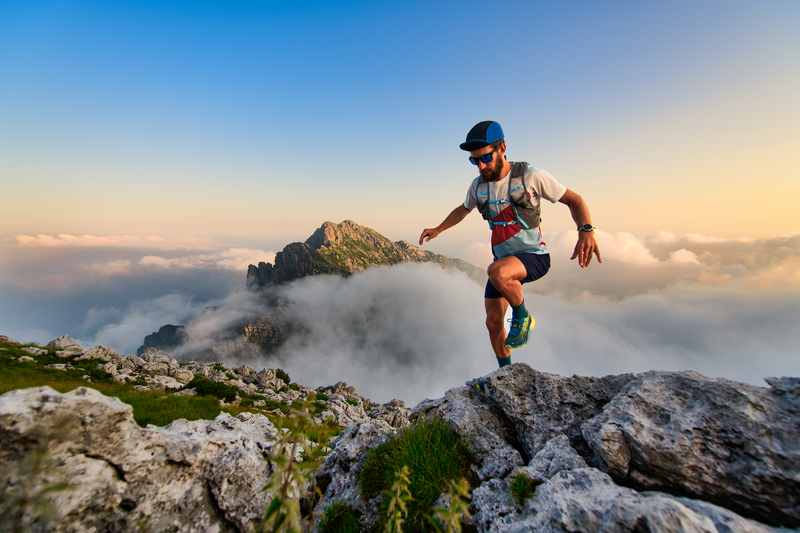
95% of researchers rate our articles as excellent or good
Learn more about the work of our research integrity team to safeguard the quality of each article we publish.
Find out more
ORIGINAL RESEARCH article
Front. Ecol. Evol. , 09 January 2023
Sec. Conservation and Restoration Ecology
Volume 10 - 2022 | https://doi.org/10.3389/fevo.2022.1104369
This article is part of the Research Topic Key Determinants of Biodiversity, Ecosystem Functioning and Restoration in Climate Change Sensitive Ecosystems View all 24 articles
Introduction: Soil organic carbon (SOC) accumulation changed with forest succession and hence impacted the SOC storage. However, the variation and underlying mechanisms about SOC during tropical forest succession are not fully understood.
Methods: Soil samples at four depths (0–10 cm, 10–20 cm, 20–40 cm and 40–60 cm), litter, and roots of 0–10 cm and 10–20 cm were collected from three forest succession stages (plantation forest, secondary forest, and old– growth forest) in the Jianfengling (JFL) National Nature Reserve in Hainan Island, China. The SOC, soil enzyme activities, physiochemical properties, the biomass of litter and roots were analyzed.
Results: Results showed that forest succession significantly increased SOC at 0–10 cm and 10–20 cm depth (from 23.00 g/kg to 33.70 g/kg and from 14.46 g/kg to 22.55 g/kg, respectively) but not at a deeper depth (20–60 cm). SOC content of the three forest succession stages decreased with increasing soil depth and bulk density (BD). With forest succession from plantation to secondary and old–growth forest, the soil pH at 0–10 cm and 10–20 cm depth decreased from 5.08 to 4.10 and from 5.52 to 4.64, respectively. Structural equation model (SEM) results showed that the SOC at depths of 0–20 cm increased with total root biomass but decreased with increasing soil pH value. The direct positive effect of soil TP on SOC was greater than the indirect negative effect of decomposition of SOC by soil acid phosphatase (AP).
Discussion: To sum up, the study highlighted there was soil P– limited in tropical forests of JFL, and the increase in TP and total root biomass inputs were main factors favoring SOC sequestration during the tropical forest succession. In addition, soil acidification is of great importance for SOC accumulation in tropical forests for forest succession in the future. Therefore, forest succession improved SOC accumulation, TP and roots contributed to soil C sequestration.
Forest soil carbon is the main component of the terrestrial ecosystem carbon pool. Small variations of this component will affect atmospheric carbon concentrations and future global climate changes (Fang et al., 2015). Tropical forests cover only 6% of the world’s land area but contain about 40% of the terrestrial ecosystem carbon (Ren et al., 2014), with old–growth forests still accumulating carbon stably in soil (Zhou et al., 2006). However, tropical primary forest areas have been decreasing under the global climate change and human disturbances over the last century (Ma et al., 2022). Studies found a reduction in soil organic carbon (SOC) during the conversion of tropical primary forests to other land–use types (Zhou et al., 2018). Conversely, when forest vegetation is restored, the SOC will increase with positive forest succession (Deng et al., 2013). Meanwhile, long–term observational data show that soil in the northern hemisphere and temperate forests has always acted as a carbon sink, but there are relatively few studies on its dynamic change in tropical forests (Zhu et al., 2020). Therefore, studying the variation characteristics of SOC during tropical forest succession is important to understand the carbon sequestration capacity of forest soil.
SOC accumulation during forest succession is largely influenced by biotic and abiotic factors (Smith, 2008; Hu and Lan, 2020). In terms of abiotic factors, TN and TP are two important factors that influence SOC by regulating SOC input and output, because nitrogen and phosphorus are critical elements for plant growth (Schleuss et al., 2019). The high N/P ratios will increase nitrogen uptake by plants, promote plant growth and increase SOC input (Peng et al., 2019). In terms of biotic factors, the accumulation of SOC depends on the balance of the input of exogenous SOC from litter and roots and the output of SOC through microbial consumption. It is important source of soil nutrients from litter decomposition that replenished by forest ecosystems (Rubino et al., 2010). Huang et al. (2011) reported that the increase of litter decomposition with forward succession help accumulate SOC. The increase of roots and their secretions can also increase SOC in the late afforestation restoration stage (Hu et al., 2016). In addition, soil microbes, one of the main decomposers of organic carbon, act on the decomposition of soil organic matter by regulating enzymatic activity. Soil microbes secreted extracellular enzymes to decompose complex organic matter for obtaining carbon and other nutrients, which also have important impacts on the sequestration of forest SOC (Cui et al., 2019). To reveal the biotic, abiotic factors and their interact effect on SOC accumulation during tropical forest succession is of importance for guiding tropical forest management and SOC sequestration.
The tropical forest in Hainan Island accounts for 31.4% of the total tropical forest area in China, which plays a key role in ecological environment construction and it’s largely affected by global climate changes (Li et al., 2019). The Jianfengling (JFL) tropical forest remains one of the few well–preserved tropical primary forests in China (Guo et al., 2015). The slash–and–burn and traditional agricultural methods used over the last century are what have caused severe damage and the subsequent restoration of tropical forests, and form an ideal area to study the variations and influencing factors of SOC during the tropical forest succession. In this study, three forest succession stages, plantation forest, secondary forest, and old–growth forest in JFL, which belong to early, middle and late succession stages were selected. The SOC and other soil physicochemical properties, soil enzyme activities, litter and roots biomass were investigated in different forest succession stages. The objectives were to: (1) explore the variations of SOC and enzyme activities at different succession stages and soil layers; (2) find the effects of soil environmental factors, litter and roots on SOC during tropical forest succession. We proposed two hypotheses: (1) SOC increases with forest succession from plantation forest to secondary forest and old–growth forest; (2) the increase of litter and root biomass is beneficial for SOC accumulation. The study results are expected to provide theoretical reference for soil carbon management in tropical forest.
The study site, the JFL National Nature Reserve, is located in the southwestern part of Hainan Island, China (18°23′–18°50″N 108°36′–109°05′E). The area has a typical tropical monsoon climate with a wet season from May to October and a dry season from November to April in the following year (Jiang et al., 2017). The mean annual temperature ranges from 25.2°C in coastal tablelands to 19.7°C in mountainous rainforest areas (Xu et al., 2015). The mean annual precipitation ranges from 1,500 to 2,600 mm, with a maximum of 3,500 mm, 80%–90% of which falls in the wet season. The soil types are mainly montane lateritic red or yellow earth according to the World Reference Base for soil resources, and granite is the main soil parent material (Yang et al., 2018).
Our study site mainly includes three forest succession types: old–growth forest (OF), secondary forest (SF), and plantation forest (PF). Three 20 m × 20 m plots were established in each forest. In total, there were nine sample plots. We used the space–for–time substitution method, which has been commonly used for similar soil changes over time under consistent climatic conditions (Walker et al., 2010). The OF is natural mature forest without human disturbance for a long time, while SF and PF are the natural regeneration forest which developed on the OF after different degrees of human disturbance. These forests have similar geographical and climatic conditions with uniform temperature and precipitation. Cryptocarya chinensis, Gironniera subaequalis, Mallotus Hookerianus (Seem.) Muell. Arg, and Nephelium topengii (Merr.) H. S. Lo are the dominant species in OF, which maintains a high biodiversity (Fang et al., 2004). The SF naturally regenerated following selective logging of OF in the 1960s and mainly consists of Castanopsis fissa, Castanopsis tonkinensis, Sapium discolor, Syzygium tephrodes, and Schefflera octophylla (Xu et al., 2009). Cunninghami alanceolata (Lamb.) Hook. is the dominant species in the PF, and it was established after being felled in the 1980s. The basic sample sites information is shown in Table 1.
Table 1. Location and basic characteristics of sampling plots in different succession stages in the Jianfengling National Nature Reserve.
In September 2021, we collected litter, root and soil samples along the forest succession stages from PF, SF, to OF. At each forest stage, three sampling plots were selected. At each plot, we divided into four subplots (10 m × 10 m), and litter was collected randomly with three replicates by litter traps (50 cm × 50 cm). A total of 27 above–ground litter samples were obtained. At each sampling plot, root samples at 0–10 cm and 10–20 cm soil depths were collected using an auger (inner diameter, 9.5 cm), and then fully soaked in water for 5 h in the laboratory, and washed with water for 20 mesh to carry out root picking. Roots were divided into two types according to root diameter: they were defined as fine roots and thick roots with root diameter ≤ 2 mm and root diameter > 2 mm, respectively. Root biomass was determined after being oven–dried at 65°C for 24 h.
The soil samples were collected at four depths (0–10 cm, 10–20 cm, 20–40 cm and 40–60 cm) using a stainless soil corer at each plot. A composite sample consisted of five soil cores, and then divided into two subsamples. In total, 72 soil subsamples were collected and transported to the laboratory with ice bags. After removing animal and plant residues, roots and other visible items, soil samples were passed through a 2 mm mesh. Finally, each sample was separated into two parts to measure (1) the basic physical and chemical properties after being air–dried, and (2) the soil enzyme activity within 1 week with soils stored at 4°C. The soil bulk density (BD) sample was collected using a cutting ring (volume of 100 cm3). We used the method of digging the soil profile, and then collected three soil samples per soil layer using cutting ring, and measured the ratio of the weight of dry soils to the volume of each soil core.
After drying at 105°C for 12 h, soil water content (SWC) was measured by mass loss. The soil pH was measured by the potentiometric method (the soil: water ratio = 1:2.5). SOC was determined by the K2Cr2O7–H2SO4 oxidation method (Bao, 2000). Soil total nitrogen (TN) and total phosphorus (TP) were extracted by the semimicro kelvin method and determined by Continuous Flow Analyzer (PROXIMA 1022/1/1, ALLIANCE Instruments, France; Zhang et al., 2019). Soil ammonium nitrogen (NH4+–N) and nitrate nitrogen (NO3−–N) contents were determined by extracting fresh soil with 2 M KCL and analyzing it using the Continuous Flow Analyzer (SKALAR San++, SKALAR Co., Netherlands). The soil available phosphorus (SAP) was determined by ammonium chloride hydrochloric acid extraction. The activities of acid phosphatase (AP), β–1,4–glucosidase (BG), β–1,4–N–acetyl–glucosaminidase (NAG) and leucine aminopeptidase (LAP) were determined by 96–well microplates (German et al., 2011; Bach et al., 2013).
Microbial nutrient limitations were determined by calculating the vector length, and angle A with enzyme activity stoichiometry vector analysis using the following formulae (Moorhead et al., 2013):
The larger the vector L, the stronger the carbon constraint. Angles A < 45° and >45° indicate that the microbes are limited by nitrogen and phosphorus, respectively.
To identify the differences in SOC, TN, TP, C:N, C:P and N:P ratios, soil enzyme activities and other soil properties in different forest succession stages and different soil layers, one–way ANOVA followed by the Least Significant Difference (LSD) test was applied (p < 0.05). On the basis of the homogeneity test of variance, two–way ANOVA followed by the LSD test were used to investigate the effects of soil depth, forest succession stages, and their interactions on SOC, TN, and TP and their stoichiometric ratios. Pearson correlation analysis was used to analyze the correlation between the soils’ properties and enzyme activities. The structural equation model (SEM), which combines path analysis and factor analysis, has been applied as causal inference in ecology, mainly using maximum–likelihood (Shipley, 2001). Before starting, to avoid multicollinearity, we used the variance inflation factor (VIF) threshold to eliminate those variables that were strongly correlated (Kock, 2015). Then, we established an a-prior model (Supplementary Figure S4) based on the known effects and relationships among the drivers of SOC. The SEM analyses were performed using R software (4.2.0) lavaan package. SPSS 19.0 (IBM SPSS Predictive Analytics Community, United States) software, Origin 2019b (Origin Lab Corp. United States), and R (R 4.2.0) were used for all statistical analyses and figures in the study.
SOC was significantly affected by soil depth, forest succession stages, and their interactions (p < 0.05; Table 2). The SOC in the four soil layers increased with forest succession from PF to SF and OF, with the highest SOC of 33.70 g/kg at the depth of 0–10 cm in OF, which was significantly higher than that of in SF (25.48 g/kg) and PF (23.00 g/kg; p < 0.05). The SOC at the depth of 10–20 cm in OF (22.55 g/kg) was significantly higher than that of in PF (14.46 g/kg). In the vertical direction, SOC at the depth of 0–40 cm significantly decreased at each forest stage, but tend to be stable below 40 cm (Figure 1).
Table 2. F-values for two–way ANOVAs on the effects of soil depth, forest succession stages, their interaction on the concentrations of soil organic carbon (SOC), total soil nitrogen (TN), total soil phosphorus (TP), C:N, C:P, and N:P ratios.
Figure 1. Variations of SOC in different forest succession stages in the Jianfengling National Nature Reserve. SOC, soil organic carbon; PF, plantation forest; SF, secondary forest; OF, old-growth forest. Different lowercase letters indicate significant differences between different soil layers at the same stage. Different capital letters indicate significant differences in the same soil layer at different forest succession stages (p < 0.05).
As shown in Table 3, the soil pH at each soil layer gradually decreased with forest succession, and the soil pH at a depth of 0–10 cm in OF was significantly lower than that in SF and PF, indicating that surface soil acidification was aggravated at the later stage of forest succession. In the vertical direction, the soil pH increased with soil depth, indicating that the acidification degree decreased with increasing soil depth. The SWC in SF was significantly lower than that in PF and OF in lower soils (0–10 cm and 10–20 cm), and the SWC in SF was significantly lower than that in OF in deeper soils (20–40 cm and 40–60 cm).
Table 3. The soil properties at different forest succession stages in the Jianfengling National Nature Reserve (n = 6).
The TN, TP and their stoichiometric ratios were significantly affected by soil depth, forest succession stages, and their interactions (Table 2). The TN in OF was significantly higher than that in SF and PF at depths of 0–10 cm and 10–20 cm (Table 3), indicating that TN increased with forest succession processes. The TN was positively linked with NH4+–N and NO3−–N (p < 0.01), and it was positively correlated with TP and BG (p < 0.05; Figure 2).
Figure 2. The correlation of different soil properties in different forest succession stages in the Jianfengling National Nature Reserve. SWC, soil water content; BD, bulk density; SOC, soil organic carbon; TN, total nitrogen; TP, total phosphorus; NH4+–N, ammonium nitrogen; NO3—N, nitrate nitrogen; SAP, soil available phosphorus; Roots, total root biomass; Litter, litter biomass; AP, acid phosphatase; BG, β–1,4–glucosidase; NAG, β–1,4–N–acetyl–glucosaminidase; LAP, leucine aminopeptidase. Significance levels: ***p < 0.001; **p < 0.01; *p < 0.05.
The TP in the four soil layers increased with forest succession and was obviously lower in PF than in SF and OF. The C:N ratio increased with forest succession but did not change significantly in different soil depths. The ratios of C:P and N:P initially decreased and then increased with forest succession, with the N:P ratio in PF significantly higher than that in the latter two stages (Table 3).
The NH4+–N in all four soil layers did not change significantly with forest succession, while the NO3−–N increased significantly. The SAP increased first and then decreased with forest succession, where the SAP in SF was obviously higher than that in PF and OF (Table 3).
The activity of BG first decreased and then increased with forest succession (Figure 3A). The activity of BG decreased with soil depth. The NAG and LAP activities decreased first and then increased (Figures 3B,C). The AP activity showed a general decreasing trend with forest succession and was obviously lower in SF than in PF and OF at a depth of 0–10 cm. In the vertical direction, there was no significant difference for AP in the different soil layer in PF and SF (Figure 3D). BG, NAG, and LAP all had significant correlations with AP activity, while the NAG activity was positively correlated with LAP (Figure 2).
Figure 3. Variations of soil enzyme activities in different forest restoration stages in the Jianfengling National Nature Reserve. (A) β–1,4–glucosidase (BG), (B) β–1,4–N–acetyl–glucosaminidase (NAG), (C) leucine aminopeptidase (LAP), and (D) acid phosphatase (AP) activities. PF, plantation forest; SF, secondary forest; OF, old-growth forest. Different lowercase letters indicate significant differences between different soil layers at the same stage. Different capital letters indicate significant differences in the same soil layer at different forest succession stages (p < 0.05).
With the progression of forest succession, the amount of litter per unit area increased first and then decreased, and the litter amount in SF was significantly higher than that of in PF and OF (Figure 4). However, the SOC, TN, and TP contents of litter did not change significantly with forest succession (Supplementary Figure S1).
Figure 4. Litter biomass in different forest succession stages in the Jianfengling National Nature Reserve. PF, plantation forest; SF, secondary forest; OF, old-growth forest. Different lowercase letters indicate significant differences at different forest succession stages (p < 0.05).
From the PF to SF and OF, the total root biomass of different soil layers increased significantly (p < 0.05). Especially, the total root biomass of the 10–20 cm soil layer was significantly higher than that of the 0–10 cm soil layer (Figure 5). In different forest succession stages, the SOC, TN, and TP contents of fine and thick roots showed an increasing trend, but not all of them were significant (Supplementary Figure S2).
Figure 5. Total root biomass in different forest succession stages in the Jianfengling National Nature Reserve. PF, plantation forest; SF, secondary forest; OF, old-growth forest. Different lowercase letters indicate significant differences between different soil layers at the same stage. Different capital letters indicate significant differences in the same soil layer at different forest succession stages (p < 0.05).
The SOC substantially negatively correlated with BD and pH, while it obviously positively correlated with TN, TP, NH4+–N and NO3−–N (Figure 2). The SEM showed that the biological and environmental factors selected in this study explained 79.9% of the variations in SOC (Figure 6A). The AP, total root biomass and pH value were the three main important factors influencing SOC (Figure 6B). The AP and TP had a direct positive effect on SOC (p < 0.01), while soil TP indirectly affected SOC through its direct effect on AP activity. Total root biomass could affect SOC through its direct effect (p < 0.05). In addition, pH had an indirect effect on SOC by mainly affecting litter biomass and enzyme activities.
Figure 6. Structural equation model (SEM) of the effect of soil properties on SOC (0–20 cm) in the Jianfengling National Nature Reserve. The model was fitted by maximum likelihood estimation. In panel (A), red arrows represent significant positive effects and blue arrows represent significant negative effects (p < 0.05), and gray dotted line indicates non–significant relationship (p > 0.05). The numbers on the line represent normalized path coefficients (*p < 0.05, **p < 0.01, ***p < 0.001). In panel (B), the total effect is the sum of direct and indirect effects. χ2, global similarity of matrix; df, degree of freedom; GFI, goodness of fit index; CFI, comparative fit index; RMSEA, approximate root mean square error; R2, variance ratio of priming effect explained by variables; SOC, soil organic carbon; TP, total phosphorus; Roots, total root biomass; Litter, litter biomass; AP, acid phosphatase; BG, β–1,4–glucosidase.
In this study, SOC showed an increasing trend from PF to SF and OF, which supports the first hypothesis. The SOC increased with tropical forest succession, which is similar to the results of previous studies in the subtropical Dinghushan Region (Shao et al., 2017), temperate Loess Plateau (Deng et al., 2013), and subtropical Dashanchong Forest Park (Xu et al., 2018). Litter and roots are the two main resources of carbon input by plants, and the changes in litter and plant roots caused by forest succession affect soil carbon cycling (Guo et al., 2005). Generally, forest succession tends to improve the soil environment, enhance soil microbial activity, improve soil fertility, and increase the input of litter and root biomass (Li et al., 2007; Hu et al., 2016). However, there were no significant differences in litter quantity and quality between different forest stages in the results of this study (Figure 4; Supplementary Figure S1), and further, we found that total root biomass had a direct and significant positive effect on SOC, while litter had no direct effect on SOC (Figure 6A). Those results indicated that the increase in the total root biomass at a later stage of forest succession was more conducive to soil carbon sequestration, which was also consistent with the results in the restoration of tropical forests in India (Lalnunzira et al., 2019). In general, fine roots contribute more to SOC because they have a large surface area and fast turnover rates, and they are the direct way of through which plant photosynthesis transports nutrients to the soil (Zhang et al., 2021). However, Sun et al. (2018) conducted a 6-year experiment on fine root decomposition and found that the decomposition rate of fine roots was slower than that of thick roots, so the contribution of fine roots and thick roots to SOC is still controversial. The results of our study showed that fine root biomass or thick root biomass alone had no significant effect on SOC, while total root biomass had a direct and significant effect on SOC (Figure 6A), which partially supported the second hypothesis. Thus, the role of roots on SOC in tropical forests still needs more attention.
In this study, from PF to OF, the SOC in top soils (0–10 cm and 10–20 cm) increased more significantly than that in deeper soils (20–40 cm and 40–60 cm; Figure 1), which was consistent with the findings of Yang et al. (2019). The physiochemical properties of top soils and deep soils were significantly different, thus, the regulate factors and mechanism of SOC varied across soil layers (Fontaine et al., 2007; Mobley et al., 2015). Generally, surface SOC accumulation is the result of interactions between environmental factor–regulated abiotic processes and microbe–regulated biotic processes (Hooper et al., 2000). Because the surface soil contains a large amount of vegetation litter, and sufficient water and air in the surface are also conducive to soil microbial activities (Zhang et al., 2021). The SOC changed significantly during forest succession in this study at the depths of 0–10 cm and 10–20 cm (Figure 1). This can be attributed to the increase in organic materials (litter and roots), weathering and microbial decomposition reducing along the forest succession (Smith, 2008). For deep soils (20–40 cm and 40–60 cm), the input of SOC is mainly from the leaching of surface SOC and a few roots. Since the soil clay proportions were higher in the study area (Jiang et al., 2021), there was less leaching of surface SOC to the deep soil. In addition, this study found a significant negative correlation between bulk density (BD) and SOC (Figure 2), which indicates that the high soil BD is also not conducive for the leaching of surface SOC to deep soil layers. A previous study also found that the low SOC in deep soil is related to the high BD in the tropical forests of Hainan Island (Yang et al., 2016).
The results of SEM suggest that AP had a direct and positive effect on SOC of the soil layer 0–20 cm (Figure 6A). The AP decreased first and then increased with forest succession, helping in the latter stage of carbon sequestration (Figure 2). Soil extracellular enzymes are associated with soil nutrient utilization, AP can change with the demand phosphorus by soil microbes (Sinsabaugh and Shah, 2012). According to the microbial nutrient allocation theory, microbes will preferentially allocate resources to secrete enzymes that mine the most limited nutrients (Sinsabaugh and Moorhead, 1994; Xiao et al., 2018). In our study, vector analysis of soil extracellular enzymes was used to assess the nutrient limitation of soil microbes (Moorhead et al., 2013). Our results showed that the vector angle of extracellular enzymes was always >45° (Supplementary Figure S3), indicating that soil microbial biomass is limited by soil phosphorus availability (DeForest and Moorhead, 2020). The soil available phosphorus contents and nutrient ecological stoichiometry ratio also show that the tropical forest soil was severely restricted by phosphorus (Table 3), which is consistent with the phosphorus limitation of soil microbe. The soil TP (0.37 g/kg) was lower than the average TP of Chinese soil (0.56 g/kg) and subtropical soil (0.52 g/kg; Han et al., 2005), and the average soil available phosphorus (SAP) was only 1.57 mg/kg. Meanwhile, the soil N:P ratio (as the index of soil to plant nutrient status) is limited by nitrogen when the N:P ratio is <14, and phosphorus when the N:P ratio is >16 (Peng et al., 2019). In our study, this ratio was almost always >16, so it was always limited by phosphorus (Table 3). Previous studies have also shown that tropical forests with high soil acidification tend to exhibit a phosphorus constraint (Waring et al., 2014; Xu et al., 2018). Generally, the change in soil phosphorus limitation will lead to a change in microbial limitation, which affects SOC accumulation (Wang et al., 2022). Hence, soil phosphorus limitation will lead to high AP activity (Wang et al., 2020). A corresponding increase in SOC decomposed by AP. That is, AP and SOC had a positive direct effect relationship, which is consistent with the results of SEM (Figure 6).
In addition, TP had a positive direct effect on SOC (Figure 6A), because soil nutrients and microorganisms were severely restricted by phosphorus in our study area. The increase in soil TP was conducive to the acquisition of phosphorus by plants, which can improve plant productivity and thus increase the input sources of SOC (Schleuss et al., 2019). In conclusion, the negative effect of AP decomposition caused by P restriction was traded off by the direct positive effect of TP on SOC, which was conducive to C sequestration.
Our results suggested that the pH value has no direct effect on SOC, but it has an indirect effect on SOC by inhibiting AP activity (Figure 6A). Previous studies also reported that soil acidification (low pH) has the potential to delay the decomposition of SOM in acid soils by changing the composition of microorganisms, thus favoring SOC accumulation (Lu et al., 2021). In our study, the pH values at a depth of 0–20 cm ranged from 4.01 to 5.52, and the pH values gradually decreased with forest succession from PF to SF and OF (Table 3), indicating that soil acidification was aggregated. Zhu et al. (2021) also found that forest succession can decrease the soil acidity, especially in topsoil. Lower soil pH inhibited the activities of soil microbial activities (Wang et al., 2018a), as the most suitable pH for soil microbe is generally in the neutral range of 6.5–7.5 (San Le et al., 2022). Studies have shown that pH value was negatively correlated with hydrolase activity, which was consistent with the negative correlation between BG, AP and pH in our study (Ghiloufi et al., 2019). Thus, increased soil acidification would be beneficial for SOC accumulation by affecting AP activity under soil nutrient limited by phosphorus (Figure 6). Under the background of future N deposition, soil acidification accelerates soil carbon sequestration in tropical forests. This has important implications to better understand and predict the effects of P limitation on soil acidification in the global tropical terrestrial ecosystems.
Forest succession increased SOC significantly at the depth of 0–20 cm from PF to SF and OF, while there were no significant changes of SOC in deeper soils (20–40 cm and 40–60 cm). Microbial nutrient limitation and N:P ratio reflected that the soil was always limited by phosphorus in the tropical forest of JFL. Except for the microbe–regulated biotic processes, the TP and total root biomass were important factors affecting SOC at depths of 0–20 cm. The increase of total root biomass input rather than litter biomass contributed to SOC sequestration during the tropical forest succession. In addition, the soil acidification caused by forest succession and nitrogen deposition is beneficial to SOC sequestration in tropical forests in the future. This study provides new insights into SOC accumulation during the tropical forest succession.
The raw data supporting the conclusions of this article will be made available by the authors, without undue reservation.
WLi, QY, and YJ: design the experiments, funding, and reviewing and editing. GX: do the experiments, data analysis, writing–original draft, and writing–reviewing and editing. XW and SM: do the experiments together. WX: put forward relevant improvement suggestions. HY, EH, XH, and WLo: reviewing and editing. All authors contributed to the article and approved the submitted version.
This research is supported by the National Key Research and Development Program of China (no. 2021YFD2200403-04), the National Natural Science Foundation of China (no. 32160291 and 41663010), and the Natural Science Foundation of Hainan Province (no. 2019RC012). The corresponding authors thank the China Scholarship Council for the financial support.
We would like to thank Jianfengling National Key Field Research Station for Tropical Forest Ecosystem.
The authors declare that the research was conducted in the absence of any commercial or financial relationships that could be construed as a potential conflict of interest.
All claims expressed in this article are solely those of the authors and do not necessarily represent those of their affiliated organizations, or those of the publisher, the editors and the reviewers. Any product that may be evaluated in this article, or claim that may be made by its manufacturer, is not guaranteed or endorsed by the publisher.
The Supplementary material for this article can be found online at: https://www.frontiersin.org/articles/10.3389/fevo.2022.1104369/full#supplementary-material
Bach, C. E., Warnock, D. D., Van Horn, D. J., Weintraub, M. N., Sinsabaugh, R. L., Allison, S. D., et al. (2013). Measuring phenol oxidase and peroxidase activities with pyrogallol, L–DOPA, and ABTS: effect of assay conditions and soil type. Soil Biol. Biochem. 67, 183–191. doi: 10.1016/j.soilbio.2013.08.022
Cui, Y. X., Fang, L. C., Guo, X. B., Wang, X., Wang, Y. Q., Zhang, Y. J., et al. (2019). Responses of soil bacterial communities, enzyme activities, and nutrients to agricultural-to-natural ecosystem conversion in the loess plateau, China. J. Soils Sediments 19, 1427–1440. doi: 10.1007/s11368-018-2110-4
DeForest, J. L., and Moorhead, D. L. (2020). Effects of elevated pH and phosphorus fertilizer on soil C, N and P enzyme stoichiometry in an acidic mixed mesophytic deciduous forest. Soil Biol. Biochem. 150:107996. doi: 10.1016/j.soilbio.2020.107996
Deng, L., Wang, K. B., Chen, M. L., Shangguan, Z. P., and Sweeney, S. (2013). Soil organic carbon storage capacity positively related to forest succession on the loess plateau, China. Catena 110, 1–7. doi: 10.1016/j.catena.2013.06.016
Fang, J. Y., Li, Y. D., Zhu, B., Liu, G. H., and Zhou, G. Y. (2004). Community structure, species diversity and status of montane rainforest in Jianfengling, Hainan Island. Biodivers. Sci. 01, 29–43. doi: 10.17520/biods.2004005
Fang, J. Y., Yu, G. R., Ren, X. B., Liu, G. H., and Zhao, X. Q. (2015). Research progress of ecosystem carbon sequestration task group of the strategic priority science and technology project of Chinese Academy of Sciences "carbon budget certification and related issues in response to climate change". Bull. Chin. Acad. Sci. 30:10. doi: 10.16418/j.issn.1000–3045
Fontaine, S., Barot, S., Barre, P., Bdioui, N., Mary, B., and Rumpel, C. (2007). Stability of organic carbon in deep soil layers controlled by fresh carbon supply. Nature 450, 277–280. doi: 10.1038/nature06275
German, D. P., Weintraub, M. N., Grandy, A. S., Lauber, C. L., Rinkes, Z. L., and Allison, S. D. (2011). Optimization of hydrolytic and oxidative enzyme methods for ecosystem studies. Soil Biol. Biochem. 43, 1387–1397. doi: 10.1016/j.soilbio.2011.03.017
Ghiloufi, W., Seo, J., Kim, J., Chaieb, M., and Kang, H. (2019). Effects of biological soil crusts on enzyme activities and microbial community in soils of an arid ecosystem. Microb. Eco. 77, 201–216. doi: 10.1007/s00248–018–1219–8
Guo, L. B., Halliday, M. J., Siakimotu, S. J. M., and Gifford, R. M. (2005). Fine root production and litter input: its effects on soil carbon. Plant Soil 272, 1–10. doi: 10.1007/s11104-004-3611-z
Guo, X. W., Luo, T. S., and Li, Y. D. (2015). Soil organic carbon density and influencing factors of primary tropical forests in Hainan Island. Acta Ecol. Sin. 35, 56–61. doi: 10.5846/stxb201404180761
Han, W., Fang, J., Guo, D., and Zhang, Y. (2005). Leaf nitrogen and phosphorus stoichiometry across 753 terrestrial plant species in China. New Phytol. 168, 377–385. doi: 10.1111/j.1469-8137.2005.01530.x
Hooper, D. U., Bignell, D. E., Brown, V. K., Lijbert, B., Mark, D. J., Wall, D. H., et al. (2000). Interactions between aboveground and belowground biodiversity in terrestrial ecosystems: patterns, mechanisms, and feedbacks. Bioscience 12, 1049–1061. doi: 10.1641/0006–3568(2000)050
Hu, N., and Lan, J. C. (2020). Impact of vegetation restoration on soil organic carbon stocks and aggregates in a karst rocky desertification area in Southwest China. J. Soils Sediments 20, 1264–1275. doi: 10.1007/s11368-019-02532-y
Hu, Y. L., Zeng, D. H., Ma, X. Q., and Chang, S. X. (2016). Root rather than leaf litter input drives soil carbon sequestration after afforestation on a marginal cropland. Forest Ecol. Manag. 362, 38–45. doi: 10.1016/j.foreco.2015.11.048
Huang, Y. H., Li, Y. L., Xiao, Y., Wenigmann, K. O., Zhou, G. Y., Zhang, D. Q., et al. (2011). Controls of litter quality on the carbon sink in soils through partitioning the products of decomposing litter in a forest succession series in South China. For. Ecol. Manag. 261, 1170–1177. doi: 10.1016/j.foreco.2010.12.030
Jiang, L., Ma, S. H., Zhou, Z., Zheng, T. L., Jiang, X. X., Cai, Q., et al. (2017). Soil respiration and its partitioning in different components in tropical primary and secondary mountain rain forests in Hainan Island, China. J. Plant Ecol. 10, rtw080–rtw799. doi: 10.1093/jpe/rtw080
Jiang, Y. M., Yang, H., Yang, Q., Liu, W. J., Li, Z. L., Mao, W., et al. (2021). The stability of soil organic carbon across topographies in a tropical rainforest. Peerj 9:e12057. doi: 10.7717/peerj.12057
Kock, N. (2015). Common method bias in PLS-SEM: a full collinearity assessment approach. Int. J. e-Collab. 11, 1–10. doi: 10.4018/ijec.2015100101
Lalnunzira, C., Brearley, F. Q., and Tripathi, S. K. (2019). Root growth dynamics during recovery of tropical mountain forest in north–East India. J. Mt Sci.–Engl 16, 2335–2347. doi: 10.1007/s11629-018-5303-9
Li, X. R., Kong, D. S., Tan, H. J., and Wang, X. P. (2007). Changes in soil and vegetation following stabilisation of dunes in the southeastern fringe of the Tengger Desert, China. Plant Soil 300, 221–231. doi: 10.1007/s11104-007-9407-1
Li, Q., Wang, B., and Deng, Y. (2019). Relationship between gap spatial pattern and understory plant diversity in tropical rain forest. Plant Ecol. 27, 273–285. doi: 10.17520/biods.2018258
Lu, X. K., Vitousek, P. M., Mao, Q. G., Gilliam, F. S., Luo, Y. Q., Turner, B. L., et al. (2021). Nitrogen deposition accelerates soil carbon sequestration in tropical forests. Proc. Natl. Acad. Sci. U. S. A. 118:e2020790118. doi: 10.1073/pnas.2020790118
Ma, S. H., Zhu, B., Chen, G. P., Ni, X. F., Zhou, L. H., Su, H. J., et al. (2022). Loss of soil microbial residue carbon by converting a tropical forest to tea plantation. Sci. Total Environ. 818:151742. doi: 10.1016/j.scitotenv.2021.151742
Mobley, M. L., Lajtha, K., Kramer, M. G., Bacon, A. R., Heine, P. R., and Richter, D. D. (2015). Surficial gains and subsoil losses of soil carbon and nitrogen during secondary forest development. Glob. Chang. Biol. 21, 986–996. doi: 10.1111/gcb.12715
Moorhead, D. L., Rinkes, Z. L., Sinsabaugh, R. L., and Weintraub, M. N. (2013). Dynamic relationships between microbial biomass, respiration, inorganic nutrients and enzyme activities: informing enzyme–based decomposition models. Front. Microbiol. 4:223. doi: 10.3389/fmicb.2013.00223
Peng, Y. F., Peng, Z. P., Zeng, X. T., and Houx, J. H. (2019). Effects of nitrogen–phosphorus imbalance on plant biomass production: a global perspective. Plant Soil 436, 245–252. doi: 10.1007/s11104–018–03927–5
Ren, H., Li, L., Liu, Q., Wang, X., Li, Y., Hui, D., et al. (2014). Spatial and temporal patterns of carbon storage in Forest ecosystems on Hainan Island, southern China. PLoS One 9:e108163. doi: 10.1371/journal.pone.0108163
Rubino, M., Dungait, J. A. J., Evershed, R. P., Bertolini, T., De Angelis, P., D'Onofrio, A., et al. (2010). Carbon input belowground is the major C flux contributing to leaf litter mass loss: evidences from a C–13 labelled–leaf litter experiment. Soil Biol. Biochem. 42, 1009–1016. doi: 10.1016/j.soilbio.2010.02.018
San Le, V., Herrmann, L., Hudek, L., Nguyen, T. B., Brau, L., and Lesueur, D. (2022). How application of agricultural waste can enhance soil health in soils acidified by tea cultivation: a review. Environ. Chem. Lett. 20, 813–839. doi: 10.1007/s10311–021–01313–9
Schleuss, P. M., Widdig, M., Heintz-Buschart, A., Guhr, A., Martin, S., Kirkman, K., et al. (2019). Stoichiometric controls of soil carbon and nitrogen cycling after long–term nitrogen and phosphorus addition in a Mesic grassland in South Africa. Soil Biol. Biochem. 135, 294–303. doi: 10.1016/j.soilbio.2019.05.018
Shao, S., Zhao, Y., Zhang, W., Hu, G. Q., Xie, H. T., Yan, J. H., et al. (2017). Linkage of microbial residue dynamics with soil organic carbon accumulation during subtropical forest succession. Soil Biol. Biochem. 114, 114–120. doi: 10.1016/j.soilbio.2017.07.007
Shipley, B. (2001). Cause and correlation in biology: A User’s guide to path analysis structural equations and causal inference. UK: Cambridge Univ. Press.
Sinsabaugh, R. L., and Moorhead, D. L. (1994). Resource allocation to extracellular enzyme production: a model for nitrogen and phosphorus control of litter decomposition. Soil Biol. Biochem. 26(10), 1305–1311. doi:, doi: 10.1016/0038-0717(94)90211-9
Sinsabaugh, R. L., and Shah, J. J. (2012). Ecoenzymatic stoichiometry and ecological theory. Annu. Rev. Ecol. Evol. Syst. 43, 313–343. doi: 10.1146/annurev-ecolsys-071112-124414
Smith, P. (2008). Land use change and soil organic carbon dynamics. Nutr. Cycl. Agroecosys. 81, 169–178. doi: 10.1007/s10705-007-9138-y
Sun, T., Hobbie, S. E., Berg, B., Zhang, H. G., Wang, Q. K., Wang, Z. W., et al. (2018). Contrasting dynamics and trait controls in first–order root compared with leaf litter decomposition. Proc. Natl. Acad. Sci. U. S. A. 115, 10392–10397. doi: 10.1073/pnas.1716595115
Walker, L. R., Wardle, D. A., Bardgett, R. D., and Clarkson, B. D. (2010). The use of chronosequences in studies of ecological succession and soil development. J. Ecol. 98, 725–736. doi: 10.1111/j.1365-2745.2010.01664.x
Wang, X. X., Cui, Y. X., Wang, Y. H., Duan, C. J., Niu, Y. A., Sun, R. X., et al. (2022). Ecoenzymatic stoichiometry reveals phosphorus addition alleviates microbial nutrient limitation and promotes soil carbon sequestration in agricultural ecosystems. J. Soils Sediments 22, 536–546. doi: 10.1007/s11368-021-03094-8
Wang, C., Lu, X. K., Mori, K., Mao, Q. G., Zhou, J. K., Zhou, G. Y., et al. (2018a). Responses of soil microbial community to continuous experimental nitrogen additions for 13 years in a nitrogen-rich tropical forest. Soil Biol. Biochem. 121, 103–112. doi: 10.1016/j.soilbio.2018.03.009
Wang, C., Mori, T., Mao, Q. G., Zhou, K. J., Wang, Z. H., Zhang, Y. Q., et al. (2020). Long-term phosphorus addition downregulates microbial investments on enzyme productions in a mature tropical forest. J. Soils Sediments 20, 921–930. doi: 10.1007/s11368-019-02450-z
Waring, B. G., Weintraub, S. R., and Sinsabaugh, R. L. (2014). Ecoenzymatic stoichiometry of microbial nutrient acquisition in tropical soils. Biogeochemistry 117, 101–113. doi: 10.1007/s10533-013-9849-x
Xiao, W., Chen, X., Jing, X., and Zhu, B. (2018). A meta-analysis of soil extracellular enzyme activities in response to global change. Soil Biol. Biochem. 123, 21–32. doi: 10.1016/j.soilbio.2018.05.001
Xu, H., Li, Y., Lin, M., Wu, J., Luo, T., Zhou, Z., et al. (2015). Community characteristics of a 60 ha dynamics plot in the tropical montane rain forest in Jianfengling, Hainan Island. Biodivers. Sci. 23, 192–201. doi: 10.17520/biods.2014157
Xu, H., Li, Y., Luo, T., Lin, M., Chen, D., Mo, J., et al. (2009). Community structure characteristics of tropical montane rain forests with different regeneration types in Jianfengling. Scientia Silvae Sinicae. 45, 14–20. doi: 10.1007/978-1-4020-9623-5_5
Xu, C. H., Xiang, W. H., Gou, M. M., Chen, L., Lei, P. F., Fang, X., et al. (2018). Effects of Forest restoration on soil carbon, nitrogen, phosphorus, and their stoichiometry in Hunan, southern China. Sustainability. 10:1874. doi: 10.3390/su10061874
Yang, H., Li, Y. D., Ren, H., Luo, T., Chen, R., and Liu, W. (2016). Soil organic carbon density and influencing factors in tropical virgin forests of Hainan Island, China. Chin. J. Ecol. 40, 292–303. doi: 10.17521/cjpe.2015.0314
Yang, H., Liu, S. R., Li, Y. D., and Xu, H. (2018). Diurnal variations and gap effects of soil CO2, N2O and CH4 fluxes in a typical tropical montane rainforest in Hainan Island, China. Ecol. Res. 33, 379–392. doi: 10.1007/s11284-017-1550-4
Yang, B., Zhang, W. H., Lu, Y. L., Zhang, W. W., and Wang, Y. A. (2019). Carbon storage dynamics of secondary Forest succession in the central loess plateau of China. Forests 10:342. doi: 10.3390/f10040342
Zhang, S., Quan, X. U., Yang, Q., Jiang, Y., Wang, X., and Liu, W. (2019). C, N, and P content and C:N ratio in sandy soil in the coastal zone of Hainan Island. Chin. J. For. Environ. 39, 398–403. doi: 10.13324/j.cnki.jfcf.2019.04.011
Zhang, C. C., Wang, Y. Q., Jia, X. X., and Shao, M. A. (2021). Estimates and determinants of soil organic carbon and total nitrogen stocks up to 5 m depth across a long transect on the loess plateau of China. J. Soils Sediments 21, 748–765. doi: 10.1007/s11368-020-02861-3
Zhang, Y. S., Yu, C. X., Xie, J. J., Du, S. T., Feng, J. X., and Guan, D. S. (2021). Comparison of fine root biomass and soil organic carbon stock between exotic and native mangrove. Catena 204:105423. doi: 10.1016/j.catena.2021.105423
Zhou, G., Liu, S., Li, Z., Zhang, D., Tang, X., Zhou, C., et al. (2006). Old–growth forests can accumulate carbon in soils. Science 314:1417. doi: 10.1126/science.1130168
Zhou, Z., Wang, C., and Luo, Y. (2018). Effects of forest degradation on microbial communities and soil carbon cycling: a global meta–analysis. Glob. Ecol. Biogeogr. 27, 110–124. doi: 10.1111/geb.12663
Zhu, X., Fang, X., Wang, L., Xiang, W., Alharbi, H. A., Lei, P., et al. (2021). Regulation of soil phosphorus availability and composition during forest succession in subtropics. For. Ecol. Manag. 502:119706. doi: 10.1016/j.foreco.2021.119706
Keywords: enzyme activity, pH value, root biomass, phosphorus limitation, litter biomass
Citation: Xing G, Wang X, Jiang Y, Yang H, Mai S, Xu W, Hou E, Huang X, Yang Q, Liu W and Long W (2023) Variations and influencing factors of soil organic carbon during the tropical forest succession from plantation to secondary and old–growth forest. Front. Ecol. Evol. 10:1104369. doi: 10.3389/fevo.2022.1104369
Received: 21 November 2022; Accepted: 09 December 2022;
Published: 09 January 2023.
Edited by:
Xiang Liu, Lanzhou University, ChinaReviewed by:
Rong Yang, Northwest Institute of Eco-Environment and Resources (CAS), ChinaCopyright © 2023 Xing, Wang, Jiang, Yang, Mai, Xu, Hou, Huang, Yang, Liu and Long. This is an open-access article distributed under the terms of the Creative Commons Attribution License (CC BY). The use, distribution or reproduction in other forums is permitted, provided the original author(s) and the copyright owner(s) are credited and that the original publication in this journal is cited, in accordance with accepted academic practice. No use, distribution or reproduction is permitted which does not comply with these terms.
*Correspondence: Yamin Jiang, amlhbmd5YW1pbjIwMDhAMTYzLmNvbQ==; Wenjie Liu,
TGl1d2pAaGFpbmFudS5lZHUuY24=
Disclaimer: All claims expressed in this article are solely those of the authors and do not necessarily represent those of their affiliated organizations, or those of the publisher, the editors and the reviewers. Any product that may be evaluated in this article or claim that may be made by its manufacturer is not guaranteed or endorsed by the publisher.
Research integrity at Frontiers
Learn more about the work of our research integrity team to safeguard the quality of each article we publish.