- 1“Emil Racoviţă” Institute of Speleology, Cluj-Napoca, Romania
- 2Research Institute on Terrestrial Ecosystems (IRET-CNR), Florence, Italy
- 3National Biodiversity Future Center (NBFC), Palermo, Italy
Groundwater ecosystems from cold polar and circumpolar regions, hot springs, as well as those developed in salt, gypsum or in volcanic rocks are one of the environments considered to exhibit extreme environmental conditions such as low (below 0°C) or high (over 45°C) temperatures, hypersaline waters, or with elevated content of toxic gases like hydrogen sulfide or methane. They represent the “unseen ecosystem beneath our feet” and are inhabited by a large diversity of organisms, persisting and flourishing under severe environmental conditions that are usually hostile to the majority of organisms. These types of groundwater ecosystems are remarkable “evolutionary hotspots” that witnessed the adaptive radiation of morphologically and ecologically diverse species, whereas the organisms living here are good models to understand the evolutionary processes and historical factors involved in speciation and adaptation to severe environmental conditions. Here, we provide an overview of the groundwater invertebrates living in continental groundwater habitats prone to extreme environmental conditions in one or more physico-chemical parameters. Invertebrates are represented by a wide variety of taxonomic groups, however dominated by crustaceans that show specific adaptations mostly metabolic, physiologic, and behavioral. Symbiotic associations among bacteria and invertebrates are also discussed enlightening this biological interaction as a potential adaptation of different groundwater invertebrates to cope with severe environmental conditions. Given the high pressures that anthropogenic activities pose on groundwater habitats worldwide, we predict that several of these highly specialized organisms will be prone to extinction in the near future. Finally, we highlight the knowledge gaps and future research approaches in these particular groundwater ecosystems by using integrative-omic studies besides the molecular approach to shed light on genetic variation and phenotypic plasticity at species and populational levels.
Introduction
There are continuous challenges to explore the groundwater ecosystems in the most remote places on Earth (i.e., aquifers from Greenland or Antarctica) and to discover new and more extreme habitats for organisms. The frontier environmental conditions for life forms on Earth are broad, encompassing extreme ranges of parameters such as temperature, salinity, toxic elements (hydrogen sulfide, methane, etc.), oxygen content, redox state and nutrient limitation (Maruyama et al., 2019). However, extreme condition is a term rather misleadingly used to define an environment where organisms live. Such “extremophile” species are highly adapted to certain conditions considered extreme for the humans or other organisms’ point of view. Extremophiles are treasures for molecular biology and functional diversity and play an essential role in understanding the origin of life and evolutionary changes through time. Life appeared in extreme conditions in the marine environment from the Palaeozoic (541 mil. Years ago) and these organisms are likely the most ancient life forms on Earth (Merino et al., 2019). They can be broadly classified into two categories: (i) species that require one or more extreme conditions to grow and (ii) extremotolerant species that can tolerate harsh conditions but actually live in milder environments (Buzzini et al., 2018).
One of the environments which exhibit extreme conditions in one or more physical and chemical parameters is groundwater (GW). Present almost everywhere belowground, GW is an invisible component of the hydrosphere and the water cycle, accounting for about 30.1% of the available freshwater on Earth and about 90% if glaciers are excluded (Griebler and Avramov, 2015). It represents the hidden part of aquatic ecosystems, lying from a few meters below ground down to considerable depths (more than 2 km deep; Schmidt and Hahn, 2012). Groundwater represents a unique ecosystem supporting aquatic life (Howarth and Moldovan, 2018). Beyond the darkness, low oxygen concentration, scarcity of nutrients and reduced interstitial spaces for living, GW can have supplementary extreme environmental conditions (Gibert et al., 1994). It is the case of GW in cold polar and circumpolar regions where the water temperature is constantly around 0°C, as well as thermal springs where the water temperature can reach over 50°C. Groundwater flowing in lava tubes and volcanic caves with fumarolic activity, where gases such as sulfur (SO2), carbon dioxide (CO2), chlorine (Cl2) and fluorine (F2) escape from cracks, is also an extreme habitat for many species. Similarly, are also the continental sulfidic groundwaters where hydrogen sulfide (H2S), methane (CH4) and ammonia (NH3) reach elevated concentrations and oxygen content is extremely low down to hypoxia (< 2 mg/L) and even anoxia (Sarbu, 2000; Engel et al., 2003). Altogether, these parameters are considered restrictive factors for the presence of life and are likely to have a direct impact on biodiversity and species adaptation (Howarth and Moldovan, 2018).
The GW ecosystems showing extreme environmental conditions support original biological communities, especially bacteria, bacteria-like organisms, protozoans, viruses, and fungi (Griebler and Lueders, 2009). However, species from distinct invertebrate groups (i.e., ice worms, rotifers, nematodes, oligochaetes, and crustaceans) (Sarbu, 2000; Dattagupta et al., 2009; Flot et al., 2014; Howarth and Moldovan, 2018) have adapted physiologically, metabolically, and behavioral to live in environments which are unlivable for most life forms.
Groundwater-adapted invertebrates are termed stygobites (sensu Gibert et al., 1994). The name stygobites is derived from “bios,” the Greek word for “life,” and “stygo,” the mythological river Styx, on which the decedents go to the underworld (Thulin and Juergen, 2008). Stygobites spend their whole life cycle underground, being adapted to the scarcity of food and low oxygen concentration, and showing a low metabolic rate and several other physiological functions working in “slow motion” (Hancock et al., 2005; Engel, 2007). They are the most likely to live and flourish in more severe conditions provided by some GW, having already a functional background to struggle with environmental difficulties. However, several stygobite species are stenotherm used to live in constant conditions provided by GW, making them vulnerable even when slight changes in some environmental parameters appear. Another category of GW species – stygophilous, occupies a wide variety of habitats both underground and in surface waters; or are strictly surface-dwelling species termed stygoxenes, that are occasionally carried out passively from the surface. However, unlike stygobites, stygoxenes and partially the stygofiles, are more vulnerable to food and oxygen deprivation being more energetic consumers and with a more intense metabolic rate in comparison with the stygobites (Gibert et al., 1994; Thulin and Juergen, 2008).
Different phyla of invertebrates inhabit the GW’s pore spaces and fissures and resulting in diverse biological communities with high levels of endemism (Sarbu et al., 1996; Thulin and Juergen, 2008; Tobler et al., 2016). However, crustaceans (amphipods, decapods, copepods, ostracods, isopods, harpacticoids) are particularly rich and diverse and dominate the GW in general, but also those underground habitats with life-threatening environmental conditions (De Jong et al., 2005; Engel, 2007; Thulin and Juergen, 2008; Wilkens et al., 2009; Papi and Pipan, 2011; Por et al., 2013; Greenway et al., 2014; Mulec et al., 2014; Galassi et al., 2016; Khalaji-Pirbalouty et al., 2018; Borko et al., 2019; Fatemi et al., 2019; Negus et al., 2020; Popa et al., 2020; Brad et al., 2021).
Groundwater species are generally prone to dual threats, on one side the impact of anthropogenic activities (e.g., excessive GW pumping, contaminants: pharmaceuticals, pesticides, fertilizers, plastics, personal care products, etc.), and the effects of global climate change on the other, mostly related to rising temperatures, reductions in precipitation levels and water scarcity (Di Lorenzo et al., 2019, 2020; Castaño-Sánchez et al., 2020; Yu et al., 2020). GW pollution is extremely complex, frequently challenging to detect, and has long-lasting effects. Groundwaters’ stable environmental factors, such as their constant temperature, lack of light, oligotrophy, and low redox potential, lead to less biotic and photolytic pollutant breakdown and, as a result, greater chemical persistence than in surface waters (Di Lorenzo et al., 2019). When compared to surface water, GW is more difficult to recover from pollution (Yu et al., 2020). The response of the highly adapted GW species to contaminants is wide-ranging, including morphological malformations, physiological changes, lowering reproductive capacity, death, migration, and/or local extinction (Goretti et al., 2019; Di Lorenzo et al., 2020). Since GW ecosystems are known for their thermal stability, it is expected that global warming will also have a significant impact on the invertebrate communities, which will likely result in an increase in their metabolic rates, food requirements, and oxygen demands (Addo-Bediako et al., 2000; Di Lorenzo and Galassi, 2017).
In this review, we provide a synthesis of invertebrates from GW ecosystems prone to extreme environmental conditions. We focus our review on continental habitats, covering GW in glacierized landscape and ice caves, thermal springs, lava tubes and volcanic caves, and sulfidic aquifers (Figure 1). There is a good knowledge of GW invertebrates worldwide with several hotspots identified, mainly caves (Culver et al., 2021). However, there has been considerably less focus on species from GW habitats showing extreme conditions in one or more environmental parameters. Understanding the environment and habitats where they live will provide a valuable framework to comprehend how these ecosystems function, the evolutionary pathways of species able to live here, their adaptive mechanisms adopted and how they are able to survive facing extreme external factors.
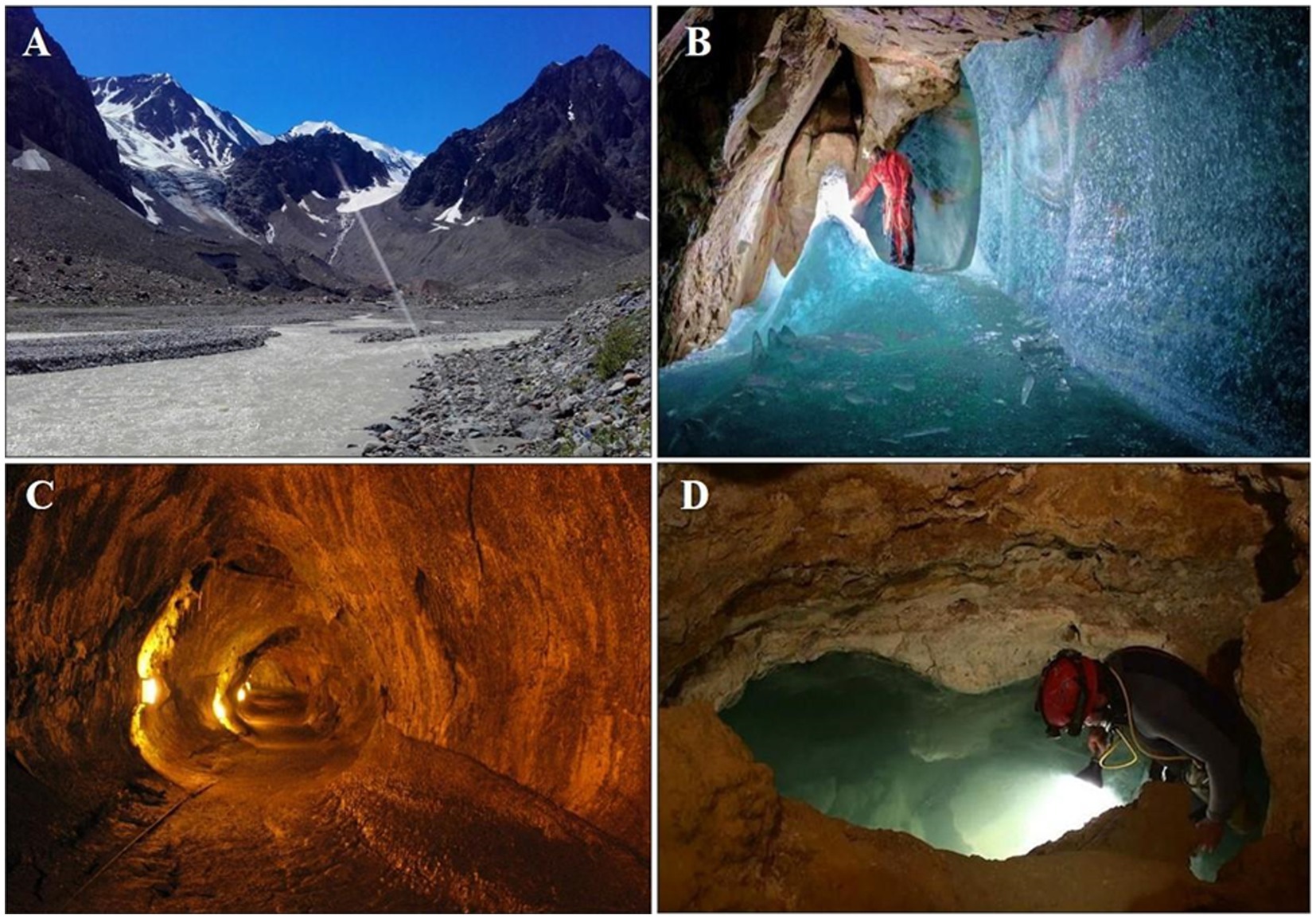
Figure 1. The diversity of groundwater habitats prone to extreme environmental conditions around the globe. (A) Aktru River, Siberia, Russia. (B) Veronica Ice Cave, Picos de Europa, Spain (Photo: Bernard Hivert). (C) Lava tube in Hawaii (Photo: D. Boyle). (D) Movile Cave, Romania (Photo: Mihai Baciu).
Living below ice
Hyporheic zone of glacier feed streams/rivers and ice caves
Groundwater in glacierized landscapes is present in glaciofluvial sand and gravel deposits (i.e., eskers) in polar regions in the Northern (Knutsson, 2008) and Southern Hemispheres (Fraser et al., 2018), as well as within the hyporheic zone (HZ) of glacier-fed rivers (Jacobsen et al., 2012; Khamis et al., 2016) and ice caves from Arctic, Antarctic, and Alpine regions (Iepure, 2018).
The eskers are one of the most common aquifers in the Northern Hemisphere (Thulin and Juergen, 2008). They can be 10–100 m thick, with an extension of 1,000–5,000 m, and continuous over long distances (100 km or more; Knutsson, 2008). The bedrock of these aquifers is dominated by crystalline (Kola Peninsula in Russia) and volcanic rocks (Iceland, Faeroe Island, Spitsbergen and Greenland), whereas the limestone is rare (Knutsson, 2008).
The Northern Hemisphere was completely covered by ice sheets during the Pleistocene glaciations affecting the survival of GW fauna (Hewitt, 2000; McInerney et al., 2014). The recurrent glaciations not only influenced the GW movements and the porosity and permeability of aquifers over time but they also significantly contributed to slowing down the GW colonization from the surface. It is hypothesized that the surface populations got extinct under the Climate Relict Hypothesis (CRH). However, adaptive shifts significantly contributed to GW speciation through parapatry (Culver and Pipan, 2019). At present, the permafrost causes permanent pore-GW freezing conditions; except for the upper active layers that melt seasonally, the thin films around the sediment grains represent the most appropriate GW habitat and a pathway for pre-adapted species to enter underground (Thulin and Juergen, 2008; McInerney et al., 2014). In glaciated regions, the constant low temperature close to, or well below, 0°C still represents the most limiting factor for GW fauna (Thulin and Juergen, 2008).
Glacierized catchments host another challenging GW habitat for invertebrate fauna located at the groundwater/surface water interface – the hyporheic zone (HZ) of glacier-feed rivers and streams (Milner et al., 2001a,b, 2008; Jacobsen et al., 2012; Figure 1A). The HZ is an ecotone zone and is defined as the region beneath and next to rivers and streams where surface and GW intermix (Boulton et al., 2008). It controls the water exchanges between surface and groundwater, ensures the cycling of carbon, energy, and nutrients, and provides a particular habitat and refuge for benthic and hyporheic invertebrates during harsh environmental conditions at the surface (Danielopol, 1989; Malard et al., 2006; Dole-Olivier et al., 2009; Iepure et al., 2013, 2022).
Glacier-fed rivers and streams are known from the Arctic up to Alpine areas across Europe (Brittain and Milner, 2001), the paramo in Central America (Cauvy-Fraunié et al., 2016), New Zealand (Milner et al., 2001a,b), Greenland (Friberg et al., 2001), Siberia (Volkov et al., 2021), Tibet (Molden et al., 2022), and Alaska (Milner et al., 2008). The Arctic and Antarctic rivers and streams have a reduced HZ, usually less than 1 m deep in permafrost, and laterally it develops up to a few meters, whereas the HZ of streams in glacierized landscapes from temperate regions extends to more than 1 m on vertical and lateral dimensions (Malard et al., 2006; Milner et al., 2008; Hansson et al., 2012).
Although the HZ is characterized by a suite of restrictive factors for invertebrates (i.e., instability of the riverbed, high content of suspended sediments that clog the interstitial pore-spaces, oligotrophic waters–low abundance and bioavailability of organic matter), the water temperature remains the most constraining parameter. In Polar Regions, the permafrost keeps the hyporheic water temperature around 0°C throughout the year (Milner et al., 2017). Conversely, in the Alpine regions, the water temperature is below zero only during winter and usually the hyporheic water is, on average, 0.7°C warmer than surface channel water (Malard et al., 2001).
Ice caves are one of the most challenging glacial environments for life (Figure 1B; Iepure, 2018). These caves are known from all climatic regions, including temperate (in the Alps, the Carpathians in Europe, Ural in Russia, Pamir in Tien-Shan and Himalaya in Asia), tropical (Cordillera Andina), Mediterranean (the Pyrenees in Spain and France, Olympus in Greece, Erciyes in Turkey) and Picos de Europa in Spain polar regions (Islands and Antarctica (Erebus Mts., Ross Island) (Persoiu and Lauritzen, 2018). Ice caves can have different origins and they can be found in karst, tectonic and volcanic rocks or can be present in permafrost (Mavlyudov, 2018; Glass et al., 2021). We are treating here only the ice caves developed in karst terrains from where GW invertebrates are usually studied (Iepure, 2018). Antarctic ice caves are mentioned in the context of identification of specific groups of GW invertebrates from sediments through eDNA metabarcoding (Fraser et al., 2018).
Ice caves from karst regions are present in the latitudinal band stretching between 19–80°N and from 30 m a.s.l. in Svalbard to 3,350 m on Mount Alberta (Canada) (Yonge, 2004; Persoiu and Lauritzen, 2018). Specific cryogenic habitats are the ice pools formed by the accumulation of percolating water from the ceiling on the ice floor or ice formations (stalagmites), beside the typical cave habitats in periglacial sectors (i.e., fissures, cracks, micro-fissures, temporary or permanent pools, and subterranean rivers and lakes (Iepure, 2018).
The variability in temperature, ranging from 0°C down to −14°C, particularly characterizes the environmental conditions in ice caves (Persoiu et al., 2011). The presence of perennial ice inside galleries causes a drop in the air temperature extending from the cave entrance to a few hundred meters, resulting in the freezing of drip water and the formation of speleothems with short or long life (Persoiu and Onac, 2012; Persoiu and Lauritzen, 2018).
Groundwater invertebrates below ice
GW habitats from glacierized catchments host relatively simple communities from different phyla, including rotifers, tardigrades, annelids, insects and crustaceans (amphipods, copepods, ostracods, isopods, and syncarids; Milner et al., 2017; Iepure, 2018; Camacho et al., 2020). In these habitats, the food webs are short with reduced trophic levels; the trophic chain is sustained by low primary productivity and oligotrophic waters, being so far known to be entirely dependent on surface allochthonous input (Hillebrand-Voiculescu et al., 2014; Iepure, 2018; Paun et al., 2019).
Several species from formerly Pliocene and Pleistocene glaciated areas are old colonizers of these GW and they are supposed to survive in the so-called subglacial refugia such are nunataks (Kristjánsson and Svavarsson, 2007; Thulin and Juergen, 2008; Dumnicka et al., 2020). However, not all surviving species can be considered true psychrophiles – freeze-tolerant species that survive ice formation in tissues, since some of them are not tolerant to cold waters but they likely survive these climatic events in GW flowing through the porous lava tubes beneath the glaciers.
Crustacean amphipod species like Crangonyx islandicus (Svavarsson and Kristjánsson, 2006) and Crymostygius thingvallensis (Kristjánsson and Svavarsson, 2004) (belonging to an endemic family) are one of the first stygobites – true GW dwellers (cf. Gibert et al., 1994), found in eskers of Island and springs. These waters flow from lava fields in the geologically youngest parts of the country, about 10,000 years old (Thulin and Juergen, 2008). Ireland hosts several species of amphipods, i.e., Niphargus wexfordensis G. Karaman, Gledhill and Holmes, 1994, Microniphargus leruthi Schellenberg, 1934, Niphargus kochianus irlandicus Schellenberg, 1932; syncarids such as Antrobathynella stammeri (Jakobi, 1954), and ostracods such as Fabaeformiscandona breuili (Paris, 1920). The formerly glaciated areas of northern Germany, Poland and Belarus host a relatively rich assemblage of cold-tolerant species comprising small species of copepods such as Parastenocaris sp., archiannelids such as Troglochaetus beranecki Delachaux, 1921 and mites, but also some Crangonyctidae amphipods (Figure 2; Kunz, 1938; Dumnicka et al., 2020). Recently a new genus and a new species of Bathynellidae, Altainella calcarata (Camacho et al., 2020) has been described from the hyporheic zone of the glacier-feed river Aktru in Altai Mountain (Russia) (Camacho et al., 2020).
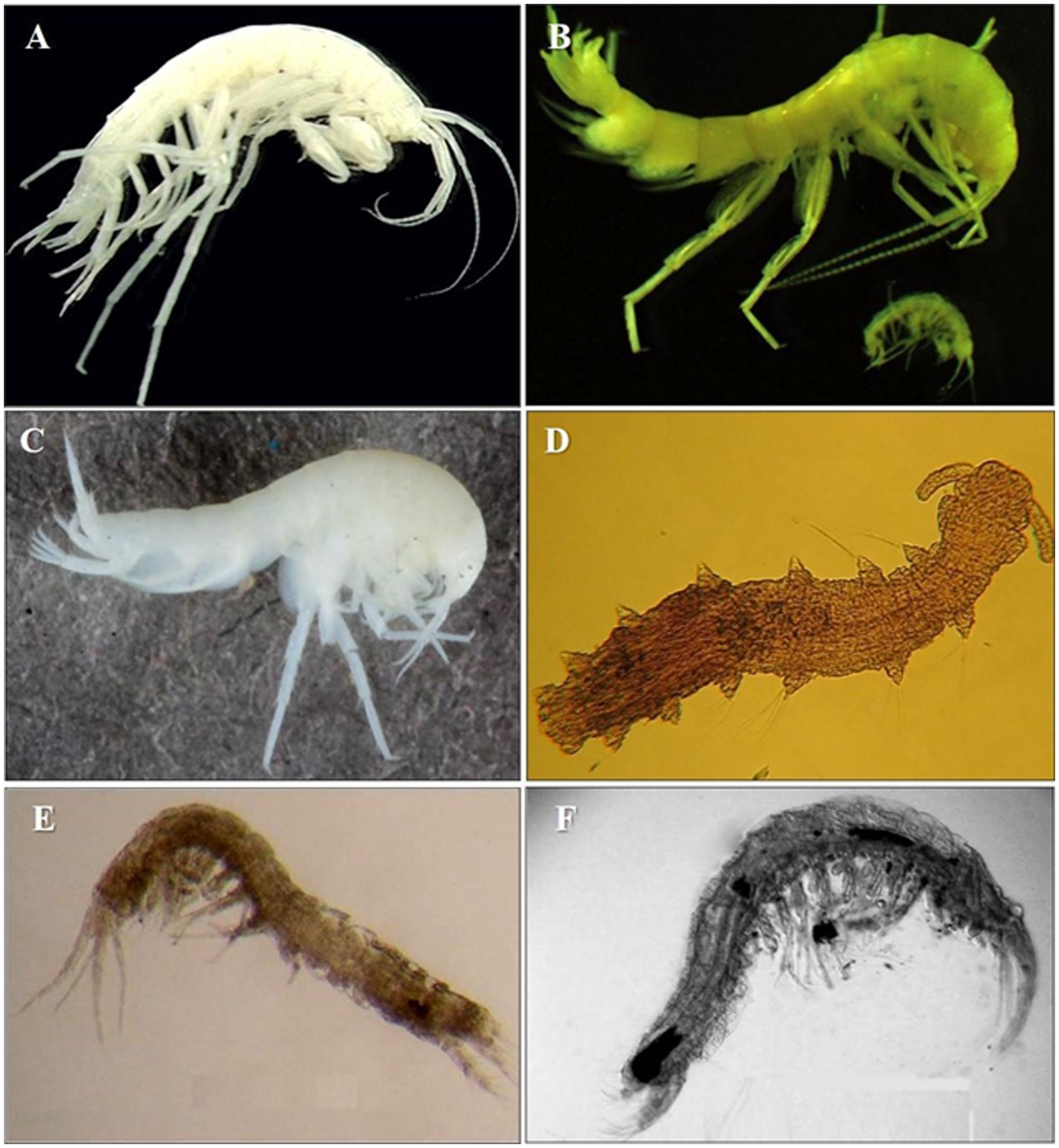
Figure 2. Ice caves and sub-glacial aquifers invertebrates’ biodiversity. (A) Amphipod Crymostygius thingvallensis found in eskers and springs of Island (Photo from Kristjánsson and Svavarsson, 2004). (B) Amphipod Stygobromus allegheniensis from Sam’s Point Ice Cave, United States (Photo: Amy Cahill). (C) Amphipod Niphargus rhenorhodanensis a European cold adapted species (downloaded from https://boldsystems.org/ ). (D) Polychaet Troglochaetus beranecki. (E) Syncarid Antrobathynella stammeri from United Kingdom (Photo: Ana Camacho). (F) Syncarid Vejdovskybathynella edelweiss from caves in northern Spain (Photo: Ana Camacho).
Several stygobitic endemic species persisted in the GW below ice sheets during glacial events, i.e., Riss-Würm glaciation in the Alpine region (including Jura, the Pyrenees, Alps and partially the Carpathians Mountains; Holsinger, 1981; Castellarin et al., 2005). Cold – tolerant stygobites were found in karstic aquifers and glaciofluvial sediments of the HZ of Alpine rivers in Austria, France, Italy, Slovenia, and Switzerland (Malard et al. 1999, 2001; Griebler and Möslacher, 2003; Castellarin et al., 2005).
Similarly, to the previous GW habitats from glacierized systems, Alpine ice caves host cold-tolerant species that push their physiological and behavioral limits to live at temperatures <0°C and avoid the thermal stress by adopting specific strategies (e.g., Iepure, 2018). The crustacean cyclopoid Speocyclops infernus (Kiefer, 1930) and the harpacticoid Bryocamptus sp. have been found to live in the epikarst zone of Snežna Ice Cave in Slovenia (Papi and Pipan, 2011). The harpacticoids Arcticocamptus cuspidatus var. ekman (Schmeil, 1893) and Elaphoidella sp., and the syncarid Bathynella natans Vejdovský, 1882 were found in pools on ice in Dobšinská Ice Cave (Slovakia; Birstein and Ljovuschkin,1967; Schminke, 1986; Camacho, 2006; Camacho and Valdecasas, 2008; Juberthie et al., 2016).
In Siberia, although being a white spot related to the knowledge of GW fauna, species of amphipods, such as Stygobromus pusillus (Holsinger, 1987), have been reported from glaciated parts. Several species of Crangonyx are endemics from ice caves in Russia, being associated with the cold waters of Kungur Ice Cave (Ural Mountains) and Ledjanaka Ice Cave (Samara basin). Crangonyx chlebinkovi ssp. maximovitchi Pankov and Pankova, 2004, first discovered in Kungur Ice Cave, has been later on found in other GW habitats (caves, wells, small springs, seeps) in the Priuralye karst area (Ural Mountains). The individuals of C. chlebinkovi ssp. maximovitchi seem to live under constant low water temperatures (0 to 5.5°C; Decu et al., 2019). The only syncarid species known here is Bathynella glacialis (Birstein and Ljovuschkin, 1967), which was found in Khabarovsk Ice Cave (Russia; Birstein and Ljovuschkin, 1967).
In northern America, species of Stygobromus, i.e., S. canadensis Holsinger, 1980; S. secundus Bousfield and Holsinger, 1981 and Bactrurus (amphipods), as well as several crustacean isopods (Salmasellus steganothrix Bowman, 1975) have been found to survive in subglacial refugia below ice sheet from Columbia Icefield in the Canadian Rocky Mountains (Bowman, 1975). Diacyclops crassicaudis brachycercus (Kiefer, 1927), a crustacean cyclopoid species, has been found in ponds and streams in Woodville Ice Cave, Nova Scotia (Moseley, 2007).
Adaptations of groundwater invertebrates to cold water
Groundwater from glacierized environments hosts a relatively large array of ectothermic (cold-blooded) organisms, termed psychrophiles, that are cold-tolerant species able to live and reproduce at low temperatures ranging from −20°C to +10°C (Iepure, 2018). These species can be divided into two groups according to the strategies they adopt to cope with thermal stress and survive at low temperatures through biochemical, physiological and metabolic processes: 1) freeze-tolerant species that survive ice formation in tissues (psychrophiles or cryophiles) and 2) freeze-avoiding organisms that tolerate low temperature but not the crystallization of the body fluids (Colson-Proch et al., 2010; Castaño-Sánchez et al., 2020; Di Lorenzo et al., 2020; Simčič and Sket, 2021).
Ice caves from the United States and Canada host several species of amphipods adapted to cold waters (Moseley, 2007). Stygobromus allegheniensis (Holsinger, 1967) is a large species fully depigmented and eyeless that has been reported from Sam’s Point Ice Cave (United States; Cahill et al., 2015) and ice caves located in the Shawangunk Ridge, New York (Espinasa et al., 2015). In Sam’s Point Ice Cave, S. allegheniensis inhabits pools that freeze only at the surface; the individual organisms likely remain at the bottom of the pool to avoid being frozen. The metabolism of S. allegheniensis seems to be adjusted to survive at low temperatures, in a similar way to the European species Niphargus rhenorhodanensis Schellenberg, 1937, a cold-adapted amphipod that accumulates cryoprotective molecules such as glycerol and free amino acids to endure extreme thermal conditions (Issartel et al., 2006). Laboratory experiments indicated that the amphipod Niphargus rhenorhodanensis can survive below 0°C for more than 60 days by accumulating large amounts of cryoprotectants (e.g., polyols, sugars, free amino acids, and antifreeze proteins) (Issartel et al., 2006; Ramløv and Friis, 2020). More specifically, this species contains an elevated concentration of trehalose (a sugar functioning as a cryoprotectant at low temperatures), as well as amino acids such as glutamine proline, alanine, lysine, and glycine acting as enzyme- stabilizers to maintain their activity (Issartel et al., 2006). It is assumed that this ability is a relict adaptation dating back to Quaternary glaciations when the temperature was around or below 0°C not only at the surface but in subterranean environments as well (Tweed et al., 2005). Stygobromus canadensis (Holsinger, 1980) is another cold-tolerant amphipod dweller of Castleguard Cave in Canada that has been found in a series of pools located at about 2 km inside the cave (Holsinger et al., 1983). The cave also hosts an asellid isopod, Salmasellus steganothrix Bowman, 1975, abundant in pools rich in sediments (Holsinger et al., 1983).
Living in hot waters
Thermal and hot springs
The springs, natural windows to the subterranean world, are considered ecological islands because of their irregular distribution and isolation from each other, making them fascinating habitats to investigate biological communities (Fattorini et al., 2016). Thermal springs associated with the geothermal activity are extreme environments located all over the world, although they are most common in areas of senescent or inactive volcanic activity (Pritchard, 1991). However, there is a second type of thermal spring unrelated to volcanism. In this case, the meteoric waters from rain and snow have heightened temperatures at depth, due to the geothermal gradient, after sinking into the ground through rock fissures and pores (Stackebrandt, 2001).
The definition of thermal springs is not consistent around the globe. In Europe, they are defined as having a temperature of more than 20°C (Warning et al., 1965), whereas, in the United States, they are described as having a temperature of at least 9°C higher than the mean annual temperature of the air in their geographic region (Warning et al., 1965). Some springs that do not freeze in winter due to natural protective circumstances can also be described as “thermal.” In places where the mean annual air temperature is low or in tropical regions, some springs that are just a few degrees warmer than the air temperature may be classified as “thermal” (Warning et al., 1965). However, the high temperature in springs can be either thermal (i.e., they have higher temperatures than the mean annual air temperature), or hot (i.e., they have a lower temperature limit of 36.7°C, the average human body temperature) (Glazier, 2012; Negus et al., 2020).
Geothermal springs are often highly stable ecosystems, with unique ecology and little variation in environmental variables like temperature and chemical composition (e.g., pH, conductivity, ionic composition, nutrient composition, oxygen concentration) over time (Szczucinska and Wasielewsk, 2013). The temperature of these springs varies widely (hot, warm, tepid, or boiling water), as does their discharge rate (Brues, 1927). The heat source could be explained either by the association of thermal water with volcanic rocks or the chemical processes beneath, such as the oxidation of iron pyrite and a few other minerals or the breakdown of radioactive elements (Warning et al., 1965).
Thermal waters have variable chemistry as they flow through underground rock channels, and their discharges generate temperature gradients and chemical precipitates that support a wide range of microbial populations while encasing their remains (Shock et al., 2005; Des Marais and Walter, 2019). As a result, hot spring systems contain a rich record of fossils, from bacteria to trees and macroscopic organisms. Thus, they are considered potential cradles for life’s genesis on Earth and maybe on other planets (Des Marais and Walter, 2019).
The chemistry of hot spring water varies from severely acidic (pH as low as 0.2) to very alkaline (pH = 11), and different organisms flourish at specific pH levels (Dodds and Whiles, 2010). Another typical feature of thermal springs, especially those at extremely high temperatures, is the reduced quantity of dissolved oxygen (Brues, 1924; Brues, 1927).
Hydrothermal springs can be classified into four major groups depending on their principal anion-based compositions (Drake et al., 2014; Des Marais and Walter, 2019):
(a) The alkaline chloride springs with an almost neutral pH are formed when GW and volcanic gases (e.g., CO2, H2S) mix with silicate rocks. When these fluids cool, they usually create siliceous sinter deposits.
(b) The acid-sulfate springs with pH values typically <4 are formed when rising H2S is oxidized to H2SO4, which combines with adjacent rocks, forming alteration compounds such as clays, oxides, and silica residue.
(c) Bicarbonate-rich springs often create CaCO3 deposits (thermogene travertine), when the ascending CO2 is of volcanic origin and dissolves the carbonatic rocks.
(d) Iron-rich springs support microbial populations and produce an abundance of flocculant iron-bearing deposits.
Groundwater invertebrates in thermal and hot springs
Even when there is no light, or the temperatures are too high, life flourishes in thermal and hot springs (e.g., photosynthetic blue-green algae live at temperatures >74°C, while non-photosynthetic bacteria flourish at ~95°C; Castenholz, 1969). The biota of thermal and hot springs is characterized by very few species. The assemblages become less diversified as the temperature rises, while certain organisms, such as cyanobacteria, prosper at warm temperatures (30–40°C; Dodds and Whiles, 2010).
Warming contributes to the simplification of food web architecture and the reduction of energy fluxes between consumers and producers (Glazier, 2012; O’Gorman et al., 2019). Temperature stability promotes a reduction in available ecological niches, which is one of the reasons for the taxonomic simplicity of thermal and hot springs (Pritchard, 1991).
The water chemistry of most thermal and hot springs is not a significant limiting factor for invertebrates (Lamberti and Resh, 1985). However, the high-water temperature is a significant constraining factor because organisms generally cannot withstand temperatures >50°C and only a few can survive at temperatures above 40°C because of the low levels of oxygen and increased oxygen consumption (Pritchard, 1991). However, high temperature and water chemistry may rule out the most sensitive species in springs, and even whole orders of invertebrates, acting either directly or indirectly through competitive interactions (Pritchard, 1991; Dodds and Whiles, 2010). The distribution of species in these springs is controlled by disparate factors, including the temperature of the source, the distance from the source, the turbulence of the stream flow, the flow speed, the elevation, the intake of water from other sources, the food availability, and the photosynthesis presence (Mason, 1939; Pritchard, 1991).
Thermal and hot springs are among the harshest environments for freshwater invertebrates (Poinar, 2015; Thorp and Covich, 2015). Metazoan life is rarely conceivable in the upwelling zone of these types of springs. However, when ambient temperatures reach 40°C or lower in lateral channels and pools, as well as in locations further downstream, circumstances become acceptable for some invertebrates (Lamberti and Resh, 1985). Aquatic invertebrates are strongly related to this water temperature gradient (40–42°C), which has been identified as a critical barrier to their distributions (Negus et al., 2020).
The metazoans in thermal and hot springs include a few species of ostracods, nematodes, oligochaetes, aquatic coleopterans, water mites, dipterans, odonates, ephemeropterans, and a few other crustaceans such as copepods and cladocerans (De Jong et al., 2005; Derso et al., 2015; Thorp and Covich, 2015; Negus et al., 2020). The aquatic invertebrate groups can show distinct seasonal variations in abundances due to life history traits such as hatching, emergence, resting stages, or end of dormancy. Aquatic insects with an aerial dispersal stage (e.g., dipterans) dominate the invertebrate assemblages in spring and summer, whilst fully aquatic taxa (e.g., ostracods, copepods, aquatic coleopterans) are substantially more abundant and frequent during the winter months (Kreiling et al., 2020).
Thermophilic ostracods have previously been discovered in hot springs with temperatures as high as 50°C (e.g., hot springs in central Idaho and southcentral Oregon United States, or in Australia) and frequently reported in travertine deposits as fossils (Wickstrom and Castenholz, 1985; De Jong et al., 2005; Negus et al., 2020). The ostracod species of the genus Potamocypris collected from a hot stream in southcentral Oregon, United States (35°C–48°C) are involved in grazing activities on microbial mats, regulating the structure of cyanobacteria communities (Wickstrom and Castenholz, 1985). In the Talaroo spring complex, Australia, thermophilic ostracods were spotted in the hottest parts of bare travertine, feasting on insects that had died in the hot water (Negus et al., 2020). Chironomidae and Hydrobiidae species were also reported at temperatures of 51–52°C in two hot spring sampling sites in Ethiopia’s East Amhara Region (Derso et al., 2015). The record for life at extremely high temperatures among all metazoan living organisms is held by the nematode Aphelenchoides sp. discovered in a New Zealand hot spring at a temperature of 61.3°C. Moreover, the temperature in the spring where the aphelenchoids were collected ranged from 58 to 69.1°C, indicating that these nematodes may likely withstand temperatures of 70°C (Mason, 1939; Poinar, 2015).
Adaptations of groundwater invertebrates to thermal and hot waters
All organisms have a temperature range in which they can grow, reproduce, and function at their best (Negus et al., 2020). Temperature is a key factor affecting the development, metabolism, and survival of invertebrates (Quinn et al., 1994). When it comes to high temperatures, organisms often have an upper critical temperature that, if exceeded, can alter or even damage biochemical processes, membrane structures, enzymes and tissues (Willmer et al., 2009). Thus, they will not be found in ecosystems that are outside of their temperature tolerance (Prosser et al., 1952; Negus et al., 2020).
Experiments have shown that at temperatures close to the lethal limit, the voluntary muscles of both vertebrates and invertebrates enter a state of rigor (Everatt et al., 2013). As a result, if organisms are unable to move, they are unable to search for food or escape from potentially dangerous environmental conditions or predators (Glazier, 2012). Insects enter a reversible coma stage before dying as a result of high temperatures (breakdowns of neural and muscular systems), which protects them from cellular damage and complete energy depletion caused by repair mechanisms, allowing them to stay alive at temperatures that would normally be lethal (Rodgers et al., 2010).
It is generally agreed that the invertebrates’ geographical distribution and abundance are influenced in part by the temperature ranges at which they can survive and function optimally (Everatt et al., 2013). Moreover, thermal tolerance also defines interactions between species by regulating species distributions (González-Tokman et al., 2020). Therefore, it is obvious that the inhabitants of hot springs are equipped with different adaptations to withstand or tolerate high temperatures (Brues, 1927).
Tolerance and responses to high temperatures are the consequence of a complex combination of internal physiological and biochemical processes, including sensory and regulatory neuronal pathways, cellular stress responses, and metabolic and hormonal responses, mechanisms which remain inadequately studied (González-Tokman et al., 2020). Sensorial processes, which involve neurons and neurotransmitters that detect an environmental signal and initiate reactions such as changes in the metabolic rate and the use of the anaerobic metabolism (metabolic rate increases exponentially with temperature), are the first physiological systems used in response to heat (Gillooly et al., 2001). For example, the blind shrimp Rimicaris exoculata Williams and Rona, 1986, an oceanic floor hot spring species, has highly developed “eyes” that are thought to detect variations of weak light emitted by the 350°C fluids of black smokers, potentially preventing the shrimp from being boiled by the heat (Van Dover, 2019).
Hormones like catecholamines (i.e., adrenaline, noradrenaline, dopamine) also regulate physiology, development, and behavior by modulating responses to high temperatures (e.g., diapause) (González-Tokman et al., 2020). It has been demonstrated that Niphargus inopinatus gradually increases its adrenaline concentration in response to a sudden heat shock of either +6 or + 12°C relative to the temperature of the collection site (Avramov et al., 2013).
However, the most studied mechanism of heat tolerance is the stress response which involves the synthesis and use of heat shock proteins (HSP). These proteins are molecular chaperones that are increased in response to a wide variety of stressors (heat, cold, starvation, infection, inflammation, or exposure to contaminants) and protect other proteins from denaturing and aggregation at high temperatures (Wang et al., 2012; González-Tokman et al., 2020). Therefore, the increased levels of HSP protect against oxidative stress (Oksala et al., 2014). The sequencing of the whole transcriptome of Allobathynella bangokensis, a subterranean aquatic crustacean occasionally found in hot springs (the subterranean region of Hongcheon-Gun, Gangwon-Do, South-Korea), revealed the presence of expressed conserved gene family sets, such as heat shock proteins, suggesting that this species has evolved adaptations involving molecular mechanisms of homeostasis (Kim et al., 2017).
Living in volcanoes
Volcanic caves
Volcanic caves, also known as lava tubes, are the remains of lava’s internal drainage conduits beneath a solidified crust, which can form in one of two ways: by crusting over a flowing lava stream or, more typically, by fluid lava continuing to stream beneath the crust (Lauritzen, 2018; Figure 1C). The basaltic volcanic terrain contains active hydrothermal systems and transmissive, heterogeneous and discontinuous aquifers formed by the mechanical fracturing during cooling and separations between distinct lava flows. These aquifers are typically unconfined and fissured, and the water table can be found at great depths (Kiernan et al., 2003; Lauritzen, 2018).
The aquifers that run through volcanic rocks provide a significant portion of water resources in several parts of the world, including islands (e.g., Canary Islands – Spain, Jeju Island – South Korea, Hawaii Islands – United States, Galapagos Islands – Ecuador) and continental regions (e.g., Northwest Ethiopia, Mount Erebus – Antarctica, Somma – Vesuvius and Roccamonfina volcanoes – Italy; Fraser et al., 2018; Pantaleone et al., 2018; Fenta et al., 2020; Ahn et al., 2021). Their productivity is heavily dependent on volcanic stratigraphy, which varies greatly, ranging from high permeability interflow zones to almost impenetrable tuffaceous layers (Ahn et al., 2021). Volcanic rocks are more chemically reactive than most other geologic formations, allowing chemolithoautotrophic microbial populations to survive in the absence of light by providing them with electron acceptors and donors (Tebo et al., 2015). The geochemical characteristics of GW are also influenced by the chemical components of rainfall as well as numerous geochemical processes (e.g., rock-water interactions) that occur when water flows from recharge to discharge regions (Fenta et al., 2020).
Groundwater invertebrates in volcanic caves
One of the most investigated subterranean faunas from volcanic regions in the world is represented by the Canary Archipelago in the eastern Atlantic (Oromi, 2018). It consists of seven islets and seven main islands, including Tenerife, the world’s largest, highest, and most diverse island, and Lanzarote, which has the most diverse volcanic anchialine ecosystems in the Eastern Atlantic. Volcanic eruptions generated all the islands over the last 20 million years (Juan et al., 2000).
Due to the impermeable nature of the volcanic rocks, the accumulation of subterranean freshwaters took place at the phreatic level in elevated areas and near the coast, where the water is more saline and habitats can be considered as anchialine or mixohaline (Oromi, 2018; Bishop et al., 2020). Survival conditions in lava tubes are also challenging, being characterized by oligotrophy, especially in the deepest sections (limited food resources, constant temperature, hypoxia, darkness, and higher CO2 concentrations; Oromi, 2018). Furthermore, in the case of anchialine habitats, there are additional challenges such as varying salinity (prevalence of Cl− and Na+ ions, transition zones between the fresh and marine water—halocline), or the presence of hydrogen sulfide caused by microbial degradation of organic detritus trapped in the water (e.g., La Corona lava tube, Lanzarote, Canary Islands, Spain—a detritus-based system; Jaume and Boxshall, 2011; Iliffe, 2018).
The variety of aquatic invertebrates in the volcanic islands of Tenerife (lava tubes and phreatic) showing markedly morphological and physiological adaptations (stygobites) sum about 44 species (Martin and Oromi, 1986). Crustaceans are the most represented and all are endemics of the archipelago, or a single island. The amphipod Pseudoniphargus fontinalis Stock, 1988 has been collected from anchialine wells in Tenerife and Gran Canarias, whereas the interstitial stygobitic Ingolfiella canariensis (Vonk and Sanchez, 1991) has been found on more than one island. However, they are clearly related to the marine environment (Vonk and Sanchez, 1991). In the lowland, where the habitats have no seawater influence, the most significant groups are copepod species (i.e., Parastenocaris sp.) and amphipods. Noteworthy to mention is the tantulocarid Stygotantalus stocki (Boxshall and Huys, 1989), an ectoparasite on harpacticoid copepods and the smaller arthropod in the world (0.1 mm) (Boxshall and Huys, 1989).
The anchialine fauna of Tenerife is well known and species have direct marine origin (Wilkens et al., 2009). The most known lava tube from Lanzarote (Tenerife) is the system Cueva de los Verdes, the 15th most extended lava tube in the world and the longest underwater cave (Wilkens et al., 2009). The cave has two parts, a submarine one of 2 km (Tunel de Atlantida) and, in the opposite direction, is Jameos del Agua and Hameo de los Lagos.
This lava tube is a detritus-based system, in which particulate organic matter is delivered via tidal exchange or penetration through the surrounding lava rock. The abundance of suspended organic matter in the water column promotes a diverse array of 77 species (37 endemic consumers), including stygobitic copepods, ostracods, amphipods, remipedes, thermosbaenaceans, mysids, decapods, isopods and molluscs, as well as a few highly specialized annelid species (Wilkens et al., 2009; Martínez and Gonzalez, 2018). The most remarkable species is the archaic remipede Speleonectes ondinae (García-Valdecasas, 1984), whose genus includes a few species inhabiting anchialine caves from West Indies and Yucatan (Schram et al., 1986) and the decapod Munidopsis polymorpha Koelbel, 1982 and endangered crustacean species (Figure 3). Palmorchestia hypogea Stock and Martín, 1988 from La Palma (Canary Islands) is one of the few terrestrial amphipods known to be adapted to volcanic cave life.
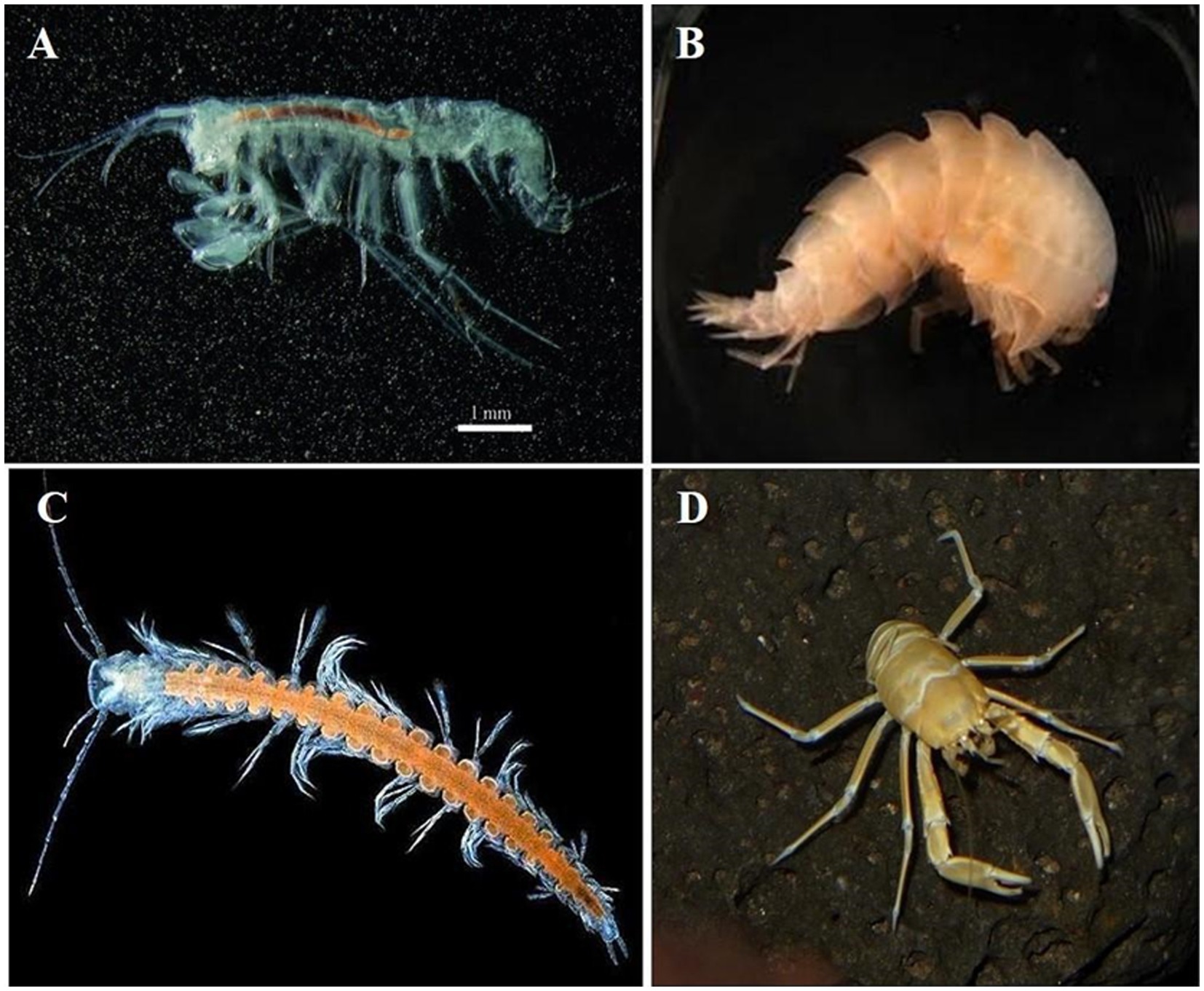
Figure 3. Invertebrates’ biodiversity found in volcanic groundwater settings. (A) Amphipod Pseudoniphargus sp. from Canary Islands, Spain (Photo: H. López). (B) Tantulocarid Stygotantalus stocki, ectoparasite on copepods (Photo: https://miniaturezoo.weebly.com/stygotantulus-stocki.html ). (C) Remiped Speleonectes ondinae from Lanzarote caves, Canary Islands, Spain (Photo: Ulrike Strecker). (D) Decapod Munidopsis polymorpha (Photo: Ondřej Machač).
A special case of underground volcanic habitat is the geothermal subglacial environments in Antarctica, such as subglacial caves on volcanic Mount Erebus (Warren Cave, 22 Blue and Harry’s dream), the world’s southernmost active volcano, which might have served as important refuges for terrestrial life on this severely glaciated continent (Wardell et al., 2003; Fraser et al., 2018). The habitability of these environments appears to be a direct outcome of the volcanic activity that generates steam, the main source of water required to sustain life (Shock and Holland, 2007). These subglacial ecosystems are frequently 10 degrees hotter than ambient air temperatures (on average ~ 0°C), may contain liquid water, and can get sunlight near their entrances or where the covering ice is thin. Overlying ice and snow are melted by volcanic heat and gas exhalations, resulting in liquid water that can seep down into the volcano, where it might come into contact with heated rocks, turning it into a stream (Tebo et al., 2015). The water in these oligotrophic caves ensures exceedingly challenging living circumstances, with liquid water at very low temperatures at or above freezing (0°C) near the surface, or extremely hot in the deepest parts (60°C), and large amounts of magmatic CO2 dissolved in water (Wardell et al., 2003; Ilanko et al., 2019). Furthermore, it is likely that gases produced by volcanic activity and the subsequent interactions between condensed steam, these gases, and subterranean minerals constitute the main energy source that supports the residing populations (Sims et al., 2020).
According to a metabarcoding (eDNA) analysis that involves mitochondrial COI gene and nuclear ribosomal 28S gene (via Ion Torrent sequencing), and nuclear ITS (via cloning), these subglacial geothermal volcanic caves are home to various eukaryotic groups, including invertebrates such as arthropods (arachnids and collembolans), oligochaetes (Achaeta sp. and Bryodrilus sp.) and nematodes (Enchodelus sp.) (Fraser et al., 2018).
Living on the edge
The sulfide-rich continental aquifers and caves
Extreme habitats, such as those rich in naturally occurring hydrogen sulfide (H2S), can be found in aquatic systems all over the world (Greenway et al., 2014). In the anoxic waters of the Proterozoic (2,500 to 541 million years ago), H2S was abundant, serving as an energy source for early forms of life and shaping the biochemical and physiological processes of organisms. When life first began, H2S was likely the most versatile molecule because it could operate as a critical organic product, reactant, proto-enzyme, proto-membrane, or an important energy source (Olson and Straub, 2016).
The freshwater springs releasing sulfide-rich waters have been spotted on every continent except Antarctica (Greenway et al., 2014). The occurrence of sulfide in these ecosystems can vary significantly and is generally linked to one of two sources (Greenway et al., 2014): (1) the volcanic activity, where a variety of compounds leach into solution during the interaction of water with the hot basaltic rock (sulfate and other sulfur compounds), which are easily converted into sulfide under highly reductive conditions; and (2) the underground oil deposits, where mineral-rich GW, including sulfate, combines with hydrocarbons produced from fossil organic matter in anoxic conditions.
High concentrations of H2S are presently found in a few aquatic settings as a result of production through biogeochemical processes such as thermal vents and cold seeps in marine environments (Table 1; Greenway et al., 2014; Tobler et al., 2016). Aquifers with sulfide-rich GW are sparsely scattered in the proximity of the sea’s shores (Sarbu, 2000; Engel, 2007). These unique ecosystems are supported by primary production through bacterial chemosynthesis (Sarbu et al., 1994a,b; Sarbu, 2000; Kumaresan et al., 2018). Here, the primary production is very high, supported by chemo-autotrophic bacteria and protozoa that derive their energy through chemical reactions with inorganic molecules such as H2S, elemental sulfur and NH3 under low oxygen environment or in anaerobic conditions (Sarbu, 2000; Engel, 2007; Frumkin et al., 2020). In detail, the sulfide-oxidizing bacteria use H2S as an energy source, as electron donors, to assimilate CO2 and produce oxidized sulfur species as a metabolic by-product (Engel, 2007).
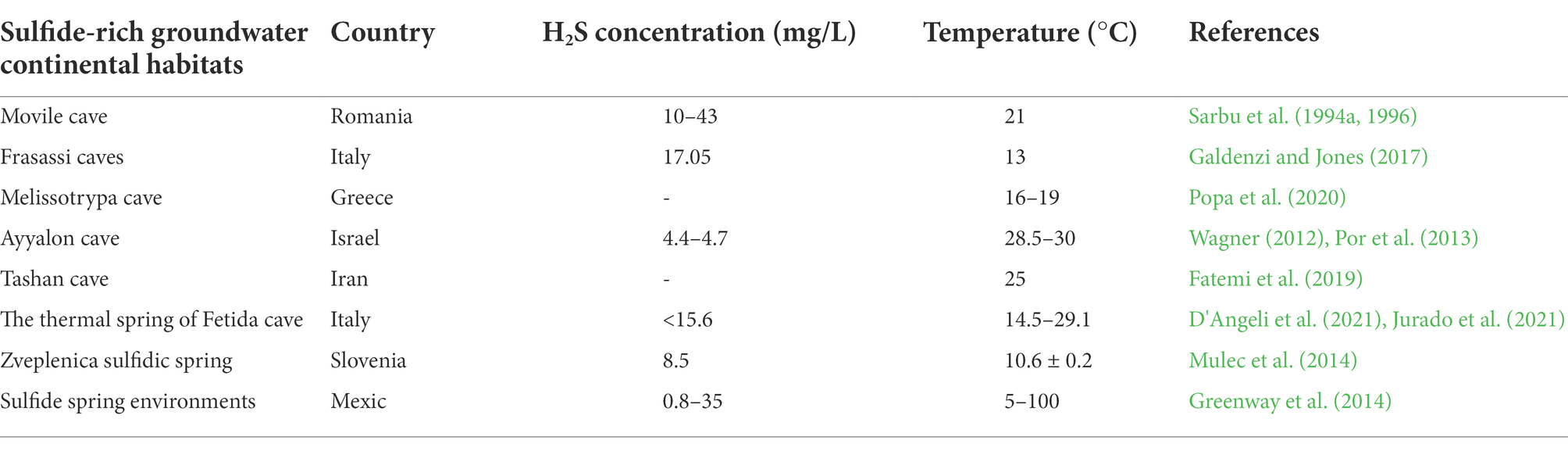
Table 1. H2S concentration and temperature in different sulfide-rich groundwater continental habitats.
Apart from nutrient and energy limitations, darkness, oxygen scarcity up to hypoxia (dissolved oxygen concentrations less than 2 mg/L) or even anoxia, and geochemically variable conditions, which usually characterize GW ecosystems (Diaz, 2001; Hancock et al., 2005; Engel, 2007), sulfidic–rich GW poses additional pressure to organism including higher average temperatures, higher concentrations of H2S, CH4 and other ions (e.g., heavy metals, bicarbonate, calcium sulfate, sodium chloride), and lower pH (Table 1; Xu et al., 1998; Sarbu, 2000; Engel, 2007; Greenway et al., 2014). The location of springs/caves, as well as the final sources of H2S generation, appears to be involved in temperature variation (Greenway et al., 2014).
On a biochemical level, H2S toxicity to invertebrates is well understood (Tobler et al., 2016). H2S easily penetrates biological membranes and invades organisms due to its lipid solubility, having different effects such as the interruption of cellular respiration, neurotoxic effects modulated by disruption of calcium homeostasis, or alteration of a wide variety of other proteins (O2 transport proteins and other 20 enzymes; Tobler et al., 2016). Its toxicity is manifested primarily through interfering with oxidative phosphorylation in mitochondria by the inhibition of cytochrome C oxidase (COX) in the mitochondrial respiratory chain, which prevents ATP production (Cooper and Brown, 2008). Therefore, exposure to H2S in the environment can affect the organisms’ capacity to survive and reproduce (Kelley et al., 2016).
In these GWs, sulfuric acid speeds up the dissolving of limestone bedrock in a process known as sulfuric acid speleogenesis, resulting in the creation of sulfidic caves (Sarbu, 2000; Northup and Lavoie, 2001; Engel et al., 2004; Porter et al., 2009; Galdenzi and Maruoka, 2018). Hydrogen sulfide-rich phreatic fluids and microbially-generated sulfuric acid likely played a significant role in the speleogenesis of about 10% of the world’s caves (Engel et al., 2004; Fatemi et al., 2019).
Sulfide concentrations vary significantly across all springs, with levels decreasing as the distance from the springheads increases due to evaporation and oxidation of sulfide molecules (Chen and Morris, 1972). The lower pH is most likely due to the presence of sulfuric acid via chemical and microbiological H2S oxidation, and it is also influenced by the region’s water buffering capacity (Xu et al., 1998; Greenway et al., 2014). Despite these challenging conditions, sulfide-rich groundwater offers ecological advantages such as enhanced resource availability, less competition by providing a larger variety of ecological niches when compared to “classical” aquifers, and reduced exposure to predators (Tobler et al., 2016). Moreover, adaptation to H2S-rich settings can also promote speciation, resulting in biodiversity hotspots with high levels of endemism (Tobler et al., 2016).
Sulfidic caves have been examined the most in terms of primary production, sulfide-oxidizing, methanotrophic, and nitrifying bacteria providing the trophic base for the entire local community in these habitats (Sarbu et al., 1996; Sarbu, 2000; Porter et al., 2009; Bauermeister et al. 2013; Flot et al., 2014; Greenway et al., 2014).
Groundwater invertebrates in sulfide-rich continental aquifers and caves
The impact of H2S on aquatic invertebrates, including the ecology and evolution of species, has primarily been investigated in cold seeps and deep-sea hydrothermal vents (Greenway et al., 2014). However, continental sulfide GW has received more attention since the first discovered ecosystem based on chemosynthesis: Movile Cave in Romania (Sarbu et al., 1996). More recently, the investigations on the aquifer to which the cave belongs (Grapa, 2022) as well as the discovery of other chemoautotrophically-based subterranean ecosystems improved the knowledge on GW diversity as well as on species adaptation to such a particular environment, i.e., Ayyalon Cave in Israel (Por et al., 2013), Melissotrypa Cave in Greece (Popa et al., 2020), Tashan Cave in Iran (Fatemi et al., 2019), and more recently, the sulfidic caves in Chechnya in the Caucasus Mountains (Chervyatsova et al., 2020).
While the richness of bacteria that live in sulfide-rich continental habitats is pretty well understood at several levels of biological organization, few studies have focused on metazoan diversity patterns (Greenway et al., 2014). Lithoautotrophic microorganisms in sulfidic habitats can sustain a relatively abundant invertebrate community, but they create environmental conditions in which only highly specialized species can live. As a result, sulfide invertebrate populations are seen as a model of “extreme lifestyle,” where the only prosperous species are those capable of tolerating low O2 and high H2S concentrations (Lee et al., 2012; Flot et al., 2014; Galassi et al., 2016; Brad et al., 2021).
Different phyla of organisms have inhabited this harsh environment for most life forms, resulting in diverse biological communities with high levels of endemism similar to marine habitats (Sarbu et al., 1996; Tobler et al., 2016). The GW invertebrate communities are characterized by a relatively large diversity of species and complex trophic levels that could be attributed to the abundant and diverse, chemosynthetically produced food source that has allowed organisms to live in and adapt to harsh environmental conditions (Engel, 2007; Por et al., 2013; Galassi et al., 2016; Popa et al., 2020; Brad et al., 2021). The invertebrate groups that live in continental sulfide-rich environments include copepods (cyclopoids, harpacticoida, calanoids), thermosbaenaceans, decapods, amphipods, isopods, ostracods, hemipterans, as well as platyhelminthes (Rhabditophora), nematodes (Chromadorea, Enoplea), annelids (Clitellata, Polychaeta), gastropods, molluscs, and rotifers. However, the crustaceans dominate the invertebrate groups in the sulfidic habitats, which are particularly rich and diverse (Figure 4; Plesa, 1989; Engel, 2007; Peterson et al., 2013; Por et al., 2013; Flot et al., 2014; Greenway et al., 2014; Mulec et al., 2014; Galassi et al., 2016; Khalaji-Pirbalouty et al., 2018; Borko et al., 2019; Fatemi et al., 2019; Mulec and Engel, 2019; Popa et al., 2020; Brad et al., 2021; Supplementary Table 1).
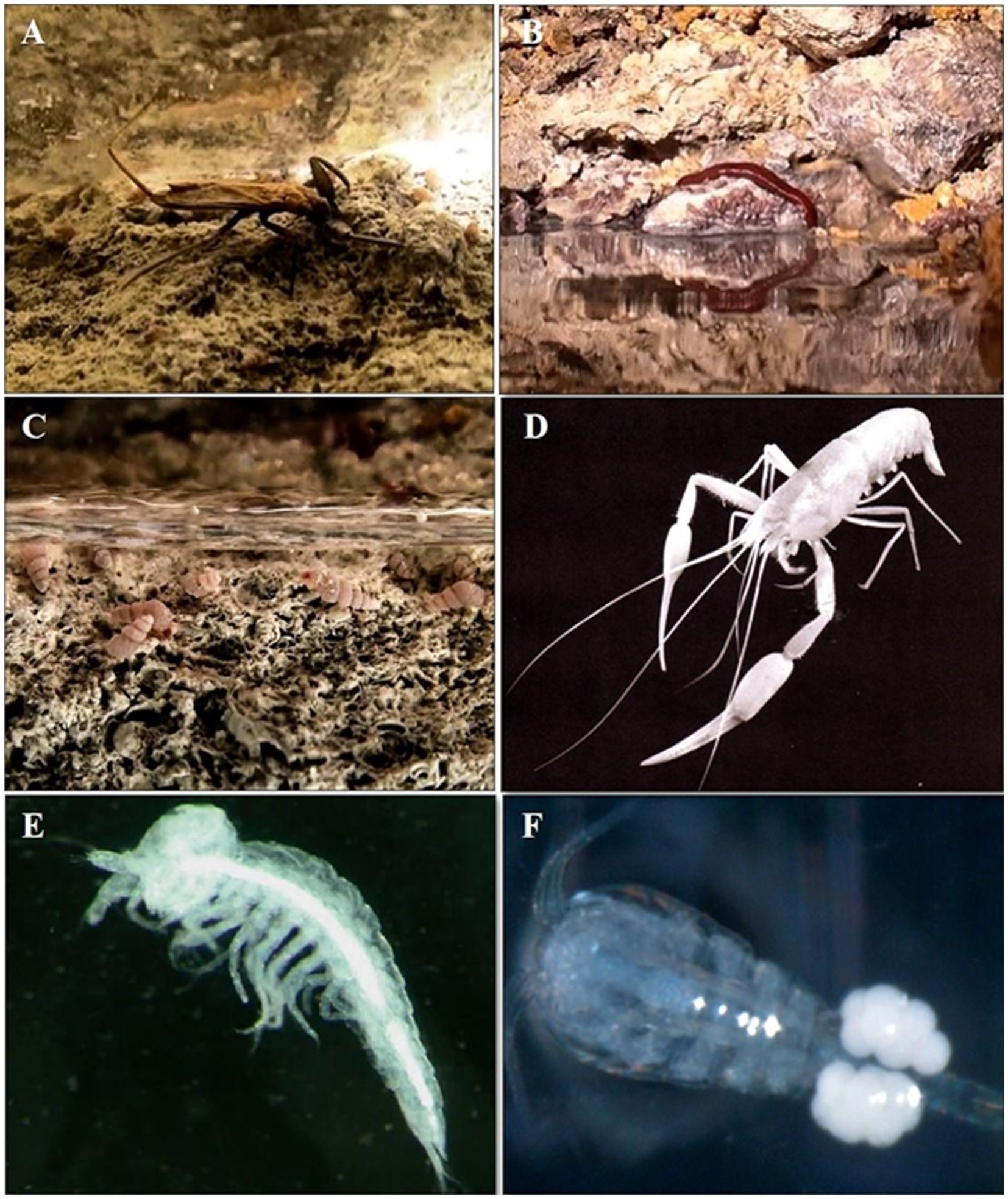
Figure 4. Sulfide-rich groundwater continental habitats invertebrates’ biodiversity. Movile Cave, Romania (Photos: Serban Sarbu): (A) water scorpion Nepa anophthalma; (B) leech Haemopis caeca; (C) gastropods Heleobia dobrogica. Ayyalon Cave, Israel (Photos: Amos Frumkin): (D) decapod Typhlocaris ayyaloni; (E) thermosbaenacean Tethysbaena ophelicola; (F) cyclopoid Metacyclops subdolus.
Among the most extensively investigated sulfide-based ecosystems is Movile Cave located at about 3 km from the Black Sea coast in the Southern Dobrogea region of Romania (Sarbu, 2000). Movile Cave is a hypogenic system generated by rising fluids via different geological and tectonic structures at various depths through various dissolution processes (Sarbu et al., 1996). This cave has one of the most diversified subterranean invertebrate populations in the world despite its isolation from the surface: 37 out of the 52 species (21 aquatic and 31 terrestrial) are endemic to the cave (Brad et al., 2021; Grapa, 2022). The trophic web is remarkably complex and the food base consists of the chemoautotrophic microorganisms (e.g., Archaea, sulfur- and methane-oxidizing bacteria, or nitrifying and denitrifying bacteria), that live in the sulfide-rich water and sediments (Chen et al., 2009; Kumaresan et al., 2014; Hillebrand-Voiculescu, 2018; Chiciudean et al., 2022). The rich cave-adapted meiofauna in Movile Cave includes arthropods, platyhelminths, annelids, rotifers, mollusks, and several species of crustaceans (Brad et al., 2021). The top predators in the cave are the water scorpions Nepa anophthalma Decu et al., 1994, which hide beneath the water’s surface and wait for prey composed of amphipods and isopods; the leech Haemopis caeca Manoleli et al., 1998, which feed on earthworms (Helodrilus sp.), and the flatworm Dendrocoelum obstinatum Stocchino and Sluys, 2013, that feed on bacteria, worms, or crustaceans (Sarbu et al., 1996, 2019). A predator–prey relationship was identified between the nematodes (Panagrolaimus sp. and Poikilolaimus sp.) and the endemic copepod Eucyclops greateri scythicus Pleșa, 1989, which predate actively on nematodes (Muschiol et al., 2008). Several of these stygobites present in the Movile Cave have also been found to live in the associated sulfidic aquifer in Mangalia area, and among them the amphipod crustacean Niphargus dancaui (Brad et al., 2015), the cyclopoids Eucyclops greateri scythicus and a new species of ostracod from the genus Pseudocandona (Danielopol, 1994; Sarbu, 2000; Grapa, 2022). Furthermore, the diversity of Movile Cave never fails to amaze us, as new inhabitants, earthworms, a new genus, Coventina sp. Marchán, (Szederjesi and Csuzdi, 2022), and a new species, Imetescolex movilensis Szederjesi, Sarbu and Csuzdi, 2022, brought the total number of species hosted here to 54 (Szederjesi et al., 2022).
The characteristic feature of the aquatic invertebrates living in Movile Cave is the ability to endure high levels of H2S and low concentrations of O2 or hypoxia (Hillebrand-Voiculescu, 2018). Despite the differences in nutrient availability, habitat, water chemistry (sulfidic vs. non-sulfidic freshwater), ancestral genetic variability, and population genetic structure, there is a parallel evolution of eye loss, depigmentation, and elongation of some appendages with all stygobionts. This was demonstrated in a study that compared Asellus aquaticus (Linnaeus, 1758) from Romania’s sulfidic waters (Movile Cave, springs and wells from the same aquifer) with a cave-adapted Asellus from freshwaters in Slovenia (Konec et al., 2015).
The Frasassi Caves system in central Italy, a flourishing oasis for underground life, is composed of a series of ramifying, mostly subterranean tunnels that run more than 30 kilometers and are organized on multiple interconnected levels in which sulfidic waters flow (Galdenzi and Jones, 2017). Moreover, the sulfide and oxygen content in the cave system’s streams and lakes fluctuate considerably, leading to the occurrence of both sulfidic and non-sulfidic water habitats with specific groups of invertebrates having different tolerances to both parameters (Macalady et al., 2008). In the sulfidic water habitats, sulfide-oxidizing chemosynthetic bacteria generate organic matter and support a rich and diversified invertebrate community, including ostracods (Mixtacandona sp., Pseudolimnocythere sp.), niphargid amphipods Niphargus ictus (Karaman, 1985), Niphargus frasassianus Karaman et al., 2010, and Niphargus montanarius Karaman et al., 2010), calanoids (Eudiaptomus intermedius Steuer, 1897), harpacticoids (Nitocrella stammeri Chappuis, 1938, Maraenobiotus sp., Nitocrella psammophila Chappuis, 1955), cyclopids (Diacyclops cosanus, Speocyclops sp.), gastropods (Islamia pusilla Piersanti, 1951), and platyhelminthes (Dendrocoelum leporii Manconi, 2017), the majority of them being endemic to this cave system (Engel, 2007; Peterson et al., 2013; Flot et al., 2014; Galassi et al., 2016; Borko et al., 2019).
The Tashan Cave, located in Southwest Iran, offers a unique hypogean chemoautotrophic environment with several pools and lakes containing GW rich in hydrogen sulfide (Fatemi et al., 2019). Trogloiranica tashanica Fatemi et al. (2019) is one of the few stygobitic gastropods reported in subterranean sulfide-rich waters, and the isopod Stenasellus tashanicus (Fatemi et al., 2019), are among the endemic invertebrate species found in this cave (Jones et al., 2014; Khalaji-Pirbalouty et al., 2018; Fatemi et al., 2019).
In the case of Ayyalon Cave from Israel, another hypogenic cave system, six of the eight species on the list are new and currently restricted to this sulfidic aquifer (Por et al., 2013). The stygobitic species list associated with the sulfidic pool (4.4–4.7 mg/L dissolved H2S) includes four crustacean species: two copepods (Metacyclops longimaxillis Defaye and Por, 2010, Metacyclops subdolus Kiefer, 1938), one thermosbaenacean (Tethysbaena ophelicola Wagner, 1994), and one decapod (Typhlocaris ayyaloni Tsurnamal, 2008), considered to be a primary consumer in this chemoautotrophic ecosystem. Metacyclops longimaxillis and Tethysbaena ophelicola are permanent inhabitants, mating in the pool’s near-anaerobic, sulfidic, and hot water (Wagner, 2012; Por et al., 2013; Frumkin et al., 2020).
More recently, biological studies have been initiated in Melissotrypa Cave in central Greece, which has both sulfidic and non-sulfidic lakes (Borko et al., 2019; Popa et al., 2020). Biological investigations in this cave led to the discovery of several stygobitic invertebrates species, some of them being strictly associated with sulfidic waters, such as platyhelminthes (Dendrocoelum sp.), annelids (Haplotaxis sp., Delaya sp.), mollusks (Iglica hellenica Falniowski and Sarbu, 2015, Daphniolla magdalenae Falniowski and Sarbu, 2015), ostracods (Mixtacandona sp.), cyclopids (Tropocyclops prasinus Fischer, 1860, Acantocyclops sp.), isopods (Turcolana lepturoides Prevorčnik, Konec and Sket, 2016), and niphargid amphipods (Niphargus gammariformis Borko, Collette, Brad, Zakšek, Flot, Vaxevanpoulos, Sarbu and Fišer, 2019; Borko et al., 2019; Popa et al., 2020.
Adaptations of groundwater invertebrates to sulfidic-rich environment
The tolerance levels and adaptations to sulfide in aquatic invertebrates have been analyzed. Sulfide exposure was combined with hypoxia or anoxia in tolerance experiments, and the effects were compared to hypoxia or anoxia alone. The findings showed that sulfide exacerbated the consequences of hypoxia and anoxia (Bagarinao, 1992). However, H2S is a gaseous messenger in cell communication as well as a regulator of physiological processes, as it acts directly on ionic channels (Peers et al., 2012). Moreover, H2S is generated internally as a byproduct of the breakdown of sulfur-containing proteins, coenzymes, enzymes, or by microbial fermentation in the alimentary tract (Vismann, 1991). Hence, it has become clear that organisms may not just try to remove H2S from their body, but rather maintain optimal amounts that allow it to function as a physiological regulator (Tobler et al., 2016). In other words, at low concentrations, H2S has a crucial physiological role in cell signaling, but at high concentrations, it is a severe respiratory toxicant (Tobler et al., 2016).
Therefore, colonizing sulfide-rich GW ecosystems requires specific adaptations to the stress of living underground besides the common ones characterizing the stygobite species usually occurring in the subterranean realm. The ability of organisms to cope with the harmful effects of H2S is mediated by a variety of mechanisms (Tobler et al., 2016). The increased tolerance reported in adapted invertebrate species living in H2S-rich habitats could be achieved by four processes (Kelley et al., 2016; Tobler et al., 2016): (1) the ability to reduce the amount of H2S that enters the body through avoidance and exclusion strategies (behavioral adaptations or morphological changes in structure and components of the integument and respiratory surfaces); (2) the reduction of toxic compounds’ adverse effects (physiological adaptations); (3) the increased capacity to regulate internal H2S levels despite the continuous environmental influx (low endogenous H2S production, detoxification or sequestration); and (4) potentially by the presence of symbiosis with sulfur-oxidizing bacteria.
The ostracod Cyprideis torosa (Jones, 1850), a geographically widespread surface species, can oxidize penetrating sulfide to non-toxic thiosulfate and sulfite and then promptly eliminate the oxidation products. It can also withstand long-term sulfidic conditions due to its strong capacity for long-term anaerobiosis, which enables the ostracod to survive or escape adverse environmental conditions (Jahn et al., 1996; De Deckker and Lord, 2017).
Some crustaceans use certain behavioral strategies to adapt to the local environmental conditions. Some Thermosbaena species, swim in an upside-down, notonectic position, often “hanging” motionless on the water surface, such as the populations of Tethysbena ophelicola from Ayyalon Cave in Israel (Fryer, 1965; Por, 2014). The thermosbaenacean’s hyponeuston-like swimming allows the specimens to be maintained in the density layer of the redox interphase, where bacteria proliferate (Por, 2014). Therefore, the ability to float on the support of water density represents a significant energy saving for the creatures in the anoxic or microxic chemoautotrophic conditions in which they reside (Por, 2014). Moreover, dorsal breeding, unique among crustaceans, is a consequence of the notonectic swimming position (Por, 2014). The marsupium with the developing eggs and embryos is suspended at the anaerobic level, safe from predation. The beating maxillipeds and pereiopods, on the other hand, keep a constant stream of oxygenated water flowing through the body (Barker, 1962; Por, 2014).
Symbioses among aquatic invertebrates and bacteria in sulfidic–rich environments
In dark, isolated and sulfide-rich marine and non-marine habitats, symbioses between invertebrates and chemosynthetic bacteria (e.g., sulfur- or methane-oxidizing) are common and have developed separately in various invertebrate groups (Bauermeister et al., 2012). At least three protist groups and invertebrates from seven phyla, including poriferans, cnidaria, mollusks, platyhelminthes, annelids, nematodes, and various arthropods, are involved in chemosynthetic symbiotic associations (Bauermeister et al., 2013; Flot et al., 2014; Tobler et al., 2016; Sogin et al., 2021).
Symbioses were essential for the origin and diversification of eukaryotes, and they continue to be a primary driving factor in evolution because they cause physiological, morphological, and developmental changes in the species involved (e.g., metabolism, pathogen defense, feeding, reproduction, ecology; Sapp, 2004; Shin et al., 2011). This type of association is most common in habitats where there is not enough organic matter to support a heterotrophic lifestyle. The benefits of associating with chemosynthetic bacteria are apparent: by collaborating with primary producers who can access energy resources such as sulfur and methane, these organisms gain access to energy sources they could not reach otherwise (Tobler et al., 2016; Sogin et al., 2020). Chemosynthetic bacteria also benefit from collaborating with eukaryotes because the host offers them a constant supply of reduced substrates, oxidants, and CO2 by hitch-hiking to areas where some vital elements are present (Flot et al., 2014; Tobler et al., 2016). Chemosynthetic symbiotic associations occur in a wide variety of shapes and sizes, reflecting how convergent evolution has allowed both hosts and bacteria to adapt to a symbiotic way of life. Some symbionts (ectosymbionts) only colonize the organism’s surfaces, whereas others (endosymbionts) dwell either extracellularly or intracellularly within their host in the integument, respiratory structures, or specialized organs of the host (Bauermeister et al., 2013; Flot et al., 2014; Tobler et al., 2016; Sogin et al., 2020).
Symbiotic relationships between organisms and chemoautotrophic microorganisms have been first evidenced around hydrothermal vents where symbiotic bacteria use reduced inorganic compounds such as sulfide and methane from venting fluid to gain energy, offering primary nutrition for the host organism (Beinart et al., 2015). Some host species, such as the gutless hydrothermal vent tube worms Riftia pachyptila Jones, 1981 or the gutless marine oligochaete Olavius algarvensis Giere, Erséus and Stuhlmacher, 1998 found in the coastal sediments of Elba Island, Italy, rely only on endosymbionts for nutrition (and excretion–Olavius): a single bacterial phylotype stored in trophosome in case of Rifftia or multiple phylotypes from different phyla housed beneath the cuticle in case of Olvius (16S rRNA gene sequencing; Childress et al., 1991; Kleiner et al., 2012).
Later on, two potential symbioses were first reported from sulfidic continental caves, such as Ayyalon Cave, Israel: an endosymbiotic relationship in the case of T. ophelicola, a preferred monophagous sulfur-bacteria-eating pelagic crustacean, which has the intestinal tracts entirely packed with bacterial cells, and an ectosymbiotic relationship in the case of the prawn T. ayyaloni, which has a bacterial layer on the body surface (Tsurnamal, 2008; Por et al., 2013).
However, the first extensive study of symbioses has been evidenced in the sulfidic waters of the Frasassi Caves system, Italy (Dattagupta et al., 2009). The symbiosis involves three Niphargus amphipod species (N. ictus, N. frasassianus, and N. montanarius) and sulfur-oxidizing Thiothrix bacterial ectosymbionts. These ectosymbionts are predominantly linked to hairs (setae) and the spines of their legs and antennae (Dattagupta et al., 2009; Bauermeister et al., 2012). The Thiothrix ectosymbionts of Frasassi-dwelling Niphargus (N. ictus and N. frasassianus) do not appear to be involved in sulfide detoxification for their hosts, according to a previous study (Bauermeister et al., 2013). However, even without their Thiotrix ectosymbionts, which had been eliminated by antibiotic treatment, the amphipods appear to have very high sulfide tolerances. It remains to be seen whether these sulfur-oxidizing bacteria provide any further advantages to their invertebrate hosts (Bauermeister et al., 2013).
A Niphargus–Thiothrix association has also been discovered in Movile Cave and the surrounding aquifer in Mangalia, Romania (Flot et al., 2014; Boanca, 2022). Six of the seven niphargid species recorded in this region (sulfidic and non-sulfidic GW) have potential Thiothrix ectosymbionts attached, similar to that seen before in Italy’s Frasassi Caves. Thus, this suggests that they could be widespread in continental sulfidic and non-sulfidic aquifers (Flot et al., 2014).
More recently, the molecular evidence (16S rDNA) of the first association between microcrustaceans (ostracods and cyclopoids) and Thiothrix was revealed in the sulfidic GW habitat of Mangalia, demonstrating the versatility of such Thiothrix-crustacean associations in sulfidic ecosystems (Boanca, 2022).
Future directions and outlooks
The study of aquatic invertebrates has propelled our knowledge of the boundaries of life in extreme habitats. However, there is a fundamental lack of studies addressing the tolerance of stygobitic ″extremophile″ metazoans to multiple extremes, and this hampers our understanding of their tolerance to the interaction between several parameters. Given the high pressures that anthropogenic activities pose on GW habitats in general and on those with ″extreme″ environmental parameters in particular, searching for life’s in GW actual limit under the combined effects of natural and anthropogenic parameters is essential.
The unique and reduced complexity of extreme GW ecosystems offers an excellent opportunity for studying the functions of natural stygobitic communities. Omics techniques have the potential to resolve patterns and ecological drivers of these ″extremophile″ assemblages, uncovering linkages between community function and environmental variables. Especially, integrative-omic studies are expected to shed light on the molecular adjustments (including epigenetic effects) that occurred during the transitions to extreme GW habitats. This will help disentangle the role of standing genetic variation and phenotypic plasticity in driving the evolution of subterranean populations in extreme GW habitats, similarly to what was observed in non-extreme cave habitats (Bilandžija et al., 2020).
Finally, thanks to ongoing scientific investigations, we still find life in unexpected, extreme GW habitats. Many invertebrates, including phylogenetically deeply rooted taxa, many of which are novel to science, are found to survive and live in extreme GW environments. Given the number of Earth ecosystems that still need to be explored in detail, we expect the current frontier of GW life to be pushed even further. Again, genetic tools, such as barcoding and metagenomics, are a critical premise to highly increase the probability of finding new extremophile invertebrate species in difficult-to-reach underground places.
Author contributions
All authors developed the idea and assisted in editing the manuscript and approved the submitted version.
Funding
This work was supported by a grant from the Romanian Ministry of Education and Research, CNCS - UEFISCDI project number PN - III - P4 - PCE - 2020 - 2843 (EVO-DEVO-CAVE) within PNCDI III. SI was also supported by grants of the Romanian Ministry of Education and Research, CNCS - UEFISCDI project number and PN-III-P4-ID-PCCF-2016-0016 (DARKFOOD) within PNCDI III.
TDL was funded by a project under the National Recovery and Resilience Plan (NRRP), Mission 4 Component 2 Investment 1.4 - Call for tender No. 3138 of 16 December 2021, rectified by Decree n.3175 of 18 December 2021 of Italian Ministry of University and Research funded by the European Union – NextGenerationEU; Award Number: Project code CN_00000033, Concession Decree No. 1034 of 17 June 2022 adopted by the Italian Ministry of University and Research, CUP B83C22002930006, Project title “National Biodiversity Future Center - NBFC”.
Conflict of interest
The authors declare that the research was conducted in the absence of any commercial or financial relationships that could be construed as a potential conflict of interest.
Publisher’s note
All claims expressed in this article are solely those of the authors and do not necessarily represent those of their affiliated organizations, or those of the publisher, the editors and the reviewers. Any product that may be evaluated in this article, or claim that may be made by its manufacturer, is not guaranteed or endorsed by the publisher.
Supplementary material
The Supplementary material for this article can be found online at: https://www.frontiersin.org/articles/10.3389/fevo.2022.1054841/full#supplementary-material
References
Addo-Bediako, A., Chown, S. L., and Gaston, K. J. (2000). Thermal tolerance, climatic variability and latitude. Proc. R. Soc. Lond. B Biol. Sci. 267, 739–745. doi: 10.1098/rspb.2000.1065
Ahn, U. S., Koh, D.-C., Heo, J., Cho, B.-W., Kim, T., and Yum, B.-W. (2021). Conceptualizing a multi-layered shingle aquifer model based on volcanic stratigraphy and water inflow to lava caves in Jeju Island, Korea. Hydrol. Process. 35:e14316. doi: 10.1002/hyp.14316
Avramov, M., Rock, T. M., Pfister, G., Schramm, K.-W., Schmidt, S. I., and Griebler, C. (2013). Catecholamine levels in groundwater and stream amphipods and their response to temperature stress. Gen. Comp. Endocrinol. 194, 110–117. doi: 10.1016/j.ygcen.2013.09.004
Bagarinao, T. (1992). Sulfide as an environmental factor and toxicant: tolerance and adaptations in aquatic organisms. Aquat. Toxicol. 24, 21–62. doi: 10.1016/0166-445X(92)90015-F
Barker, D. (1962). A study of Thermosbaena mirabilis (malacostraca, Peracarida) and its reproduction. Q. J. Microsc. Sci. 103, 261–286.
Bauermeister, J., Assig, K., and Dattagupta, S. (2013). Exploring the sulfide tolerance of ectosymbiotic Niphargus amphipods from the Frasassi caves, Central Italy. Int. J. Speleol. 42, 141–145. doi: 10.5038/1827-806X.42.2.6
Bauermeister, J., Ramette, A., and Dattagupta, S. (2012). Repeatedly evolved host-specific ectosymbiosis between sulfur-oxidizing bacteria and amphipods living in a cave ecosystem. PLoS One 7:e50254. doi: 10.1371/journal.pone.0050254
Beinart, R. A., Gartman, A., Sanders, J. G., Luther, G. W., and Girguis, P. R. (2015). The uptake and excretion of partially oxidized sulfur expands the repertoire of energy resources metabolized by hydrothermal vent symbioses. Proc. R. Soc. B 282:20142811. doi: 10.1098/rspb.2014.2811
Bilandžija, H., Hollifield, B., Steck, M., Meng, G., Ng, M., Koch, A. D., et al. (2020). Phenotypic plasticity as a mechanism of cave colonization and adaptation. elife 9:e51830. doi: 10.7554/eLife.51830
Birstein, J. A., and Ljovuschkin, S. I. (1967). Biospeleologica sovietica 33. The order Bathynellacea (Crustacea, malacostraca) in the U.R.S.S. I. Family Bathynellidae. Bull. Moscow Soc. 72, 51–66.
Bishop, R. E., Humphreys, W., and Jaume, D. (2020). “Subterranean and anchialine waters,” in Evolution and biogeography. eds. M. Thiel and G. Poore (New York), 8.
Boanca, F. (2022). Investigating the presence of sulfur-oxidizing bacteria on microcrustaceans from the groundwater system of Mangalia (Romania). Master’s thesis, University Babes-Bolyai: Cluj-Napoca, Romania.
Borko, Š., Collette, M., Brad, T., Zakšek, V., Flot, J.-F., Vaxevanopoulos, M., et al. (2019). Amphipods in a Greek cave with sulphidic and non-sulphidic water: phylogenetically clustered and ecologically divergent. Syst. Biodivers. 17, 558–572. doi: 10.1080/14772000.2019.1670273
Boulton, A. J., Fenwick, G. D., Hancock, P. J., and Harvey, M. S. (2008). Biodiversity, functional roles and ecosystem services of groundwater invertebrates. Invertebr. Syst. 22, 103–116. doi: 10.1071/IS07024
Bowman, T. E. (1975). Three new troglobitic asellids from western North America (Crustacea: Isopoda: Asellidae). Int. J. Speleol. 7, 339–356. doi: 10.5038/1827-806X.7.4.3
Boxshall, G. A., and Huys, R. (1989). New tantulocarid, Stygotantulus stocki, parasitic on harpacticoid copepods, with an analysis of the phylogenetic relationships within the Maxillopoda. J. Crust. Biol. 9, 126–140. doi: 10.2307/1548454
Brad, T., Fišer, C., Flot, J.-F., and Sarbu, S. M. (2015). Niphargus dancaui sp. nov. (Amphipoda, Niphargidae) – a new species thriving in sulfidic groundwaters in southeastern Romania. Eur. J. Taxon. 164, 1–28. doi: 10.5852/ejt.2015.164
Brad, T., Iepure, S., and Sarbu, S. M. (2021). The chemoautotrophically based Movile cave groundwater ecosystem, a hotspot of subterranean biodiversity. Diversity 13:128. doi: 10.3390/d13030128
Brittain, J. E., and Milner, A. M. (2001). Ecology of glacier-fed rivers: current status and concepts. Freshw. Biol. 46, 1571–1578. doi: 10.1046/j.1365-2427.2001.00845.x
Brues, C. T. (1924). Observations on animal life in the thermal waters of Yellowstone Park, with a consideration of the thermal environment. Proc. Am. Acad. Arts Sci. 59, 371–438. doi: 10.2307/20026104
Buzzini, P., Turchetti, B., and Yurkov, A. (2018). Extremophilic yeasts: the toughest yeasts around? Yeast 35, 487–497. doi: 10.1002/yea.3314
Cahill, A., Kavanagh, A., McCahill, A., Scott, A., and Espinasa, L. (2015). “Phylogenetic Analysis of Several New Populations of Stygobromus allegheniensis (Allegheny Cave Amphipod),” in Ice Caves of the Shawangunk Ridge, NY.
Camacho, A. I. (2006). An annotated checklist of Syncarida (Crustacea, malacostraca) in the world. Zootaxa 1374, 1–54. doi: 10.11646/zootaxa.1374.1.1
Camacho, A. I., Mas-Peinado, P., Iepure, S., Perina, G., Dorda, B. A., Casado, A., et al. (2020). Novel sexual dimorphism in a new genus of Bathynellidae from Russia, with a revision of phylogenetic relationships. Zool. Scr. 49, 47–63. doi: 10.1111/zsc.12387
Camacho, A. I., and Valdecasas, A. G. (2008). Global diversity of syncarids (Syncarida; Crustacea) in freshwater. Hydrobiologia 595, 257–266. doi: 10.1007/s10750-007-9021-5
Castaño-Sánchez, A., Hose, G. C., and Reboleira, A. S. P. S. (2020). Salinity and temperature increase impact groundwater crustaceans. Sci. Rep. 10:12328. doi: 10.1038/s41598-020-69050-7
Castellarin, A., Vogel, R. M., and Matalas, N. C. (2005). Probabilistic behavior of a regional envelope curve. Water Resour. Res. 41:W06018. doi: 10.1029/2004WR003042
Castenholz, R. W. (1969). Thermophilic blue-green algae and the thermal environment. Bacteriol. Rev. 33, 476–504. doi: 10.1128/br.33.4.476-504.1969
Cauvy-Fraunié, S., Andino, P., Espinosa, R., Calvez, R., Jacobsen, D., Dangles, O., et al. (2016). Ecological responses to experimental glacier-runoff reduction in alpine rivers. Nat. Commun. 7:12025. doi: 10.1038/ncomms12025
Chen, K., and Morris, J. (1972). Kinetics of oxidation of aqueous sulfide by O2. Environ. Sci. Technol. 6, 529–537. doi: 10.1021/es60065a008
Chen, Y., Wu, L., Boden, R., Hillebrand, A., Kumaresan, D., Moussard, H., et al. (2009). Life without light: microbial diversity and evidence of sulfur- and ammonium-based chemolithotrophy in Movile cave. ISME J. 3, 1093–1104. doi: 10.1038/ismej.2009.57
Chervyatsova, O. Y., Potapov, S. S., Kuzmina, L. Y., Dublyansky, Y. V., Sadykov, S. A., Kiseleva, D. V., et al. (2020). Sulfuric acid speleogenesis in the North Caucasus: Sharo-Argun valley caves (Chechen Republic, Russia). Geomorphology 369:107346. doi: 10.1016/j.geomorph.2020.107346
Chiciudean, I., Russo, G., Bogdan, D. F., Levei, E. A., Faur, L., Hillebrand-Voiculescu, A., et al. (2022). Competition-cooperation in the chemoautotrophic ecosystem of Movile cave: first metagenomic approach on sediments. Environ. Microbiome. 17:44. doi: 10.1186/s40793-022-00438-w
Childress, J. J., Fisher, C. R., Favuzzim, J. A., Kochevar, R. E., Sanders, N. K., and Alayse, A. M. (1991). Sulfide-driven autotrophic balance in the bacterial symbiont-containing hydrothermal vent tubeworm, Riftia pachyptila. Jones. Biol. Bull. 180, 135–153. doi: 10.2307/1542437
Colson-Proch, C., Morales, A., and Hervant, F. (2010). First cellular approach of the effects of global warming on groundwater organisms: a study of the HSP70 gene expression. Cell Stress Chaper. 15, 259–270. doi: 10.1007/s12192-009-0139-4
Cooper, C. E., and Brown, G. C. (2008). The inhibition of mitochondrial cytochrome oxidase by the gases carbon monoxide, nitric oxide, hydrogen cyanide and hydrogen sulfide: chemical mechanism and physiological significance. J. Bioenerg. Biomembr. 40, 533–539. doi: 10.1007/s10863-008-9166-6
Culver, D. C., Deharveng, L., Pipan, T., and Bedos, A. (2021). An overview of subterranean biodiversity hotspots. Diversity 13:487. doi: 10.3390/d13100487
Culver, D., and Pipan, T. (2019). The biology of caves and other subterranean habitats. Oxford: Oxford University Press. doi: 10.1093/oso/9780198820765.001.0001,
D'Angeli, I. M., DeWaele, J., Fiorucci, A., Vigna, B., Bernasconi, S. M., Florea, L. J., et al. (2021). Hydrogeology and geochemistry of the sulfur karst springs at Santa Cesarea Terme (Apulia, southern Italy). Hydrogeol. J. 29, 481–498. doi: 10.1007/s10040-020-02275-y
Danielopol, D. (1989). Groundwater fauna associated with riverine aquifers. J. North Am. Benthol. Soc. 8, 18–35. doi: 10.2307/1467399
Danielopol, D. (1994). “Ostracoda,” in Stygofauna Mundi. ed. L. Botosaneanu Mitteilungen Aus Dem Museum Für Naturkunde in Berlin. Zoologisches Museum Und Institut Für Spezielle Zoologie (Berlin) (Brill, Leiden), 259–294.
Dattagupta, S., Schaperdoth, I., Montanari, A., Mariani, S., Valley, J. W., and Macalady, J. L. (2009). A novel symbiosis between chemoautotrophic bacteria and a freshwater cave amphipod. ISME J. 3, 935–943. doi: 10.1038/ismej.2009.34
De Deckker, P., and Lord, A. (2017). Cyprideis torosa: a model organism for the Ostracoda? J. Micropalaeontol. 36, 3–6. doi: 10.1144/jmpaleo2016-100
De Jong, G. D., Canton, S. P., and Chadwick, J. W. (2005). Macroinvertebrates occurring in sunbeam hot springs, an absolutely hot spring in Idaho, USA. J. Freshw. Ecol. 20, 611–613. doi: 10.1080/02705060.2005.9664779
Decu, V., Juberthie, C., Iepure, S., Gheorghiu, V., and Nazareanu, G. (2019). An overview on the subterranean fauna from Central Asia. Ecol. Montenegrina 20, 163–188. doi: 10.37828/em.2019.20.14
Derso, S., Beyene, A., Melaku, G., and Ambelu, A. (2015). Ecological status of hot springs in eastern Amhara region: macroinvertebrates diversity. Am. Acad. Sci. Res. J. Eng.Technol. Sci. 14, 1–22.
Des Marais, D. J., and Walter, M. R. (2019). Terrestrial hot spring systems: introduction. Astrobiology 19, 1419–1432. doi: 10.1089/ast.2018.1976
Di Lorenzo, F., Crisafi, F., La Cono, V., Yakimov, M., Molinaro, A., and Silipo, A. (2020). The structure of the lipid a of gram-negative cold-adapted bacteria isolated from antarctic environments. Mar. Drugs 18:592. doi: 10.3390/md18120592
Di Lorenzo, T., Di Marzio, W. D., Fiasca, B., Diana, M. P. G., Korbel, K., Iepure, S., et al. (2019). Recommendations for ecotoxicity testing with stygobiotic species in the framework of groundwater environmental risk assessment. Sci. Total Environ. 681, 292–304. doi: 10.1016/j.scitotenv.2019.05.030
Di Lorenzo, T., and Galassi, D. (2017). Effect of temperature rising on the stygobitic crustacean species Diacyclops belgicus: does global warming affect groundwater populations? Water 9:951. doi: 10.3390/w9120951
Di Lorenzo, T., Hose, G. C., and Galassi, D. M. P. (2020). Assessment of different contaminants in freshwater: origin, fate and ecological impact. Water 12:1810. doi: 10.3390/w12061810
Diaz, R. J. (2001). Overview of hypoxia around the world. J. Environ. Qual. 30:275. doi: 10.2134/jeq2001.302275x
Dodds, W. K., and Whiles, M. R. (2010). Unusual or extreme habitats. J. Freshw. Ecol. 375–398. doi: 10.1016/b978-0-12-374724-2.0
Dole-Olivier, M. J., Castellarini, F., Coineau, N., Galassi, D. M. P., Martin, P., Mori, N., et al. (2009). Towards an optimal sampling strategy to assess groundwater biodiversity: comparison across six European regions. Freshw. Biol. 54, 777–796. doi: 10.1111/j.1365-2427.2008.02133.x
Drake, B. D., Campbell, K. A., Rowland, J. V., Guido, D. M., Browne, P. R. L., and Rae, A. (2014). Evolution of a dynamic paleo-hydrothermal system at Mangatete, Taupo volcanic zone, New Zealand. J. Volcanol. Geotherm. Res. 282, 19–35. doi: 10.1016/j.jvolgeores.2014.06.010
Dumnicka, E., Galas, J., Najberek, K., and Urban, J. (2020). The influence of Pleistocene glaciations on the distribution of obligate aquatic subterranean invertebrate fauna in Poland. Zool. Anz. 286, 90–99. doi: 10.1016/j.jcz.2020.04.003
Engel, A. S. (2007). Observations on the biodiversity of sulfidic karst habitats. J. Caves Karst. Stud. 69, 187–206. doi: 10.4236/ns.2013.54A002
Engel, A. S., Lee, N., Porter, M. L., Stern, L. A., Bennett, P. C., and Wagner, M. (2003). Filamentous “Epsilonproteobacteria” dominate microbial mats from sulfidic cave springs. Appl. Environ. Microbiol. 69, 5503–5511. doi: 10.1128/AEM.69.9.5503-5511.2003
Engel, A. S., Stern, L. A., and Bennett, P. C. (2004). Microbial contributions to cave formation: new insights into sulfuric acid speleogenesis. Geology 32, 369–272. doi: 10.1130/G20288.1
Espinasa, L., McCahill, A., Kavanagh, A., Espinasa, J., Scott, A., and Cahill, A. (2015). A troglobitic amphipod in the ice caves of the Shawangunk ridge: behavior and resistance to freezing. Subterr. Biol. 15, 95–104. doi: 10.3897/subtbiol.15.4733
Everatt, M. J., Bale, J. S., Convey, P., Worland, M. R., and Hayward, S. A. L. (2013). The effect of acclimation temperature on thermal activity thresholds in polar terrestrial invertebrates. J. Insect Physiol. 59, 1057–1064. doi: 10.1016/j.jinsphys.2013.08.003
Fatemi, Y., Malek Hosseini, M.-J., Falniowski, A., Hofman, S., Kuntner, M., and Grego, J. (2019). Description of a new genus and species as the first gastropod species from caves in Iran. J. Caves Karst. Stud. 81, 233–243. doi: 10.4311/2019LSC0105
Fattorini, S., Borges, P. A. V., Fiasca, B., and Galassi, D. M. P. (2016). Trapped in the web of water: groundwater-fed springs are island-like ecosystems for the meiofauna. Ecol. Evol. 6, 8389–8401. doi: 10.1002/ece3.2535
Fenta, M. C., Anteneh, Z. L., Szanyi, J., and Walker, D. (2020). Hydrogeological framework of the volcanic aquifers and groundwater quality in Dangila town and the surrounding area, Northwest Ethiopia. Groundw. Sustain. Dev. 11:100408. doi: 10.1016/j.gsd.2020.100408
Flot, J.-F., Bauermeister, J., Brad, T., Hillebrand-Voiculescu, A., Sarbu, S. M., and Dattagupta, S. (2014). Niphargus-Thiothrix associations may be widespread in sulphidic groundwater ecosystems: evidence from southeastern Romania. Mol. Ecol. 23, 1405–1417. doi: 10.1111/mec.12461
Fraser, C. I., Connell, L., Lee, C. K., and Cary, S. C. (2018). Evidence of plant and animal communities at exposed and subglacial (cave) geothermal sites in Antarctica. Polar Biol. 41, 417–421. doi: 10.1007/s00300-017-2198-9
Friberg, N., Milner, A. M., Svendsen, L. M., Lindegaard, C., and Larsen, S. E. (2001). Macroinvertebrate stream communities along regional and physico-chemical gradients in western Greenland. Freshw. Biol. 46, 1753–1764. doi: 10.1046/j.1365-2427.2001.00857.x
Frumkin, A., Dimentman, C., and Naaman, I. (2020). Biogeography of living fossils as a key for geological reconstruction of the East Mediterranean: Ayyalon-Nesher Ramla system. Israel. Quat. Int. 624, 168–180. doi: 10.1016/j.quaint.2020.11.036
Fryer, G. (1965). Studies on the functional morphology and feeding mechanism of Monodella argentarii Stella (Crustacea: Thermosbaenacea). Trans. Roy. Soc. Edinb. 57, 59–90.
Galassi, D. M. P., Fiasca, B., Di Lorenzo, T., Montanari, A., Porfirio, S., and Fattorini, S. (2016). Groundwater biodiversity in a chemoautotrophic cave ecosystem: how geochemistry regulates microcrustacean community structure. Aquat. Ecol. 51, 75–90. doi: 10.1007/s10452-016-9599-7
Galdenzi, S., and Jones, D. S. (2017). “The Frasassi caves: a “classical” active hypogenic cave,” in Cave and karst Systems of the World. eds. A. Klimchouk, et al., Springer, Cham. 143–159.
Galdenzi, S., and Maruoka, T. (2018). Sulfuric acid caves in Calabria (South Italy): cave morphology and sulfate deposits. Geomorphology. doi: 10.1016/j.geomorph.2018.12.014
Gibert, J., Danielopol, D.L., and Stanford, J.A. (1994). Groundwater ecology. San Diego (CA): Academic Press.
Gillooly, J. F., Brown, J., West, G., Savage, V., and Charnov, E. (2001). Effects of size and temperature on metabolic rate. Science 293, 2248–2251. doi: 10.1126/science.1061967
Glass, T. W., Breed, G. A., Iwahana, G., Kynoch, M. C., Robards, M. D., Williams, C. T., et al. (2021). Permafrost ice caves: an unrecognized microhabitat for Arctic wildlife. Ecology 102:e03276. doi: 10.1002/ecy.3276
Glazier, D. S. (2012). Temperature affects food-chain length and macroinvertebrate species richness in spring ecosystems. Freshw. Sci. 31, 575–585. doi: 10.1899/11.058.1
González-Tokman, D., Córdoba-Aguilar, A., Dáttilo, W., Lira-Noriega, A., Sánchez-Guillén, R. A., and Villalobos, F. (2020). Insect responses to heat: physiological mechanisms, evolution and ecological implications in a warming world. Biol. Rev. 95, 802–821. doi: 10.1111/brv.12588
Goretti, E., Pallottini, M., Pagliarini, S., Catasti, M.La, Porta, G., Selvaggi, R., et al. (2019). Use of larval morphological deformities in Chironomus plumosus (Chironomidae: Diptera) as an indicator of freshwater environmental contamination (Lake Trasimeno, Italy). Water 12:1. doi: 10.3390/w12010001
Grapa, S. (2022). The structure of crustacean communities (Cyclopoida. Copepoda) from the mesothermal aquifer from Mangalia (Dobrogea, Romania). Master’s thesis, University Babes-Bolyai, Cluj-Napoca, Romania.
Greenway, R., Arias-Rodriguez, L., Diaz, P., and Tobler, M. (2014). Patterns of macroinvertebrate and fish diversity in freshwater sulphide springs. Diversity 6, 597–632. doi: 10.3390/d6030597
Griebler, C., and Avramov, M. (2015). Groundwater ecosystem services: a review. Freshw. Sci. 34, 355–367. doi: 10.1086/679903
Griebler, C., and Lueders, T. (2009). Microbial biodiversity in groundwater ecosystems. Freshw. Biol. 512, 649–677. doi: 10.1111/j.1365-2427.2008.02013.x
Griebler, C., and Mösslacher, F. (2003). “Grundwasser. Eine ökosystemare Betrachtung” in Grundwasserökologie, Wien UTB-Facultas Verlag. eds. C. Griebler and F. Mösslacher, 253–310. doi: 10.36198/9783825221119
Hancock, P. J., Boulton, A. J., and Humphreys, W. F. (2005). Aquifers and hyporheic zones: towards an ecological understanding of groundwater. Hydrogeol. J. 13, 98–111. doi: 10.1007/s10040-004-0421-6
Hansson, L., Hylander, S., Dartnall, H., Lidström, S., and Svensson, J. (2012). High zooplankton diversity in the extreme environments of the McMurdo Dry Valley lakes. Antarct. Sci. 24, 131–138. doi: 10.1017/S095410201100071X
Hewitt, G. (2000). The genetic legacy of the quaternary ice ages. Nature 405, 907–913. doi: 10.1038/35016000
Hillebrand-Voiculescu, A. (2018). “Researches in sulphide-based ecosystems” in Cave ecology, ecological studies. eds. O. Moldovan, Ľ. Kováč, and S. Halse, (Cham: Springer), 235, 351–368. doi: 10.1007/978-3-319-98852-8_16
Hillebrand-Voiculescu, A., Itcus, C., Ardelean, I., Pascu, D., Persoiu, A., Rusu, A., et al. (2014). Searching for cold-adapted microorganisms in the underground glacier of Scarisoara ice cave, Romania. Acta Carsologica/Karsoslovni Zbornik 43, 319–329. doi: 10.3986/ac.v43i2-3.604
Holsinger, J. R. (1980). Stygobromus canadensis, a new subterranean amphipod crustacean (Crangonyctidae) from Canada, with remarks on Wisconsin refugia. Canadian J. of Zool. 58, 290–297. doi: 10.1139/z80-034
Holsinger, J. R., Mort, J. S., and Recklies, A. D. (1983). The subterranean crustacean fauna of Castleguard cave, Columbia Icefields, Alberta, Canada and its zoogeographic significance. Arct. Antarct. Alp. Res. 15, 543–549. doi: 10.2307/1551240
Howarth, F. G., and Moldovan, O. T. (2018). “The ecological classification of cave animals and their adaptations,” in Cave ecology. Vol. 235.(Cham: Ecological Studies, Springer), 41–67.
Iepure, S. (2018). “Ice caves fauna,” in Ice Caves. eds. A. Persoiu and S. E. Lauritzen. 1st ed (Amsterdam, Netherlands: Elsevier Science and Technology Book), 163–171.
Iepure, S., Gomez-Ortiz, D., Lillo-Ramos, J., Rasines-Ladero, R., and Di Lorenzo, T. (2022). Applying electrical resistivity tomography and biological methods to assess the hyporheic zone water exchanges in two Mediterranean stream-reaches. Water. ISSN, 14, 2073–4441. doi: 10.4236/ns.2013.54A002
Iepure, S., Martinez-Hernandez, V., Herrera, S., Rasines-Ladero, R., and de Bustamante, I. (2013). Response of microcrustacean communities from the surface-groundwater interface to water contamination in urban river system of the Jarama basin (Central Spain). Environ. Sci. Pollut. Res. 20, 5813–5826. doi: 10.1007/s11356-013-1529-9
Ilanko, T., Fischer, T. P., Kyle, P., Curtis, A., Lee, H., and Sano, Y. (2019). Modification of fumarolic gases by the ice-covered edifice of Erebus volcano, Antarctica. J. Volcanol. Geotherm. Res. doi: 10.1016/j.jvolgeores.2019.05
Iliffe, T. M. (2018). Collecting and processing crustaceans from anchialine and marine caves. J. Crustac. Biol. 38, 374–379. doi: 10.1093/jcbiol/ruy011
Issartel, J., Voituron, Y., Odagescu, V., Baudot, A., Guillot, G., Ruaud, J. P., et al. (2006). Freezing or supercooling: how does an aquatic subterranean crustacean survive exposures at subzero temperatures? J. Exp. Biol. 209, 3469–3475. doi: 10.1242/jeb.02387
Jacobsen, D., Milner, A. M., Brown, L. E., and Dangles, O. (2012). Biodiversity under threat in glacier-fed river systems. Nat. Clim. Chang. 2, 361–364. doi: 10.1038/nclimate1435
Jahn, A., Gamenick, I., and Theede, H. (1996). Physiological adaptations of Cyprideis torosa (Crustacea, Ostracoda) to hydrogen sulphide. Mar. Ecol. Prog. Ser. 142, 215–223. doi: 10.3354/meps142215
Jaume, D., and Boxshall, G. A. (2011). Life In Extreme Ocean Environments: Anchialine Caves in Marine Ecology. eds. Duarte, C.M., Helgueras, A.L., EOLSS, 1, 21.
Jones, D. S., Schaperdoth, I., and Macalady, J. L. (2014). Metagenomic evidence for sulfide oxidation in extremely acidic cave biofilms. Geomicrobiol J. 31, 194–204. doi: 10.1080/01490451.2013.834008
Juan, C., Emerson, B. C., Oromı́, P., and Hewitt, G. M. (2000). Colonization and diversification: towards a phylogeographic synthesis for the Canary Islands. Trends Ecol. Evol. 15, 104–109. doi: 10.1016/S0169-5347(99)01776-0
Juberthie, C., Sidorov, D., Decu, V., Mikhaljova, E., and Semenchenko, K. (2016). Subterranean fauna from Siberia and Russian Far East. Encyclopedia Biospeologica (Siberia-Far East special issue). Ecol. Mont. 7, 507–529. doi: 10.37828/em.2016.7.21
Jurado, V., D’Angeli, I., Martin-Pozas, T., Cappelletti, M., Ghezzi, D., Gonzalez-Pimentel, J. L., et al. (2021). Dominance of Arcobacter in the white filaments from the thermal sulfidic spring of Fetida cave (Apulia, southern Italy). Sci. Total Environ. 800:149465. doi: 10.1016/j.scitotenv.2021.149465
Kelley, J. L., Arias-Rodriguez, L., Patacsil Martin, D., Yee, M.-C., Bustamante, C. D., and Tobler, M. (2016). Mechanisms underlying adaptation to life in hydrogen sulfide–rich environments. Mol. Biol. Evol. 33, 1419–1434. doi: 10.1093/molbev/msw020
Khalaji-Pirbalouty, V., Fatemi, Y., Malek-Hosseini, M. J., and Kuntner, M. (2018). A new species of Stenasellus Dollfus, 1897 from Iran, with a key to the western Asian species (Crustacea, Isopoda, Stenasellidae). ZooKeyss 766, 39–50. doi: 10.3897/zookeys.766.23239
Khamis, K., Brown, L. E., Hannah, D. M., and Milner, A. M. (2016). Glacier–groundwater stress gradients control alpine river biodiversity. Ecohydrology 9, 1263–1275. doi: 10.1002/eco.1724
Kiernan, K., Wood, C., and Middleton, G. (2003). Aquifer structure and contamination risk in lava flows: insights from Iceland and Australia. Environ. Geol. 43, 852–865. doi: 10.1007/s00254-002-0707-8
Kim, B.-M., Kang, S., Ahn, D.-H., Kim, J.-H., Ahn, I., Lee, C.-W., et al. (2017). First insights into the subterranean crustacean Bathynellacea transcriptome: transcriptionally reduced opsin repertoire and evidence of conserved homeostasis regulatory mechanisms. PLoS One 12:e0170424. doi: 10.1371/journal.pone.0170424
Kleiner, M., Wentrup, C., Lott, C., Teeling, H., Wetzel, S., Young, J., et al. (2012). Metaproteomics of a gutless marine worm and its symbiotic microbial community reveal unusual pathways for carbon and energy use. Proc. Natl. Acad. Sci. 109, E1173–E1182. doi: 10.1073/pnas.1121198109
Knutsson, G. (2008). Hydrogeology in the Nordic countries. Episodes 31, 148–154. doi: 10.18814/epiiugs/2008/v31i1/020
Konec, M., Prevorčnik, S., Sarbu, S. M., Verovnik, R., and Trontelj, P. (2015). Parallels between two geographically and ecologically disparate cave invasions by the same species, Asellus aquaticus (Isopoda, Crustacea). J. Evol. Biol. 28, 864–875. doi: 10.1111/jeb.12610
Kreiling, A.-K., O’Gorman, E. J., Pálsson, S., Benhaïm, D., Leblanc, C. A., Ólafsson, J. S., et al. (2020). Seasonal variation in the invertebrate community and diet of a top fish predator in a thermally stable spring. Hydrobiologia 848, 531–545. doi: 10.1007/s10750-020-04409-5
Kristjánsson, B. K., and Svavarsson, J. (2004). Crymostygidae, a new family of subterranean freshwater gammaridean amphipods (Crustacea) recorded from subarctic Europe. J. Nat. Hist. 38, 1881–1894. doi: 10.1080/00222930310001597295
Kristjánsson, B., and Svavarsson, J. (2007). Subglacial Refugia in Iceland enabled groundwater amphipods to survive glaciations. Am. Nat. 170:292. doi: 10.1086/518951
Kumaresan, D., Stephenson, J., Doxey, A. C., Bandukwala, H., Brooks, E., Hillebrand-Voiculescu, A., et al. (2018). Aerobic proteobacterial methylotrophs in Movile cave: genomic and metagenomic analyses. Microbiome 6:1. doi: 10.1186/s40168-017-0383-2
Kumaresan, D., Wischer, D., Stephenson, J., Hillebrand-Voiculescu, A., and Murrell, J. C. (2014). Microbiology of Movile cave—a chemolithoautotrophic ecosystem. Geomicrobiol J. 31, 186–193. doi: 10.1080/01490451.2013.839764
Kunz, H. (1938). Die sandbewohnenden Copepoden von Helgoland, I. Teil. (Studien an marinen Copepoden. II). Kiel. Meeresforsch. 2, 223–254.
Lamberti, G. A., and Resh, V. H. (1985). Distribution of benthic algae and macroinvertebrates along a thermal gradient. Hydrobiologia 128, 13–21. doi: 10.1007/BF00008935
Lauritzen, S. E. (2018). “Physiography of the caves” in Cave ecology, ecological studies. eds. O. Moldovan, Ľ. Kováč, and S. Halse, vol. 235 (Cham: Springer), 7–21. doi: 10.1007/978-3-319-98852-8_2
Lee, N. M., Meisinger, D. B., Aubrecht, R., Kovacik, L., Saiz-Jimenez, C., Baskar, S., et al. (2012). “Caves and karst environments” in Life at extremes: Environments, organisms and strategies for survival. CAB international. ed. E. M. Bell (London: CABI International), 320–344. doi: 10.1079/9781845938147.0320
Macalady, J. L., Dattagupta, S., Schaperdoth, I., Jones, D. S., Druschel, G. K., and Eastman, D. (2008). Niche differentiation among sulfur-oxidizing bacterial populations in cave waters. ISME J. 2, 590–601. doi: 10.1038/ismej.2008.25
Malard, F., Mangin, A., Uehlinger, U., and Ward, J. V. (2001). Thermal heterogeneity in the hyporheic zone of a glacial floodplain. Can. J. Fish. Aquat. Sci. 58, 1319–1335. doi: 10.1139/f01-079
Malard, F., Tockner, K., and Ward, J. V. (1999). Shifting dominance of subcatchment water sources and flow paths in a glacial floodplain, Val Roseg. Switzerland. Arct. Antarct. Alp. 31, 135–150. doi: 10.1080/15230430.1999.12003291
Malard, F., Uehlinger, U., Zah, R., and Tockner, K. (2006). Flood-pulse and riverscape dynamics in a braided glacial river. Ecology 87, 704–716. doi: 10.1890/04-0889
Martin, J. L., and Oromí, P. (1986). An ecological study of Cueva de los Roques lava tube (Tenerife, Canary Islands). J. Nat. Hist. 20, 375–388. doi: 10.1080/00222938600770281
Martínez, A., and Gonzalez, B. C. (2018). “Volcanic anchialine habitats of Lanzarote” in Cave ecology, ecological studies. eds. O. Moldovan, Ľ. Kováč, and S. Halse, vol. 235 (Cham: Springer), 399–414. doi: 10.1007/978-3-319-98852-8_19
Maruyama, S., Kurokawa, K., Ebisuzaki, T., Sawaki, Y., Konomi, S., and Santosh, M. (2019). Nine requirements for the origin of Earth's life: not at the hydrothermal vent, but in a nuclear geyser system. Geosci. Front. 10, 1337–1357. doi: 10.1016/j.gsf.2018.09.011
Mason, I. L. (1939). Studies on the fauna of an Algerian hot spring. J. Exp. Biol. 16, 487–498. doi: 10.1242/jeb.16.4.487
Mavlyudov, B. R. (2018) in Ice genesis and types of ice caves. eds. A. Persoiu and S.-E. Lauritzen (Ice Caves: Elsevier), 33–68. doi: 10.1016/B978-0-12-811739-2.00032-2
McInerney, C. E., Maurice, L., Robertson, A. L., Knight, L. R., Arnscheidt, J., Venditti, C., et al. (2014). The ancient Britons: groundwater fauna survived extreme climate change over tens of millions of years across NW Europe. Mol. Ecol. 23, 1153–1166. doi: 10.1111/mec.12664
Merino, N., Aronson, H. S., Bojanova, D. P., Feyhl-Buska, J., Wong, M. L., Zhang, S., et al. (2019). Living at the extremes: extremophiles and the limits of life in a planetary context. Front. Microbiol. 10:780. doi: 10.3389/fmicb.2019.00780
Milner, A. M., Brittain, J. E., Castella, E., and Petts, G. E. (2001b). Trends of macroinvertebrate community structure in glacier-fed rivers in relation to environmental conditions: a synthesis. Freshw. Biol. 46, 1833–1847. doi: 10.1046/j.1365-2427.2001.00861.x
Milner, A. M., Khamis, K., Battin, T. J., Brittain, J. E., Barrand, N. E., Füreder, L., et al. (2017). Glacier shrinkage driving global changes in downstream systems. Proc. Natl. Acad. Sci. 114, 9770–9778. doi: 10.1073/pnas.1619807114
Milner, A. M., Robertson, A. L., Monaghan, K. A., Veal, A. J., and Flory, E. A. (2008). Colonization and development of an Alaskan stream community over 28 years. Front. Ecol. Environ. 6, 413–419. doi: 10.1890/060149
Milner, A., Taylor, R. C., and Winterbourn, M. J. (2001a). Longitudinal distribution of macroinvertebrates in two glacier-fed New Zealand rivers. Freshw. Biol. 46, 1765–1776. doi: 10.1046/j.1365-2427.2001.00856.x
Molden, D. J., Shrestha, A. B., Immerzeel, W. W., Maharjan, A., Rasul, G., Wester, P., et al. (2022). “The great glacier and snow-dependent rivers of Asia and climate change: heading for troubled waters,” in Water security under climate change. Water resources development and management. eds. A. K. Biswas and C. Tortajada (Singapore: Springer). doi: 10.1007/978-981-16-5493-0_12
Moseley, M. (2007). Acadian biospeleology: composition and ecology of cave fauna of Nova Scotia and southern New Brunswick. Canada. Int. J. Speleol. 36, 1–21. doi: 10.5038/1827-806X.36.1.1
Mulec, J., and Engel, A. S. (2019). Karst spring microbial diversity differs across an oxygen-sulphide ecocline and reveals potential for novel taxa discovery. Acta Carsologica 48, 129–143. doi: 10.3986/ac.v48i1.4949
Mulec, J., Oarga, A., Schiller, E. K., Persoiu, A., Holko, L., and Šebela, S. (2014). Assessment of the physical environment of epigean invertebrates in a unique habitat: the case of a karst sulfidic spring. Slovenia. Ecohydrology 8, 1326–1334. doi: 10.1002/eco.1585
Muschiol, D., Markovi’, C. M., Threis, I., and Traunspurger, W. (2008). Predatory copepods can control nematode populations: a functional-response experiment with Eucyclops subterraneus and bacterivorous nematodes. Fundam. Appl. Limnol. Arch. Für Hydrobiol. 172, 317–324. doi: 10.1127/1863-9135/2008/0172-0317
Negus, P. M., Marshall, J. C., Steward, A. L., and Mcgregor, G. B. (2020). Aquatic biota in hot water: thermal gradients in rheocrene hot spring discharges as analogues for the effects of climate warming. Knowl. Manag. Aquat. Ecosyst. 421:49. doi: 10.1051/kmae/2020042
Northup, D. E., and Lavoie, K. H. (2001). Geomicrobiology of caves: a review. Geomicrobiol J. 18, 199–222. doi: 10.1080/01490450152467750
O’Gorman, E. J., Petchey, O. L., Faulkner, K. J., Gallo, B., Gordon, T. A. C., Neto-Cerejeira, J., et al. (2019). A simple model predicts how warming simplifies wild food webs. Nat. Clim. Chang. 9, 611–616. doi: 10.1038/s41558-019-0513-x
Oksala, N. K. J., Ekmekçi, F. G., Özsoy, E., Kirankaya, Ş., Kokkola, T., Emecen, G., et al. (2014). Natural thermal adaptation increases heat shock protein levels and decreases oxidative stress. Redox Biol. 3, 25–28. doi: 10.1016/j.redox.2014.10.003
Olson, K. R., and Straub, K. D. (2016). The role of hydrogen sulfide in evolution and the evolution of hydrogen sulfide in metabolism and signaling. Physiology 31, 60–72. doi: 10.1152/physiol.00024.2015
Oromi, P. (2018). “Researches in lava tubes: analysis and synthesis” in Cave ecology, ecological studies. eds. O. Moldovan, Ľ. Kováč, and S. Halse, vol. 235 (Cham: Springer), 369–381. doi: 10.1007/978-3-319-98852-8_17
Pantaleone, D. V., Vincenzo, A., Fulvio, C., Silvia, F., Cesaria, M., Giuseppina, M., et al. (2018). Hydrogeology of continental southern Italy. J. Maps 14, 230–241. doi: 10.1080/17445647.2018.1454352
Papi, F., and Pipan, T. (2011). Ecological studies of an epikarst community in Snežna jama na planini Arto – an ice cave in north Central Slovenia. Acta Carsologica 40, 505–513. doi: 10.3986/ac.v40i3.62
Paun, V. I., Icaza, G., Lavin, P., Marin, C., Tudorache, A., Persoiu, A., et al. (2019). Total and potentially active bacterial communities entrapped in a late glacial through Holocene ice core from Scarisoara ice cave, Romania. Front. Microbiol. 10:1193. doi: 10.3389/fmicb.2019.01193
Peers, C., Bauer, C., Boyle, J. P., Scragg, J. L., and Dallas, M. L. (2012). Modulation of ion channels by hydrogen sulfide. Antioxid. Redox Signal. 17, 95–105. doi: 10.1089/ars.2011.4359
Persoiu, A., and Onac, B. P. (2012). “Ice in caves,” in Encyclopedia of caves. 2nd Edn. eds. W. B. White and D. C. Culler. (Burlington, MA, USA: Elsevier), 399–404.
Persoiu, A., Onac, B. P., Wynn, J., Bojar, A. V., and Holmgren, K. (2011). Stable isotopes behavior during cave ice formation by water freezing in Scarisoara ice cave, Romania. J. Geophys. Res. 116:D02111. doi: 10.4236/ns.2013.54A002
Peterson, D., Finger, K., Iepure, S., Mariani, S., Montanari, A., and Namiotko, T. (2013). Ostracod assemblages in the Frasassi caves and adjacent sulfidic spring and Sentino River in the Northeastern Apennines of Italy. J. Cave Karst. Stud. 75, 11–27. doi: 10.4311/2011PA0230
Plesa, C. (1989). Étude Préliminaire Des Cyclopides (Crustacea, Copepoda) de La Grotte “Peştera de La Movile”, Mangalia (Roumanie). Misc. Speologica Romanica 1, 39–45.
Poinar, G. O. (2015). “Phylum Nemata,” in Thorp and Covich’s Freshwater Invertebrates (Fourth Edition), Eds. James H. Thorp, D. Christopher Rogers, Academic Press, 273–302. doi: 10.1016/B978-0-12-385026-3.00014-0
Popa,, Brad, T., Vaxevanopoulos, M., Giurginca, A., Baba, S. C., Iepure, S., et al. (2020). Rich and diverse subterranean invertebrate communities inhabiting Melissotrypa cave in Central Greece. Trav. Institut de Spéol. "Émile Racovitza" 58, 65–78.
Por, F. D. (2014). Sulfide shrimp? Observations on the concealed life history of the Thermosbaenacea (Crustacea). Subterr. Biol. 14, 63–77. doi: 10.3897/subtbiol.14.7927
Por, F., Dimentman, C., Frumkin, A., and Naaman, I. (2013). Animal life in the chemoautotrophic ecosystem of the hypogenic groundwater cave of Ayyalon (Israel): a summing up. Nat. Sci. 5, 7–13. doi: 10.4236/ns.2013.54A002
Porter, M. L., Engel, A. S., Kane, T. C., and Kinkle, B. K. (2009). Productivity–diversity relationships from chemolithoautotrophically based sulfidic karst systems. Int. J. Speleol. 38, 27–40. doi: 10.5038/1827-806X.38.1.4
Pritchard, G. (1991). Insects in thermal springs. Mem. Ent. Soc. Can. 123, 89–106. doi: 10.4039/entm123155089-1
Prosser, C. L., Bishop, D., Brown, F., Jahn, T., and Wulff, V. J. (1952). Comparative animal physiology. Saunders Com., 341–381.
Quinn, J. M., Steele, G. L., Hickey, C. W., and Vickers, M. L. (1994). Upper thermal tolerances of twelve New Zealand stream invertebrate species. N. Z. J. Mar. Freshwater Res. 28, 391–397. doi: 10.1080/00288330.1994.9516629
Ramløv, H., and Friis, D. S. (2020). Antifreeze proteins volume 2: Biochemistry molecular biology and applications. Springer International Publishing.
Rodgers, C. I., Armstrong, G. A. B., and Robertson, R. M. (2010). Coma in response to environmental stress in the locust: a model for cortical spreading depression. J. Insect Physiol. 56, 980–990. doi: 10.1016/j.jinsphys.2010.03.030
Sapp, J. (2004). The dynamics of symbiosis: an historical overview. Canad. J. Bot. 82, 1046–1056. doi: 10.1139/b04-055
Sarbu, S. M. (2000). “Movile cave: a chemoautotrophically based groundwater ecosystem” in Subterranean ecosystems. eds. H. Wilkens, D. C. Culver, and W. F. Humphreys (Springer, Cham: Elsevier), 319–343.
Sarbu, S. M., Kane, T. C., and Kinkle, B. K. (1996). A chemoautotrophically based cave ecosystem. Science 272, 1953–1955. doi: 10.1126/science.272.5270.1953
Sarbu, S. M., Kinkle, B. K., Vlasceanu, L., Kane, T. C., and Popa, R. (1994a). Microbiological characterization of a sulfide-rich groundwater ecosystem. Geomicrobiol J. 12:175. doi: 10.1080/01490459409377984
Sarbu, S. M., Lascu, C., and Brad, T. (2019). “Dobrogea: Movile Cave” in Cave and karst systems of the world. eds. G. M. L. Ponta and B. P. Onac, 429–436. doi: 10.1007/978-3-319-90747-5_48
Sarbu, S. M., Vlasceanu, L., Popa, R., Sheridan, P., Kinkle, B. K., and Kane, T. C. (1994b). Microbial mats in a thermomineral sulfurous cave. In Microbial Mats. NATO ASI Series. eds. Stal, L. J., Caumette, P. 35, 45–50. Springer, Berlin, Heidelberg. doi: 10.1007/978-3-642-78991-5_4
Schmidt, S. I., and Hahn, H. J. (2012). What is groundwater and what does this mean to fauna? – an opinion. Limnologica 42, 1–6. doi: 10.1016/j.limno.2011.08.002
Schminke, H. K. (1986). “Syncarida,” in Stygofauna Mundi. ed. L. Botosaneanu, A faunistic, distributional and ecological synthesis of the world fauna inhabiting subterranean waters (Leiden: E.J. Brill/Dr. W. Backhuys), 389–404.
Schram, F. R., Yager, J., and Emerson, M. J. (1986). Remipedia. Part I. systematics. Mem. San Diego Soc. Nat. Hist. 15, 1–60.
Shin, S. C., Kim, S.-H., You, H., Kim, B., Kim, A. C., and Lee, K.-A. (2011). Drosophila microbiome modulates host developmental and metabolic homeostasis via insulin signaling. Science 334, 670–674. doi: 10.1126/science.1212782
Shock, E. L., and Holland, M. E. (2007). Quantitative habitability. Astrobiology 7, 839–851. doi: 10.1089/ast.2007.0137
Shock, E. L., Holland, M., Meyer-Dombard, D. R., and Amend, J. P. (2005). “Geochemical sources of energy for microbial metabolism in hydrothermal ecosystems: obsidian Pool, Yellowstone National Park,” in Geothermal biology and geochemistry in Yellowstone National Park. eds. W. P. Inskeep and T. R. McDermott (Bozeman: Thermal Biology Institute, Montana State University Publications), 95–109.
Simčič, T., and Sket, B. (2021). Ecophysiological responses of two closely related epigean and hypogean Niphargus species to hypoxia and increased temperature: do they differ? Int. J. Speleol. 50, 111–120. doi: 10.5038/1827-806X.50.2.2369
Sims, K. W., Aster, R., Gaetani, G., Blichert-toft, J., Wallace, P., Mattioli, G., et al. (2020). “Mount Erebus volcano: an exceptional natural laboratory for studying alkaline magmatism and open-conduit volcano behavior” in Volcanism in Antarctica: 200 million years of subduction, rifting and continental break-up. eds. J. L. Smellie, K. S. Panter, and A. Geyer (London, UK: Geological Society:)
Sogin, E. M., Kleiner, M., Borowski, C., Gruber-Vodicka, H. R., and Dubilier, N. (2021). Life in the dark: phylogenetic and physiological diversity of chemosynthetic symbioses. Annu. Rev. Microbiol. 75, 695–718. doi: 10.1146/annurev-micro-051021-123130
Sogin, E. M., Leisch, N., and Dubilier, N. (2020). Chemosynthetic symbioses. Curr. Biol. 30, R1137–R1142. doi: 10.1016/j.cub.2020.07.050
Stackebrandt, E. (2001). “Bacterial biodiversity,” in Encyclopedia of biodiversity. ed. S. A. Levin (Elsevier), 325–337. doi: 10.1016/b0-12-226865-2/00023-7
Szczucinska, A. M., and Wasielewski, H. (2013). Seasonal water temperature variability of springs from porous sediments in Gryzynka Valley, Western Poland. Quaest. Geogr. 32, 111–117. doi: 10.2478/quageo-2013-0019
Szederjesi, T., Fernandez, M. D., Csuzdi, C., Sarbu, S., Pavlíček, T., Krízsik,, et al. (2022). Three in one: molecular phylogeny of the genus Helodrilus (Crassiclitellata: Lumbricidae) with a description of two new genera and two new species. Zool. J. Linnean Soc. 69:zlac069. doi: 10.1093/zoolinnean/zlac069
Tebo, B. M., Davis, R. E., Anitori, R. P., Connell, L. B., Schiffman, P., and Staudigel, H. (2015). Microbial communities in dark oligotrophic volcanic ice cave ecosystems of Mt. Erebus, Antarctica. Front. Microbiol. 6:179. doi: 10.3389/fmicb.2015.00179
Thorp, J. H., and Covich, A. P. (2015). Overview of inland water habitats. Thorp and Covich's freshwater invertebrates. Academic Press. 23–56
Thulin, B., and Juergen, H.H. (2008). Ecology and living conditions of groundwater fauna (SKB-TR--08-06). Sweden:Svensk Kärnbränslehantering AB, Stockholm.
Tobler, M., Passow, C. N., Greenway, R., Kelley, J. L., and Shaw, J. H. (2016). The evolutionary ecology of organisms inhabiting hydrogen sulfide–rich environments. Annu. Rev. Ecol. Evol. Syst. 47, 239–262. doi: 10.1146/annurev-ecolsys-121415-032418
Tsurnamal, M. (2008). A new species of the stygobiotic blind prawn Typhlocaris Calman 1909 (Decapoda, Palaemonidae, Typhlocaridinae) from Israel. Crustaceana 81, 487–501. doi: 10.1163/156854008783797534
Tweed, F. S., Roberts, M. J., and Russell, A. J. (2005). Hydrologic monitoring of supercooled discharge from Icelandic glaciers. Quat. Sci. Rev. 24, 2308–2318. doi: 10.1016/j.quascirev.2004.11.020
Van Dover, C. L. (2019). Forty years of fathoming life in hot springs on the ocean floor. Nature 567, 182–184. doi: 10.1038/d41586-019-00728-3
Vismann, B. (1991). Physiology of sulfide detoxification in the isopod Saduria (Mesidotea) entomon. Mar. Ecol. Prog. Ser. 76, 283–293. doi: 10.3354/meps076283
Volkov, I. V., Zemtsov, V. A., Erofeev, A. A., Babenko, A. S., Volkova, A. I., and Callaghan, T. V. (2021). The dynamic land-cover of the Altai Mountains: perspectives based on past and current environmental and biodiversity changes. Ambio 50, 1991–2008. doi: 10.1007/s13280-021-01605-y
Vonk, R., and Sanchez, E. (1991). A new marine interstitial ingolfiellid (Crustacea, Amphipoda, Ingolfiellidea) from Tenerife and Hierro. Hydrobiologia 223, 293–299. doi: 10.1007/BF00047646
Wagner, H. P. (2012). Tethysbaena ophelicola n. sp. (Thermosbaenacea), a new prime consumer in the Ophel biome of the Ayyalon cave, Israel. Crustaceana 85, 1571–1587. doi: 10.1163/156854012x651646
Wang, H., Li, K., Zhu, J. Y., Fang, Q., Ye, G. Y., Wang, H., et al. (2012). Cloning and expression pattern of heat shock protein genes from the endoparasitoid wasp, Pteromalus puparum in response to environmental stresses. Arch. Insect Biochem. Physiol. 79, 247–263. doi: 10.1002/arch.21013
Wardell, L. J., Kyle, P. R., and Campbell, A. R. (2003). Carbon dioxide emissions from fumarolic ice towers, mount Erebus volcano, Antarctica. Geol. Soc. London Spec. Pub. 213, 231–246. doi: 10.1144/gsl.sp.2003.213.01.14
Warning, G. A., Blankenship, R. R., and Bentall, R. (1965). Thermal springs of the United States and other countries of the world - a summary. Revised by R.R Blankenship and ray Bentall. U.S. Geol. Survey Prof. Paper 492:383. doi: 10.3133/PP492
Wickstrom, C. E., and Castenholz, R. W. (1985). Dynamics of Cyanobacterial and Ostracod interactions in an Oregon hot spring. Ecology 66, 1024–1041. doi: 10.2307/1940563
Wilkens, H., Iliffe, T. M., Oromí, P., Martínez, A., Tysall, T. N., and Koenemann, S. (2009). The Corona lava tube, Lanzarote: geology, habitat diversity and biogeography. Mar. Biodivers. 39, 155–167. doi: 10.1007/s12526-009-0019-2
Willmer, P., Stone, G., and Johnston, I. (2009). Environmental physiology of animals. Sudbury, MA, USA: John Wiley & Sons.
Xu, Y., Schoonen, M. A. A., Nordstrom, D. K., Cunningham, K. M., and Ball, J. W. (1998). Sulfur geochemistry of hydrothermal waters in Yellowstone National Park: I. the origin of thiosulfate in hot spring water. Geochim. Cosmochim. Act. 62, 3729–3743. doi: 10.1016/S0016-7037(98)00269-5
Yonge, C. (2004). “Ice in caves,” in Encyclopedia of cave and karst science. ed. J. Gunn (London: Fitzroy-Dearborn Publishers, Ltd.), 435–437.
Keywords: ice caves, glacial aquifers, volcanic caves, thermal aquifers, sulfide-rich aquifers, invertebrates, adaptations
Citation: Pop MM, Di Lorenzo T and Iepure S (2023) Living on the edge – An overview of invertebrates from groundwater habitats prone to extreme environmental conditions. Front. Ecol. Evol. 10:1054841. doi: 10.3389/fevo.2022.1054841
Edited by:
Yixin Zhang, Soochow University, ChinaCopyright © 2023 Pop, Di Lorenzo and Iepure. This is an open-access article distributed under the terms of the Creative Commons Attribution License (CC BY). The use, distribution or reproduction in other forums is permitted, provided the original author(s) and the copyright owner(s) are credited and that the original publication in this journal is cited, in accordance with accepted academic practice. No use, distribution or reproduction is permitted which does not comply with these terms.
*Correspondence: Sanda Iepure, c2FuZGEuaWVwdXJlQGFjYWRlbWlhLWNqLnJv