- 1Department of Biological Sciences, Fordham University, Bronx, NY, United States
- 2Institute of Psychology, Polish Academy of Sciences, Warsaw, Poland
- 3Department of Animal Physiology, Faculty of Biology, Institute of Functional Biology and Ecology, University of Warsaw, Warsaw, Poland
- 4Center for Behavioral Brain Sciences, Institute for Pharmacology and Toxicology, Otto-von-Guericke University Magdeburg, Magdeburg, Germany
- 5Department of Ecology and Evolutionary Biology, University of California Los Angeles, Los Angeles, CA, United States
- 6Laboratory of Veterinary Ethology, The University of Tokyo, Tokyo, Japan
- 7Biology Teaching Laboratory, Faculty of Biology, University of Warsaw, Warsaw, Poland
- 8Department of Biological Sciences and the Louis Calder Center—Biological Field Station, Fordham University, Armonk, NY, United States
Mismatches between highly-standardized laboratory predatory assays and more realistic environmental conditions may lead to different outcomes. Understanding rodents’ natural responses to predator scents is important. Thus, field studies on the same and related species are essential to corroborate laboratory findings to better understand the contexts and motivational drives that affect laboratory responses to predator scents. However, there are too few field assays to enable researchers to study factors that influence these responses in genetically variable populations of wild rodents. Therefore, we placed laboratory-style chambers and remote-sensing devices near multiple colonies of two species of wild mice (Apodemus agrarius and Apodemus flavicollis) to test dual-motivational drives (appetitive and aversive) in a ‘familiar’, yet natural environment. A highly-palatable food reward was offered daily alongside scents from coyotes, lions, rabbits, and both wet and dry controls. In all but two instances (n = 264), animals entered chambers and remained inside for several minutes. Animals initiated flight twice, but they never froze. Rather, they visited chambers more often and stayed inside longer when predatory scents were deployed. The total time spent inside was highest for lion urine (380% longer than the dry control), followed by coyote scent (75% longer), dry control and lastly, herbivore scents (no difference). Once inside the chamber, animals spent more time physically interacting with predatory scents than the herbivore scent or controls. Our findings support the common assumption that rodents fail to respond as overtly to predatory scents in the field compared to what has been observed in the laboratory, possibly due to their varying motivational levels to obtain food. More time spent interacting with scents in the field was likely a function of ‘predator inspection’ (risk assessment) once subjects were in a presumed safe enclosure. We conclude this sort of chamber assay can be useful in understanding the contexts and motivational drives inherent to field studies, and may help interpret laboratory results. Our results also suggest more attention should be given to subtle behaviors such as scent inspection in order to better understand how, and when, environmental stimuli evoke fear in rodents.
Introduction
The expression of fear is among the most ancestral and salient behaviors among animals (Blumstein, 2020). Fear can be defined as a putatively adaptive response to predatory threats that is expressed through a suite of ancestral physiological and behavioral responses that function to increase an individual’s likelihood of survival. A better understanding of factors that cause fear (Krishnan, 2014), its physiological effects (Vlaeyen and Linton, 2000; Thompson et al., 2012), and the mechanisms employed to mitigate its consequences (Clinchy et al., 2011; Fendt et al., 2020) are central to understanding anxieties in humans (Gorman, 2008; Kinsella and Monk, 2009), wildlife conservation and welfare (Berger, 2007; Gaynor et al., 2021), and wildlife management (Blumstein et al., 2002; Owen et al., 2017). Predator scents in the form of dander, urine or feces are among the most common olfactory cues used to elicit fearful responses in both laboratory and free-ranging animal assays (Apfelbach et al., 2005; Jones et al., 2016).
Animals may have either overt or covert responses to predatory scents, and these can be influenced by the context under which they experience the stimulus (Owen et al., 2017; Matusz et al., 2019). Such contexts may include: conflicting motivational drives (e.g., aversive and appetitive), variation in the intensity of the fear-evoking cue (Barnes et al., 2002; Hawkins et al., 2007; Sánchez-González et al., 2018), prey vulnerability (Parsons and Blumstein, 2010a; Bilodeau et al., 2013; Kuijper et al., 2013; Apfelbach et al., 2015), visual peripheral information (De Franceschi et al., 2016), climatic conditions (Orrock and Danielson, 2009), or as a function of environmental enrichment (Rampon et al., 2000). Yet our understanding of fear in rodents has been somewhat limited because the factors that cause fear in domesticated, laboratory animals are quite different to what naturally causes fear among wild animals (Bligh et al., 1990; Apfelbach et al., 2005; Yin et al., 2011; Cremona et al., 2015; Mobbs et al., 2018).
Despite the myriad of factors that drive fear, standardized laboratory studies trade-off ecological validity for greater control of confounding variables (Bateson and Martin, 2021). Thus, these studies remove the natural contexts that may determine the direction (or valence) of the outcome. In contrast, wild animals live in environments that trigger conflicting motivational drives, and thus, might react more strongly to the drive that was strongest at the time of stimulus, For instance, a hungry animal in the wild may be less likely to avoid, or withdraw from, a predator scent, if the scent is near a potential food source. Similarly, a reproductively mature animal may be less likely to withdraw from a predator scent if a potential mate is nearby. Lastly, an animal in a risky environment such as under a full moon (which might increase the ability of predators to locate prey) in the absence of shelter, may respond more strongly to predatory cues (Orrock et al., 2004; Orrock and Danielson, 2009). The divergence of field and laboratory results is especially notable in research involving behavior and endocrinology, because such studies are particularly susceptible to environmental influences (Calisi and Bentley, 2009).
Laboratory and field studies may also differ in the type and duration of responses typically being quantified. Many field studies that use predator cues are applied studies, and thus, primarily seek to attract or deter wildlife (Greggor et al., 2020). Researchers in these types of projects are not seeking answers to pathological questions requiring physiological data. Instead, they are examining responses such as: reduced foraging time or effort (Mills and Wajnberg, 2008), the amount of food left in a food patch (GUD; giving up density (Haapakoski et al., 2018)), or the amount of time spent in an agriculture area that is ‘guarded’ with a predator scent. Such responses might simply be due to repugnance, distaste, or aversion and have little relation to actual fear (Nolte et al., 1994; Kimball and Nolte, 2006). Therefore, applied response variables often include more overt responses such as flight (Parsons and Blumstein, 2010b), flight initiation distance (Cooper Jr. and Frederick, 2007), or retreats from a preferred food source (Parsons and Blumstein, 2010a). By contrast, laboratory research focused on pharmacology or pathology might be more likely to measure physiological parameters, or more sensitive measures that are not easily measured in the field such as grooming (van Erp et al., 1994), motor coordination (Deacon, 2013), or even eye-tracking (Payne and Raymond, 2017). Thus, field tests intended for laboratory research must be sensitive enough to consider subtle, as well as obvious, responses.
While the contexts under which adaptive responses to predatory threats may vary, the responses to a predator cue can also vary, and even change over time. In a recent study, wild urban brown (Rattus norvegicus) and black (Rattus rattus) rats, at first, displayed no obvious responses to cat fur, either behaviorally or physiologically (as measured by quantifying glucocorticoids). However, over a period of weeks, subtle changes in behavior were established (Fardell et al., 2021), suggesting risks are assessed over time with increased exposure and perceived severity of the threat. Yet even though behaviors changed, their physiology remained unchanged, and the authors suggested this change would have gone undetected in the laboratory (Fardell et al., 2021). The specific nature of risk assessment might be partly responsible for the misinterpretation of laboratory and especially field experiments. Prey will often approach scents to obtain more information about them, a behavior referred to as ‘predator inspection’ (FitzGibbon, 1994; Fishman, 1999), rather than forgoing foraging, mating or other resource-gathering opportunities when risks are not substantial (Krebs et al., 1983). To an applied researcher, when a prey approaches a predator scent it is usually considered as a failure of the scent to repel an animal, but it could just as likely reflect the animal’s motivation to better assess risks before giving up resources (Pitcher et al., 1986; Brown, 1999).
Though there are several factors in which laboratory studies and field studies differ, it appears that laboratory studies show more consistent responses as compared to the field (Apfelbach et al., 2005). In particular, rodent responses to predator cues, when studied under field conditions, are often more variable and less predictable (Stryjek et al., 2021). Clearly field studies, sometimes referred to as ‘real world’ studies (Matusz et al., 2019), demonstrate a range of contexts that are more consistent with conditions experienced by people expressing pathological conditions (Oppenheim, 2019). It has thus been argued that a better understanding of the actual contexts or motivational drives that alleviate fear is necessary to ensure future breakthroughs in psychology and medicine (Manjili, 2013; Drucker, 2016; Oppenheim, 2019; Fendt et al., 2020). Yet, the principal reason more field studies have not been used reflects the difficulties of studying wild animals in accessible field settings (Mobbs et al., 2018; Stryjek et al., 2021).
As a first step toward merging laboratory and field approaches, the most parsimonious approach may be to simply move laboratory-style behavioral testing chambers into the field and incorporate additional species, contexts, or motivational drives (Stryjek et al., 2021). Chambers provide an enclosed area to isolate and monitor target animals that enter, while also reducing risk of direct predation, since animals are not exposed to an open field or moonlight (Orrock et al., 2004; Orrock and Danielson, 2009). The goals of this study were firstly to examine the ability of a chamber assay to elicit subtle and overt behavioral responses from the dual-motivation (appetitive and aversive) drives of two species of peri-urban, wild rodents. For the second goal of the study, we hypothesized that two species of wild rodents (Apodemus agrarius and Apodemus flavicollis), would spend less time inside a chamber ‘guarded’ by predator scents than a control chamber, and respond more overtly to scents from predators than scents from a herbivore or two types of control.
Materials and methods
Study site
This study was conducted on a private property in a suburban area of Warsaw, central Poland (52°20′20.00″N; 21°03′30.00″E, altitude: 80 m). The landscape was dominated by meadows surrounded by forests and was very sparsely built-up. On this property, two populations of wild striped field mice (A. agrarius) and yellow-necked mice (A. flavicollis) were identified near bushes in very close vicinity to meadows and forests.
Subjects
We selected two wild species of mice (A. agrarius and A. flavicollis) because they are closely-related to the most common laboratory animal, Mus musculus (Rubtsov et al., 2015; Knitlová and Horáček, 2017), and because they are abundant within our study area (Goszczyński, 1979; Matić et al., 2007). There has been minimal laboratory work done with these species (Wang et al., 2011). Though, as a forest-dwelling species, A. flavicollis are said to display a wide range of defensive responses to predator (Mustela nivalis) odor in the field (Borowski, 2000). Individuals were not marked, but based on the frequency of visits, body size, observable differences in coat patterns, and individual characteristics such as scars, or crooked tails, we positively identified 20 unique individuals (12 A. agrarius and 8 A. flavicollis). Based on previous trapping in the area (Stryjek, pers. comm), the population size was likely between 50 and 100 individuals of each species. All tested animals were identified as adults based on their body size. We were unable to identify the sex of tested animals because the video vantage was from above.
Chambers
Chambers have long-been used in laboratory research with rodents (Toubas et al., 1990; Maren, 2008; Qiu et al., 2014; La-Vu et al., 2020). In particular, several neuroscientists requested we help develop field-versions of captive chamber assays so as to provide more natural insights into the observed laboratory responses (Parsons, pers. comm). Of course, these neuroscientists recognize that chambers are artificial structures. Yet, animals adapted to urban environments, will obviously utilize human-made harborage. Because animals have an opportunity to habituate to chambers, and due to the provision of shelter from predators and the elements, chambers can be frequently utilized even without food attractants. Once animals are inside, a range of natural contexts can be recorded. Chambers are particularly good for testing olfactory scent discrimination because they enclose a scent within a sheltered environment that can be more easily contained and monitored than in an open field. Multiple chambers can be used so that there is always a control available so that we minimize the risk of animals not returning to the chambers. Controls can therefore be spatial (in alternate chambers) or temporal, according to the treatment schedule (Table 1). Therefore, we constructed two chambers (Figures 1A, C) made of 12 mm waterproof plywood which were painted with odorless acrylic paint (Luxens, Leroy Merlin, France). The internal floor dimensions were 35 × 40 cm with walls 70 cm high. Plastic sewer pipes (70 mm diameter and 50 cm long; Certus, Cieszyn, Poland) were attached to the entrance holes. The bottom of each chamber was covered with 1 cm of rinsed sand and replaced after every treatment. We pseudo-randomized treatments by using separate Latin Square Designs for each chamber, arranged so that only one predator scent would be present at any given time.
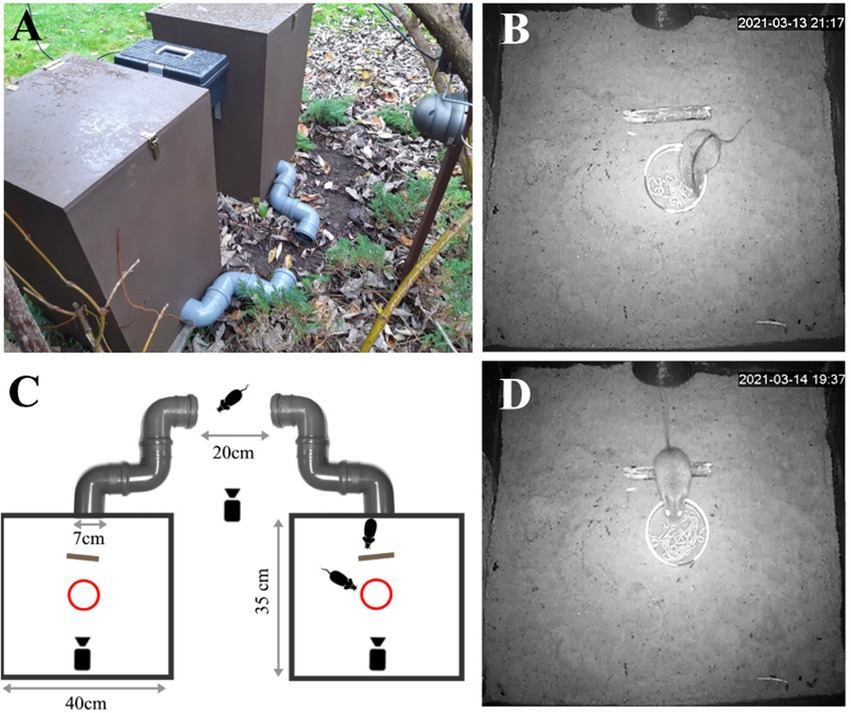
Figure 1. Experimental design. (A) Two wooden chambers deployed in a garden bordered by forest and meadows; (B) A video frame showing a striped field mouse (Apodemus agrarius) feeding inside a chamber; (C) Top view of the experimental setup schematic; (D) A video still showing a yellow-necked mouse (Apodemus flavicollis) inside the chamber.
Scent probes
We selected scents from non-native canids and felids because we were interested in innate responses (e.g., this allowed us to standardize animal experience with predators across the population). We assumed that prey animals this small should have potentially fearful responses to a variety of predators (Preisser and Orrock, 2012; Apfelbach et al., 2015), particularly given the mechanism underlying such responses (Ferrero et al., 2011). Thus, we expected a strong response to scents from both canids and felids. Samples of coyote urine (Canis latrans) were purchased from PredatorPee Inc. (Bangor, ME, United States). Urine was collected at the Warsaw Zoo from male and female African lions (Panthera leo) that had been fed meat from rabbit and beef. Urine was collected via a purpose-built sewer system that enabled urine collection from a slanted concrete floor. The urine was bottled and kept in a refrigerator at + 3°C until use. Rabbit urine was collected directly from a cage of an uncastrated adult male rabbit (Oryctolagus cuniculus) and used as a treatment directly after collection.
Procedure
The experiment took place between 23 January and 9 April 2021 during the winter and spring season. Temperatures ranged from –17 to 20°C. Prior to the actual tests, animals had been lured and habituated to the bait and the chambers for ca. 3 months. Animals were baited to the chambers in the evenings (just after dusk), because this time period reflects the usual peak of nocturnal rodent activity (Stryjek et al., 2013). We used 5 g of chocolate-nut cream (Nuss Milk Krem; i.e., Nutella™ (Weihong and Veitch, 1999; Jackson et al., 2016; Stryjek et al., 2019). To allow for visual assessment of food intake, the food spread was applied via syringe evenly on the surface of 70 mm Petri dishes. The vials were cleaned with a scentless soap and fresh portion of the cream was provided daily. To eliminate possible scent markings and any possible treatment odors, the entrance pipes were thoroughly cleaned with a scentless soap and a 1-cm-thick sand layer was replaced before each trial.
Behaviors recorded
We recorded the total duration (seconds) and the number of occurrences of the following behaviors. Visits-the number of times animals were captured on video entering the chamber. Time spent inside chamber-total time in seconds that animals spent inside the chamber. Flight-the number of times an animal obviously retreated quickly, usually by backing away from the cue, often while vigilant. Freeze-a sudden immobility of the whole body, usually lasting less than 1 s. Withdrawal-the number of times the animal slowly or methodically backed away from the cue, whether obviously vigilant or not. Foraging effort-the amount of time spent consuming or interacting with the food bowl. Scent inspection-the number and time spent (s) interacting (e.g., non-incidental touching or sniffing) with the scent probe.
On treatment days, 1 ml of coyote, lion or herbivore control (rabbit) urine was applied to scent probes made from 10 cm-long × 12 mm thick birch twigs that were placed 4 cm from vials (Figures 1B–D). In the second chamber, 1 ml of tap water was applied as a first type of ‘wet’ control. We also used a dry control (a dry scent probe that otherwise had no scent applied to it) because water can be an attractant, or even enhance scents. All scents, except the dry scent probe, were alternated through the two chambers randomly such that there was always one ‘safe’ chamber and one chamber with a predatory scent (i.e., all subjects had two choices, food + scent, or food + control; Table 1). Each scent and the wet probe were presented three times (in three blocks) with each exposure lasting 2 days. The dry scent probe was then presented for up to 4 days between each of the three blocks. We presented the dry probe for this length of time because having a scent present in either chamber might lead to a negative valence throughout the area (sometimes called an area effect) and cause animals to avoid both chambers, and thus prematurely end the study as occurred in (Parsons and Blumstein, 2010b). For remote monitoring, we connected a series of three infrared cameras with motion detection (Easycam EC-116-SCH; Naples, FL, United States) to a single digital video recorder (Easycam EC-7804 T; Naples, FL, United States). This equipment allowed detection and subsequent recording at all hours for the duration of the study.
Statistics
We used Poisson regression to assess whether each behavior significantly differed by treatment, using the GENMOD procedure using log link and each mouse species as repeated subjects. For each outcome of interest, we re-fitted the Poisson model and altered the treatment reference category in order to identify significantly different pairs of treatments (e.g., pairwise comparisons). To assess whether there were significant differences in responses between the two species, we used Poisson regression with an interaction term between species and treatment. We report our results as Incident Rate Ratios (IRR) where a rate of 1.0 implies the outcome occurs at the same rate between treatments. We used a t-test to determine whether there were differences between chambers. Analyses were performed using SAS/STAT (Cary, NC, United States, Version 9.4).
Ethics statement
This observational study was a largely non-invasive experiment based on the surveillance of free-ranging animals that were free to enter or ignore experimental chambers with food and video cameras. Thus, it did not require permission of the local ethics committee for animal experimentation. The study was carried out on private land with permission of its owners, and all procedures were conducted in accordance with the Polish Animal Protection Act (August 21, 1997). The study was designed and carried out in compliance with the ARRIVE guidelines (Kilkenny et al., 2010).
Results
Visits and overt responses by wild animals
Nearly all instances that a palatable food reward was available in the chamber elicited ‘voluntary’ visitation into the test chambers by two species of wild rodents (264 visits from 266 baits). Overt changes rarely occurred in this study. Flight only occurred twice throughout the project and one instance happened during a control phase. Animals never demonstrated withdrawal nor froze.
Time spent in the chamber
When compared to the dry scent probe, mice spent a total of 75% more time (IRR = 1.74) when the coyote urine treatment was present (p < 0.0001; Figure 2). The total time spent in the chamber was 380% (IRR = 3.84) longer when the lion treatment was present (p < 0.0001). The herbivore treatment, rabbit, was not significantly different from the dry probe. The total time spent in the chamber was 65% (IRR = 1.65) longer when the wet scent probe control (water) was present (p < 0.0001). Both species spent sufficient time inside the chamber to enable monitoring of their full range of behaviors. Once inside the chamber, A. agrarius spent 84.5 s inside, while A. flavicollis spent an average of 106.5 s inside. Though, the total time in chamber was not significantly different between the two species (p > 0.1). There were several species × treatment interactions. Compared to A. flavicollis, when the lion treatment was present, the total time by A. agrarius in the chamber was four times longer than when the dry scent probe control was present (IRR = 4.03; p < 0.0001)). Compared to A. flavicollis, when the rabbit treatment was present, A. agrarius’ total time in the chamber was three times longer than when the dry scent probe control was present (IRR = 2.99; p < 0.05). Compared to A. flavicollis, when the water control was present, A. agrarius time in the chamber was four times longer than when the dry scent probe control was present (IRR = 4.03; p < 0.05). Animals did not show a preference for either chamber; animals spent an average of 278.6 s in chamber A and 279.9 s in chamber B (p > 0.9).
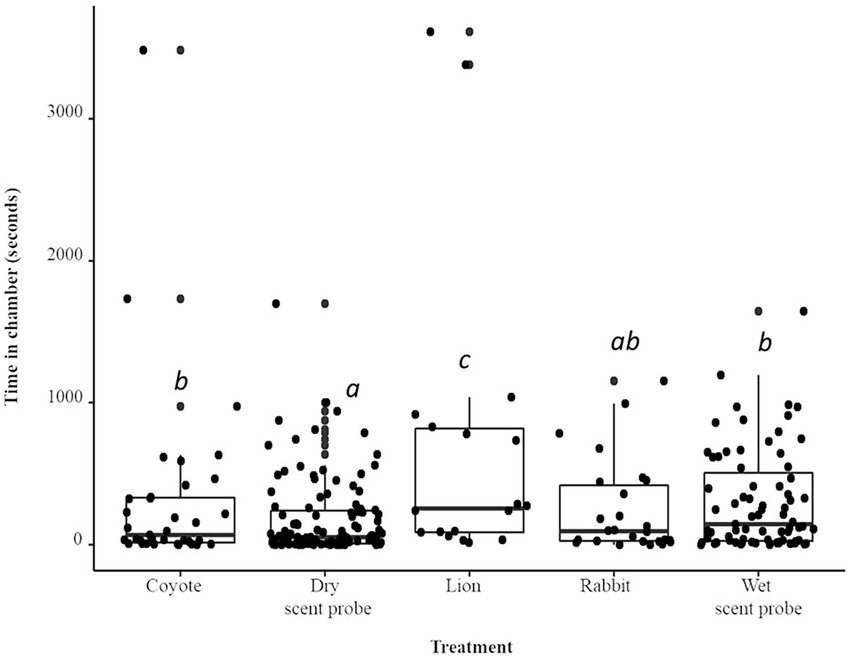
Figure 2. Boxplot and jittered scatterplot of visits to the chamber by two species of wild rodent, striped (A. agrarius) and yellow-necked (A. flavicollis) mice. Pairwise comparisons in superscript are significant at the level of p < 0.005.
Scent inspection: Interactions with the scent probe
Compared to the dry scent probe, when the lion treatment was present there were nearly five times more interactions with the stick (IRR = 4.92; p < 0.001; Figure 3), there were 3.5 times more interactions (IRR = 3.49) when the coyote treatment was present (p < 0.0001). The animals interacted with the scent probe 3.4 times more often (IRR = 3.38) when rabbit scent was present (p < 0.0001). The number of interactions with the scent probe when the water treatment was present was not significantly different from the dry stick (p > 0.1). There was a species × treatment interaction. As compared to A. flavicollis, when the water control was present, A. agrarius interacted with the scent probe 4.5 times more often compared to the dry scent probe (IRR = 4.48; p < 0.05).
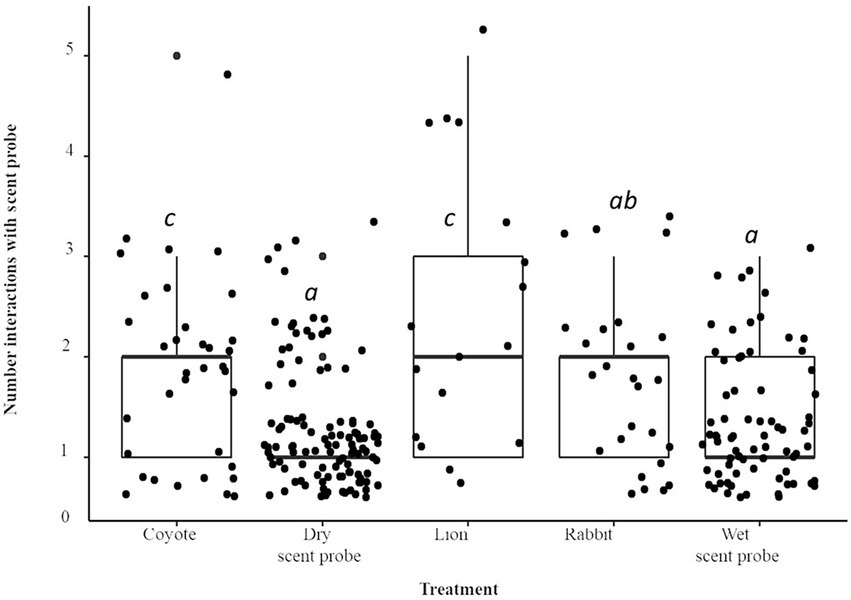
Figure 3. Boxplot and jittered scatterplot of interactions with the scent probe by two species of wild rodent, striped (A. agrarius) and yellow-necked (A. flavicollis) mice. Pairwise comparisons in superscript are significant at the level of p < 0.005.
Foraging effort: Time spent at bowl
Compared to the dry scent probe, the total time spent interacting with the bowl was 78% longer (IRR = 1.78) when the coyote treatment was present (p < 0.001; Figure 4). The total time spent interacting with the bowl was 45% longer (IRR = 1.48) when the lion treatment was present (p < 0.001). The rabbit treatment was not statistically significant. The total time spent interacting with the bowl was 40% longer (IRR = 1.40) when the water control was present. There was a species × treatment interaction: A. flavicollis spent 12% more time interacting with the bowl as compared to A. agrarius (IRR = 1.12; p < 0.05).
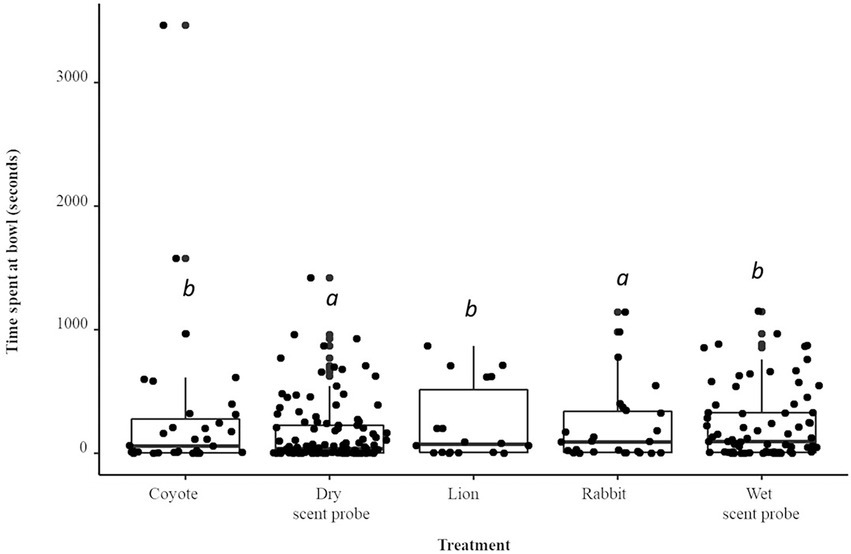
Figure 4. Boxplot and jittered scatterplot of foraging effort by two species of wild rodent, striped (A. agrarius) and yellow-necked (A. flavicollis) mice. Pairwise comparisons in superscript are significant at the level of p < 0.005.
Discussion
Wild rodents differ from laboratory animals in that they are typically more genetically variable, they are less likely to be satiated, and they are influenced by a suite of natural conditions. All of these facets are experimentally controlled in laboratory situations. By placing well-known laboratory-style chambers (Toubas et al., 1990; Maren, 2008; La-Vu et al., 2020) in close proximity to known wild rodent populations, we were able to successfully attract and study overt and subtle responses shown by two species of free-ranging rodents. Both species are closely-related to the most common laboratory rodents, Mus musculus (Knitlová and Horáček, 2017). Unlike most traditional tests where captive animals are forced to interact with a cue, animals that are attracted to an assay, ‘choose’ to interact.
Several factors led us to believe that this bioassay could be potentially promising. As in many field studies, we were able to directly compare the influence of dual-motivational drives (food vs. predator scents) on wild rodents. This assay is unique to the field however, in that it mimics a laboratory assay, whereby experimental areas are divided into zones, compartments and entry pipes that can be alternated between treatments and controls. Unlike traditional field tests, the high walls and enclosure of the chamber assay may help rodents feel ‘safe’. Some rodents prefer enclosed spaces, in particular wall surfaces (e.g., thigmotaxis; Harris et al., 2009; Stryjek and Modlińska, 2013) because they perceived reduced predation risk in these areas. Indeed, virtually all individuals that entered the chamber eventually foraged in the experimental chamber. We limited our remote sensing to three bottom-facing, infrared, motion-detecting cameras, and from this information we were able to detect individuals. However, one could also capture animals directly in the chambers and microchip animals to improve confidence of individual identity. Because we assume that animals in the wild are not satiated as laboratory animals are, we suggest this could be a potentially promising assay to understand dual-motivational drives.
To address our second goal of the experiment, we were able to determine how both species differed in their responses to multiple predator scents and two types of controls. We have also learned that wild rodents responded in both overt and subtle ways to predator scents as compared to an herbivore scent. While we were aware that animals often approach a predator scent, we were surprised at the magnitude of the response (e.g., a positive valence toward a risk scent is almost perceived as a type of ‘attraction’; Parsons et al., 2018). Indeed, our experimental assay, designed with multiple days of the dry scent probe being present in both chambers, was intended to discourage animals from not returning to forage because they perceived the chamber as particularly aversive or fearful. The increased visitation in proximity to lion feces is consistent with the mice obtaining additional information about a potential threat prior to responding (Dugatkin and Godin, 1992). This type of predator inspection is common among small vertebrates (Pitcher et al., 1986; Dugatkin and Godin, 1992; Brown, 1999; Brown and Paige, 2000; Parsons et al., 2018). Lastly, they employed subtle behaviors (such as scent inspection) that implies animals felt safe enough inside the enclosure to investigate a cue that could represent danger had it been provided under riskier contexts.
The conflicting drives of highly-palatable food and predator scents presented concurrently could have attenuated the overall strength of the response. For instance, it is possible that had no attractant been available, then rodents could have had a stronger aversive response when approaching the scents. This could partly explain the perception that predator scents in the laboratory have stronger or more consistent responses. Again, we can only assume that risk assessment by inspection allowed the animals to recognize a tolerable level of risk in these scents. It is likely that these approaches allowed the mice to attend to parameters such as the recentness or ‘freshness’ of the scents (Barnes et al., 2002; Hawkins et al., 2007) or intensity of the cue (Preisser and Orrock, 2012). Predators have been historically active year-round in this region, and thus we expect mice to remain sensitive to predator cues even in the coldest weather. It is possible, however, that mice might have to investigate scents more closely during cold temperatures because the volatile constituents might not disperse as well as during warmer weather (Muller-Schwarze, 2006). We also wonder whether the mice would have responded differently to native predators. While we assume that defenseless mammals should be fearful of any predator scent, known or unknown (Apfelbach et al., 2015), we wonder if previous experience with the predator would have resulted in a faster decision (Apfelbach et al., 2005). Regardless, a primary function of inspection may be to approach close enough to engage other modalities (Munoz and Blumstein, 2012; Lecker et al., 2015) and, by doing so, acquire more information about the true risk of predation.
The subtle changes we found, however, matter in an ecological context because they represent lost time feeding and changes to the energy budget from the trade-off in feeding effort (Lima and Dill, 1990; Lima, 1998; Lima and Bednekoff, 1999; Luttbeg and Sih, 2004; Luttbeg, 2017). Additionally, subtle responses illustrate the potential for variable responses to threats. In some circumstances, subtle responses can be strengthened by modifying the intensity (Preisser and Orrock, 2012), and/or the duration of the predator cue (Lecker et al., 2015; Parsons et al., 2018) or including other modalities (Munoz and Blumstein, 2012) to reinforce the cues in targeted applications (Lecker et al., 2015). The idea here is that a subtle cue can be amplified synergistically or additively by other cues. So for applied outcomes, even the most subtle of responses should not be ignored, because subtle consistent responses could be useful (Lecker et al., 2015). For laboratory support or validation, our findings show that other factors contributed to the direction of the response. Importantly, we think we have shown that context mattered as much as the type of cue being measured. We assessed interactions between species and treatment to rule out species differences in explaining overall responses. However, interactions between species were minimal with both species approaching predator scents.
We conclude that the assay was successful in recruiting rodents into laboratory chambers placed in the wild where a range of contexts may be assessed using genetically-variable animals. Our results also suggest the importance of feeding drive as a motivation for prey animals to engage in tests while taking risks to approach a predator scent. Chamber studies may be a relatively easy and convenient means to attract large populations of wild rodents, whom we assume will have relatively natural responses, while maintaining a high degree of control over their identification and behaviors over time. The contexts that were most important in the field, could be targeted for replication in the laboratory, while remaining contexts deemed as ‘noise’ could be removed for standardization.
Future directions
A key benefit of laboratory research is that the number of individuals can be specified with precision whereas in the field, we must rely on animals electing to participate in these experiments. And, in the field, there is the risk of inadvertent pseudo-replication (which may not be a huge problem depending upon individual differences (Oksanen, 2001)). If wild individuals were trapped before the study and given passive integrative transponder (PIT) tags, tracking free-living individual responses to treatments would be possible (Parsons et al., 2016, 2019). It is also possible that machine learning algorithms could be used to remotely identify individual rats from their dorsal pelage and/or behavior. Even with relatively small sample sizes, field results may highlight differences exhibited by captive animals and this may permit creating more realistic captive situations. For instance, while testing the effects of predator scents on two species of wild mice, Stryjek et al. (2021) discovered a unique (and previously unknown) behavior that occurred only in very low temperatures that would not have been possible to discover in a standard laboratory assay because the temperature was a variable that was precisely controlled. It is also likely that a range of variables traditionally used in the laboratory can be measured inside a field chamber. Such variables may include fecal glucocorticoids, temperature fluctuations (Stryjek et al., 2021), scales to record changing weights (Parsons et al., 2016), speed of movement, grooming (van Erp et al., 1994) and eye-tracking (Payne and Raymond, 2017) among other possibilities. We look forward to the insights gained from realistic field assays and to these insights being used to create more realistic laboratory assays to study fear.
Author’s note
Predator scents often elicit greater antipredator responses to prey species in the laboratory than in the field. More field assays are needed to support compelling laboratory findings. Two species of wild mice, Apodemus agrarius and Apodemus flavicollis, do not have detectable antipredator responses to predator scents in the field. Wild mice instead approach predator scents placed inside the test apparatus. These subtle, but meaningful responses, might reflect predator scent inspection, rather than escape. Field assays can help validate laboratory findings and provide a more realistic understanding of fear responses.
Data availability statement
The raw data supporting the conclusions of this article will be made available by the authors, without undue reservation.
Ethics statement
Ethical review and approval was not required for the animal study because this observational study was a largely non-invasive experiment based on the surveillance of free-ranging animals that were free to enter or ignore experimental chambers with food and video cameras. Thus, it did not require permission of the local ethics committee for animal experimentation. The study was carried out on private land with permission of its owners, and all procedures were conducted in accordance with the Polish Animal Protection Act (August 21, 1997). The study was designed and carried out in compliance with the ARRIVE guidelines (Kilkenny et al., 2010).
Author contributions
RS and MP designed the experiment and wrote the first version of the manuscript. RS conducted the study. RS and MC scored videos. MP, RS, PB, MF, DB, YK, MC, and JM-S participated in the writing and editing of the manuscript. All authors contributed to the article and approved the submitted version.
Funding
This project was self-funded by the authors.
Acknowledgments
We thank Andrzej Kruszewicz, Anna Jakucińska, and Janusz Rak from the Warsaw Zoo (Warszawskie Zoo) for permission, and assistance, in collection of lion urine samples.
Conflict of interest
The authors declare that the research was conducted in the absence of any commercial or financial relationships that could be construed as a potential conflict of interest.
Publisher’s note
All claims expressed in this article are solely those of the authors and do not necessarily represent those of their affiliated organizations, or those of the publisher, the editors and the reviewers. Any product that may be evaluated in this article, or claim that may be made by its manufacturer, is not guaranteed or endorsed by the publisher.
References
Apfelbach, R., Blanchard, C. D., Blanchard, R. J., Hayes, R. A., and McGregor, I. S. (2005). The effects of predator odors in mammalian prey species: a review of field and laboratory studies. Neurosci. Biobehav. Rev. 29, 1123–1144. doi: 10.1016/j.neubiorev.2005.05.005
Apfelbach, R., Parsons, M. H., Soini, H. A., and Novotny, M. V. (2015). Are single odorous components of a predator sufficient to elicit defensive behaviors in prey species? Front. Neurosci. 9:263. doi: 10.3389/fnins.2015.00263
Barnes, M. C., Persons, M. H., and Rypstra, A. L. (2002). The effect of predator chemical cue age on antipredator behavior in the wolf spider Pardosa milvina (Araneae: Lycosidae). J. Insect Behav. 15, 269–281. doi: 10.1023/A:1015493118836
Bateson, M., and Martin, P. (2021). Measuring Behaviour: An Introductory Guide. Cambridge: Cambridge University Press.
Berger, J. (2007). Fear, human shields and the redistribution of prey and predators in protected areas. Biol. Lett. 3, 620–623. doi: 10.1098/rsbl.2007.0415
Bilodeau, F., Gauthier, G., and Berteaux, D. (2013). Effect of snow cover on the vulnerability of lemmings to mammalian predators in the Canadian Arctic. J. Mammal. 94, 813–819. doi: 10.1644/12-MAMM-A-260.1
Bligh, J., Voigt, K., Braun, H., Brück, K., and Heldmaier, G. (1990). Thermoreception and Temperature Regulation. Springer: New York, NY.
Blumstein, D. T. (2020). The Nature of Fear: Survival Lessons From the Wild. Harvard University Press, Cambridge, MA.
Blumstein, D. T., Mari, M., Daniel, J. C., Ardron, J. G., Griffin, A. S., and Evans, C. S. (2002). Olfactory predator recognition: wallabies may have to learn to be wary. Anim. Conserv. 5, 87–93. doi: 10.1017/S1367943002002123
Borowski, Z. (2000). The odour of terrestrial predator as an olfactory risk signal detected by rodents, and its consequences. Biol. Bull. Poznan 37, 59–169.
Brown, G. E. (1999). Godin J-GJ: who dares, learns: chemical inspection behaviour and acquired predator recognition in a characin fish. Anim. Behav. 57, 475–481. doi: 10.1006/anbe.1998.1017
Brown, G. E., and Paige, J. A. (2000). Godin J-GJ: chemically mediated predator inspection behaviour in the absence of predator visual cues by a characin fish. Anim. Behav. 60, 315–321. doi: 10.1006/anbe.2000.1496
Calisi, R. M., and Bentley, G. E. (2009). Lab and field experiments: are they the same animal? Horm. Behav. 56, 1–10. doi: 10.1016/j.yhbeh.2009.02.010
Clinchy, M., Schulkin, J., Zanette, L. Y., Sheriff, M. J., McGowan, P. O., and Boonstra, R. (2011). The neurological ecology of fear: insights neuroscientists and ecologists have to offer one another. Front. Behav. Neurosci. 5:21. doi: 10.3389/fnbeh.2011.00021
Cooper, W. E. Jr., and Frederick, W. G. (2007). Optimal flight initiation distance. J. Theor. Biol. 244, 59–67. doi: 10.1016/j.jtbi.2006.07.011
Cremona, T., Mella, V. S., Webb, J. K., and Crowther, M. S. (2015). Do individual differences in behavior influence wild rodents more than predation risk? J. Mammal. 96, 1337–1343. doi: 10.1093/jmammal/gyv142
De Franceschi, G., Vivattanasarn, T., Saleem, A. B., and Solomon, S. G. (2016). Vision guides selection of freeze or flight defense strategies in mice. Curr. Biol. 26, 2150–2154. doi: 10.1016/j.cub.2016.06.006
Drucker, D. J. (2016). Never waste a good crisis: confronting reproducibility in translational research. Cell Metab. 24, 348–360. doi: 10.1016/j.cmet.2016.08.006
Dugatkin, L. A., and Godin, J.-G. J. (1992). Prey approaching predators: a cost-benefit perspective. Ann. Zool. Fennici. JSTOR 29, 233–252.
Fardell, L. L., Bedoya-Pérez, M. A., Dickman, C. R., Crowther, M. S., Pavey, C. R., and Narayan, E. J. (2021). Are physiological and behavioural responses to stressors displayed concordantly by wild urban rodents? Sci. Nat. 108, 1–15. doi: 10.1007/s00114-020-01716-8
Fendt, M., Parsons, M. H., Apfelbach, R., Carthey, A., Dickman, C. R., Endres, T., et al. (2020). Context and trade-offs characterize real-world threat detection systems: a review and comprehensive framework to improve research practice and resolve the translational crisis. Neurosci. Biobehav. Rev. 115, 25–33. doi: 10.1016/j.neubiorev.2020.05.002
Ferrero, D. M., Lemon, J. K., Fluegge, D., Pashkovski, S. L., Korzan, W. J., Datta, S. R., et al. (2011). Detection and avoidance of a carnivore odor by prey. Proc. Natl. Acad. Sci. 108, 11235–11240. doi: 10.1016/j.neubiorev.2020.05.002
Fishman, M. A. (1999). Predator inspection: closer approach as a way to improve assessment of potential threats. J. Theor. Biol. 196, 225–235. doi: 10.1006/jtbi.1998.0834
FitzGibbon, C. D. (1994). The costs and benefits of predator inspection behaviour in Thomson's gazelles. Behav. Ecol. Sociobiol. 34, 139–148. doi: 10.1007/BF00164184
Gaynor, K. M., Cherry, M. J., Gilbert, S. L., Kohl, M. T., Larson, C. L., Newsome, T. M., et al. (2021). An applied ecology of fear framework: linking theory to conservation practice. Anim. Conserv. 24, 308–321. doi: 10.1111/acv.12629
Gorman, J. M. (2008). Fear and Anxiety: The Benefits of Translational Research. American Psychiatric Publishing, Inc. Washington, DC.
Goszczyński, J. (1979). Density estimation for an urban population of the field mouse. Acta Theriol. 24, 417–419. doi: 10.4098/AT.arch.79-38
Greggor, A. L., Berger-Tal, O., and Blumstein, D. T. (2020). The rules of attraction: the necessary role of animal cognition in explaining conservation failures and successes. Annu. Rev. Ecol. Evol. Syst. 51, 483–503. doi: 10.1146/annurev-ecolsys-011720-103212
Haapakoski, M., Hardenbol, A., and Matson, K. D. (2018). Exposure to chemical cues from predator-exposed conspecifics increases reproduction in a wild rodent. Sci. Rep. 8, 1–9. doi: 10.1038/s41598-018-35568-0
Harris, A. P., D'Eath, R. B., and Healy, S. D. (2009). Environmental enrichment enhances spatial cognition in rats by reducing thigmotaxis (wall hugging) during testing. Anim. Behav. 77, 1459–1464. doi: 10.1016/j.anbehav.2009.02.019
Hawkins, L., Magurran, A., and Armstrong, J. (2007). Innate abilities to distinguish between predator species and cue concentration in Atlantic salmon. Anim. Behav. 73, 1051–1057. doi: 10.1016/j.anbehav.2006.08.011
Jackson, M., Hartley, S., and Linklater, W. (2016). Better food-based baits and lures for invasive rats Rattus spp. and the brushtail possum Trichosurus vulpecula: a bioassay on wild, free-ranging animals. J. Pest. Sci. 89, 479–488. doi: 10.1007/s10340-015-0693-8
Jones, M. E., Apfelbach, R., Banks, P. B., Cameron, E. Z., Dickman, C. R., Frank, A., et al. (2016). A nose for death: integrating trophic and informational networks for conservation and management. Front. Ecol. Evol. 4:124. doi: 10.3389/fevo.2016.00124
Kilkenny, C., Browne, W. J., Cuthill, I. C., Emerson, M., and Altman, D. G. (2010). Improving bioscience research reporting: the ARRIVE guidelines for reporting animal research. PLoS Biol. 8:e1000412. doi: 10.1371/journal.pbio.1000412
Kimball, B., and Nolte, D. (2006). “Animal tissue-based herbivore repellents: scary odours or altered palatability” in Advances in Vertebrate Pest Management (Furth, Germany: Filander Verlag), 59–72.
Kinsella, M. T., and Monk, C. (2009). Impact of maternal stress, depression & anxiety on fetal neurobehavioral development. Clin. Obstet. Gynecol. 52, 425–440. doi: 10.1097/GRF.0b013e3181b52df1
Knitlová, M., and Horáček, I. (2017). Late Pleistocene–Holocene paleobiogeography of the genus Apodemus in Central Europe. PLoS One 12:e0173668. doi: 10.1371/journal.pone.0173668
Krebs, J. R., Stephens, D. W., and Sutherland, W. J. (1983). Perspectives in Optimal Foraging. Cambridge: Cambridge University Press.
Krishnan, V. (2014). Defeating the fear: new insights into the neurobiology of stress susceptibility. Exp. Neurol. 261, 412–416. doi: 10.1016/j.expneurol.2014.05.012
Kuijper, D. P. J., De Kleine, C., Churski, M., Van Hooft, P., Bubnicki, J., and Jędrzejewska, B. (2013). Landscape of fear in Europe: wolves affect spatial patterns of ungulate browsing in Białowieża primeval Forest, Poland. Ecography 36, 1263–1275. doi: 10.1111/j.1600-0587.2013.00266.x
La-Vu, M., Tobias, B. C., Schuette, P. J., and Adhikari, A. (2020). To approach or avoid: an introductory overview of the study of anxiety using rodent assays. Front. Behav. Neurosci. 14:145. doi: 10.3389/fnbeh.2020.00145
Lecker, C. A., Parsons, M. H., Lecker, D. R., Sarno, R. J., and Parsons, F. E. (2015). The temporal multimodal influence of optical and auditory cues on the repellent behavior of ring-billed gulls (Larus delewarensis). Wildl. Res. 42, 232–240. doi: 10.1071/WR15001
Lima, S. L. (1998). Nonlethal effects in the ecology of predator-prey interactions. Bioscience 48, 25–34. doi: 10.2307/1313225
Lima, S. L., and Bednekoff, P. A. (1999). Temporal variation in danger drives antipredator behavior: the predation risk allocation hypothesis. Am. Nat. 153, 649–659. doi: 10.1086/303202
Lima, S. L., and Dill, L. M. (1990). Behavioral decisions made under the risk of predation: a review and prospectus. Can. J. Zool. 68, 619–640. doi: 10.1139/z90-092
Luttbeg, B. (2017). Re-examining the causes and meaning of the risk allocation hypothesis. Am. Nat. 189, 644–656. doi: 10.1086/691470
Luttbeg, B., and Sih, A. (2004). Predator and prey habitat selection games: the effects of how prey balance foraging and predation risk. Isr. J. Zool. 50, 233–254. doi: 10.1560/L6QV-UA5T-RDR7-L7QG
Manjili, M. H. (2013). Opinion: Translational research in crisis. Available at: https://www.the-scientist.com/opinion/opinion-translational-research-in-crisis-38724 (Accessed August 5, 2022).
Maren, S. (2008). Pavlovian fear conditioning as a behavioral assay for hippocampus and amygdala function: cautions and caveats. Eur. J. Neurosci. 28, 1661–1666. doi: 10.1111/j.1460-9568.2008.06485.x
Matić, R., Vukićević-Radić, O. D., Stamenković, S., and Kataranovski, D. (2007). Can large home ranges be due to social dominance in Apodemus flavicollis? Archiv. Biol. Sci. 59, P61–P62. doi: 10.2298/ABS0704061M
Matusz, P. J., Dikker, S., Huth, A. G., and Perrodin, C. (2019). Are we ready for real-world neuroscience? J. Cogn. Neurosci. :h6. doi: 10.1162/jocn_e_01276
Mills, N. J., and Wajnberg, E. (2008). “Optimal foraging behavior and efficient biological control methods” in Behavioral Ecology of Insect Parasitoids: From Theoretical Approaches to Field Applications. (Oxford: Blackwell), 3–30. doi: 10.1002/9780470696200.ch1
Mobbs, D., Trimmer, P. C., Blumstein, D. T., and Dayan, P. (2018). Foraging for foundations in decision neuroscience: insights from ethology. Neuroscience 13:19. doi: 10.1038/s41583-018-0010-7
Munoz, N. E., and Blumstein, D. T. (2012). Multisensory perception in uncertain environments. Behav. Ecol. 23, 457–462. doi: 10.1093/beheco/arr220
Nolte, D. L., Mason, J. R., Epple, G., Aronov, E., and Campbell, D. L. (1994). Why are predator urines aversive to prey? J. Chem. Ecol. 20, 1505–1516. doi: 10.1007/BF02059876
Oksanen, L. (2001). Logic of experiments in ecology: is pseudoreplication a pseudoissue? Oikos 94, 27–38. doi: 10.1034/j.1600-0706.2001.11311.x
Oppenheim, R. W. (2019). Adult hippocampal neurogenesis in mammals (and humans): the death of a central dogma in neuroscience and its replacement by a new dogma. Dev. Neurobiol. 79, 268–280. doi: 10.1002/dneu.22674
Orrock, J. L., and Danielson, B. J. (2009). Temperature and cloud cover, but not predator urine, affect winter foraging of mice. Ethology 115, 641–648. doi: 10.1111/j.1439-0310.2009.01654.x
Orrock, J. L., Danielson, B. J., and Brinkerhoff, R. J. (2004). Rodent foraging is affected by indirect, but not by direct, cues of predation risk. Behav. Ecol. 15, 433–437. doi: 10.1093/beheco/arh031
Owen, M. A., Swaisgood, R. R., and Blumstein, D. T. (2017). Contextual influences on animal decision-making: significance for behavior-based wildlife conservation and management. Integr. Zool. 12, 32–48. doi: 10.1111/1749-4877.12235
Parsons, M. H., Apfelbach, R., Banks, P. B., Cameron, E. Z., Dickman, C. R., Frank, A. S., et al. (2018). Biologically meaningful scents: a framework for understanding predator–prey research across disciplines. Biol. Rev. 93, 98–114. doi: 10.1111/brv.12334
Parsons, M. H., and Blumstein, D. T. (2010a). Feeling vulnerable? Indirect risk cues differently influence how two marsupials respond to novel dingo urine. Ethology 116, 972–980. doi: 10.1111/j.1439-0310.2010.01810.x
Parsons, M. H., and Blumstein, D. T. (2010b). Familiarity breeds contempt: kangaroos persistently avoid areas with experimentally deployed dingo scents. PLoS One 5:e10403. doi: 10.1371/journal.pone.0010403
Parsons, M. H., Deutsch, M. A., Dumitriu, D., and Munshi-South, J. (2019). Differential responses by city rats (Rattus norvegicus) toward male or female-produced pheromones in sheltered and high-risk presentations. J. Urban Ecol. 5:juz009. doi: 10.1093/jue/juz009
Parsons, M. H., Sarno, R. J., and Deutsch, M. A. (2016). A detailed protocol to enable safe-handling, preemptive detection, and systematic surveillance of rat-vectored pathogens in the urban environment. Front. Public Health 4:132. doi: 10.3389/fpubh.2016.00132
Payne, H. L., and Raymond, J. L. (2017). Magnetic eye tracking in mice. elife 6:e29222. doi: 10.7554/eLife.29222
Pitcher, T., Green, D., and Magurran, A. (1986). Dicing with death: predator inspection behaviour in minnow shoals. J. Fish Biol. 28, 439–448. doi: 10.1111/j.1095-8649.1986.tb05181.x
Preisser, E. L., and Orrock, J. L. (2012). The allometry of fear: interspecific relationships between body size and response to predation risk. Ecosphere 3, 1–27. doi: 10.1890/ES12-00084.1
Qiu, Q., Scott, A., Scheerer, H., Sapkota, N., Lee, D. K., Ma, L., et al. (2014). Automated analyses of innate olfactory behaviors in rodents. PLoS One 9:e93468. doi: 10.1371/journal.pone.0093468
Rampon, C., Tang, Y.-P., Goodhouse, J., Shimizu, E., Kyin, M., and Tsien, J. Z. (2000). Enrichment induces structural changes and recovery from nonspatial memory deficits in CA1 NMDAR1-knockout mice. Nat. Neurosci. 3, 238–244. doi: 10.1038/72945
Rubtsov, N., Karamysheva, T., Bogdanov, A., Kartavtseva, I., Bochkarev, M., and Iwasa, M. (2015). Comparative analysis of DNA homology in pericentric regions of chromosomes of wood mice from genera Apodemus and Sylvaemus. Russ. J. Genet. 51, 1233–1242. doi: 10.1134/S1022795415120091
Sánchez-González, B., Planillo, A., Navarro-Castilla, Á., and Barja, I. (2018). The concentration of fear: mice’s behavioural and physiological stress responses to different degrees of predation risk. Sci. Nature 105, 1–10. doi: 10.1007/s00114-018-1540-6
Stryjek, R., Kalinowski, A., and Parsons, M. (2019). H: unbiased sampling for rodents and other small mammals: how to overcome neophobia through use of an electronic-triggered live trap-a preliminary test. Front. Ecol. Evol. 7:11. doi: 10.3389/fevo.2019.00011
Stryjek, R., and Modlińska, K. (2013). A thigmotaxis-based method of recapturing and transporting small mammals in the laboratory. Lab Anim. 42, 321–324. doi: 10.1038/laban.328
Stryjek, R., Modlińska, K., Turlejski, K., and Pisula, W. (2013). Circadian rhythm of outside-nest activity in wild (WWCPS), albino and pigmented laboratory rats. PLoS One 8:e66055. doi: 10.1371/journal.pone.0066055
Stryjek, R., Parsons, M. H., and Bebas, P. (2021). A newly discovered behavior (‘tail-belting’) among wild rodents in sub zero conditions. Sci. Rep. 11, 1–7. doi: 10.1038/s41598-021-01833-y
Stryjek, R., Parsons, M. H., Fendt, M., and Święcicki, J. (2021). Bębas P: a methodological review of free-ranging rat assays as context-enriched supplements to traditional laboratory models. J. Neurosci. Methods 362:109303. doi: 10.1016/j.jneumeth.2021.109303
Thompson, R. S., Strong, P. V., and Fleshner, M. (2012). Physiological consequences of repeated exposures to conditioned fear. Behav. Sci. 2, 57–78. doi: 10.3390/bs2020057
Toubas, P. L., Abla, K. A., Cao, W., Logan, L. G., and Seale, T. W. (1990). Latency to enter a mirrored chamber: a novel behavioral assay for anxiolytic agents. Pharmacol. Biochem. Behav. 35, 121–126. doi: 10.1016/0091-3057(90)90215-4
van Erp, A. M., Kruk, M. R., Meelis, W., and Willekens-Bramer, D. C. (1994). Effect of environmental stressors on time course, variability and form of self-grooming in the rat: handling, social contact, defeat, novelty, restraint and fur moistening. Behav. Brain Res. 65, 47–55. doi: 10.1016/0166-4328(94)90072-8
Vlaeyen, J. W., and Linton, S. J. (2000). Fear-avoidance and its consequences in chronic musculoskeletal pain: a state of the art. Pain 85, 317–332. doi: 10.1016/S0304-3959(99)00242-0
Wang, Z., Wang, B., and Lu, J. (2011). Behavioral and physiological responses of striped field mice (Apodemus agrarius) to predator odor. Integr. Zool. 6, 334–340. doi: 10.1111/j.1749-4877.2011.00262.x
Weihong, J., and Veitch, C. (1999). CRAIG JL: an evaluation of the efficiency of rodent trapping methods: the effect of trap arrangement, cover type, and bait. N. Z. J. Ecol. 23, 45–51.
Keywords: aversive and appetitive drives, field contexts, laboratory rodents, lab to field study, landscape of fear, ‘real world’ research, translational research, scent inspection
Citation: Parsons MH, Stryjek R, Bebas P, Fendt M, Blumstein DT, Kiyokawa Y, Chrzanowski MM and Munshi-South J (2023) Why are predator cues in the field not more evocative? A ‘real world’ assay elicits subtle, but meaningful, responses by wild rodents to predator scents. Front. Ecol. Evol. 10:1054568. doi: 10.3389/fevo.2022.1054568
Edited by:
Michael Sheriff, University of Massachusetts Dartmouth, United StatesReviewed by:
Richard Anthony Peters, La Trobe University, AustraliaTinglei Jiang, Northeast Normal University, China
Copyright © 2023 Parsons, Stryjek, Bebas, Fendt, Blumstein, Kiyokawa, Chrzanowski and Munshi-South. This is an open-access article distributed under the terms of the Creative Commons Attribution License (CC BY). The use, distribution or reproduction in other forums is permitted, provided the original author(s) and the copyright owner(s) are credited and that the original publication in this journal is cited, in accordance with accepted academic practice. No use, distribution or reproduction is permitted which does not comply with these terms.
*Correspondence: Michael H. Parsons, ✉UGFyc29ucy5ITWljaGFlbEBnbWFpbC5jb20=; Rafal Stryjek, ✉UnN0cnlqZWtAd3AucGw=
†These authors have contributed equally to this work and share first authorship