- 1Rubenstein Ecosystem Science Laboratory, University of Vermont, Burlington, VT, United States
- 2WasserCluster Lunz, Lunz am See, Austria
Species invasions can lead to ecological regime shifts by altering food web structure and changing nutrient cycling. Stable isotopes are a powerful tool to understand the potential and realized impacts of invasive species on food webs, especially when used in tandem with other dietary tracers. An invasion by one of the most notorious freshwater invaders in North America, the quagga mussel (Dreissena bugensis), is imminent in Lake Champlain, United States. An invasion by this filter feeder has the potential to drastically alter energy pathways and destabilize pelagic fisheries via bottom-up impacts. However, the extent and magnitude of these impacts depend on the current food web structure of the mid-trophic pelagic food web, which was previously not well described. We used Bayesian stable isotope mixing models informed by stomach content analysis to identify which energy pathways are currently most important to mid-trophic level fishes. We determined that in the Main Lake basin, the spring phytoplankton bloom and deep chlorophyll layer – the resources most vulnerable to quagga mussels – provide a disproportionate amount of support to the pelagic food web via zooplankton and the migrating macroinvertebrate Mysis. The food web in the Northeast Arm of Lake Champlain is supported by epilimnetic phytoplankton, which is more protected from the filtration effects of quagga mussels than the deep chlorophyll layer. However, the Northeast Arm will likely not provide a high-quality foraging refuge to coldwater pelagic fish due to unfavorable oxythermal conditions. The mid-trophic food web of Lake Champlain—and consequently piscivores who rely on these prey—may be vulnerable to the impending quagga mussel invasion if migratory Mysis are not able to shift their diet to benthic resources.
1. Introduction
Understanding food web structure and energy flow is critical for successful management and conservation of ecosystems threatened by anthropogenic change (Vander Zanden et al., 2006; Naman et al., 2022). For example, species invasions often lead to ecological regime shifts and have major impacts on ecosystem services (e.g., Charles and Dukes, 2007; Pejchar and Mooney, 2009); therefore, understanding the effects of invaders may be one of the most important ways managers can anticipate and effectively respond to ecosystem change. We must first understand the relative importance of top-down or bottom-up processes and the basal resources most critical for at-risk native species to evaluate the potential impact of an introduced species. Understanding the factors that favor invasion, how similar systems responded to the same invasive species, and the biogeochemical and ecological configuration of the at-risk system are necessary for anticipating the socio-ecological consequences of an invasion (David et al., 2017; Flood et al., 2020).
The invasive zebra [Dreissena polymorpha (Pallas, 1771)] and quagga (D. bugensis Andrusov, 1897) mussels are major drivers of ecological change in fresh waters of North America. The mechanisms and impacts of their invasions have been extensively documented (e.g., Limburg et al., 2010; Karatayev et al., 2015; Strayer et al., 2019). Dreissenid mussels are ecosystem engineers (Sousa et al., 2009) and are one the most successful freshwater invaders in the northern hemisphere (e.g., Higgins and Vander Zanden, 2010; Nalepa and Schloesser, 2014). They have high fecundity (Keller et al., 2007) and filtration capacity (Higgins and Vander Zanden, 2010), and can create extensive, dense colonies that are highly effective at suspension feeding. As a result, dreissenids sequester nutrients and phytoplankton from the water column to the benthos, resulting in system oligotrophication (Higgins and Vander Zanden, 2010). By sequestering nutrients and phytoplankton during annual turnover events, dreissenids incrementally reduce pelagic productivity over time, which has led to the disappearance of spring phytoplankton blooms, reduction of the deep chlorophyll layer (DCL), and increased benthic algal blooms in many lakes (e.g., Cecala et al., 2008; Higgins and Vander Zanden, 2010; Pothoven and Fahnenstiel, 2013). In the Laurentian Great Lakes, reductions in pelagic primary production are associated with declines of important invertebrate prey species, such as zooplankton, Mysis, and Diporeia spp. (Nalepa et al., 2009; Higgins and Vander Zanden, 2010; Johannsson et al., 2011); consequently, growth and biomass of mid-trophic level fishes often decline after mussels become established (Pothoven and Madenjian, 2008; Eppehimer et al., 2019). The extent of these impacts depends on whether one or both species of mussel has invaded a system.
Zebra mussels typically precede quagga mussels and spread to new systems quickly, but high densities are usually confined to littoral zones (Karatayev et al., 2015; Knight et al., 2018). Quagga mussels, despite spreading more slowly to new systems, reach greater densities than zebra mussels because they have higher growth and filtration rates, colonize deeper profundal areas, and outcompete and rapidly displace zebra mussels in shallow areas (Mills et al., 1999; Karatayev et al., 2015; Metz et al., 2018). Thus, quagga mussels often have more extensive ecosystem impacts than zebra mussels (Karatayev et al., 2015). Because the impacts of dreissenids are fairly predictable, management agencies may have time to adjust policies (e.g., adjust stocking rates or game fish catch limits) in response to an early invasion to partially offset predicted food web impacts.
Lake Champlain, United States/Canada, has only been invaded by zebra mussels, and therefore the opportunity exists to inform management actions that could help counteract the food web impacts of an imminent quagga mussel invasion. Zebra mussels were first discovered in the lake in 1993 but have been primarily limited to depths shallower than 25 m (Marsden et al., 2013; Knight et al., 2018). Quagga mussels have not yet invaded, despite their presence in the nearby St. Lawrence and Hudson Rivers (Figure 1; Riccardi et al., 1996; Strayer et al., 2020). An invasion is looming, given the connectivity of these systems to Lake Champlain (Figure 1). Hull inspections of vessels entering Lake Champlain from the Great Lakes are rare, but inspection of a vessel entering the lake from the Richelieu River (Figure 1) in 2016 found 30% of a sample of dreissenids on the hull were adult quagga mussels (Marsden, unpublished data). While the impacts of zebra mussels on Lake Champlain’s food web and water quality have been limited (e.g., Smeltzer et al., 2012; Knight et al., 2018), quagga mussels could colonize all depths of Lake Champlain, to the maximum depth of 122 m (Mills et al., 1996) and outcompete existing zebra mussel colonies (Ginn et al., 2018). Thus, quagga mussels are likely to have a greater impact on the Lake Champlain system than the established zebra mussel population. Although profundal quagga mussel colonies may have limited epilimnetic effects in large lakes with stratification (e.g., Karatayev et al., 2015), filtration during spring turnover could substantially reduce the spring phytoplankton bloom, which maybe an important source of food for pelagic primary consumers (e.g., Pothoven and Vanderploeg, 2022). Further, any production in the hypolimnion is vulnerable to filtration by quagga mussels. In lakes with a deep chlorophyll layer, quagga mussels can substantially reduce hypolimnetic production (e.g., Fahnenstiel et al., 2010; Malkin et al., 2012; Pothoven and Fahnenstiel, 2013). Consequently, quagga mussels could propagate bottom-up impacts in Lake Champlain by reducing the pelagic resources that support the pelagic fish community, exacerbating growing top-down pressure from a recovering lake trout (Salvelinus namaycush) population (Marsden et al., 2018; Wilkins and Marsden, 2021), resulting in a “trophic squeeze.”
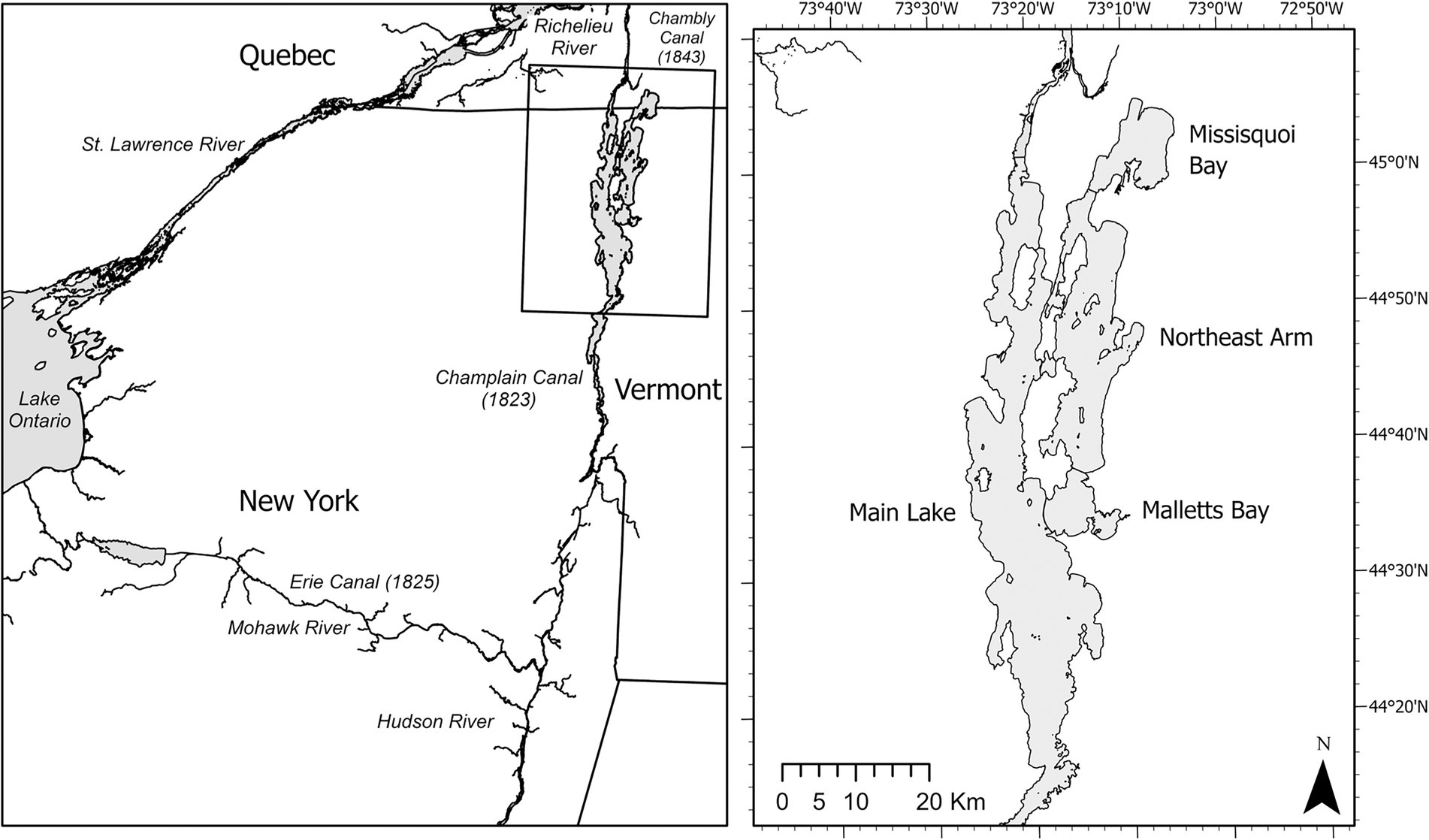
Figure 1. Map of Lake Champlain and its basins, showing connectivity of Lake Champlain and adjacent waterways. The Hudson River connects to Lake Champlain via the Champlain Canal in the south, and the lake flows north via the Richelieu River into the St. Lawrence River. Quagga mussels are established in Lake Ontario, the Hudson River, and the St. Lawrence River.
Lake Champlain’s salmonid populations were re-introduced by stocking in the early 1970s; however, natural recruitment of lake trout did not begin until 2012, and Atlantic salmon recruitment is severely limited by dams. The most important prey for adult lake trout and Atlantic salmon (Salmo salar) in Lake Champlain are pelagic alewife (Alosa pseudoharengus) and rainbow smelt (Osmerus mordax), and the benthic slimy sculpin (Cottus cognatus; Kirn and LaBar, 1996; Simonin et al., 2018; Marsden et al., 2022). A wild population of lake trout is becoming established, and wild age-0 lake trout rely on the macroinvertebrate Mysis as a primary prey (Marsden et al., 2022). Stocking numbers have been reduced because an increased abundance of predators due to natural recruitment may add predation pressure to prey fishes (Marsden et al., 2018; Wilkins and Marsden, 2021). Rainbow smelt and alewife feed on a combination of crustacean zooplankton and Mysis, and rainbow smelt are also cannibalistic (Labar, 1993). However, the relative importance of each prey group is unknown, especially Mysis, which represents an important link between benthic and pelagic habitats (Stockwell et al., 2020). The impact of quagga mussels on Mysis, and consequently on lake trout recruitment, will depend on the relative contributions of benthic and pelagic resources to Mysis diets.
The role of Mysis in the Lake Champlain food web is of particular importance because they are omnivorous and exhibit diel vertical migration (Euclide et al., 2017; O’Malley et al., 2018). The daily movement between benthic and pelagic habitat allows Mysis to feed on both detritus and planktonic resources (O’Malley and Stockwell, 2019), and exposes them to predation by both benthic (e.g., slimy sculpin, age-0 lake trout) and pelagic fishes (e.g., Gamble et al., 2011a, 2011b). We do not know the relative contribution of detritus, epilimnetic phytoplankton, and hypolimnetic phytoplankton (directly or via zooplankton and benthic invertebrates) to Mysis production or the contribution of Mysis to the mid-trophic level fish community in Lake Champlain, and thus we do not know how dependent the mid-trophic level fish community is on pelagic versus benthic energy pathways and thus its consequent vulnerability to quagga mussels.
We hypothesized that alewife and rainbow smelt primarily rely on pelagic energy pathways, specifically the spring phytoplankton bloom and DCL. As such, a reduction in hypolimnetic pelagic resources by quagga mussels would lead to declines in key zooplankton prey and consequent declines in pelagic planktivorous fish populations. Subsequently, pelagic piscivore populations may face a bottom-up-induced prey decline, which would reduce both the ecological integrity and recreational fishing opportunities in Lake Champlain. In contrast, because slimy sculpins rely on benthic energy pathways we hypothesize that they will be un-impacted or positively impacted by quagga mussels, therefore sculpin could partially offset the impacts on piscivores who forage in both benthic and pelagic habitats. We conducted bi-weekly sampling of the lower and middle food web of Lake Champlain’s two largest basins over 7 months, then used Bayesian isotope mixing models (MixSIAR; Stock et al., 2018) to identify the importance of benthic versus pelagic resources for Lake Champlain’s mid-trophic level fish community. We then used the model results to estimate the susceptibility of the same fish community to a quagga mussel invasion due to concurrent top-down and bottom-up pressures.
2. Materials and methods
2.1. Sample sites
Lake Champlain is a large, freshwater lake situated among northwestern Vermont, northeastern New York, United States, and southern Quebec, Canada. Water flows northward into the Richelieu River, and then into the St. Lawrence River (Figure 1). The Chambly Canal allows boat traffic, a potential invasion vector, to bypass rapids in the Richelieu River, while the Champlain Canal creates a pathway for invasive species by connecting the southern end of the lake to the Hudson River and Erie Canal (Marsden and Hauser, 2009). Causeways and islands separate the 193-km-long lake into four major basins (Figure 1), each with contrasting morphometry and trophic conditions. The Main Lake is the largest (up to 19 km wide) and deepest basin (maximum 122 m) and is mesotrophic. The Northeast Arm is the second largest basin by area, with a maximum depth of 49 m, and is eutrophic. Cyanobacteria blooms are common in both basins in summer, but more severe and expansive in the Northeast Arm. Openings in the causeways that separate the basins are shallow (1–7 m) and narrow (<100 m), so passage of cold-water species between basins is presumed to be restricted to the non-stratified season. Slimy sculpin, rainbow smelt, and lake whitefish populations in the Main Lake and Northeast Arm are not genetically isolated (Euclide et al., 2018, 2019, 2020), and lake trout are found in the the Northeast Arm in winter but not during the stratified seasons, indicating that transfer of fishes and nutrients does occur between basins. Water flow is mostly from the Northeast Arm into the Main Lake, which may account for the slow colonization of zebra mussels into the Northeast Arm (Marsden and Langdon, 2012).
We sampled a 40-m and a 100-m deep site in the Main Lake and a 40-m deep site in the Northeast Arm (Figure 1). At each site, we sampled the lower food web (invertebrates, phytoplankton, sediment) biweekly and the full food web (fish, Mysis, and lower food web) monthly from May to November, 2019. On all sampling dates we recorded mean Secchi depth (m) and measured water-column profiles using a CastAway temperature-depth probe (SonTek©, San Diego, CA, United States) or Seabird CTD (Sea-Bird Scientific).
2.2. Sample collection
We collected three replicate samples of phytoplankton, zooplankton, benthic invertebrate, and sediment (proxy for detritus) every 2 weeks at each site. Integrated photic zone water samples for phytoplankton were collected by lowering a 25-m garden hose (2-cm diameter) with weight on the end to a depth of 2.5× the Secchi depth and emptying the filled hose into a bucket. The sample was mixed, then poured into a 4-L opaque Nalgene bottle. Zooplankton were collected from the whole water column using a 150-μm Bongo net, then concentrated into a 250-ml sample jar. Benthic invertebrates were sampled using a 152 × 152 mm Ponar grab. All samples were kept in a cooler on ice until returning to the lab.
At each 40-m site, we conducted daytime bottom trawls, and at the 100-m site we conducted daytime and nighttime midwater trawls to target alewife, smelt, and sculpin. Trawl depths varied ±10 m. Bottom trawls were not conducted at the 100-m site due to gear limitations. Fish were measured (total length; TL, in mm) on board and separated by size class; 20 of each size class of each species was collected at each site. Alewife were split into small (<100 mm), medium (100–200 mm), and large (>200 mm) size classes; rainbow smelt into small (<100 mm), medium (100–150 mm), and large (>150 mm) classes; and sculpin into small (<60 mm) and large (>60 mm) classes. All fish were promptly frozen onboard before transferring to a −20°C freezer at the laboratory.
2.3. Laboratory processing and analysis
Water samples were refrigerated and then filtered within 24 h of collection onto 1.2-μm glass fiber filters for stable isotope analysis. Zooplankton samples were filtered through a 350-μm sieve to remove filamentous algae, then left to settle for 30–60 min to separate from remaining phytoplankton. The top clear layer was poured off and inspected and picked for any remaining debris or large phytoplankton, then the concentrated sample was added to a scintillation vial in preparation for drying and stable isotope analysis. Benthic invertebrate samples were sieved to remove large debris, then picked for all conspicuous taxa, grouped by major taxonomic group (chironomids, oligochaetes, amphipods, gastropods), then rinsed with deionized water. Each Mysis replicate was sorted into juvenile (TL < 10 mm; from the tip of the rostrum to the tip of the telson) and adult (TL > 10 mm) size classes and counted; 10–20 individuals of each size class were then rinsed in deionized water and grouped into scintillation vials in preparation for drying and stable isotope analysis. Fish were measured for total length (mm) and wet weight (g), then dissected. Dorsal muscle tissue plugs were taken for isotope analysis, and stomachs were removed, weighed, then preserved in 90% ethanol. Fish, invertebrate, phytoplankton, and sediment samples were then prepared for bulk isotope analysis by drying at 40°C for 24–48 h, depending on density and water content, then homogenized (with the exception of filtered samples) with a mortar and pestle or a glass rod in a scintillation vial. Homogenized samples were then subsampled, phytoplankton were scraped from filters, weighed (μm), and packed in tin capsules for bulk stable isotope analysis. All isotope samples were analyzed for δ13C and δ15N at the UC Davis Stable Isotope Facility using a PDZ Europa ANCA-GSL elemental analyzer interfaced to a PDZ Europa 20–20 isotope ratio mass spectrometer (Sercon Ltd., Cheshire, United Kingdom) with reference material Vienna PeeDee Belemnite and air for carbon and nitrogen, respectively (SD was 0.2‰ for 13C and 0.3‰ for 15N). Internal duplicates (n = 22) indicated samples were well-homogenized (paired t-test; p = 0.35 for δ13C and p = 0.82 for δ15N).
2.4. Stomach analysis
Fish stomach contents were identified by microscope and prey were coarsely grouped as amphipods, oligochaetes, zooplankton, detritus, terrestrial insects, Mysis, fish, or eggs. Each item was assigned a percent of total composition by weight. For the first 20 stomachs of each fish species, each prey group was weighed separately to determine percent composition; percent composition of each taxon was subsequently estimated by eye for the remaining stomachs. We evaluated this protocol by comparing the precise measurements of percent composition to those estimated by eye; estimates were within 10% error of true percent composition by weight. Estimates were also within 5% error among individual technicians.
2.5. Trophic discrimination factors
We calculated δ15N and δ13C trophic discrimination factors (TDFs, ΔyX) for fish using our isotope and diet data because the isotope mixing space better aligned with consumer isotope data relative to data calculated with literature TDFs (e.g., Caut et al., 2009; Bastos et al., 2017). For each size class of each fish species, we calculated the mean dietary contributions of each prey type (pprey) using our stomach data. We then used (pprey) to weigh the relative importance of each prey’s nitrogen and carbon isotope values when calculating the difference in δ15N and δ13C between the consumer and the prey (the TDF) using the following equation:
For size classes that consumed primarily one prey source (e.g., small alewife were exclusively zooplanktivorous), we calculated the TDF as the difference between consumer and prey isotope values. All fish δ13C data were lipid-corrected with the following equation: Δ13C = −3.32 + 0.99 × C:N (Post et al., 2007). Literature TDF values were used for zooplankton and Mysis (Brauns et al., 2018). Final TDF values used in the models are listed in Table 1.
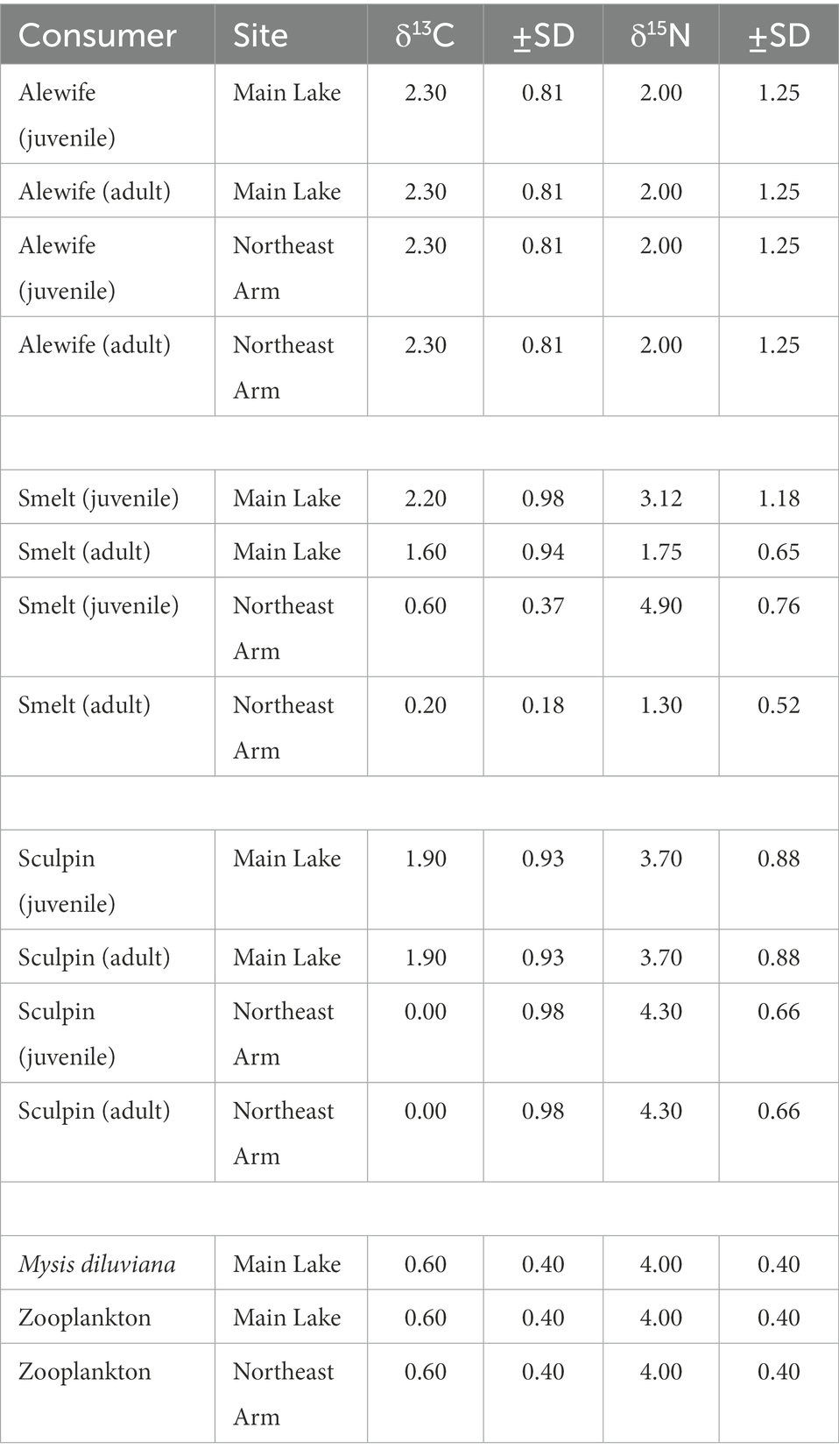
Table 1. Trophic enrichment factors (TEF) used in the Bayesian mixing models for each consumer in the different life stages (juveniles × adult) and sites of Lake Champlain.
2.6. Estimating pelagic × benthic contributions to fish and invertebrates
To evaluate the relative contributions of pelagic and benthic resources to mid-trophic level fishes, we used Bayesian mixing models (MixSIAR; Stock et al., 2018). For each fish species at each site, two models were run: a prey-based model, and a primary producer-based model (using average δ13C and δ15N of the respective sources). For Mysis and zooplankton, only the primary producer model was employed. The invertebrate samples were divided into Early Season (May–July) and Late Season (August–October) because zooplankton isotopic turnover is a few weeks (Emery et al., 2015) – zooplankton tissues reflect consumption from 1 to 2 weeks before collection. Isotopic turnover for juvenile fish, however, is from 1 to 3 months (Oliveira et al., 2017; Hernández-Urcera et al., 2022), and this time lag between consumption and consumer isotopic assimilation must be considered in the models for more reliable results (Vander Zanden and Rasmussen, 2001; Hussey et al., 2014; Lanari et al., 2021; Possamai et al., 2021).
Due to the possible ontogenetic shifts in the diet of the fish species and Mysis, these consumers were categorized into size classes based on their total length (Mysis: TL < 10 mm small, and TL > 10 mm large; Fish: see section 2.2). Diets of medium and large size classes of rainbow and alewife were similar, therefore we combined medium and large fish (hereafter referred to as “large” fish) within each species in the models to increase the sample size. Size class was included as a fixed factor in the model. The sources included in the prey-based model were selected based on the stomach contents of each fish species/size class, and the seasonal averages of δ13C and δ15N values of the prey species were used. We used the seasonal averages of δ13C and δ15N of small rainbow smelt as the values for prey fish, as these were the only fish species identified in stomachs. Mysis had a low abundance in the Northeast Arm, therefore models for this species were only run in the Main Lake. In the producer models, sources were selected based on their presence/absence at the site. Source δ13C and δ15N values for epilimnetic phytoplankton and detritus were seasonal averages. The δ13C and δ15N values for the spring bloom were an average of all Main Lake samples collected during spring turnover (April and May), and we assumed the isotopic composition of the bloom in the Northeast Arm was similar. The isotopic values for the DCL were taken from the literature (Francis et al., 2011); this source was only included in the Main Lake models, as the Northeast Arm is too productive to develop a DCL.
Trophic discrimination factors (TDF) used for each fish species/site were calculated based on the stomach content analysis and δ13C and δ15N values of prey species. For all Bayesian models, no informative priors were used, because (i) no prior information is available for producers’ contributions to these consumers in this system, and (ii) although we had stomach content information for these species to include in the prey-based models, informative priors can bias models with a small number of samples (Brown et al., 2018). Moreover, our models represent a season with rapid isotopic turnover of producers, but isotopic assimilation into tissues is relatively slow, therefore diet information may differ from the modeled diet based on the stable isotopes. The mixing models were fitted using the Markov Chain Monte Carlo (MCMC) method, running 100,000 simulations for each model and discarding the first 50,000 simulations used for burn-in. If the model did not reach good diagnostics (Gelman-Rubin Diagnostic <1.05, and Geweke Diagnostic ±1.96), we ran the model again using 300,000 simulations with 200,000 burn-ins. Results were reported as the median (50%) and 95% Bayesian credibility intervals of the estimated contributions. Bayesian analyses were performed using JAGS 4.3.1 (Denwood, 2016), and the models were performed by MixSIAR package (Stock and Semmens, 2016) in R 4.2.0 (R Core Team, 2022).
3. Results
Diet data and mixing models indicated that mid-trophic fish in Lake Champlain generally rely on pelagic pathways, via zooplankton and Mysis as their primary food resources, with some variation between basins. In the Main Lake, zooplankton and Mysis dominated the stomach contents of pelagic fish, while Mysis and benthic invertebrates were more prevalent in the stomachs of benthic fish (Figure 2). In the Northeast Arm, Mysis abundance is low; amphipods and zooplankton were the primary prey sources for pelagic fish, and amphipods and other benthic invertebrates (oligochaetes and chironomids) dominated benthic fish stomachs. The prey-based MixSIAR models largely aligned with the stomach content analysis, and the producer-based models revealed that seasonal resources are very important in sustaining the Lake Champlain food web.
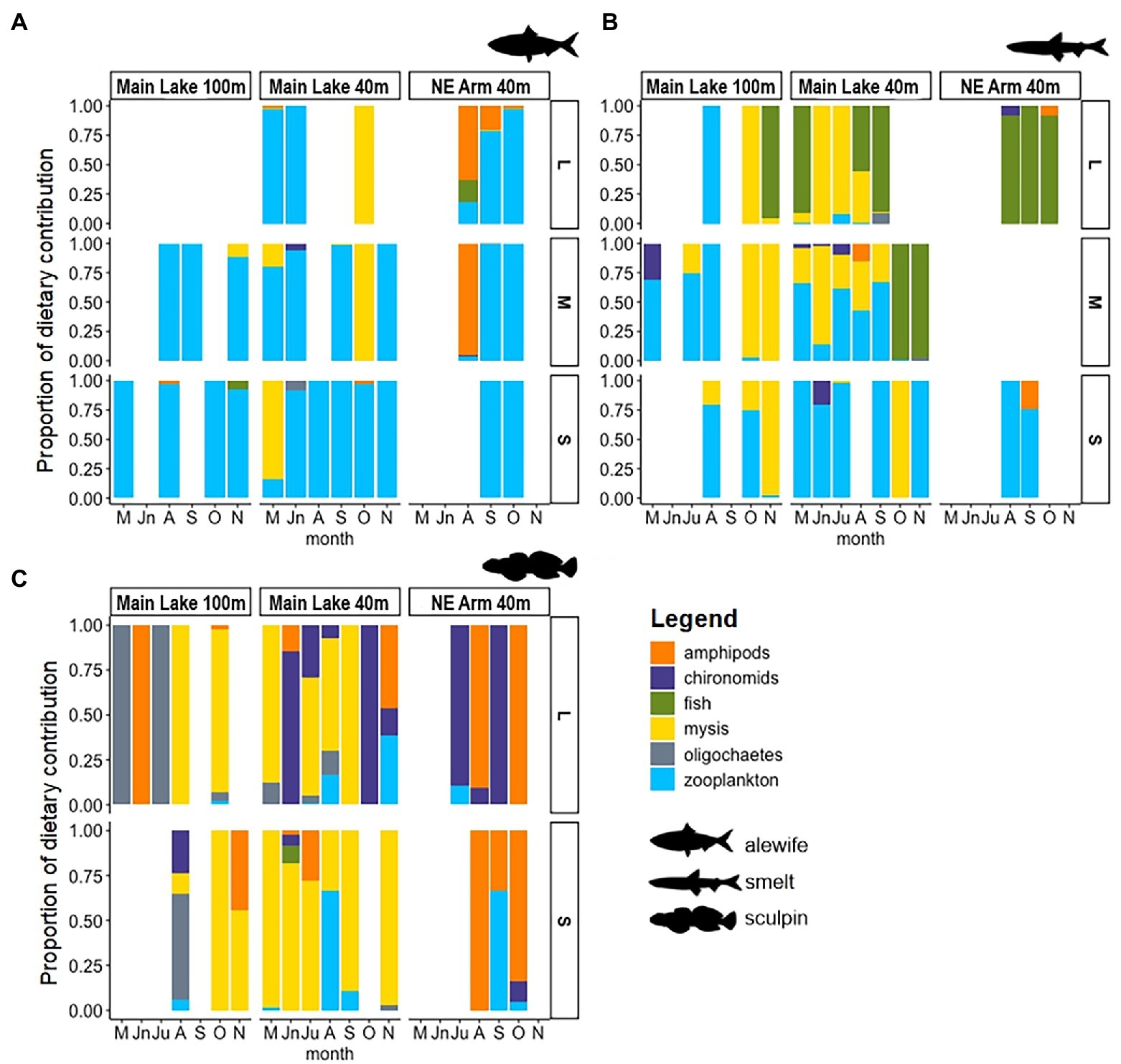
Figure 2. Stomach content composition of three different sizes (small, medium, and large) of (A) alewife (n = 124), (B) rainbow smelt (n = 137), and (C) slimy sculpin (n = 152) for three sites in Lake Champlain: Main Lake at 100-m depth, Main Lake at 40-m depth, and Northeast Arm at 40-m depth. Sampling period months start in May (M) and end November (N) 2019. ‘Fish’ in the figure legend corresponds to juvenile rainbow smelt.
3.1. Alewife
Alewife consumed primarily zooplankton across all life stages and at both sites (Figure 2A). Mysis (Main Lake) and amphipods (Northeast Arm) occasionally dominated (>60%) stomach contents of large alewife, depending on the time of year (early season for Mysis and late season for Amphipoda; Figure 2A; Supplementary Table 1). The prey-based mixing models indicated that small and large alewife at both sites acquired the majority of their energy from zooplankton; zooplankton diet contributions were greater than 80% in the Main Lake, and greater than 60% in the Northeast Arm (Figure 3A; Supplementary Table 1). The primary producer-based models suggested that phytoplankton (55% contribution) and the spring bloom (44% contribution) were similarly important for sustaining alewife during the summer and autumn in the Main Lake, for both size groups of alewife. However, in the Northeast Arm, spring bloom had lower importance (<35%); epilimnetic phytoplankton production was the major contributor of carbon to small (72%) and large (66%) alewife (Figure 3B; Supplementary Table 2).
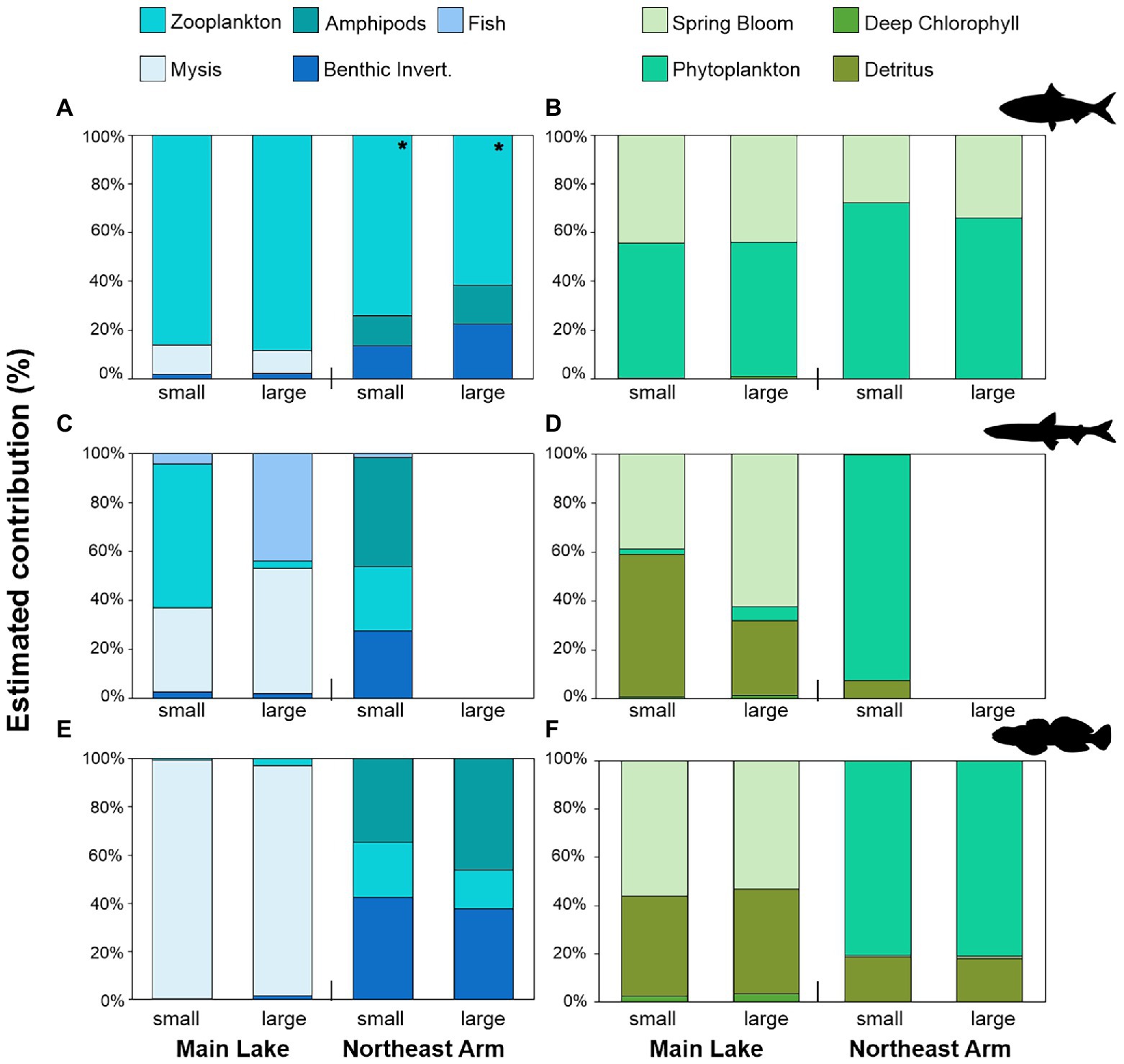
Figure 3. Relative contributions (%) of each carbon source to the fish species estimated by MixSIAR models. Contributions were estimated for small and large individuals from two sites in Lake Champlain (Main Lake and Northeast Arm). (A) Prey contributions to alewife, (B) producer contributions to alewife, (C) prey contributions to rainbow smelt, (D) producer contributions to rainbow smelt, (E) prey contributions to slimy sculpin, and (F) producer contributions to slimy sculpin. Mysis, Mysis diluviana; Benthic Invert., benthic invertebrates including bivalves, insect larvae, gastropods, and oligochaetes. Spring bloom refers to a spring phytoplankton bloom that occurred during spring turnover in May 2019. Phytoplankton refers to epilimnetic phytoplankton. * Prey-based models for alewife in the Northeast Arm included the contribution of zooplankton from the Main Lake.
3.2. Rainbow smelt
Rainbow smelt diets exhibited an ontogenetic shift where small individuals primarily preyed on zooplankton and Mysis at both sites, while large individuals had higher consumption of Mysis and juvenile rainbow smelt (Figure 2B). Prey-based MixSIAR models also showed ontogenetic shifts in rainbow smelt diet, with higher contributions of zooplankton to the small individuals (57%), and Mysis (50%) and fish (43%) to the large smelt in the Main Lake (Figure 3C; Supplementary Table 1). Northeast Arm prey-based models did not resolve well because large rainbow smelt did not fit within the isotope mixing space (Supplementary Figure 1A), whereas stomach data indicated a diet of almost exclusively juvenile smelt; issues of sample size and tissue turnover relative to timing of movement to the Northeast Arm may explain this discrepancy. Small rainbow smelt from the Northeast Arm had a large contribution of amphipods (43%) in the diet (Figure 3C), but this result did not align with stomach content data, which indicated a diet of almost exclusively zooplankton (Figure 2B). Producer-based models showed inverted patterns for small and large rainbow smelt in the Main Lake. Benthic energy (detritus) was the largest contributor to diets of small individuals (57%), followed by the pelagic spring phytoplankton bloom (>38%). This pattern was the opposite for large individuals, with detritus the smallest contributor (30%) and the spring bloom the largest (62%; Figure 3D; Supplementary Table 2). For small rainbow smelt in the Northeast Arm, the producer-based model showed contributions >90% from epilimnetic phytoplankton (Figure 3D), more aligned to the stomach contents results than the prey-based model. Primary-producer based models for large rainbow smelt in the Northeast Arm were not well resolved (Supplementary Figure 1B).
3.3. Slimy sculpin
In the Main Lake, the diets of small slimy sculpins comprised mainly Mysis, while large individuals also preyed on benthic invertebrates such as chironomids and amphipods (Figure 2C). The slimy sculpins captured in the Northeast Arm showed the same pattern for both small and large individuals, with the diet comprised entirely of amphipods and other benthic invertebrates (Figure 2C). The prey-based MixSIAR model showed a similar diet for both small and large slimy sculpin in Main Lake, with Mysis composing more than 90% of the diet (Figure 3E; Supplementary Table 1). For the Northeast Arm, the model could not distinguish well between amphipods and benthic invertebrate contributions (Supplementary Figure 2), given both prey groups likely rely on benthic basal resources (Figure 3E). The combined contributions of amphipods and benthic invertebrates were > 70% for both small and large slimy sculpin. In the producer-based MixSIAR models, the spring bloom showed a great contribution to slimy sculpin in both small (55%) and large (52%) body sizes in the Main Lake, followed by high contributions of detritus (41 and 43% for small and large, respectively; Figure 3F; Supplementary Table 2). In the Northeast Arm, pelagic resources were the main contributor to slimy sculpin diets, with an estimated contribution >80% for both small and large individuals (Figure 3F; Supplementary Table 2).
3.4. Mysis diluviana
Mysis were abundant in the Main Lake but rare in the Northeast Arm. Models showed some isotopic differences between small and large individuals and across the open water season. During the early season (May–June), our model suggested small Mysis preyed primarily on zooplankton (67%), while zooplankton (40%) and detritus (30%) contributed almost equally to the large individuals (Figure 4A; Supplementary Table 3). The spring bloom was not identified as an important resource for Mysis foraging, with contributions of just 15%. However, the model to estimate large Mysis diets in early season was not well resolved among sources (Supplementary Figure 3C). In the late season, an ontogenic pattern was observed, with benthic resources serving as the major contributor to small Mysis (>55%), while pelagic sources were the major contributor to large Mysis (68%; Figure 4A; Supplementary Table 3).
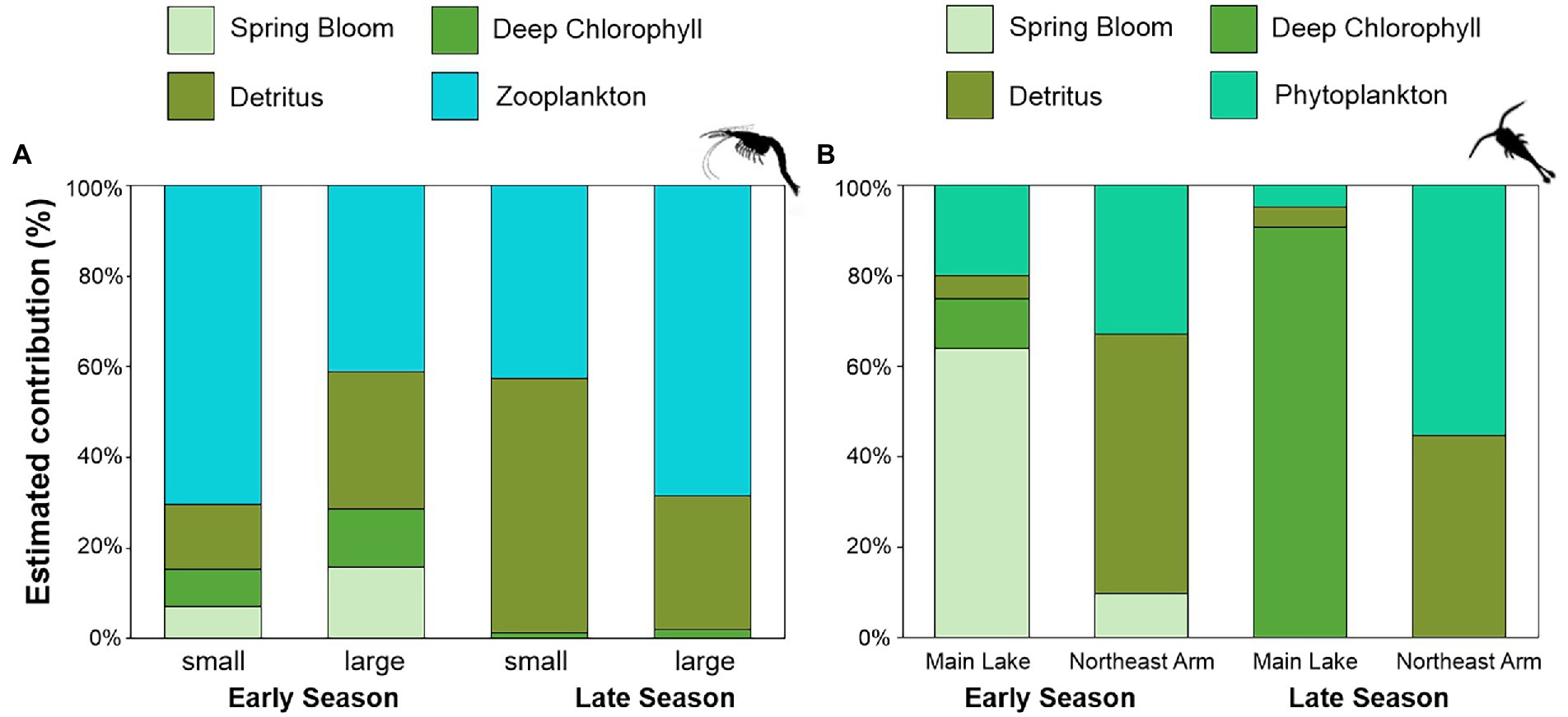
Figure 4. Relative contributions (%) of each prey source to consumers estimated by MixSIAR models. (A) Contributions to Mysis estimated for small and large individuals in Main Lake, Lake Champlain during the early (May–July) and late (August–November) seasons. (B) Contributions to zooplankton estimated in both sites (Main Lake and Northeast Arm) during early and late seasons. Spring bloom refers to a spring phytoplankton bloom that occurred during spring turnover in May 2019. Phytoplankton refers to epilimnetic phytoplankton.
3.5. Zooplankton
The MixSIAR models indicated that, during the early season, spring blooms compose more than 60% of the energy sources for zooplankton in Main Lake, with the remainder from epilimnetic phytoplankton (19%) and the DCL (10%) (Figure 4B; Supplementary Table 3). In the Northeast Arm, the model could not distinguish well between contributions from detritus versus phytoplankton (Supplementary Figure 4), but estimated 57% from detritus and 33% from epilimnetic phytoplankton (Figure 4B; Supplementary Table 3). During the late season, the DCL is an important resource for the zooplankton in the Main Lake (90%), while in the Northeast Arm the main contributors to zooplankton diets are phytoplankton (55%), and detritus (44%; Figure 4B; Supplementary Table 3).
4. Discussion
Carbon and nitrogen stable isotopes are commonly used to define trophic connections in aquatic systems through carbon flow, organic contamination, and ecosystem functioning (Ishikawa et al., 2017; Dias et al., 2018; Possamai et al., 2020). We used stable isotopes to predict the potential effects of an imminent biological invasion on the aquatic food chain and species interaction. Moreover, by combining stable isotope methodology with stomach content data, we were able to calculate specific trophic discrimination factors to better resolve our mixing models. Our field and modeling results suggest a quagga mussel invasion in Lake Champlain could have a large impact on the pelagic food web, but this effect would likely be stronger in the Main Lake than in the isolated Northeast Arm. The current Main Lake lower food web is heavily supported by the spring phytoplankton bloom early in the open-water season, then support shifts to the DCL later in the summer. Both sources of primary production are important for zooplankton; together they contribute >70% of the energy for zooplankton vs. < 20% from epilimnetic phytoplanktonic production. Zooplankton is a primary prey of rainbow smelt and Mysis, and the predominant prey for alewife (Figure 5A). If quagga mussels reduce the biomass of the spring phytoplankton bloom and DCL as observed in the Great Lakes (e.g., Vanderploeg et al., 2010; Pothoven and Fahnenstiel, 2013), these energy pathways will likely weaken (Figure 5B) and zooplankton biomass will decline, presumably to the detriment of their predators (e.g., Pothoven and Madenjian, 2008; Nalepa et al., 2009; Higgins and Vander Zanden, 2010; Eppehimer et al., 2019).
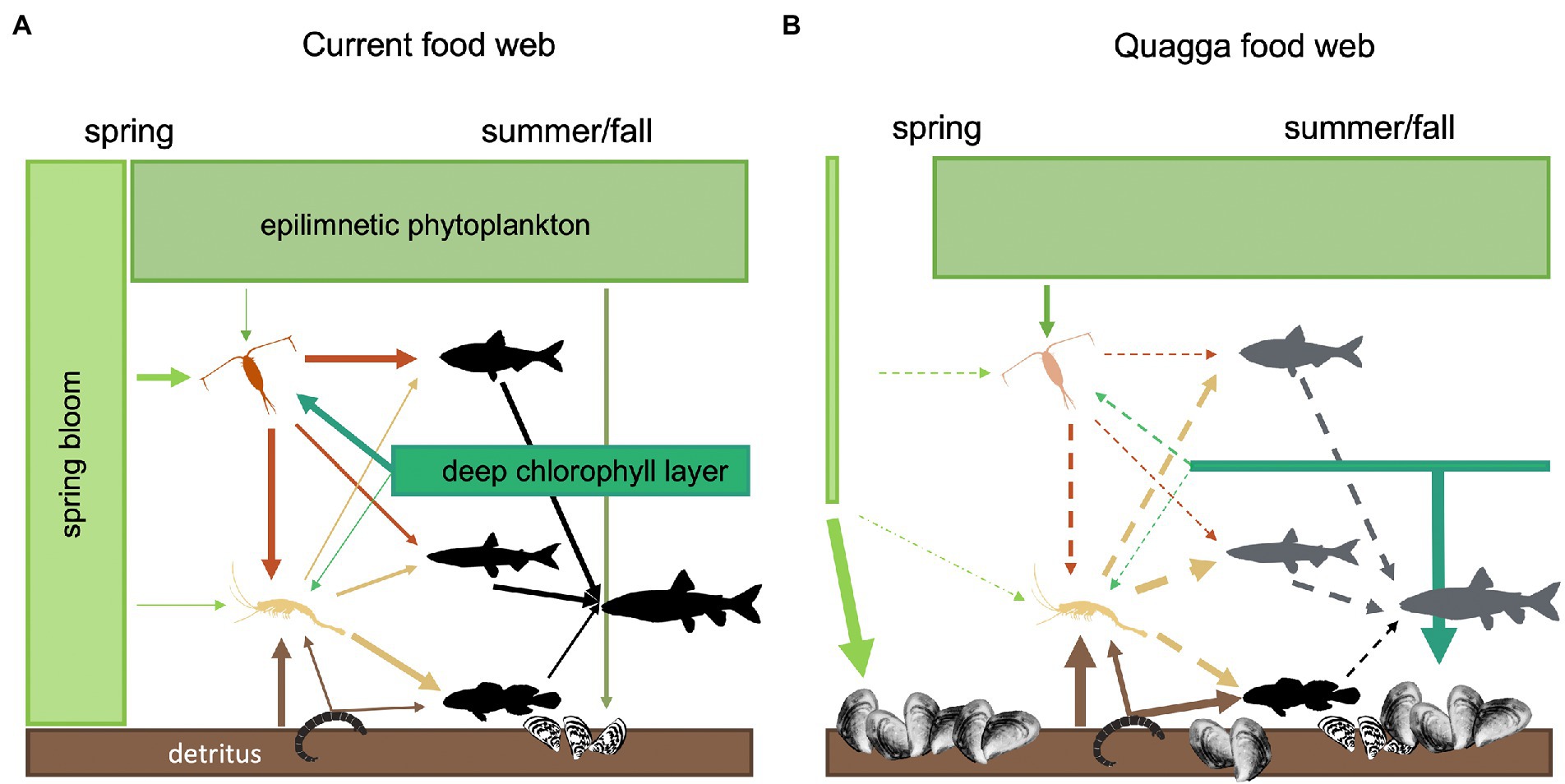
Figure 5. Conceptual figure of (A) current energy pathways and (B) our assessment of how quagga mussels may impact energy flow once established in the Main Lake of Lake Champlain. The orange copepod represents zooplankton populations, the yellow Mysis represents Mysis populations, the fish (from top to bottom) represent alewife, rainbow smelt, lake trout, and slimy sculpin populations. Size of the boxes represents biomass of the basal resource pool. Line thickness indicates relative strength of each energy pathway before and after invasion. Dotted lines indicate a weak or uncertain pathway. Faded color in the quagga food web (B) indicates uncertain population sustainability. If a quagga invasion weakens the most important pelagic energy sources (the spring bloom and deep chlorophyll layer) to the pelagic food web in the Main Lake (B), and stocking of predator populations continues at current rates, mid-trophic level fish may experience a “trophic squeeze.”
Although the mixing models did not detect a DCL contribution to fish, the DCL likely contributes to these consumers late in the year, but did not appear in our models due to tissue turnover time. Isotopic turnover for fish can be about 1–4 months longer than zooplankton (Emery et al., 2015; Oliveira et al., 2017), and potentially up to 6 months longer for cold water fish species (Skinner et al., 2017). Consequently, if the DCL is important to fish species, it would be detected during the winter. Unfortunately, we do not have samples during winter time to corroborate this hypothesis, but given our model results indicate the DCL is important for the lower food web, we can infer that it is also likely important for zooplanktivorous fishes.
Mysis is also an important component of the Lake Champlain food web, supporting both pelagic and benthic fish populations. The impact on Mysis of losing spring bloom and DCL production is still uncertain, as their dietary plasticity may allow them to shift to a predominantly benthic diet. Our models agree with existing literature (Hrycik et al., 2015) and indicate zooplankton have high importance for both juvenile and adult Mysis in Lake Champlain. In addition to zooplankton, Mysis were also strongly supported by the detrital pathway (detritus and macroinvertebrates), especially when the spring bloom was absent. Benthic habitats are often an underestimated resource for Mysis (Stockwell et al., 2020) and quagga mussels do not seem to negatively impact benthic invertebrate biomass (Ward and Ricciardi, 2007; Ozersky et al., 2011). In fact, increased production of detritus and the production of pseudofeces by quagga mussels may present a novel dietary resource for Mysis. If so, pelagic fish that rely on Mysis (e.g., rainbow smelt) may have their energetic pathway shifted from pelagic to benthic, without large dietary shifts or losses to population biomass. However, preliminary fatty acid and deuterium fatty acid data indicate pelagic prey are an important source of essential fatty acids for Mysis (Supplementary Figures 5, 6). For instance, an essential dietary fatty acid (Docosahexaenoic acid, DHA) was not present in high enough concentrations in sediment for compound-specific isotopic analysis, which indicates this fatty acid is not readily available for benthic consumers. Further, Mysis fatty acid deuterium values aligned with zooplankton more than benthic invertebrates, indicating essential nutrients likely come from pelagic resources (Supplementary Figure 6). Therefore, if Mysis shift to a predominantly benthic diet after quagga mussels establish, their quality as a prey resource may decline, consequently impacting slimy sculpin and rainbow smelt. For example, the physiological condition of Mysis in Lake Huron may have declined due to either invasion-induced competition and/or dreissenid-related loss of diatoms and zooplankton (Mida Hinderer et al., 2012). Additionally, the projected loss of zooplankton biomass may increase predation pressure on Mysis by zooplanktivorous pelagic fish (e.g., alewife, rainbow smelt), and therefore negatively impact Mysis population size; however, this may be mitigated by top-down pressures from lake trout on Mysis predators, as wild lake trout recruitment is increasing in Lake Champlain (Marsden et al., 2018; Wilkins and Marsden, 2021). Despite the uncertainty around food quality, the ability of Mysis to make use of benthic energy sources may make them a key player in maintaining some amount of stability in the mid-trophic level food web in the Main Lake. This dietary plasticity of consumers is argued to contribute to the capacity of lakes to adapt to stressors such as invasive species (McMeans et al., 2016). However, Mysis population densities already experienced an apparent rapid decline in the mid-1990s (Ball et al., 2015), and so the ability of Myisis to adapt to the arrival of quagga mussels is uncertain.
In contrast with the Main Lake, the mid-trophic level fish community in the Northeast Arm may not be as susceptible to a quagga mussel invasion. The basin is eutrophic and epilimnetic production is too high to allow the formation of a DCL in the hypolimnion. Our models suggested epilimnetic production was the most important basal energy resource for zooplankton in this basin. Consequently, pelagic fish – and even slimy sculpin – were primarily supported by epilimnetic phytoplankton via zooplankton. The epilimnion is often more protected from filtration by dreissenids than the hypolimnion (Fahnenstiel et al., 2010) and given the Northeast Arm is eutrophic during summer stratification, dreissenid-induced decreases in epilimnetic production would be more incremental and unlikely to decrease enough to compromise the availability of this basal resource. Further, the volume of epilimnetic production is much greater than the volume of production in the DCL, such that more time would be needed to see meaningful decreases in epilimnetic production relative to the DCL. Lastly, quagga mussels colonize deep waters prior to littoral areas, and thus access the DCL before epilimnetic production. Therefore, we do not expect the food web in the Northeast Arm to be as severely impacted by a quagga invasion. The spring bloom was minimally important for zooplankton in this basin; however, we used the isotope values for the bloom in the Main Lake in our Northeast Arm models, as sampling in the Northeast Arm did not start until after spring turnover. Therefore, if the isotopic composition of the spring bloom in the Northeast Arm differs from the Main Lake (for example, due to differences in phytoplankton community composition or CO2 availability; Vuorio et al., 2006), we may not have accurately represented this potential resource. Future studies should explore biomass and relative importance of hypolimnetic and epilimnetic phytoplankton in the Northeast Arm food web to better assess the potential impact of quagga mussels in this basin.
The impacts of quagga mussel invasion on productivity could potentially lead to greater spatial segregation among basins of mid-trophic level fish populations in Lake Champlain. Rainbow smelt are more confined to the Main Lake (Bruel et al., 2021) while alewife may fare better in the warmer Northeast Arm. Although the Northeast Arm may be more resistant to the impacts of quagga mussels than the Main Lake on important basal resource pools, it may not provide a high-quality alternative foraging habitat for rainbow smelt relative to the Main Lake because of differences in water quality and resource availability. Water temperature and productivity is higher, dissolved oxygen is lower, and depth is shallower in the Northeast Arm than in the Main Lake, and rainbow smelt abundance in the Northeast Arm is generally declining (Bruel et al., 2021). Additionally, as lake temperatures warm due to climate change (O’Reilly et al., 2015; Woolway et al., 2020), the low availability of appropriate oxythermal habitat for pelagic coldwater species such as rainbow smelt in the Northeast Arm will continue to decrease. Alewife, which have a higher thermal tolerance than rainbow smelt (Simonin et al., 2012), may fare better in the Northeast Arm, where zooplankton populations may be less at-risk to the impacts of an invasion. However, when alewife first invaded Lake Champlain, they became the dominant pelagic species, overlapping with the rainbow smelt niche (Marsden and Langdon, 2012; Simonin et al., 2012, 2018). Alewife could pose a competitive risk to rainbow smelt if they can adapt to the food web changes introduced by quagga mussels. Therefore, populations of both species may necessarily become more spatially segregated between the Main Lake and Northeast Arm. Further, the potential lower availability of food for rainbow smelt in the Main Lake combined with the higher competitive ability of alewife could pose a risk to rainbow smelt stocks after quagga mussels become established in Lake Champlain.
Another mid-trophic fish, the slimy sculpin, may provide some relief to piscivores if pelagic mid-trophic species decline. The primary carbon source for slimy sculpin is provided by the benthic pathway (via detrital epilimnetic carbon) and is unlikely to be impacted by a quagga mussel invasion (Ward and Ricciardi, 2007; Ozersky et al., 2011). Slimy sculpin is a dominant prey of juvenile lake trout in the Great Lakes (Elrod, and OˈGorman, R., 1991; Owens and Bergstedt, 1994; Madenjian et al., 2005). Therefore, regardless of the losses in the rainbow smelt population, lake trout may switch to slimy sculpin as their primary prey in Lake Champlain, assuming the recent re-emergence of wild recruitment (Marsden et al., 2018) is unrelated to the increased prey availability introduced by the alewife invasion (which is unlikely; Lesser et al., in review).
Although our models provided robust results for the majority of the species and basins, isotope values of detritus and phytoplankton cannot be readily resolved, which means the majority of detritus is likely derived from epilimnetic phytoplankton – suggesting other phytoplankton (the spring bloom and DCL) is more likely to be consumed before contributing to the detrital pool. The importance of non-epilimnetic phytoplankton in the Main Lake models supports this possibility; they are available for less time, so any signal they contribute to detritus could become diluted by epilimnetic phytoplankton. Resolving the differences in detrital and epilimnetic energy pathways is less important for understanding the potential impacts of a quagga invasion on zooplankton and mid-trophic level fishes than resolving differences among pelagic resource pools, given quagga mussels will primarily impact the spring bloom and DCL (Vanderploeg et al., 2010; Pothoven and Fahnenstiel, 2013). However, we do need to resolve the importance of benthic and pelagic/epilimnetic pathways to determine the potential impact of quagga mussels on Mysis, whose diet includes both detritus and benthic invertebrates. The preliminary data on hydrogen stable isotopes of fatty acids provided more precise insight by indicating where certain essential nutrients (DHA, EPA) were derived, but the sample size was exploratory. Finally, the contributions of littoral production to the pelagic food web are unknown, but likely important. Dreissenids shunt production away from offshore areas to littoral zones; therefore, organisms that move laterally between habitats could potentially access littoral energy subsidies if sufficient food is no longer present in offshore pelagic zones. To fully resolve benthic, epilimnetic, and littoral energy sources in Lake Champlain, we need a three-isotope mixing model; sulfur would likely be a useful third isotope to use in future studies (e.g., Croisetière et al., 2009).
An inverse pattern in δ15N of epilimnetic phytoplankton and detritus was observed from May to November. Early in the season, phytoplankton was less enriched in δ15N relative to detritus, and gradually increased by +2‰ across the sampling period (Supplementary Figure 4). Detritus exhibited the opposite pattern – more enriched at the beginning of the season and depleted by −2‰ over the course of the sampling period. The time sequence of phytoplankton production, senescence, and sinking, and the inverse pattern in δ15N of epilimnetic phytoplankton and detritus from spring to autumn supports the hypothesis that detritus in Lake Champlain primarily comprises epilimnetic phytoplankton. Moreover, this pattern in phytoplankton δ15N suggests that after the early season pool of nutrients is depleted, nitrogen remineralization in the water column commences and phytoplankton δ15N becomes enriched (Möbius, 2013). This hypothesis explains the lack of resolution between epilimnetic phytoplankton and detritus in the mixing models. We used the δ15N and δ13C averages of all seasons in the models, which removed the monthly differences of the isotopic values. In the future, sampling phytoplankton and detritus 2 months before the initial fish sampling will help resolve differences in isotopic turnover between producers and consumers (Vander Zanden and Rasmussen, 2001; Lanari et al., 2021; Possamai et al., 2021) and provide the opportunity to apply the MixSIAR models using early and late season averages to better resolve pathways (as we did with Mysis and zooplankton models).
5. Conclusion
We used carbon and nitrogen stable isotopes and diet data to address how a biological invasion may disrupt ecosystem processes and linkages. By recognizing important trophic links in the ecosystem and retrieving the impacts of invasions in similar systems, we can predict the potential effects of a biological invasion in the system of our interest. Describing Lake Champlain’s food web structure and quantifying energy flow is important to elucidate the relative importance of bottom-up and top-down processes and the risk a quagga mussel invasion poses to the forage food web. While the impacts of quagga mussels on a system will be context-dependent, the ability of quagga mussels to sequester nutrients and productivity and consequently reduce the biomass of the spring bloom and DCL does not lead to a positive outlook for the Main Lake food web. To add complexity, a number of other species are expected to invade in the near future, adding further uncertainty to the stability of the food web. For example, another potential invader, round goby (Neogobius melanostomus; George et al., 2021), can forage on quagga mussels (Walsh et al., 2007), but their ability to sufficiently control quagga populations to mitigate the impacts on planktonic basal resources will depend on the timing and success of each invasion. Future work is needed to (1) better elucidate the relative importance of pelagic and benthic energy pathways with a third isotope, (2) determine whether Mysis will be able to shift and survive on a more benthic diet, and (3) determine whether lateral resource movement from the littoral zone could subsidize the pelagic food web after a quagga mussel invasion. However, our models provide fairly strong evidence that the mid-trophic food web in the Main Lake of Lake Champlain is at risk if quagga mussels invade, due to a combination of bottom-up and top-down pressures. The importance of the Main Lake as forage habitat relative to the Northeast Arm during the open-water season means some of the most important basal energy resources could be lost after invasion. This research can inform managers about which courses of action (e.g., reduce lake trout stocking) may best reduce the socio-ecological impacts of an invasion. Moreover, we demonstrate how simple stable isotope techniques can provide insights into the consequences of biological invasion, and thus replicated in other systems to address similar problems and predict how invasive species affect bottom-up and top-down effects.
Data availability statement
The raw data supporting the conclusions of this article will be made available by the authors, without undue reservation.
Ethics statement
This work was conducted with a Vermont state collectors permit using best practices as outlined in the “Guidelines for the Use of Fishes in Research” by the American Fisheries Society (https://fisheries.org/docs/wp/Guidelines-for-Use-of-Fishes.pdf). Vertebrates used in this research were dead when acquired and did not require an Institutional Animal Care and Use Committee protocol.
Author contributions
AC contributed to the data collection, managed laboratory processing and data, conducted the data analyses, and led manuscript development. BP contributed to data analysis and manuscript writing. MK analyzed samples for fatty acid and deuterium, and contributed to writing. JM and JS conceived the research, managed data collection, and contributed to manuscript writing. All authors made significant intellectual contributions to the manuscript.
Funding
This work was supported by funds made available for Lake Champlain Research by Senator Patrick Leahy through the Great Lakes Fishery Commission (Award Number 2018_MAR_95003).
Acknowledgments
We thank the captain and crew of the R/V Melosira, technicians Russell Dauksis, Cecilia Vichi, Katharina Winter, and Samuel Karl-Kaemmer, and undergraduate students Bethany Smith, Madeline Lerz, Danielle Berger, Amelia Koval, Grace Hemmelgarn, and Posy Labombard for their assistance in the field and lab. Members of the Stockwell and Marsden Laboratories provided valuable field and lab assistance, and feedback on the manuscript. Ben Marcy-Quay provided the map in Figure 1.
Conflict of interest
The authors declare that the research was conducted in the absence of any commercial or financial relationships that could be construed as a potential conflict of interest.
Publisher’s note
All claims expressed in this article are solely those of the authors and do not necessarily represent those of their affiliated organizations, or those of the publisher, the editors and the reviewers. Any product that may be evaluated in this article, or claim that may be made by its manufacturer, is not guaranteed or endorsed by the publisher.
Supplementary material
The Supplementary material for this article can be found online at: https://www.frontiersin.org/articles/10.3389/fevo.2022.1061636/full#supplementary-material
References
Ball, S. C., Mihuc, T. B., Myers, L. W., and Stockwell, J. D. (2015). Ten-fold decline in Mysis diluviana in Lake Champlain between 1975 and 2012. J. Great Lakes Res. 41, 502–509. doi: 10.1016/j.jglr.2015.03.002
Bastos, R. F., Corrêa, F., Winemiller, K. O., and Garcia, A. M. (2017). Are you what you eat? Effects of trophic discrimination factors on estimates of food assimilation and trophic position with a new estimation method. Ecol. Indic. 75, 234–241. doi: 10.1016/j.ecolind.2016.12.007
Brauns, M., Boëchat, I. G., de Carvalho, A. P. C., Graeber, D., Gücker, B., Mehner, T., et al. (2018). Consumer-resource stoichiometry as a predictor of trophic discrimination (Δ13C, Δ15N) in aquatic invertebrates. Freshw. Biol. 63, 1240–1249. doi: 10.1111/fwb.13129
Brown, C. J., Brett, M. T., Adame, M. F., Stewart-Koster, B., and Bunn, S. E. (2018). Quantifying learning in biotracer studies. Oecologia 187, 597–608. doi: 10.1007/s00442-018-4138-y
Bruel, R., Marsden, J. E., Pientka, B., Staats, N., Mihuc, T., and Stockwell, J. D. (2021). Rainbow smelt population responses to species invasions and change in environmental condition. J. Great Lakes Res. 47, 1171–1181. doi: 10.1016/j.jglr.2021.04.008
Caut, S., Angula, E., and Courchamp, F. (2009). Variation in discrimination factors (Δ15N and Δ13C): the effect of diet isotopic values and applications for diet reconstruction. J. Appl. Ecol. 46, 443–453. doi: 10.1111/j.1365-2664.2009.01620.x
Cecala, R. K., Mayer, C. M., Schulz, K. L., and Mills, E. L. (2008). Increased benthic algal primary production in response to the invasive zebra mussel (Dreissena polymorpha) in a productive ecosystem, Oneida Lake, New York. J. Integr. Plant. Biol. 50, 1452–1466. doi: 10.1111/j.1744-7909.2008.00755.x
Charles, H., and Dukes, J. S. (2007). “Impacts of invasive species on ecosystem services” in Biological Invasions. ed. W. Nentwig (Berlin, Heidelberg, Germany: Springer)
Croisetière, L., Hare, L., Tessier, A., and Cabana, G. (2009). Sulphur stable isotopes can distinguish trophic dependence on sediments and plankton in boreal lakes. Freshw. Biol. 54, 1006–1015. doi: 10.1111/j.1365-2427.2008.02147.x
David, P., Thebault, E., Anneville, O., Duyck, P. F., Chapuis, E., and Loeuille, N. (2017). Impacts of invasive species on food webs: a review of empirical data. Adv. Ecol. Res. 56, 1–60. doi: 10.1016/bs.aecr.2016.10.001
Denwood, M. J. (2016). Runjags: an R package providing interface utilities, model templates, parallel computing methods and additional distributions for MCMC models in JAGS. J. Stat. Softw. 71, 1–25. doi: 10.18637/jss.v071.i09
Dias, P. S., Cipro, C. V., Colabuonan, F. I., Taniguchi, S., and Montone, R. C. (2018). Persistent organic pollutants and stable isotopes in seabirds of the Rocas Atoll, equatorial Atlantic, Brazil. Mar. Ornithol. 46, 139–148.
Elrod, J. H., and OˈGorman, R. (1991). Diet of juvenile lake trout in southern Lake Ontario in relation to abundance and size of prey fishes, 1979–1987. Trans. Am. Fish. Soc. 120, 290–302. doi: 10.1577/1548-8659(1991)120<0290:DOJLTI>2.3.CO;2
Emery, K. A., Wilkinson, G. M., Ballard, F. G., and Pace, M. L. (2015). Use of allochthonous resources by zooplankton in reservoirs. Hydrobiologia 758, 257–269. doi: 10.1007/s10750-015-2338-6
Eppehimer, D. E., Bunnell, D. B., Armenio, P. M., Warner, D. M., Eaton, L. A., Wells, D. J., et al. (2019). Densities, diets, and growth rates of larval alewife and bloater in a changing Lake Michigan ecosystem. Trans. Am. Fish. Soc. 148, 755–770. doi: 10.1002/tafs.10171
Euclide, P. T., Flores, N., Wargo, M., Kilpatrick, C. W., and Marsden, J. E. (2018). Lack of genetic population structure of slimy sculpin in a large, fragmented lake. Ecol. Freshw. Fish 27, 699–709. doi: 10.1111/eff.12385
Euclide, P. T., Hansson, S., and Stockwell, J. D. (2017). Partial diel vertical migration in an omnivorous macroinvertebrate, Mysis diluviana. Hydrobiologia 787, 387–396. doi: 10.1007/s10750-016-2982-5
Euclide, P., Marsden, J. E., and Kilpatrick, W. C. (2019). Genetic structure of lake whitefish (Coregonus clupeaformis) in Lake Champlain, Vermont, 100 years after commercial fishery closure. J. Great Lakes Res. 45, 1310–1319. doi: 10.1016/j.jglr.2019.09.010
Euclide, P., Pientka, B., and Marsden, J. E. (2020). Genetic versus demographic stock structure of rainbow smelt in a large fragmented lake. J. Great Lakes Res. 46, 622–632. doi: 10.1016/j.jglr.2020.02.009
Fahnenstiel, G., Pothoven, S., Vanderploeg, H., Klarer, D., Nalepa, T., and Scavia, D. (2010). Recent changes in primary production and phytoplankton in the offshore region of southeastern Lake Michigan. J. Great Lakes Res. 36, 20–29. doi: 10.1016/j.jglr.2010.03.009
Flood, P. J., Duran, A., Barton, M., Mercado-Molina, A. E., and Trexler, J. C. (2020). Invasion impacts on functions and services of aquatic ecosystems. Hydrobiologia 847, 1571–1586. doi: 10.1007/s10750-020-04211-3
Francis, T. B., Schindler, D. E., Holtgrieve, G. W., Larson, E. R., Scheuerell, M. D., Semmens, B. X., et al. (2011). Habitat structure determines resource use by zooplankton in temperate lakes. Ecol. Lett. 14, 364–372. doi: 10.1111/j.1461-0248.2011.01597.x
Gamble, A. E., Hrabik, T. R., Stockwell, J. D., and Yule, D. L. (2011a). Trophic connections in Lake Superior part I: the offshore fish community. J. Great Lakes Res. 37, 541–549. doi: 10.1016/j.jglr.2011.06.003
Gamble, A. E., Hrabik, T. R., Stockwell, J. D., and Yule, D. L. (2011b). Trophic connections in Lake Superior part II: the nearshore fish community. J. Great Lakes Res. 37, 550–560. doi: 10.1016/j.jglr.2011.06.008
George, S. D., Baldigo, B. P., Rees, C. B., Bartron, M. L., and Winterhalter, D. (2021). Eastward expansion of round goby in New York: assessment of detection methods and current range. Trans. Am. Fish. Soc. 150, 258–273. doi: 10.1002/tafs.10290
Ginn, B. K., Bolton, R., Coulombe, D., Fleischaker, T., and Yerex, G. (2018). Quantifying a shift in benthic dominance from zebra (Dreissena polymorpha) to quagga (Dreissena rostriformis bugensis) mussels in a large, inland lake. J. Great Lakes Res. 44, 271–282. doi: 10.1016/j.jglr.2017.12.003
Hernández-Urcera, J., Carneiro, M. D. D., and Planas, M. (2022). Turnover rates and diet–tissue discrimination factors of nitrogen and carbon stable isotopes in seahorse Hippocampus reidi juveniles following a laboratory diet shift. Animals 12:1232. doi: 10.3390/ani12101232
Higgins, S. N., and Vander Zanden, M. J. (2010). What a difference a species makes: a meta–analysis of dreissenid mussel impacts on freshwater ecosystems. Ecol. Monogr. 80, 179–196. doi: 10.1890/09-1249.1
Hrycik, A. R., Simonin, P. W., Rudstam, L. G., Parrish, D. L., Pientka, B., and Mihuc, T. B. (2015). Mysis zooplanktivory in Lake Champlain: a bioenergetics analysis. J. Great Lakes Res. 41, 492–501. doi: 10.1016/j.jglr.2015.03.011
Hussey, N. E., MacNeil, M. A., McMeans, B. C., Olin, J. A., Dudley, S. F., Cliff, G., et al. (2014). Rescaling the trophic structure of marine food webs. Ecol. Lett. 17, 239–250. doi: 10.1111/ele.12226
Ishikawa, N. F., Chikaraishi, Y., Ohkouchi, N., Murakami, A. R., Tayasu, I., Togashi, H., et al. (2017). Integrated trophic position decreases in more diverse communities of stream food webs. Sci. Rep. 7:2130. doi: 10.1038/s41598-017-02155-8
Johannsson, O. E., Bowen, K. L., Holeck, K. T., and Walsh, M. G. (2011). Mysis diluviana population and cohort dynamics in Lake Ontario before and after the establishment of Dreissena spp., Cercopagis pengoi, and Bythotrephes longimanus. Can. J. Fish. Aquat. Sci. 68, 795–811. doi: 10.1139/f2011-028
Karatayev, A. Y., Burlakova, L. E., and Padilla, D. K. (2015). Zebra versus quagga mussels: a review of their spread, population dynamics, and ecosystem impacts. Hydrobiologia 746, 97–112. doi: 10.1007/s10750-014-1901-x
Keller, R. P., Drake, J. M., and Lodge, D. M. (2007). Fecundity as a basis for risk assessment of nonindigenous freshwater molluscs. Cons. Biol. 21, 191–200. doi: 10.1111/j.1523-1739.2006.00563.x
Kirn, R. A., and Labar, G. W. (1996). Growth and survival of rainbow smelt, and their role as prey for stocked salmonids in Lake Champlain. Trans. Am. Fish. Soc. 125, 87–96. doi: 10.1577/1548-8659(1996)125<0087:GASORS>2.3.CO;2
Knight, J. C., O’Malley, B. P., and Stockwell, J. D. (2018). Lake Champlain offshore benthic invertebrate community before and after zebra mussel invasion. J. Great Lakes Res. 44, 283–288. doi: 10.1016/j.jglr.2018.01.004
Labar, G. W. (1993). Use of bioenergetics models to predict the effect of increased lake trout predation on rainbow smelt following sea lamprey control. Trans. Am. Fish. Soc. 122, 942–950. doi: 10.1577/1548-8659(1993)122<0942:UOBMTP>2.3.CO;2
Lanari, M., Possamai, B., Garcia, A. M., and Copertino, M. (2021). Seasonal and El Niño southern oscillation-driven variations in isotopic and elemental patterns among estuarine primary producers: implications for ecological studies. Hydrobiologia 848, 593–611. doi: 10.1007/s10750-020-04462-0
Limburg, K. E., Luzadis, V. A., Ramsey, M., Schulz, K. L., and Mayer, C. M. (2010). The good, the bad, and the algae: perceiving ecosystem services and disservices generated by zebra and quagga mussels. J. Great Lakes Res. 36, 86–92. doi: 10.1016/j.jglr.2009.11.007
Madenjian, C. P., Hondorp, D. W., Desorcie, T. J., and Holuszko, J. D. (2005). Sculpin community dynamics in Lake Michigan. J. Great Lakes Res. 31, 267–276. doi: 10.1016/S0380-1330(05)70258-6
Malkin, S. Y., Silsbe, G. M., Smith, R. E. H., and Howell, T. (2012). A deep chlorophyll maximum nourishes benthic filter feeders in the coastal zone of a large clear lake. Limnol. Oceanogr. 57, 735–748. doi: 10.4319/lo.2012.57.3.0735
Marsden, J. E., and Hauser, M. (2009). Exotic species in Lake Champlain. J. Great Lakes Res. 35, 250–265. doi: 10.1016/j.jglr.2009.01.006
Marsden, J. E., Kozel, C. L., and Chipman, B. D. (2018). Recruitment of lake trout in Lake Champlain. J. Great Lakes Res. 44, 166–173. doi: 10.1016/j.jglr.2017.11.006
Marsden, J. E., and Langdon, R. W. (2012). The history and future of Lake Champlain’s fishes and fisheries. J. Great Lakes Res. 38, 19–34. doi: 10.1016/j.jglr.2011.09.007
Marsden, J. E., Schumacher, M. N., Wilkins, P. D., Marcy-Quay, B., Alger, B., Rokosz, K., et al. (2022). Diet differences between wild and stocked age-0 to age-3 lake trout indicate influence of early rearing environments. J. Great Lakes Res. 48, 782–789. doi: 10.1016/j.jglr.2022.02.004
Marsden, J. E., Stangel, P., and Shambaugh, A. D. (2013). “Influence of environmental factors on zebra mussel population expansion in Lake Champlain” in Quagga and Zebra Mussels: Biology, Impacts, and Control. eds. T. F. Nalepa and D. W. Schloesser (Boca Raton, FL: CRC Press), 1994–2010.
McMeans, B. C., McCann, K. S., Tunney, T. D., Fisk, A. T., Muir, A. M., Lester, N., et al. (2016). The adaptive capacity of lake food webs: from individuals to ecosystems. Ecol. Monogr. 86, 4–19. doi: 10.1890/15-0288.1
Metz, O., Temmen, A., von Oheimb, K. C. M., Albrecht, C., Schubert, P., and Wilke, T. (2018). Invader vs. invader: intra- and interspecific competition mechanisms in zebra and quagga mussels. Aquat. Invasions 13, 472–480. doi: 10.3391/ai.2018.13.4.05
Mida Hinderer, J. L., Jude, D. J., Schaeffer, J. S., Marner, D. M., and Scavia, D. (2012). Lipids and fatty acids of Mysis diluviana in lakes Michigan and Huron, 2008. J. Great Lakes Res. 38, 93–97. doi: 10.1016/j.jglr.2011.07.001
Mills, E. L., Chrisman, J. R., Baldwin, B., Owens, R. W., O’Gorman, R., Howell, T., et al. (1999). Changes in the dreissenid community in the lower Great Lakes with emphasis on southern Lake Ontario. J. Great Lakes Res. 25, 187–197. doi: 10.1016/S0380-1330(99)70727-6
Mills, E. L., Rosenberg, G., Spidle, A. P., Ludyanksu, M., Pligin, Y., and May, B. (1996). A review of the biology and ecology of the quagga mussel (Dreissena bugensis), a second species of freshwater dreissenid introduced to North America. Am. Zool. 36, 271–286. doi: 10.1093/icb/36.3.271
Möbius, J. (2013). Isotope fractionation during nitrogen remineralization (ammonification): implications for nitrogen isotope biogeochemistry. Geochim. Cosmochim. Acta 105, 422–432. doi: 10.1016/j.gca.2012.11.048
Nalepa, T. F., Fanslow, D. L., and Lang, G. (2009). Transformation of the offshore benthic community in Lake Michigan: recent shift from the native amphipod Diporeia spp. to the invasive mussel Dreissena rostriformis bugensis. Freshw. Biol. 54, 466–479. doi: 10.1111/j.1365-2427.2008.02123.x
Nalepa, T. F., and Schloesser, D. W. (Eds.) (2014). Quagga and Zebra Mussels: Biology, Impacts, and Control. CRC Press, Boca Raton, FL.
Naman, S. M., White, S. M., Bellmore, J. R., McHugh, P. A., Kaylor, M. J., Baxter, C. V., et al. (2022). Food web perspectives and methods for riverine fish conservation. WIREs Water 9:e1590. doi: 10.1002/wat2.1590
O’Malley, B. P., Dillon, R. A., Paddock, R. W., Hansson, S., and Stockwell, J. D. (2018). An underwater video system to assess abundance and behavior of epibenthic Mysis. Limnol. Oceanogr. Methods 16, 868–880. doi: 10.1002/lom3.10289
Oliveira, M. C. L. M., Mont Alverne, R., Sampaio, L. A., Tesser, M. B., Ramos, L. R. V., and Garcia, A. M. (2017). Elemental turnover rates and trophic discrimination in juvenile Lebranche mullet Mugil liza under experimental conditions. J. Fish Biol. 91, 1241–1249. doi: 10.1111/jfb.13408
O’Malley, B. P., and Stockwell, J. D. (2019). Diel feeding behavior in a partially migrant Mysis population: a benthic-pelagic comparison. Food Webs 20:e00117. doi: 10.1016/j.fooweb.2019.e00117
O’Reilly, C. M., Sharma, S., Gray, D. K., Hampton, S. E., Read, J. S., Rowley, R. J., et al. (2015). Rapid and highly variable warming of lake surface waters around the globe. Geophys. Res. Lett. 42, 10–773. doi: 10.1002/2015GL066235
Owens, R. W., and Bergstedt, R. A. (1994). Response of slimy sculpins to predation by juvenile lake trout in southern Lake Ontario. Trans. Am. Fish. Soc. 123, 28–36. doi: 10.1577/1548-8659(1994)123<0028:ROSSTP>2.3.CO;2
Ozersky, T., Barton, D. R., and Evans, D. O. (2011). Fourteen years of dreissenid presence in the rocky littoral zone of a large lake: effects on macroinvertebrate abundance and diversity. J. N. Am. Benth. Soc. 30, 913–922. doi: 10.1899/10-122.1
Pejchar, L., and Mooney, H. A. (2009). Invasive species, ecosystem services and human well-being. Trends in Ecol. Evol. 24, 497–504. doi: 10.1016/j.tree.2009.03.016
Possamai, B., Hoeinghaus, D. J., and Garcia, A. M. (2021). Shifting baselines: integrating ecological and isotopic time lags improves trophic position estimates in aquatic consumers. Mar. Ecol. Prog. Ser. 666, 19–30. doi: 10.3354/meps13682
Possamai, B., Hoeinghaus, D. J., Odebrecht, C., Abreu, P. C., Moraes, L. E., Santos, A. C., et al. (2020). Freshwater inflow variability affects the relative importance of allochthonous sources for estuarine fishes. Estuar. Coasts 43, 880–893. doi: 10.1007/s12237-019-00693-0
Post, D. M., Layman, C. A., Arrington, D. A., Takimoto, G., Quattrochi, J., and Montana, C. G. (2007). Getting to the fat of the matter: models, methods and assumptions for dealing with lipids in stable isotope analyses. Oecologia 152, 179–189. doi: 10.1007/s00442-006-0630-x
Pothoven, S. A., and Fahnenstiel, G. L. (2013). Recent change in summer chlorophyll a dynamics of southeastern Lake Michigan. J. Great Lakes Res. 39, 287–294. doi: 10.1016/j.jglr.2013.02.005
Pothoven, S. A., and Madenjian, C. P. (2008). Changes in consumption by alewives and lake whitefish after dreissenid mussel invasions in lakes Michigan and Huron. N. Am. J. Fish Manag. 28, 308–320. doi: 10.1577/M07-022.1
Pothoven, S. J., and Vanderploeg, H. A. (2022). Variable changes in zooplankton phenology associated with the disappearance of the spring phytoplankton bloom in Lake Michigan. Freshw. Biol. 67, 365–377. doi: 10.1111/fwb.13846
R Core Team (2022). R: A Language and Environment for Statistical Computing. Vienna, Austria: R Foundation for Statistical Computing.
Riccardi, A., Whoriskey, F. G., and Rasmussen, J. B. (1996). Impact of the Dreissena invasion on native unionid bivalves in the upper St. Lawrence River. Can. J. Fish. Aquat. Sci. 53, 1434–1444. doi: 10.1139/f96-068
Simonin, P. W., Parrish, D. L., Rudstam, L. G., Sullivan, P. J., and Pientka, B. (2012). Native rainbow smelt and nonnative alewife distribution related to temperature and light gradients in Lake Champlain. J. Great Lakes Res. 38, 115–122. doi: 10.1016/j.jglr.2011.06.002
Simonin, P. W., Rudstam, L. G., Parrish, D. L., Pientka, B., and Sullivan, P. J. (2018). Piscivore diet shifts and trophic level change after alewife establishment in Lake Champlain. Trans. Am. Fish. Soc. 147, 939–947. doi: 10.1002/tafs.10080
Skinner, M. M., Cross, B. K., and Moore, B. C. (2017). Estimating in situ isotopic turnover in rainbow trout (Oncorhynchus mykiss) muscle and liver tissue. J. Freshw. Ecol. 32, 209–217. doi: 10.1080/02705060.2016.1259127
Smeltzer, E., Shambaugh, A. D., and Stangel, P. (2012). Environmental change in Lake Champlain revealed by long-term monitoring. J. Great Lakes Res. 38, 6–18. doi: 10.1016/j.jglr.2012.01.002
Sousa, R., Guiterrez, J. L., and Aldridge, D. C. (2009). Non-indigenous invasive bivalves as ecosystem engineers. Biol. Invasions 11, 2367–2385. doi: 10.1007/s10530-009-9422-7
Stock, B. C., Jackson, A. L., Ward, E. J., Parnell, A. C., Phillips, D. L., and Semmens, B. X. (2018). Analyzing mixing systems using a new generation of Bayesian tracer mixing models. PeerJ 6:e5096. doi: 10.7717/peerj.5096
Stock, B. C., and Semmens, B. X. (2016). Unifying error structures in commonly used biotracer mixing models. Ecology 97, 2562–2569. doi: 10.1002/ecy.1517
Stockwell, J. D., O’Malley, B. P., Hanson, S., Chapina, R. J., Rudstam, L. G., and Weidel, B. C. (2020). Benthic habitat is an integral part of freshwater Mysis ecology. Freshw. Biol. 65, 1997–2009. doi: 10.1111/fwb.13594
Strayer, D. L., Adamovich, B. V., Adrian, R., Aldridge, D. C., Balohg, C., Burlakova, L. E., et al. (2019). Long-term population dynamics of dreissenid mussels (Dreissena polymorpha and D. rostriformis): a cross-system analysis. Ecosphere 10:e02701. doi: 10.1002/ecs2.2701
Strayer, D. L., Fischer, D. T., Hamilton, S. K., Malcolm, H. M., Pace, M. L., and Solomon, C. T. (2020). Long-term variability and density dependence in Hudson River Dreissena populations. Freshw. Biol. 65, 474–489. doi: 10.1111/fwb.13444
Vander Zanden, M. J., Olden, J. D., and Gratton, C. (2006). “Food-web approaches in restoration ecology” in Foundations of Restoration Ecology. eds. M. A. Palmer, J. B. Zedler, and D. A. Falk (Washington, DC: Island Press), 165–189.
Vander Zanden, M. J., and Rasmussen, J. B. (2001). Variation in δ15N and δ13C trophic fractionation: implications for aquatic food web studies. Limnol. Oceanogr. 46, 2061–2066. doi: 10.4319/lo.2001.46.8.2061
Vanderploeg, H. A., Liebig, J. R., Nalepa, T. F., Fahnenstiel, G., and Pothoven, S. A. (2010). Dreissena and the disappearance of the spring phytoplankton bloom in Lake Michigan. J. Great Lakes Res. 36, 50–59. doi: 10.1016/j.jglr.2010.04.005
Vuorio, K., Meili, M., and Sarvala, J. (2006). Taxon-specific variation in the stable isotopic signatures (δ13C and δ15N) of lake phytoplankton. Freshw. Biol. 51, 807–822. doi: 10.1111/j.1365-2427.2006.01529.x
Walsh, M. G., Dittman, D. E., and O’Gorman, R. (2007). Occurrence and food habits of the round goby in the profundal zone of southwestern Lake Ontario. J. Great Lakes Res. 33, 83–92. doi: 10.3394/0380-1330(2007)33[83:OAFHOT]2.0.CO;2
Ward, J. M., and Ricciardi, A. (2007). Impacts of Dreissena invasions on benthic macroinvertebrate communities: a meta-analysis. Diversity and Distrib. 13, 155–165. doi: 10.1111/j.1472-4642.2007.00336.x
Wilkins, P. D., and Marsden, J. E. (2021). Spatial and seasonal comparisons of growth of wild and stocked juvenile lake trout in Lake Champlain. J. Great Lakes Res. 47, 204–212. doi: 10.1016/j.jglr.2020.11.007
Keywords: dreissenid mussels, MixSIAR, alewife, rainbow smelt, Mysis, food webs
Citation: Chiapella A, Possamai B, Marsden JE, Kainz MJ and Stockwell JD (2023) Contrasting energy pathways suggest differing susceptibility of pelagic fishes to an invasive ecosystem engineer in a large lake system. Front. Ecol. Evol. 10:1061636. doi: 10.3389/fevo.2022.1061636
Edited by:
Rona A. R. McGill, University of Glasgow, United KingdomReviewed by:
Zachary Feiner, Wisconsin Department of Natural Resources, United StatesMichael Rennie, Lakehead University, Canada
Copyright © 2023 Chiapella, Possamai, Marsden, Kainz and Stockwell. This is an open-access article distributed under the terms of the Creative Commons Attribution License (CC BY). The use, distribution or reproduction in other forums is permitted, provided the original author(s) and the copyright owner(s) are credited and that the original publication in this journal is cited, in accordance with accepted academic practice. No use, distribution or reproduction is permitted which does not comply with these terms.
*Correspondence: Ariana Chiapella, ✉ ariana.chiapella@uvm.edu