densiometer – Bartlesville, OK, United States), standing in the center of the plot and recording measurements in the four cardinal directions. We measured understory density using a 1.9-meter-tall metal pole marked with sighting targets placed at 13 evenly spaced intervals. An individual held the pole upright in at the plot boundary in each of four cardinal directions and an individual standing at the center point of each plot counted how many sighting targets were completely unobstructed by vegetation. We recorded canopy height using a laser rangefinder to measure the height of the tallest tree. We counted the number of trees > 10 cm and > 50 cm diameter at breast height (DBH) and the number of Cecropia spp., a species of tree that can be used as a proxy for disturbance (Didham and Lawton, 1999). We recorded elevation using a handheld GPS device (Garmin Foretrex 601, Garmin International, Inc., Olathe, KS, United States). For all forest plots, to estimate the distance to the edge of the forest, we calculated the distance between plot center and the nearest forest edge using the most recent high resolution (5 m) imagery available (PlanetScope, Planet labs, San Francisco, CA, United States) in QGIS (QGIS Development Team, 2020).
Data Analysis
Principal Component Analysis
We used a Principal Component Analysis (PCA) to reduce the dimensionality of the environmental variables and avoid using correlated variables for the abundance, species richness, and community composition analyses. Because many variables were highly correlated with elevation (soil moisture, leaf litter, understory density), we excluded these from the PCA and conducted separate analyses on elevation (below). We treated each 10 × 10 m plot as an independent sampling unit. We conducted each set of analyses separately for juveniles (including seedlings) and adults (including sub-adults).
Species Abundance and Richness Models
We modeled species abundance and richness with generalized linear models to account for non-normal distributions in response variables. We used a Poisson distribution for species richness models (O’Hara and Kotze, 2010) and a negative binomial distribution for palm abundance models (O’Hara and Kotze, 2010). Both distributions model non-negative count data. The negative binomial distribution allows for more variance in the response variable and accordingly accounts for overdispersion. We tested these models against models with other distributions, plotting and comparing residuals and fitted values to check for residual patterns (Zuur et al., 2009). We used PC1, PC2, and elevation as fixed effects. We performed each regression analysis on each transect separately (N = 10). We then calculated the mean regression coefficients from the separate transect analyses, and, using a one-sample t-test, determined if the means were different from zero (cf. Normand et al., 2006; Browne and Karubian, 2016). We quantified the variance around the mean estimated regression coefficients using standard deviation. Performing the regression analyses on transects separately allowed us to remove those that were spatially autocorrelated to ensure that our results were not pseudo-replicated. This method also allowed us to assess how patterns vary between transects by using the variance around the mean. To test for spatial autocorrelation within our models, we used Mantel tests with 999 permutations (Giraldo et al., 2018). We created a distance matrix from the geographical data of each plot, as well as a distance matrix from the residuals of each of the regression models for abundance and richness. Using these matrices, the Mantel test indicated whether there was a significant correlation between the two. The geographical distance matrix was log10-transformed, as logarithmic distance decay is expected to occur due to dispersal limitation (Condit et al., 2002) and based on Haversine distance. We detected significant levels of spatial autocorrelation for the model assessing juvenile richness in one transect, T5 (Supplementary Table 2) and subsequently removed this transect from our analysis. Removing the regression coefficient associated with this model from the one-sample t-test did not change the qualitative outcome of the results.
Community Composition Analysis
Partial Mantel tests assess the correlation between two distance matrices while controlling for a third, allowing us to analyze the effects of spatially independent environmental variables on species composition. Following Kristiansen et al. (2012), we conducted analyses for both juveniles and adults. In each we tested a species composition matrix, using data from all the transects combined, against each of the most informative Principal Components from the PCA as well as elevation while controlling for the geographical distance matrix. Controlling for geographical distance addresses the effect of spatial autocorrelation within the transect sample design. For the juvenile and adult species composition matrices, we used presence-absence data and computed dissimilarity using Sørensen distance (Legendre and Legendre, 1998). To estimate the distances between Principal Component scores as well as elevation, we used Euclidean distance.
To further assess the compositional patterns among juveniles and adults, we employed non-metric multidimensional scaling (NMDS). We removed species with <5 adult individuals or <10 juvenile individuals from the respective analyses, considering these rare species, to reduce noise in the multivariate analysis (McCune et al., 2002). NMDS ordinations were based on dissimilarity matrices generated from species abundance data and Bray–Curtis distance. To evaluate how species composition varied along principal components and elevation, we calculated multiple regression of environmental variables along the ordination axes with 999 permutations post hoc. To visualize the association between environmental variables and community composition, we overlayed these results on the NMDS axes.
Functional Trait Analyses
To assess how functional traits and environmental variables co-vary, we utilized RLQ (a multivariate analysis) and fourth-corner (a bivariate analysis) methods, carrying them out for adults and juveniles. Both analyses rely on information from three tables: R (environmental variable data), Q (functional trait data), and L (species distribution data) (Dray et al., 2014).
Environmental variables included were canopy cover, leaf litter depth, elevation, distance to forest edge, number of Cecropia, number of trees DBH > 10 cm, and number of trees DBH > 50 cm. We removed canopy height, soil moisture, and understory density from the analysis based on their high correlation with the remaining variables. We selected species functional traits based on which were available for all species in “PalmTraits 1.0, a species-level functional trait database of palms worldwide” (Kissling et al., 2019). Traits included were climbing habit, erect habit, solitary stem habit, stem armature, leaf armature, maximum stem height, maximum stem diameter, strata, average fruit length, fruit size, and fruit color conspicuousness (Supplementary Material, Table 3). The following were analyzed as binary variables: climbing habit, erect habit, solitary stem habit, stem and leaf armature, strata, and fruit color conspicuousness.
RLQ is a multivariate analysis that allows the qualitative assessment of the relationships between environmental variables and functional traits mediated by species abundances. The analysis is visualized in an ordination plot, with axes that explain functional trait and environmental variable cross-covariance. Before performing RLQ analysis, each table necessitates a separate ordination. For table L, we applied Correspondence Analysis; for table R, a Principal Component Analysis; and for table Q, a Hill-Smith analysis (Hill and Smith, 1976).
The fourth-corner method evaluates the association between pairs of single variables (i.e., one functional trait and one environmental variable) corresponding to a matrix containing trait-environment association measures. This means here we are testing bivariate associations as opposed to performing a multivariate analysis like the RLQ analysis (Legendre et al., 1997; Dray and Legendre, 2008; Dray et al., 2014). To control type I error, we combined outputs from two permutation models: model 2, which tests the null hypothesis that the environment does not influence the distribution of species with fixed functional traits and model 4, which tests the null hypothesis that the functional traits do not influence the distribution of species with fixed environmental conditions (ter Braak et al., 2012). We performed these tests sequentially, only performing the second test if we rejected the null hypothesis of the first, which further fixes type I error (ter Braak et al., 2012). Because the fourth-corner method deals with bivariate associations and our study considered many functional traits and environmental variables, the number of tests was high, necessitating adjusting p-values (Dray et al., 2014). Here, we used the false discovery rate method (Benjamini and Hochberg, 1995) to control for the error rate.
After performing RLQ analyses and fourth-corner methods separately, we combined the two approaches, addressing some of the issues that arise from the respective analyses (Dray et al., 2014). RLQ provides no significance tests, and our factorial maps were hard to interpret due to the large number of variables considered. Fourth-corner does not consider covariation among environmental variables and produced no significant results when p-values were adjusted. To remedy these issues, we used fourth-corner methods to test the associations between the RLQ axes and the functional traits/environmental variables. Here, we performed model 2 and model 4 permutation tests sequentially (ter Braak et al., 2012) and adjusted p-values using the false discovery rate method (Benjamini and Hochberg, 1995), as outlined above.
All data analyses were performed in R v3.5.2 (R Core Team, 2018). Poisson models and negative binomial models were performed with the ‘glmmTMB’ package (Brooks et al., 2017). Distance matrices, NMDS ordination, NMDS overlays, Mantel tests, and Partial Mantel tests were performed with the ‘vegan’ package (Oksanen et al., 2019). RLQ and fourth-corner analyses were performed with the ‘ade4’ package (Dray and Dufour, 2007).
Results
Summary Data
The 9,750 m2 sampled in our transects included 7,440 m2 forest (76.3%), 1,590 m2 pasture (16.3%), and 720 m2 crop land (7.1%). Sixteen species of palms and 2,989 individuals were identified. Of the 2,989 individuals, 2949 (99%) were found in forest, with 17 found in pasture (all adults) and 23 found in crop land (11 adults, 12 juveniles). In pasture and crop land, Iriartea deltoidea was the most prevalent species, accounting for 64.3% of adults and 25% of juveniles (Table 1). Due to the low number of palms in the non-forested habitat types, we restricted remaining analyses to only forested habitat.
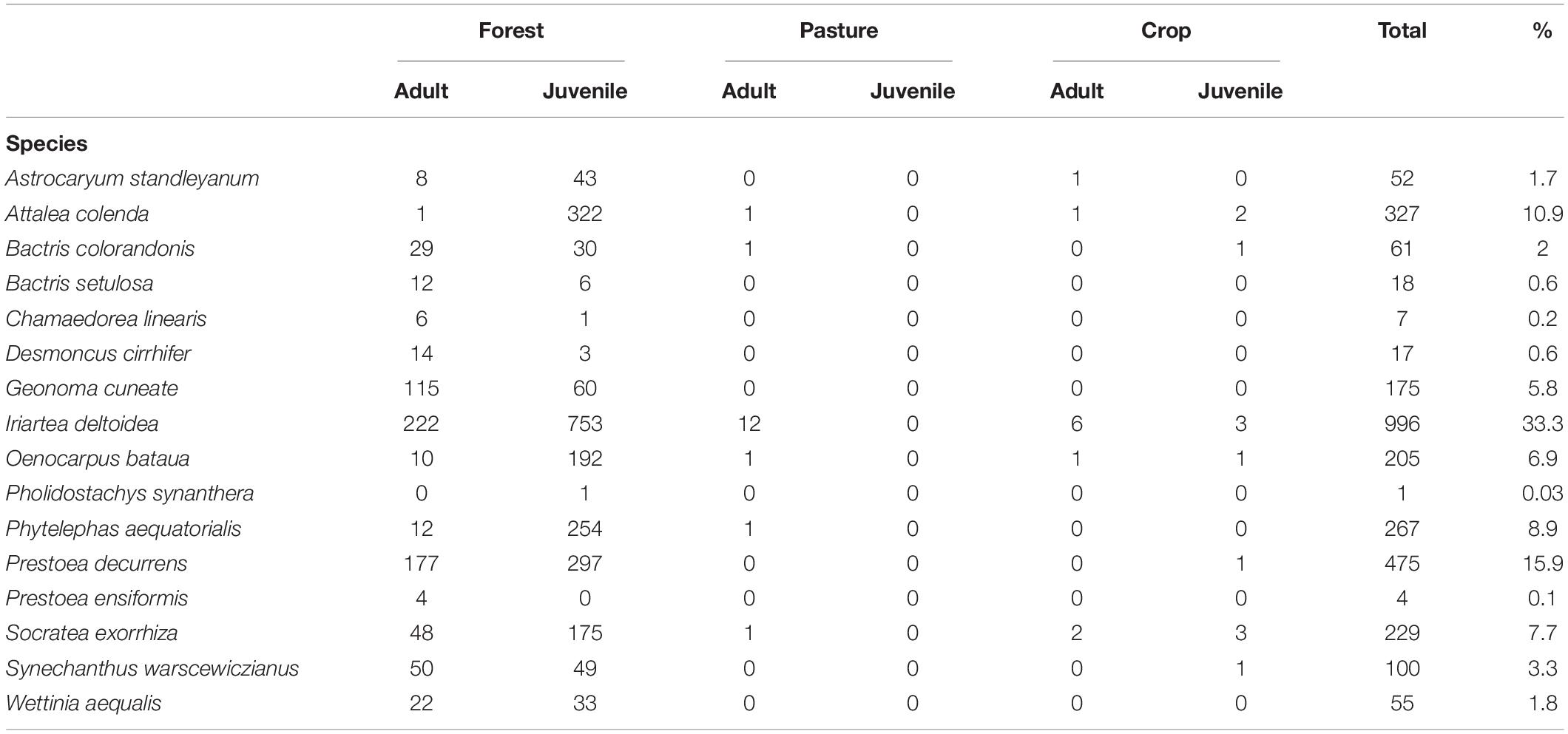
Table 1. Number of individuals of adults (adults and sub-adults) and juveniles (juveniles and seedlings) found in each habitat within the 965 plots in and around the Mache-Chindul Reserve, north-western Ecuador.
Of the 2,949 individuals found in forest, 2,219 (75%) were juveniles and 730 (25%) were adults. For adults, three abundant species accounted for 70% of individuals recorded: I. deltoidea (31%), P. decurrens (24%), and Geonoma cuneata (16%). For juveniles, the three most abundant species (62% of individuals) were I. deltoidea (34%), Attalea colenda (15%), and P. decurrens (13%) (Table 1).
PCA Results
The PCA analysis produced two axes that account for 49.6% of the variance in the six environmental variables used. PC1 accounted for 28.35% of the variance and is dominated by canopy cover, canopy height and density of the pioneer species Cecropia. Thus, we treated PC1 as an index of forest age, with higher values representing younger forests. PC2, which accounted for 21.25% of the variance, was associated with the tree density. High PC2 scores are associated with a higher density of small trees (DBH > 10 cm) and low scores are associated with a higher density of large trees (DBH > 50 cm) (Table 2).
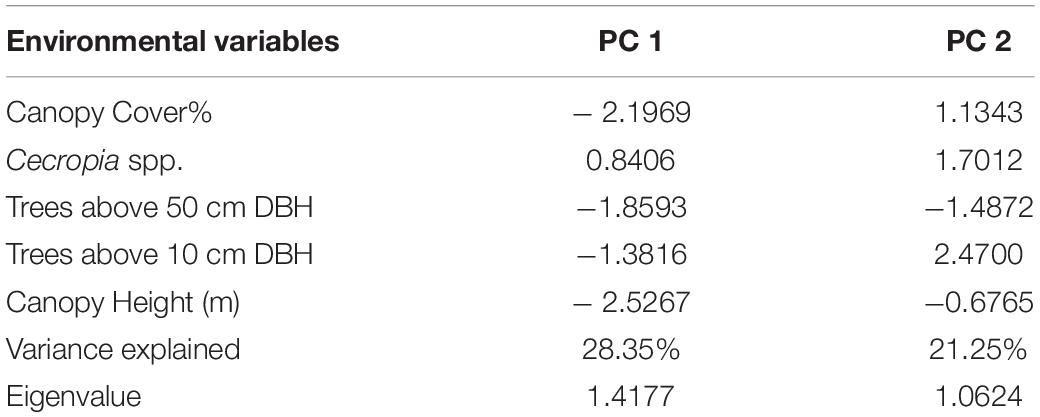
Table 2. The loadings of the nine environmental variables on the first two axes of a Principal Component Analysis, the variance explained by each axis, and the eigenvalues of each axis.
Richness, Abundance, and Community Composition in Forest
Younger forests (as measured by PC1) were associated with decreased abundance of adults and juveniles, decreased adult species richness, and a change in community composition for adults and juveniles (Tables 3, 4). PC1 dissimilarity was more strongly associated with compositional dissimilarity for the juvenile palm community compared to the adult palm community (Table 4). Changes in tree density, as measured by PC2, were not associated with abundance, species richness, or changes in community composition for either age class (Tables 3, 4). As elevation increased, there was an associated decrease in juvenile abundance and a change in adult community composition, but no change in richness for either age class (Tables 3, 4). The patterns of change in richness and abundance varied markedly between transects, as indicated by the variance around the mean regression coefficients (Table 3).

Table 3. Estimated regression coefficients ± standard deviation for the abundance and species richness of adult and juvenile palms.
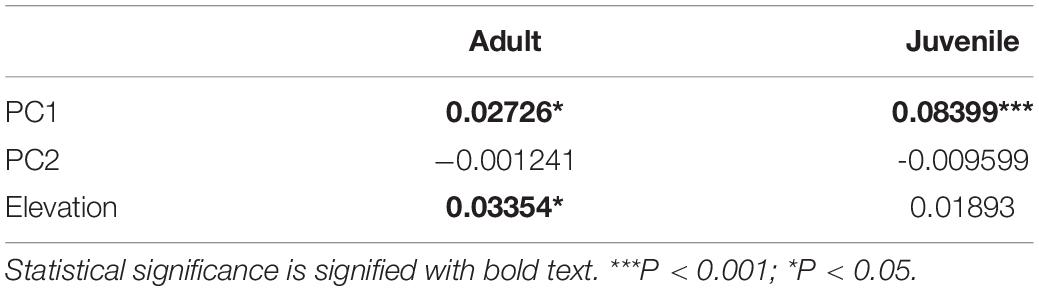
Table 4. Mantel r statistics according to partial Mantel tests of 10 transects (n = 16 species) located in northwestern Ecuador.
The above results on community composition are largely corroborated by NMDS ordination, which indicated that PC1 significantly affected composition in adults and nearly significantly affected juveniles (Table 5). Visualized in the NMDS plots, the second ordination axes reflected differences in species composition across forest age, as indicated by PC1 (Table 5 and Figure 2). For adults, the younger sites and associated species are to the top of the ordination space. For juveniles, the younger sites and associated species are to the bottom of the ordination space. Neither PC2 nor elevation significantly affected composition in this analysis (Table 5).
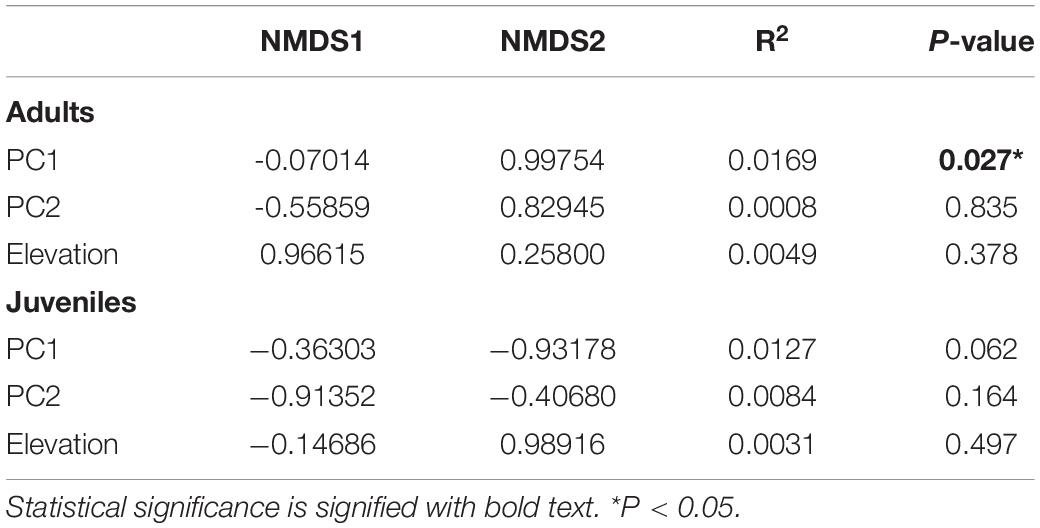
Table 5. Correlation coefficients of environmental variables according to a non-metric multidimensional scaling ordination of 10 transects (n = 16 species) in northwestern Ecuador.
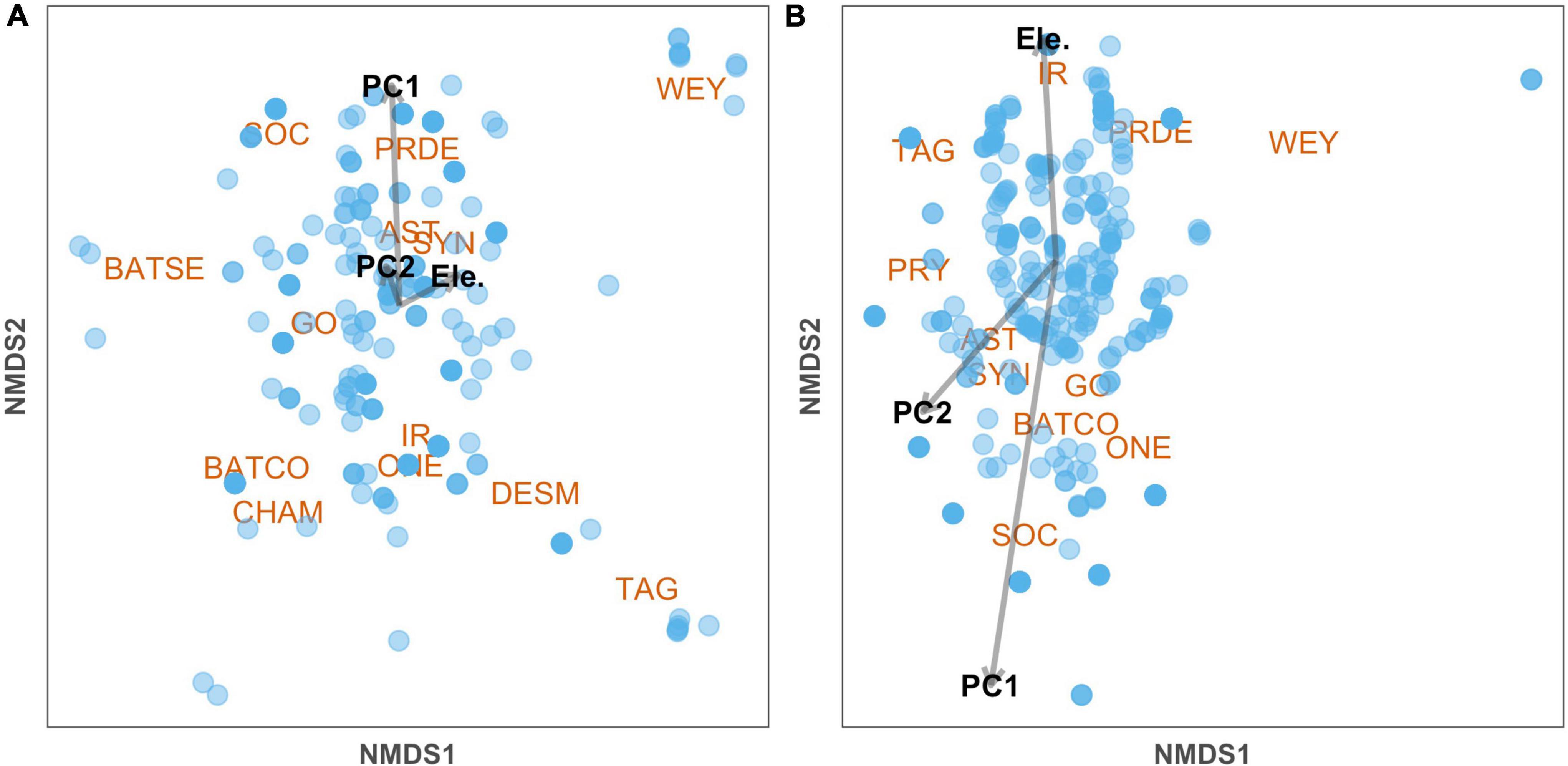
Figure 2. Non-metric multidimensional scaling (NMDS) with projection of environmental variables in 10 transects in northwestern Ecuador, based on the dissimilarity of species abundance data and Bray-Curtis distance for A adults (Stress = 0.069) and B juveniles (Stress = 0.14).
Functional Trait Analysis
For juveniles, the first axis of the RLQ ordination indicates when trees DBH > 10 cm decrease and distance from edge increases (Figure 3A), palm species with armed stems decrease and species with non-solitary stems increase (Figure 3C). This is exemplified by Astrocaryum standleyanum, which has low scores on the axis (Figure 3E), and a solitary, armed stem. For adults, the first axis of the RLQ ordination revealed a positive relationship between elevation, distance to edge, trees DBH > 10 cm (Figure 3B) and palms species with non-solitary stems, non-erect stems, and climbing stems (Figure 3D). This is exemplified by Desmoncus cirrhifer, which has high scores on the axis (Figure 3F), and a stem with non-solitary, non-erect, and climbing habit. The fourth-corner analysis revealed no associations between individual traits and environmental variables when p-values were adjusted for multiple tests.
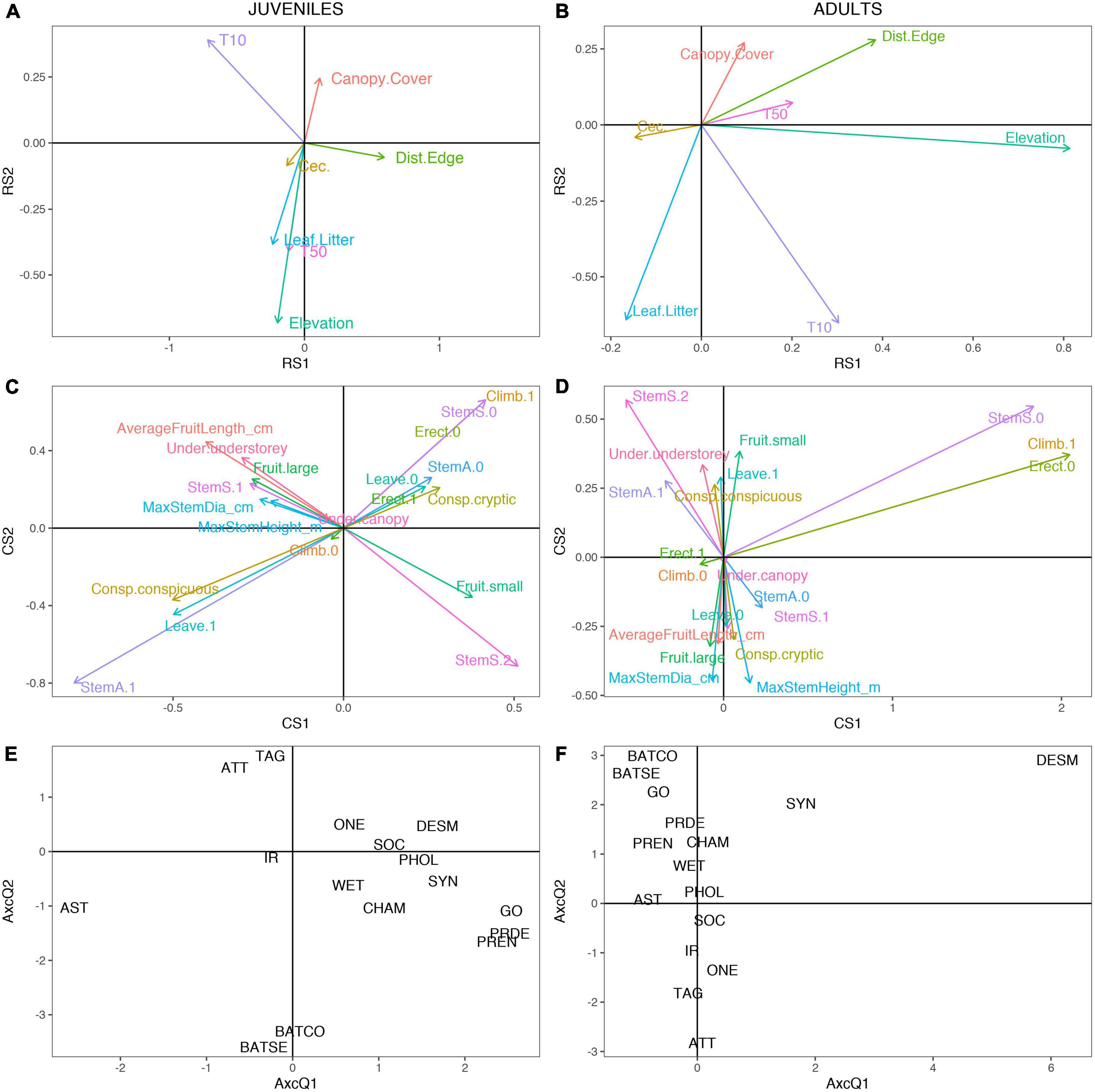
Figure 3. Results of the first two axes of RLQ analysis for juveniles (A,C,E) and adults (B,D,F). (A,B) visualize the coefficients of the environmental variables. (C,D) visualize the functional traits of palm species. (E,F) visualize the scores of the palm species. Codes for species, environmental variables, and traits are available in Supplementary Tables 3–5.
Combining the approaches, there were associations for adults between the second RLQ axis and three functional traits: maximum stem height (p-adjusted = 0.00667), maximum stem diameter (p-adjusted = 0.00667), and solitary stem habit (p-adjusted = 0.0145). The second RLQ axis represents forest maturity, as along it canopy cover and distance from edge of forest increase and trees DBH > 10 cm and leaf litter decrease (Figure 3B). Along this axis, the maximum stem height decreased, maximum stem diameter decreased, and solitarily stemmed palms became less common (Figure 3D). No other associations between the RLQ axes and functional traits were significant after p-values were adjusted, and no significant relationships were found between the axes and the environmental variables.
Discussion
Because of their outsized importance for ecological processes and humans, identifying how Neotropical palm tree diversity, abundance, composition, and functional traits vary in relation to forest age sheds important light on the value of regenerating (i.e., secondary) forests for the conservation of biodiversity and the well-being of human residents. Working in a biodiversity hotspot in northwest Ecuador, we found that abundance decreased and community composition changed for both juveniles and adults in younger, regenerating forests relative to older, more intact forests. Adult species richness also decreased in younger forests, while juvenile species richness remained unaffected. We also documented decreased maximum stem height and diameter among adults, but not juveniles, in more mature forests and an impact of elevation on both juvenile abundance and adult community composition. These results suggest that secondary forests have less diverse palm communities in our study area, emphasizing the importance of undisturbed forests for these iconic components of the tropical flora and the associated food webs and other biotic processes they help to support.
Contrasting patterns between juvenile and adult palms can be useful in inferring future trajectories of palm communities in secondary forests. Our results seem to imply that the palm community will recover in richness as forests age, but with a distinctive composition of species compared to more pristine old-growth forests. The projection of sustained compositional change points to a persistent presence of ‘winners’ and ‘losers’ (Tabarelli et al., 2012), shaped by tolerance to anthropogenic disturbance. In these recovering habitats, available species may be those that are robust against the altered biotic and abiotic factors associated with forest clearing secondary succession. Reduced abundance among juveniles in secondary forests may also point to these forests supporting fewer palms in addition to different subsets of species. For example, Prestoea decurrens is a species that is more abundant in mature forests as juveniles but is more abundant in younger forests as adults (Figure 2). This incongruency may lead to a decrease in P. decurrens in regenerating forests over time if they fail to reproduce in these areas. However, there was marked variation between transects in their responses to forest age, so this may depend on location context. It is also worth noting that the cumulative amount of forest at the landscape level has also been decreasing in the past few decades (Van der Hoek, 2017), which may impact contemporary regeneration processes vs. those occurring in the past.
In the current study, increased elevation affected adult community composition and was associated with a minor decrease in juvenile abundance. Alternatively, Browne and Karubian (2016)’s study in the same area found increased elevation changed community composition and increased abundance for both juvenile and adult palms. These differences between the two studies may be due to the highly variable nature of the effect of elevation on communities, indicating that palm communities respond to elevation in a location-dependent manner. This is supported by the high degree of variation we found between transects in their responses to elevation.
Within the adult palm community, three functional traits representing variation in light acquisition strategies (Chazdon, 1991; Westoby, 1998; Svenning, 2000) were associated with varied regeneration stages: more mature forests were characterized by species with decreased (1) maximum stem height, (2) maximum stem diameter, and (3) solitary stem habit (Tabarelli et al., 2012). Functional traits related to light acquisition may be particularly sensitive to changes in microclimate, like those seen in forest regeneration (Zambrano et al., 2019). Palms of smaller stature, having greater shade tolerance (Chazdon, 1991; Svenning, 2002), may be replaced by species that occur preferentially in areas with increased light levels or species that occur irrespective of light availability in younger forests. For example, Craven et al. (2018) found evidence of functional convergence in light acquisition strategies during secondary succession in a wet tropical forest plant community. Along the successional gradient, trait variation was reduced due to the increased competitive advantage afforded to certain trait combinations. Our results suggest this may be occurring in our study area as well, indicating a potential functional shift in the biota. However, associations between traits and succession were found only in adults. Many palm species of all strata are able to remain in deep shade as acaulescent juveniles in their establishment phase (Kahn and de Granville, 1992; Charles-Dominique et al., 2003). When light availability is increased and reaches the acaulescent juveniles, either by natural or anthropogenic disturbance, taller, solitary palms may become common due to their ability to utilize the increased light, growing quickly and transitioning from a juvenile to an adult (Martínez-Ramos et al., 1988; de Granville, 1992; Cintra and Horna, 1997; Pintaud, 2006). This may explain why we only see the variation in stem height, diameter, and solitary stem habit in adult palms. In addition, our functional trait analyses did not include leaf traits, which may be more important in determining the shade tolerance of juveniles (Poorter et al., 2008).
The influence of shade tolerance on palm composition may be visualized by the abundance of the species Socratea exorrhiza and Phytelephas aequatorialis across a gradient of forest age. S. exorrhiza is a canopy palm that appears to be more abundant in younger forests (Figure 2) and is tolerant to a variety of environments. Other studies have found it to be dominant in regenerating secondary stands as well (Norden et al., 2009), potentially in part because of its ability to tolerate different light regimes. Conversely, P. aequatorialis (Escobar et al., 2022), an understory palm more abundant in older forests (Figure 2), regenerates best in shaded environments (Palacios and Jaramillo, 2005). This may limit the species’ ability to thrive in younger forests.
Alternatively, differences in stem height, stem diameter, and solitary stem habit may reflect the fact that some canopy palms may be left standing in pastures or agricultural land as ‘remnants’, around which regenerating forests may grow when the lands are abandoned. Canopy palms often remain as isolated trees, while hardwoods and understory palms are cleared (Guevara et al., 1998; Harvey et al., 2004). The high abundance of remnants, and their presence as a seed source, may catalyze a proliferation of the remnant canopy species in regenerating forest patches (Aldrich and Hamrick, 1998). The abundant, limited subset of remnant canopy palms may be over-represented relative to other functional groups in younger, regenerating forests relative to mature, intact forests. Other studies have found an overrepresentation of remnant trees in nearby forest patches as well (Aldrich and Hamrick, 1998) and have found that remnant trees are able to influence the composition of a regenerating forest for decades (Schlawin and Zahawi, 2008; Sandor and Chazdon, 2014). The proliferation of remnant palms in regenerating habitats may be reflected by the abundance of Oenocarpus bataua juveniles in younger forest, as O. bataua are often left as remnants in the study area. The potential influence of remnant trees in this study highlights the need to consider remnant trees when attempting to quantify patterns of biodiversity. It also points to a need to better understand the impacts of remnant palms on downstream ecological processes (e.g., frugivory) and on the abundance and diversity of species they support.
Utilizing species richness, community composition, and functional trait analysis in concert helped us to produce a more cohesive view of palm communities. Across a gradient of forest regeneration, the juvenile community changed in composition but not in species richness, pointing to the phenomenon of plant species richness recovering more quickly than species composition in tropical forests (Finegan, 1996; Liebsch et al., 2008; Martin et al., 2013; Rozendaal et al., 2019). As such, our study adds to global patterns of uncoupling between species richness and changes in community composition (Blowes et al., 2019) and stresses the importance of utilizing both species richness and community composition.
Our study is one of the first to address how functional traits vary along environmental axes within the palm community in the study area. Our results highlight how functional traits differ between adult and juvenile communities, both through the RLQ ordination (Figure 3) and through the fourth-corner analysis results. Utilizing functional trait composition pinpointed potential mechanisms for diversity loss, highlighting the value of combining trait analyses with traditional metrics of diversity. Our results may be of particular value as palms are often removed in functional trait analyses of plant communities due to their differences in stem and leaf structure compared to dicot trees (Boukili and Chazdon, 2017), further restricting our understanding of functional trait patterns during succession.
Alongside evidence that secondary forest environments are selecting for different functional traits and contribute to shifts in diversity and composition, it is worth noting that other drivers are likely influencing the palm community. Species that may establish and survive in secondary habitats under ideal conditions may experience decreased success because of landscape-scale processes that can degrade forest habitat. In a highly degraded landscape like that of our study, these processes may include forest loss at the landscape scale (Rocha-Santos et al., 2016) and the intensity of previous land use (Karp et al., 2012; Jakovac et al., 2015). Both of these processes can independently affect palm diversity (Vandermeer et al., 2010; Benchimol et al., 2017). Forest loss can alter the diversity, density, and/or distance traveled by animal seed dispersers (Montúfar et al., 2011; Browne and Karubian, 2018) in addition to reducing proximity of regrowth to old-growth seed sources (Norden et al., 2009), with implications for dispersal outcomes of palm species. Intense previous land uses may alter abiotic factors vital to the regeneration of many palm species (Eiserhardt et al., 2011), like soil quality and nutrient availability (Lawrence et al., 2007; Runyan et al., 2012). The changes in the palm communities observed here are most likely due to multiple, potentially synergistic explanations (Benchimol et al., 2017). Moreover, our data were collected during one season, and the predictions on community change may change with a multi-year study. Future studies interested in palm communities, particularly when studies concern seedlings, should prioritize long-term data collection (Norden et al., 2009). Due to the site-specific and time-sensitive nature of the myriad factors that can influence community assembly processes, we caution the generalization of our results. We also caution interpretation of our results as general trends due to the possible effects of spatial autocorrelation, a longstanding issue in ecological survey data when transects are used.
Our results add to a line of evidence suggesting that palm communities are susceptible to changes caused by human-induced deforestation. Our study, to our knowledge, is the first to examine the effect of forest age on functional trait composition in palm communities. Our findings suggest that smaller palms, like P. aequatorialis, may be at greater risk in the palm community as undisturbed forest continues to disappear. While the juvenile community did not exhibit changes in functional trait composition, this may change over time. As the proportion of secondary forest increases, sensitive adult species may be limited to increasingly smaller areas and face increasing competition from remnant trees and less sensitive species. Over time, composition may continue to become more dissimilar and diversity lost.
Data Availability Statement
The raw data supporting the conclusions of this article will be made available by the authors, without undue reservation.
Author Contributions
JK and SL designed the research. SL, KN, JO, DC, and JJ contributed to data collection. SL and KN performed the statistical analyses. KN produced the figures. SL wrote the first draft of the manuscript. SL, KN, and JK contributed to revision. All authors contributed to the article and approved the submitted version.
Funding
Funding was provided by the Conservation Food and Health Foundation; Disney Conservation Fund; National Science Foundation (EAGER #1548548 and BEE # 2039842); Tulane University (Newcomb-Tulane College; Newcomb College Institute); and the United States Fish and Wildlife Service Neotropical Migratory Bird Act. We conducted work under permits from the Ecuadorian Ministry of the Environment (#010-2014-IC-FLO-FAU-DPE-MA).
Conflict of Interest
The authors declare that the research was conducted in the absence of any commercial or financial relationships that could be construed as a potential conflict of interest.
Publisher’s Note
All claims expressed in this article are solely those of the authors and do not necessarily represent those of their affiliated organizations, or those of the publisher, the editors and the reviewers. Any product that may be evaluated in this article, or claim that may be made by its manufacturer, is not guaranteed or endorsed by the publisher.
Acknowledgments
We thank the Ecuadorian Ministry of the Environment, the Jatun Sacha Foundation, and landowners in and around the Mache-Chindul Reserve for maintaining these forests and granting access. We also thank the Fundacion para la Conservacion de los Andes Tropicales (FCAT), particularly Margarita Baaquero, Luis Carrasco, Fernando Castillo and Nelson Gonzalez for logistical support.
Supplementary Material
The Supplementary Material for this article can be found online at: https://www.frontiersin.org/articles/10.3389/fevo.2022.678125/full#supplementary-material
References
Achard, F., Eva, H. D., Stibig, H. J., Mayaux, P., Gallego, J., Richards, T., et al. (2002). Determination of deforestation rates of the world’s humid tropical forests. Science 297, 999–1002. doi: 10.1126/science.1070656
Aldrich, P. R., and Hamrick, J. L. (1998). Reproductive dominance of pasture trees in a fragmented tropical forest mosaic. Science 281, 103–105. doi: 10.1126/science.281.5373.103
Araia, M. G., Chirwa, P. W., and Assédé, E. S. P. (2019). Contrasting the effect of forest landscape condition to the resilience of species diversity in a human modified landscape: implications for the conservation of tree species. Land 9:4. doi: 10.3390/land9010004
Arroyo-Rodríguez, V., Aguirre, A., Benítez-Malvido, J., and Mandujano, S. (2007). Impact of rain forest fragmentation on the population size of a structurally important palm species: Astrocaryum mexicanum at Los Tuxtlas, Mexico. Biol. Conservation 138, 198–206. doi: 10.1016/j.biocon.2007.04.016
Arroyo-Rodríguez, V., Melo, F. P. L., Martínez-Ramos, M., Bongers, F., Chazdon, R. L., Meave, J. A., et al. (2017). Multiple successional pathways in human-modified tropical landscapes: new insights from forest succession, forest fragmentation and landscape ecology research: multiple successional pathways. Biol. Rev. 92, 326–340. doi: 10.1111/brv.12231
Baez, S., and Balslev, H. (2007). Edge effects on palm diversity in rain forest fragments in western Ecuador. Biodiversity Conservation 16, 2201–2211. doi: 10.1007/s10531-007-9159-5
Barlow, J., Gardner, T. A., Araujo, I. S., Avila-Pires, T. C., Bonaldo, A. B., Costa, J. E., et al. (2007). Quantifying the biodiversity value of tropical primary, secondary, and plantation forests. Proc. Natl. Acad. Sci. U S A. 104, 18555–18560. doi: 10.1073/pnas.0703333104
Benchimol, M., Talora, D. C., Mariano-Neto, E., Oliveira, T. L. S., Leal, A., Mielke, M. S., et al. (2017). Losing our palms: the influence of landscape-scale deforestation on Arecaceae diversity in the Atlantic forest. Forest Ecol. Manag. 384, 314–322. doi: 10.1016/j.foreco.2016.11.014
Benjamini, Y., and Hochberg, Y. (1995). Controlling the false discovery rate: a practical and powerful approach to multiple testing. J. R. Stat. Soc. Ser. B Methodol. 57, 289–300. doi: 10.1111/j.2517-6161.1995.tb02031.x
Bernal, R., Torres, C., García, N., Isaza, C., Navarro, J., Vallejo, M. I., et al. (2011). Palm management in South America. Bot. Rev. 77, 607–646. doi: 10.1007/s12229-011-9088-6
Blowes, S. A., Supp, S. R., Antão, L. H., Bates, A., Bruelheide, H., Chase, J. M., et al. (2019). The geography of biodiversity change in marine and terrestrial assemblages. Science 366, 339–345. doi: 10.1126/science.aaw1620
Boukili, V. K., and Chazdon, R. L. (2017). Environmental filtering, local site factors and landscape context drive changes in functional trait composition during tropical forest succession. Perspect. Plant Ecol. Evol. Systemat. 24, 37–47. doi: 10.1016/j.ppees.2016.11.003
Bradshaw, C. J., Sodhi, N. S., and Brook, B. W. (2009). Tropical turmoil: a biodiversity tragedy in progress. Front. Ecol. Environ. 7:79–87. doi: 10.1890/070193
Brooks, M., Kristensen, K., van Benthem, K., Magnusson, A., Berg, C., Nielsen, A., et al. (2017). glmmTMB balances speed and flexibility among packages for zero-inflated generalized linear mixed modeling. R J. 9, 378–400. doi: 10.32614/rj-2017-066
Browne, L., and Karubian, J. (2016). Diversity of palm communities at different spatial scales in a recently fragmented tropical landscape. Botan. J. Linnean Soc. 182, 451–464. doi: 10.1111/boj.12384
Browne, L., and Karubian, J. (2018). Habitat loss and fragmentation reduce effective gene flow by disrupting seed dispersal in a neotropical palm. Mol. Ecol. 27, 3055–3069. doi: 10.1111/mec.14765
Capers, R. S., Chazdon, R. L., Brenes, A. R., and Alvarado, B. V. (2005). Successional dynamics of woody seedling communities in wet tropical secondary forests: seedling dynamics in tropical secondary forests. J. Ecol. 93, 1071–1084. doi: 10.1111/j.1365-2745.2005.01050.x
Charles-Dominique, P., Chave, J., Dubois, M.-A., De Granville, J. J., Riera, B., and Vezzoli, C. (2003). Colonization front of the understorey palm Astrocaryum sciophilum in a pristine rain forest of French Guiana: spatial patterns of Astrocaryum sciophilum. Global Ecol. Biogeography 12, 237–248. doi: 10.1046/j.1466-822X.2003.00020.x
Chazdon, R. L. (2008). Chance and determinism in tropical forest succession. Trop. For. Community Ecol. 10, 384–408.
Chazdon, R. L. (1991). Plant size and form in the understory palm genus geonoma: are species variations on a theme? Am. J. Botany 78, 680–694. doi: 10.1002/j.1537-2197.1991.tb12592.x
Cintra, R., and Horna, V. (1997). Seed and seedling survival of the palm Astrocaryum murumuru and the legume tree Dipteryx micrantha in gaps in Amazonian forest. J. Trop. Ecol. 13, 257–277. doi: 10.1017/s0266467400010440
Clark, J. L., Neill, D. A., and Asanza, M. (2006). Floristic checklist of the mache-chindul mountains of Northwestern ecuador. Contribut. U S Natl. Herb. 54, 1–180.
Condit, R., Pitman, N., Leigh, E. G., Chave, J., Terborgh, J., Foster, R. B., et al. (2002). Beta-diversity in tropical forest trees. Science 295, 666–669. doi: 10.1126/science.1066854
Craven, D., Hall, J. S., Berlyn, G. P., Ashton, M. S., and van Breugel, M. (2018). Environmental filtering limits functional diversity during succession in a seasonally wet tropical secondary forest. J. Veg. Sci. 29, 511–520. doi: 10.1111/jvs.12632
de Granville, J. J. (1992). Life forms and growth strategies of Guianan palms as related to their ecology. Bull. I. Fr. Étud. And. 21, 533–548.
Dıìaz, S., and Cabido, M. (2001). Vive la différence: plant functional diversity matters to ecosystem processes. Trends Ecol. Evol. 16, 646–655. doi: 10.1016/s0169-5347(01)02283-2
Díaz, S., Purvis, A., Cornelissen, J. H. C., Mace, G. M., Donoghue, M. J., Ewers, R. M., et al. (2013). Functional traits, the phylogeny of function, and ecosystem service vulnerability. Ecol. Evol. 3, 2958–2975. doi: 10.1002/ece3.601
Didham, R. K., and Lawton, J. H. (1999). Edge structure determines the magnitude of changes in microclimate and vegetation structure in tropical forest fragments1. Biotropica 31, 17–30. doi: 10.1111/j.1744-7429.1999.tb00113.x
Dodson, C. H., and Gentry, A. H. (1991). Biological extinction in western ecuador. Ann. Missouri Botan. Garden 78:273. doi: 10.2307/2399563
Dray, S., and Dufour, A. B. (2007). The ade4 package: implementing the duality diagram for ecologists. J. Statistical Software 22, 1–20.
Dray, S., and Legendre, P. (2008). Testing the species traits-environment relationships: the fourth-corner problem revisited. Ecology 89, 3400–3412. doi: 10.1890/08-0349.1
Dray, S., Choler, P., Dolédec, S., Peres-Neto, P. R., Thuiller, W., Pavoine, S., et al. (2014). Combining the fourth-corner and the RLQ methods for assessing trait responses to environmental variation. Ecology 95, 14–21. doi: 10.1890/13-0196.1
Eiserhardt, W. L., Svenning, J. C., Kissling, W. D., and Balslev, H. (2011). Geographical ecology of the palms (Arecaceae): determinants of diversity and distributions across spatial scales. Ann. Bot. 108, 1391–1416. doi: 10.1093/aob/mcr146
Escobar, S., Helmstetter, A. J., Montúfar, R., Couvreur, T. L., and Balslev, H. (2022). Phylogenomic relationships and historical biogeography in the South American vegetable ivory palms (Phytelepheae). Mol. Phylogenet. Evol. 166:107314. doi: 10.1016/j.ympev.2021.107314
Finegan, B. (1996). Pattern and process in neotropical secondary rain forests: the first 100 years of succession. Trends Ecol. Evol. 11, 119–124. doi: 10.1016/0169-5347(96)81090-1
Food and Agriculture Organization of the United Nations [FAO] (2010). Global Forest Resources Assessment. Rome: FAO. Main report. FAO Forestry Paper 163.
Galetti, M., Donatti, C. I., Pires, A. S., Guimarães, P. R., and Jordano, P. (2006). Seed survival and dispersal of an endemic Atlantic forest palm: the combined effects of defaunation and forest fragmentation. Botan. J. Linnean Soc. 151, 141–149. doi: 10.1111/j.1095-8339.2006.00529.x
Gardner, T. A., Barlow, J., Parry, L. W., and Peres, C. A. (2007). Predicting the uncertain future of tropical forest species in a data vacuum. Biotropica 39, 25–30. doi: 10.1111/j.1744-7429.2006.00228.x
Gardner, T. A., Barlow, J., Sodhi, N. S., and Peres, C. A. (2010). A multi-region assessment of tropical forest biodiversity in a human-modified world. Biol. Conserv. 143, 2293–2300. doi: 10.1016/j.biocon.2010.05.017
Gentry, A. H. (1986). Species richness and floristic composition of Chocó region plant communities. Caldasia 15, 71–91.
Gibson, L., Lee, T. M., Koh, L. P., Brook, B. W., Gardner, T. A., Barlow, J., et al. (2011). Primary forests are irreplaceable for sustaining tropical biodiversity. Nature 478, 378–381. doi: 10.1038/nature10425
Giraldo, R., Caballero, W., and Camacho-Tamayo, J. (2018). Mantel test for spatial functional data. AStA Adv. Statistical Anal. 102, 21–39. doi: 10.1007/s10182-016-0280-1
Goulding, M., and Smith, N. (2007). Palms: Sentinels for Amazon Conservation. St. Louis, MO: Missouri Botanical Garden Press.
Green, P. T., Harms, K. E., and Connell, J. H. (2014). Nonrandom, diversifying processes are disproportionately strong in the smallest size classes of a tropical forest. Proc. Natl. Acad. Sci. U S A. 111, 18649–18654. doi: 10.1073/pnas.1321892112
Guevara, S., Laborde, J., and Sánchez, G. (1998). Are isolated remnant trees in pastures a fragmented canopy? Selbyana 19, 34–43.
Harvey, C. A., Tucker, N. I., and Estrada, A. (2004). “Live fences, isolated trees, and windbreaks: tools for conserving biodiversity in fragmented tropical landscapes,” in Agroforestry and Biodiversity Conservation in Tropical Landscapes, eds G. Schroth, G. A. B. Fonseca, C. A. Harvey, C. Gascon, H. L. Vasconcelos, and A. M. N. Izac (Washington, DC: Island Press).
Hill, M., and Smith, A. (1976). Principal component analysis of taxonomic data with multi-state discrete characters. Taxon 25, 249–255. doi: 10.2307/1219449
Jakovac, C. C., Peña-Claros, M., Kuyper, T. W., and Bongers, F. (2015). Loss of secondary-forest resilience by land-use intensification in the Amazon. J. Ecol. 103, 67–77. doi: 10.1111/1365-2745.12298
Kahn, F., and de Granville, J.-J. (1992). Palms in Forest Ecosystems of Amazonia, Ecological Studies. Berlin, NY.: Springer-Verlag.
Karp, D. S., Rominger, A. J., Zook, J., Ranganathan, J., Ehrlich, P. R., and Daily, G. C. (2012). Intensive agriculture erodes β-diversity at large scales. Ecol. Lett. 15, 963–970. doi: 10.1111/j.1461-0248.2012.01815.x
Kissling, W. D., Balslev, H., Baker, W. J., Dransfield, J., Göldel, B., Lim, J. Y., et al. (2019). PalmTraits 1.0, a species-level functional trait database of palms worldwide. Sci. Data 6:178. doi: 10.1038/s41597-019-0189-0
Kleemann, J., Zamora, C., Villacis-Chiluisa, A. B., Cuenca, P., Koo, H., Noh, J. K., et al. (2022). Deforestation in continental ecuador with a focus on protected areas. Land 11:268. doi: 10.3390/land11020268
Kostel-Hughes, F., Young, T. P., and Carreiro, M. M. (1998). Forest leaf litter quantity and seedling occurrence along an urban-rural gradient. Urban Ecosystems 2, 263–278.
Kristiansen, T., Svenning, J. C., Eiserhardt, W. L., Pedersen, D., Brix, H., Munch Kristiansen, S., et al. (2012). Environment versus dispersal in the assembly of western Amazonian palm communities: Amazonian palm community composition: inundation, soil and dispersal. J. Biogeography 39, 1318–1332. doi: 10.1111/j.1365-2699.2012.02689.x
Laurance, W. F., Nascimento, H. E. M., Laurance, S. G., Andrade, A. C., Fearnside, P. M., Ribeiro, J. E. L., et al. (2006). Rain forest fragmentation and the proliferation of successional trees. Ecology 87, 469–482. doi: 10.1890/05-0064
Lawrence, D., D’Odorico, P., Diekmann, L., DeLonge, M., Das, R., and Eaton, J. (2007). Ecological feedbacks following deforestation create the potential for a catastrophic ecosystem shift in tropical dry forest. Proc. Natl. Acad. Sci. U S A. 104, 20696–20701. doi: 10.1073/pnas.0705005104
Legendre, P., Galzin, R., and Harmelin-Vivien, M. L. (1997). Relating behavior to habitat: solutions to thefourth-corner problem. Ecology 78, 547–562. doi: 10.1890/0012-9658(1997)078[0547:rbthst]2.0.co;2
Letcher, S. G., and Chazdon, R. L. (2009). Rapid recovery of biomass, species richness, and species composition in a forest chronosequence in Northeastern costa rica: rapid forest recovery in Costa Rica. Biotropica 41, 608–617. doi: 10.1111/j.1744-7429.2009.00517.x
Liebsch, D., Marques, M. C. M., and Goldenberg, R. (2008). How long does the Atlantic Rain Forest take to recover after a disturbance? changes in species composition and ecological features during secondary succession. Biol. Conserv. 141, 1717–1725. doi: 10.1016/j.biocon.2008.04.013
Macía, M. J., Armesilla, P. J., Cámara-Leret, R., Paniagua-Zambrana, N., Villalba, S., Balslev, H., et al. (2011). Palm uses in northwestern south america: a quantitative review. Botan. Rev. 77, 462–570. doi: 10.1007/s12229-011-9086-8
Martin, P. A., Newton, A. C., and Bullock, J. M. (2013). Carbon pools recover more quickly than plant biodiversity in tropical secondary forests. Proc. R. Soc. B. 280:20132236. doi: 10.1098/rspb.2013.2236
Martínez-Ramos, M., Sarukhan, J., and Piñero, D. (1988). “The demography of tropical trees in the context of forest gap dynamics: the case of Astrocaryum mexicanum at Los Tuxtlas tropical rain forest,” in Plant Population Ecology: The 28th Symposium of the British Ecological Society, eds MJ Davy, A. J. Hutchings, and AR Watkinson (Oxford: Blackwell).
McCune, B., Grace, J. B., and Urban, D. L. (2002). Analysis of Ecological Communities. Gleneden Beach, OR: MjM software design.
Melo, F. P. L., Arroyo-Rodríguez, V., Fahrig, L., Martínez-Ramos, M., and Tabarelli, M. (2013). On the hope for biodiversity-friendly tropical landscapes. Trends Ecol. Evol. 28, 462–468. doi: 10.1016/j.tree.2013.01.001
Montúfar, R., Anthelme, F., Pintaud, J. C., and Balslev, H. (2011). Disturbance and resilience in tropical american palm populations and communities. Bot. Rev. 77, 426–461. doi: 10.1007/s12229-011-9085-9
Muñoz, G., Trøjelsgaard, K., and Kissling, W. D. (2019). A synthesis of animal-mediated seed dispersal of palms reveals distinct biogeographical differences in species interactions. J. Biogeogr. 46, 466–484. doi: 10.1111/jbi.13493
Muscarella, R., Emilio, T., Phillips, O. L., Lewis, S. L., Slik, F., Baker, W. J., et al. (2020). The global abundance of tree palms. Global Ecol. Biogeogr. 29, 1495–1514. doi: 10.1111/geb.13123
Myers, N., Mittermeier, R. A., Mittermeier, C. G., da Fonseca, G. A. B., and Kent, J. (2000). Biodiversity hotspots for conservation priorities. Nature 403, 853–858. doi: 10.1038/35002501
Norden, N., Chazdon, R. L., Chao, A., Jiang, Y.-H., and Vílchez-Alvarado, B. (2009). Resilience of tropical rain forests: tree community reassembly in secondary forests. Ecol. Lett. 12, 385–394. doi: 10.1111/j.1461-0248.2009.01292.x
Normand, S., Vormisto, J., Svenning, J.-C., Grández, C., and Balslev, H. (2006). Geographical and environmental controls of palm beta diversity in paleo-riverine terrace forests in Amazonian Peru. Plant Ecol. 186, 161–176. doi: 10.1007/s11258-006-9120-9
O’Hara, R., and Kotze, J. (2010). Do not log-transform count data. Nat. Prec. 1, 118–122. doi: 10.1111/j.2041-210x.2010.00021.x
Oksanen, J., Guillaume Blanchet, F., Friendly, M., Kindt, R., Legendre, P., McGlinn, D., et al. (2019). vegan: Community Ecology Package. R package version 2.5–6.
Paine, C. E. T., Baraloto, C., Chave, J., and Hérault, B. (2011). Functional traits of individual trees reveal ecological constraints on community assembly in tropical rain forests. Oikos 120, 720–727. doi: 10.1111/j.1600-0706.2010.19110.x
Palacios, W., and Jaramillo, N. (2005). Ecological forest species groups in Northeastern Ecuador and their importance for the managenment of indigenous forest. Lyonia 6, 55–75.
Pintaud, J. (2006). The impact of forest disturbance on the palms of New Caledonia. Palms-Lawrence 50:123.
Poorter, L., Wright, S. J., Paz, H., Ackerly, D. D., Condit, R., Ibarra-Manríquez, G., et al. (2008). Are functional traits good predictors of demographic rates? Evid. Five Neotrop. Forests Ecol. 89, 1908–1920. doi: 10.1890/07-0207.1
R Core Team (2018). R: A Language and Environment for Statistical Computing. Vienna: R Foundation for Statistical Computing.
Rocha-Santos, L., Pessoa, M. S., Cassano, C. R., Talora, D. C., Orihuela, R. L. L., Mariano-Neto, E., et al. (2016). The shrinkage of a forest: landscape-scale deforestation leading to overall changes in local forest structure. Biol. Conserv. 196, 1–9. doi: 10.1016/j.biocon.2016.01.028
Rozendaal, D. M. A., Bongers, F., Aide, T. M., Alvarez-Dávila, E., Ascarrunz, N., Balvanera, P., et al. (2019). Biodiversity recovery of neotropical secondary forests. Sci. Adv. 5:eaau3114. doi: 10.1126/sciadv.aau3114
Rudel, T. K., Defries, R., Asner, G. P., and Laurance, W. F. (2009). Changing drivers of deforestation and new opportunities for conservation. Conserv. Biol. 23, 1396–1405. doi: 10.1111/j.1523-1739.2009.01332.x
Runyan, C. W., D’Odorico, P., and Lawrence, D. (2012). Effect of repeated deforestation on vegetation dynamics for phosphorus-limited tropical forests: coupled vegetation-phosphorus dynamics. J. Geophys. Res. 117:G001841. doi: 10.1029/2011JG001841
Sandor, M. E., and Chazdon, R. L. (2014). Remnant trees affect species composition but not structure of tropical second-growth forest. PLoS One 9:e83284. doi: 10.1371/journal.pone.0083284
Sardeshpande, M., and Shackleton, C. (2019). Wild edible fruits: a systematic review of an under-researched multifunctional NTFP (Non-Timber Forest Product). Forests 10:467. doi: 10.3390/f10060467
Schlawin, J. R., and Zahawi, R. A. (2008). ‘Nucleating’ succession in recovering neotropical wet forests: the legacy of remnant trees. J. Veg. Sci. 19, 485–492. doi: 10.3170/2008-8-18387
Su, J. C., Debinski, D. M., Jakubauskas, M. E., and Kindscher, K. (2004). Beyond species richness: community similarity as a measure of cross-taxon congruence for coarse-filter conservation. Conserv. Biol. 18, 167–173. doi: 10.1111/j.1523-1739.2004.00337.x
Svenning, J. C. (2000). Growth strategies of clonal palms (Arecaceae) in a neotropical rainforest, Yasuni, Ecuador. Aust. J. Bot. 48:167. doi: 10.1071/BT98048
Svenning, J. C. (2002). Crown illumination limits the population growth rate of a neotropical understorey palm (Geonoma macrostachys, Arecaceae). Plant Ecol. 159, 185–199. doi: 10.1023/A:1015520116260
Tabarelli, M., Aguiar, A. V., Ribeiro, M. C., Metzger, J. P., and Peres, C. A. (2010). Prospects for biodiversity conservation in the Atlantic Forest: lessons from aging human-modified landscapes. Biol. Conserv. 143, 2328–2340. doi: 10.1016/j.biocon.2010.02.005
Tabarelli, M., Peres, C. A., and Melo, F. P. L. (2012). The ‘few winners and many losers’ paradigm revisited: emerging prospects for tropical forest biodiversity. Biol. Conserv. 155, 136–140. doi: 10.1016/j.biocon.2012.06.020
Tamayo, F., Torres, B., Fischer, R., Lajones, A., Cervantes, R., Corozo, C., et al. (2020). “Characterization of forest landscapes in the ecuadorian Northwest: deforestation and sociocultural aspects,” in Deforestación En Paisajes Forestales Tropicales Del Ecuador: Bases Científicas Para Perspectivas Políticas, ed. Universidad Estatal Amazo’nica - Instituto Johann Heinrich von Thünen (Puyo: Universidad Estatal Amazónica - Instituto Johann Heinrich von Thüen).
ter Braak, C. J., Cormont, A., and Dray, S. (2012). Improved testing of species traits-environment relationships in the fourth-corner problem. Ecology 93, 1525–1526. doi: 10.1890/12-0126.1
ter Steege, H., Pitman, N. C. A., Sabatier, D., Baraloto, C., Salomao, R. P., Guevara, J. E., et al. (2013). Hyperdominance in the Amazonian tree Flora. Science 342:1243092. doi: 10.1126/science.1243092
Tomlinson, P. B. (2006). The uniqueness of palms. Botan. J. Linnean Soc. 151, 5–14. doi: 10.1111/j.1095-8339.2006.00520.x
Van der Hoek, Y. (2017). The potential of protected areas to halt deforestation in Ecuador. Environ. Conserv. 44, 124–130. doi: 10.1017/S037689291700011X
Vandermeer, J., Perfecto, I., and Schellhorn, N. (2010). Propagating sinks, ephemeral sources and percolating mosaics: conservation in landscapes. Landscape Ecol. 25, 509–518. doi: 10.1007/s10980-010-9449-2
Villar, N., Paz, C., Zipparro, V., Nazareth, S., Bulascoschi, L., Bakker, E. S., et al. (2020). Frugivory underpins the nitrogen cycle. Funct. Ecol. 35, 357–368. doi: 10.1111/1365-2435.13707
Violle, C., Navas, M. L., Vile, D., Kazakou, E., Fortunel, C., Hummel, I., et al. (2007). Let the concept of trait be functional! Oikos 116, 882–892. doi: 10.1111/j.0030-1299.2007.15559.x
Wang, Y. H., and Augspurger, C. (2004). Dwarf palms and cyclanths strongly reduce neotropical seedling recruitment. Oikos 107, 619–633. doi: 10.1111/j.0030-1299.2004.13328.x
Westoby, M. (1998). A leaf-height-seed (LHS) plant ecology strategy scheme. Plant Soil 199, 213–227. doi: 10.1023/A:1004327224729
Wright, J. S. (2010). The future of tropical forests. Ann. N. Y. Acad. Sci. 1195, 1–27. doi: 10.1111/j.1749-6632.2010.05455.x
Zambrano, J., Garzon-Lopez, C. X., Yeager, L., Fortunel, C., Cordeiro, N. J., and Beckman, N. G. (2019). The effects of habitat loss and fragmentation on plant functional traits and functional diversity: what do we know so far? Oecologia 191, 505–518. doi: 10.1007/s00442-019-04505-x
Keywords: Arecaceae, functional traits, community composition, abundance, diversity, Chocó, species richness, secondary forest
Citation: Lueder S, Narasimhan K, Olivo J, Cabrera D, Jurado JG, Greenstein L and Karubian J (2022) Functional Traits, Species Diversity and Species Composition of a Neotropical Palm Community Vary in Relation to Forest Age. Front. Ecol. Evol. 10:678125. doi: 10.3389/fevo.2022.678125
Received: 08 March 2021; Accepted: 24 March 2022;
Published: 27 April 2022.
Edited by:
Kelly M Andersen, Nanyang Technological University, SingaporeReviewed by:
Daisy Cárate Tandalla, University of Göttingen, GermanyGerardo Avalos, University of Costa Rica, Costa Rica
Copyright © 2022 Lueder, Narasimhan, Olivo, Cabrera, Jurado, Greenstein and Karubian. This is an open-access article distributed under the terms of the Creative Commons Attribution License (CC BY). The use, distribution or reproduction in other forums is permitted, provided the original author(s) and the copyright owner(s) are credited and that the original publication in this journal is cited, in accordance with accepted academic practice. No use, distribution or reproduction is permitted which does not comply with these terms.
*Correspondence: Jordan Karubian, amtAdHVsYW5lLmVkdQ==