- Centre for Functional Biodiversity, School of Life Sciences, University of KwaZulu-Natal, Pietermaritzburg, South Africa
Gas chromatography-electroantennographic detection (GC-EAD), typically combined with a flame ionization detector (FID), is a widely used technique for studying insect semiochemicals. Combining mass spectrometry (MS) with GC-EAD is extremely beneficial, but has seldom been adopted, possibly due to the practical challenges associated with incorporating an MS (under vacuum) into a GC-EAD system. We describe a novel method of incorporating MS into a GC-EAD system, where the FID is maintained in the system rather than being replaced by an MS. With this method, effluent is still split between EAD and FID, with the FID being used to assign components that elicit antennal responses, as in a normal GC-FID/EAD system. The MS is introduced via a second effluent splitter inserted before the split between the EAD and the FID. This method allows for EAD, FID, and MS data to be obtained from a single injection, which is especially useful for analysis of thermally desorbed and solid-phase microextraction (SPME) samples. This configuration is also relatively simple to implement and resolves some of the practical challenges associated with dividing effluent between a detector at atmospheric pressure (a live antenna) and a detector under vacuum (the MS). We present test runs with hawkmoth antennae and floral volatiles to demonstrate the effectiveness of this system, and discuss the challenges and practical solutions to incorporating MS into a GC-EAD system that retains an FID detector.
Introduction
Coupled gas chromatography-electroantennographic detection (GC-EAD) is a widely-used technique to study insect semiochemicals (Struble and Arn, 1984; Cork et al., 1990; Schiestl and Marion-Poll, 2002). The technique uses electroantennography (the use of a live insect antenna as a detector) to identify individual bioactive components in blends of volatile organic compounds (VOCs) that have been separated by capillary-column GC (Schneider, 1957; Moorhouse et al., 1969; Arn et al., 1975). This is achieved by splitting the GC effluent between an insect antenna and a GC detector so that physiological responses by the antenna (depolarization in response to chemical stimulation) can be observed in parallel with the GC detector response in order to locate bioactive compounds in samples.
GC-EAD is typically combined with a flame ionization detector (FID). FID offers good sensitivity to a range of VOC classes and has the advantage of being relatively simple to operate, but identification of active compounds is limited to information about their Kováts retention indices and the shape of peaks. More accurate identification of active compounds requires analysis of samples on a GC coupled to a mass spectrometer (GC-MS). This step can be difficult and time-consuming because chromatograms derived from FID are not identical to those derived from MS (due to their compound-specific differences in sensitivity), and because differences in column parameters and detector operating pressures (atmospheric versus vacuum) induce variation in relative retention times which can make it challenging to link peaks from the FID chromatogram to those of the total ion chromatogram (TIC) from the MS. A further limitation is that some sampling methods, such as thermal desorption and solid-phase microextraction (SPME), require that the entire sample be used for a single injection so sampling has to be replicated to generate a second injection on GC-MS. Given these limitations, there are strong practical benefits to directly combining MS with the GC-EAD set-up in order to obtain both EAD responses and mass spectra (for identification of active compounds) from a single injection. Despite the obvious benefits, very few practitioners routinely operate systems in which an MS is incorporated into the GC-EAD setup.
Previously described GC-EAD systems that have incorporated an MS (Weissbecker et al., 2004; Schott et al., 2013; Bohman and Peakall, 2014; Li et al., 2021) have replaced the FID with an MS. Here, we propose an alternative method of incorporating MS into a GC-EAD system where the FID is maintained in the system. With this method, effluent is still split between EAD and FID, with the FID being used to assign components that elicit antennal responses, as in a normal GC-FID/EAD system. The MS is introduced via a second splitter inserted before the effluent split between the EAD and the FID. Active compounds are identified by manual alignment of the FID chromatogram with the TIC from the MS and comparison of Kováts retention indices on each chromatogram. Using this method, it is possible to obtain EAD, FID, and MS data from a single injection. Combining two detectors (FID and MS) with varying sensitivities to different types of compounds has the advantage of broadening the range of compound types that can be detected. The system described here is particularly valuable for analysis of thermally desorbed samples where repeat injections of a sample are not possible.
This method also resolves one of the common challenges experienced when incorporating an MS into a GC-EAD system: when an FID (operating at atmospheric pressure) is replaced with an MS (operating under vacuum), the column dimensions and outlet pressures after the effluent split are no longer identical resulting in a non-linear relationship between the elution times of individual compounds at the antenna compared to the MS. This is due to the residence time in the deactivated columns between the splitter and the outlets being a function of the linear velocity of the carrier gas, which in turn is a function of column diameter, temperature and outlet pressure (Jennings et al., 1997). This makes it challenging to obtain simultaneous elution of compounds at both the EAD and MS outlets for the duration of a temperature programmed GC run, although the severity of the effect can be ameliorated by using specific lengths and dimensions of deactivated column (established with GC calculators) between the splitter and the EAD and MS outlets. With our method, the MS is introduced before the split between EAD and FID, and the TIC from the MS is not used to assign active compounds, so the effect of the MS on relative retention times (and the resulting non-linear shift in retention times between the antennal output relative to the MS output) does not affect the assignment of active compounds.
The method we describe is relatively simple to implement and resolves some of the practical challenges associated with dividing effluent between a detector at atmospheric pressure (a live antenna) and a detector under vacuum (the MS). The effectiveness of this method is illustrated with examples of EAD results obtained with hawkmoth antennae and floral volatiles.
Materials and equipment
Gas chromatograph, mass spectrometer, and flame ionization detector
The GC-MS/FID system comprises a Bruker 450 gas chromatograph (GC) with a flame ionization detector (FID) coupled to a Varian 1200 single quadrupole mass spectrometer (MS) via a standard transfer line exiting the oven to the right of the GC. The GC was factory-modified to add a second transfer line passing through the flow compartment and exiting the GC to the left. This 40 cm long transfer line was manufactured by Winkler AG (Heidelberg, Germany) and combined with a Horst HT 30 (Horst GmbH, Lorsch, Germany) temperature controller. The GC and MS are operated with Bruker MS Workstation (version 6.8). The GC is fitted with two Bruker 1079 PTV injector ports. For injection of solvent extracts using standard septum injection, injector ports are used with Varian coated 11.5 mm BTO septa (part no: CR298777) and Bruker 54 mm x 5.0 mm outer diameter x 3.4 mm internal diameter split/splitless injector liners with a glass frit (part no: 392611946). For direct thermal desorption of headspace samples, injector ports are modified with a Bruker (formerly Varian) ChromatoProbe thermal desorption device (Amirav and Dagan, 1997; Dötterl et al., 2005) and used with inverted Bruker 54 mm × 5.0 mm outer diameter x 3.4 mm internal diameter split/splitless open/no frit injector liners (part no: 392611945). The two injector ports are connected to a polar fused silica capillary column (SGE SolGel Wax; 30 m × 0.32 mm ID, 0.25 μm film thickness) and a non-polar fused silica capillary column (Restek Rxi-5Sil MS; 30 m × 0.32 mm ID, 0.25 μm film thickness), respectively. The two analytical columns are plumbed in a confluent configuration (Figure 1A; Cork et al., 1990; Shuttleworth and Johnson, 2020) with the distal end of each connected to opposite ports of a Gerstel Graphpack®-3D/2 crosspiece effluent splitter (sulfinert® coated). With this configuration, the column that is not being used for analysis serves to deliver make-up gas to the confluence (Shuttleworth and Johnson, 2020). One of the remaining two ports on the splitter is connected to an 11.8 m length of deactivated fused silica column (0.15 mm ID) which exits the GC oven via a heated transfer line (held at 240°C) leading to the MS on the right. The fourth port is connected to a 0.3 m length of deactivated fused silica column (0.32 mm ID) which leads to a second effluent splitter (Valco Stainless Steel Tee, Inert treated, part no: ZT.5; VICI AG International). The remaining two ports of the second effluent splitter are connected to equal lengths (2 m) of deactivated fused silica column (0.32 mm ID), one of which leads to the FID while the other exits the GC oven via the heated transfer line (held at 200°C) to the left and delivers effluent to the electroantennographic detection (EAD) apparatus. Columns are connected to the Gerstel effluent splitter using Graphpack®-3D/2 metal-jacketed graphite ferrules (suitable for high pressures and temperatures) and Graphpack®-3D/2 screws. Columns are connected to the Valco effluent splitter with VICI Valcon polyimide ferrules (part no: FS. 4–5; VICI AG International).
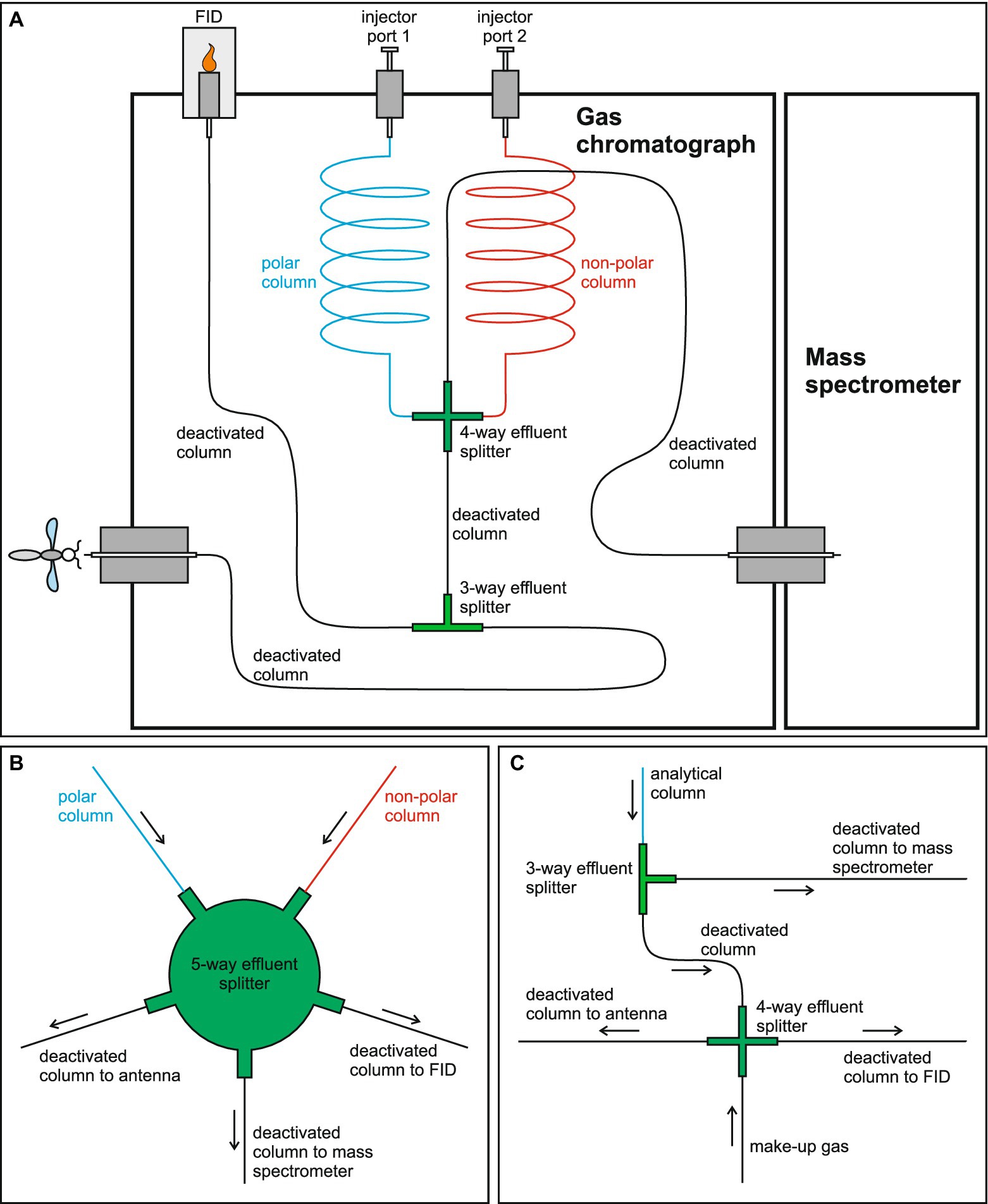
Figure 1. Plumbing diagram for the gas chromatography-electroantennographic detection (GC-EAD) system incorporating a flame ionization detector (FID) and a mass spectrometer (MS) described in this study, and two possible alternative configurations that would likely achieve similar outcomes. (A) Plumbing diagram for the system described and tested in this study. (B) Plumbing diagram for an alternative configuration in which the two effluent splitters are replaced with a single 5-way splitter. (C) Plumbing diagram for a system which incorporates only a single analytical column with an independent source of make-up gas introduced at the split between EAD and FID. Arrows in (B) and (C) indicate the direction of gas flow. The alternative configurations shown in (B) and (C) have not been tested.
Electroantennographic detection apparatus
Electroantennographic detection (EAD) apparatus was supplied by Ockenfels Syntech GmbH (Germany) and comprises an EAG Combi Probe, mounted on an MP-15 micromanipulator and connected to a 2-channel USB Intelligent Data Acquisition Controller (IDAC-2). The IDAC-2 is connected to the FID such that the antennal responses from the probe and FID outputs are recorded simultaneously. Antennae are placed in a stream of filtered air provided by a CS55 Stimulus Controller and delivered to the antenna through an 8 mm internal diameter glass tube at a flow rate of ca. 4 L min−1. Air is humidified by bubbling through distilled water before it reaches the antenna. The tip of the capillary column from the GC is introduced to the glass tube ca. 10 cm before the antenna such that the effluent is carried onto the antennal preparation by the humidified air. To minimize the effect of bench vibration created by the MS, the (wooden) bench was divided into two physically separated parts between the GC and the EAD apparatus. The EAD apparatus is also placed on a TMC TableTop CSP Passive Benchtop Vibration Isolator (TMC-Ametek, Peabody, Massachusetts; part no. 66–501). The EAD apparatus is enclosed in a TMC BenchTop Faraday Cage (TMC-Ametek, Peabody, Massachusetts; part no. 81–334-03) to minimize possible electromagnetic interference emanating from the MS. The EAD apparatus is operated using GCEAD 2014 version 1.2.5 (2014-05-03).
Materials and methods
Representative electroantennographic detection experiments
To demonstrate the effectiveness of this system for EAD experiments, we present three representative examples of test runs conducted with antennae of Temnora pseudopylas hawkmoths (Sphingidae) and either a blend of synthetic volatile standards or samples of floral volatiles collected from Satyrium parviflorum (Orchidaceae) and Rothmannia globosa (Rubiaceae) flowers. Temnora pseudopylas is not a known visitor of these flowers, but the flowers of both species emit blends of volatiles typical of moth-pollinated flowers and were likely to contain some components that would stimulate responses from T. pseudopylas antennae. Floral volatiles were collected with a dynamic headspace extraction method described in Shuttleworth and Johnson (2009). Volatiles from S. parviflorum were sampled (from 25 flowers on a single plant for a sampling duration of 20 min) at a site on Naudes Neck Pass (Eastern Cape Province, South Africa) in February 2013. Volatiles from R. globosa were sampled (from 2 flowers on a single plant for a sampling duration of 15 min) from a garden plant in Pietermaritzburg (KwaZulu-Natal Province, South Africa) in September 2012. The standard blend comprised 21 compounds across a range of compound classes, diluted 1:1,000 in acetone (composition summarized in Table 1). Three microliters of this blend were injected for each run. Moths used for these runs were collected in Howick (KwaZulu-Natal, South Africa) in April 2022. For each of the EAD experiments presented here, the moth’s antenna was excised at the base and tip and was mounted between pulled glass pipettes filled with ¼ strength Ringer solution (prepared from tablets supplied by Merck, Germany, catalog no: 1.15525.0001). Pipettes were held in stainless steel electrode holders with 0.4 mm diameter silver wires connecting the Ringer in the pipettes to the indifferent electrode (base of the antenna) and the EAG Combi Probe (tip of the antenna).
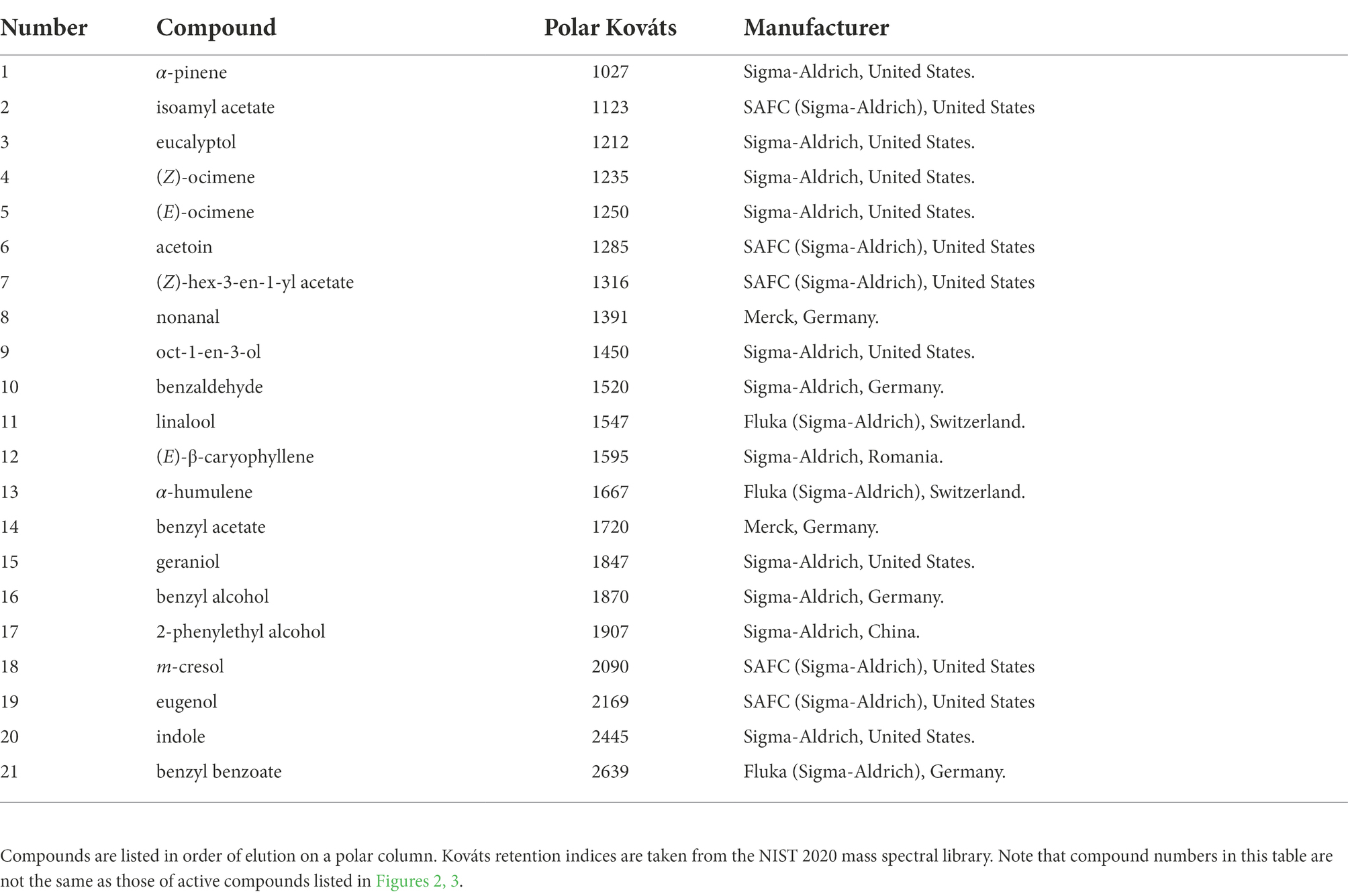
Table 1. Synthetic standards included in the blend used for the test injections with Temnora pseudopylas antennae.
Gas chromatograph and mass spectrometer parameters for electroantennographic detection experiments
For all test runs, volatiles were separated on the polar column (SGE SolGel Wax). The non-polar column was used to deliver make-up gas at the first effluent split by virtue of the confluent configuration of columns (discussed in Shuttleworth and Johnson, 2020). In all runs, the injector for the non-polar column (in this instance functioning as a non-analytical column, serving to deliver make-up gas) was held at 40°C with a 10:1 split. For thermally desorbed samples (runs with floral volatiles collected from S. parviflorum and R. globosa), the injector for the polar column (the analytical column in these runs) was held at 40°C for 2 min with a 20:1 split (to flush air introduced when opening the ChromatoProbe device to load samples), and then increased to 200°C at 200°C min−1, held for 10 min, and finally increased to 250°C at 200°C min−1 and held for 4 min at 250°C. After the initial 2 min, the split was removed (for the thermal desorption phase) and then a 10:1 split was introduced after 4.2 min for the remainder of the run (to maintain a clean injector port and reduce carryover of volatiles between runs). For septum injections (runs with the synthetic standard blend), a 10:1 split was used for the duration of the run and the injector temperature was raised from a starting point of 40°C to 200°C at 200°C min−1, held for 5 min, and then increased to 250°C at 200°C min−1 and held for 4 min at 250°C. For all runs, the GC oven was held at 40°C for 3 min and then ramped up to 260°C at 20°C min−1 and held at 260°C for 6 min. Helium (99.999% purity) was used as the carrier gas and, due to the confluent configuration of the columns, as the make-up gas which is introduced at the first effluent splitter. The GC was operated in constant flow mode, with the column flow rates set to 8 ml min−1 through the polar (analytical) column and 20 ml min−1 through the non-polar (non-analytical make-up gas column; see Shuttleworth and Johnson, 2020 for discussion of these flow rates). Realized flow rates at the EAD and FID outlets were 12.9 ml min−1 (measured with the column oven at 40°C). The mass spectrometer was used in electron-impact ionization (EI) mode at 70 eV with the detector voltage set by Extended Dynamic Range (EDR) and recording mass fragments between 30 and 350 amu.
Active compounds which were not components of the synthetic standards blend (or were impurities in this blend) were tentatively identified using Varian MS Workstation (version 7.0) and NIST MS Search (version 2.4) with the NIST 2020 mass spectral library. Library identifications were confirmed by comparison of calculated linear (non-isothermal) n-alkane Kováts retention indices (Van den Dool and Kratz, 1963) with published values.
Results
The apparatus described here yielded GC-EAD runs comparable to those that we have obtained on a more conventional GC-FID/EAD system (described in Shuttleworth and Johnson, 2020). Temnora pseudopylas antennae displayed clear depolarizations in response to 18 of the 21 components of our synthetic standard blend, as well as to β-caryophyllene oxide and two unidentified terpenoids which were impurities or contaminants in this blend (Figure 2A). Runs with volatile samples collected from Rothmannia globosa and Satyrium parviflorum flowers yielded 10 and eight active compounds, respectively (Figures 2B,C). Oxygenated aromatics elicited particularly strong depolarizations (sometimes in excess of 1 mV) in all runs, which is similar to results reported for some other hawkmoths (Raguso and Light, 1998; Hoballah et al., 2005; Johnson et al., 2020). Antennal depolarizations were well-aligned with peaks in the FID chromatogram for early eluting compounds, but a slight delay in the antennal responses became apparent as the run progressed reaching a maximum delay of ca. 2.5 s between the FID peak and the antennal response to the last eluting peak in the run (compare responses to indole and benzyl benzoate with responses to early eluting compounds in Figure 2A).
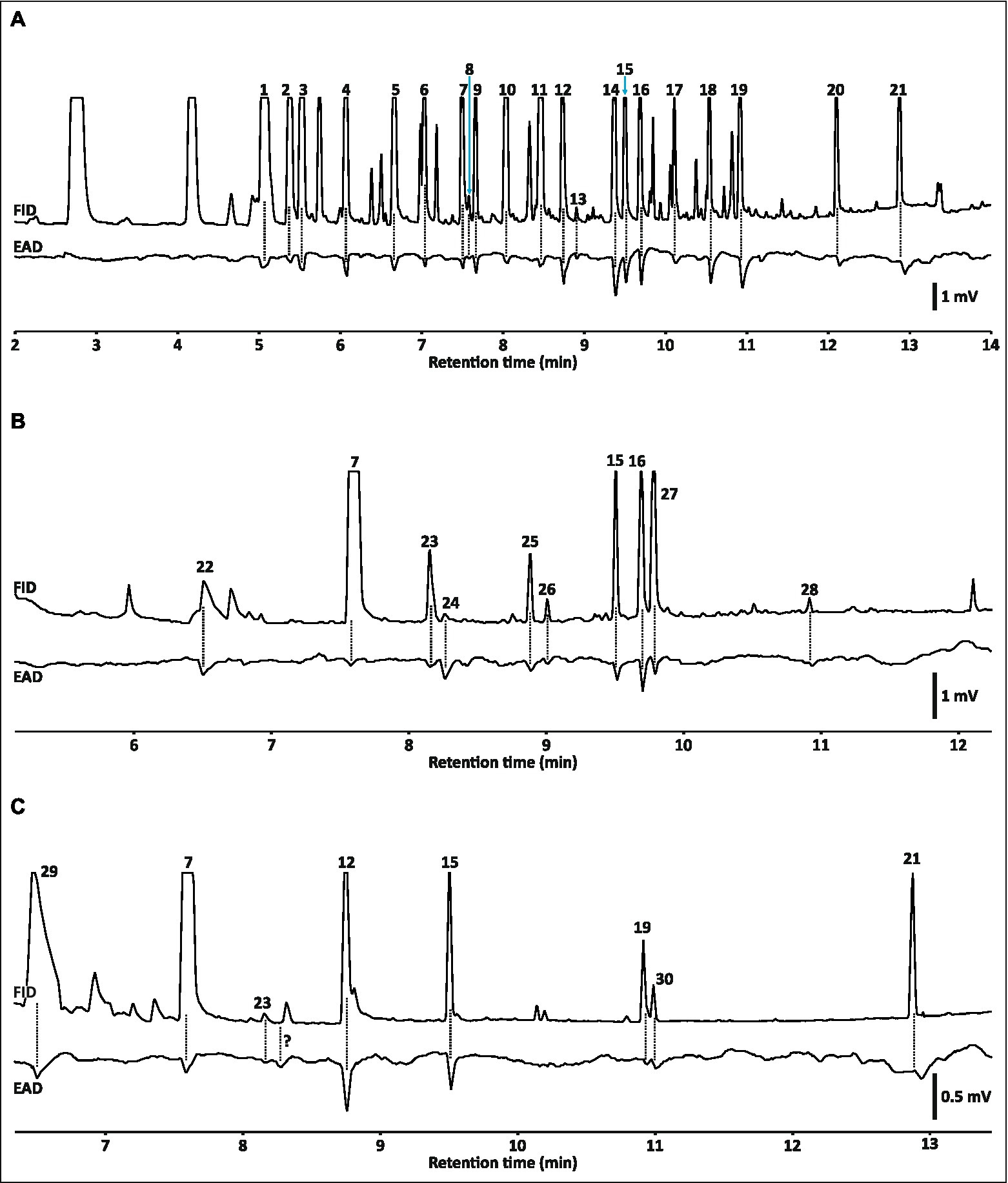
Figure 2. Examples of electroantennographic detection (EAD) runs obtained with Temnora pseudopylas (Sphingidae) antennae and the apparatus described in this study (scale bars refer only to the EAD trace). Refer to Figure 3 for comparison of the FID chromatograms with the total ion chromatograms (TIC) obtained from the MS that were used to identify peaks for active compounds. (A) Antennal responses to components of a blend of synthetic standards. Note that some responses were to impurities or contaminants in the standard blend. Peaks are cropped here as the chromatogram has been amplified along the y-axis in order to show minor peaks (see Figure 3A for the full chromatogram). (B) Antennal responses to volatiles emitted by Rothmannia globosa flowers. (C) Antennal responses to volatiles emitted by Satyrium parviflorum flowers. “?” indicates an apparent antennal depolarization with no corresponding peak. Scale bars refer only to EAD trace. EAD-active compounds: 1, eucalyptol; 2, (Z)-ocimene; 3, (E)-ocimene; 4, (Z)-hex-3-en-1-yl acetate; 5, nonanal; 6, oct-1-en-3-ol; 7, benzaldehyde; 8, unidentified terpenoid; 9, linalool; 10, (E)-β-caryophyllene; 11, α-humulene; 12, benzyl acetate; 13, unidentified terpenoid; 14, geraniol; 15, benzyl alcohol; 16, 2-phenylethyl alcohol; 17, β-caryophyllene oxide; 18, m-cresol; 19, eugenol; 20, indole; 21, benzyl benzoate; 22, unidentified aliphatic alcohol; 23, methyl benzoate; 24, unidentified compound; 25 α-farnesene; 26, methyl salicylate; 27, benzyl nitrile; 28, unidentified terpenoid; 29, anisole; 30, methylisoeugenol. Tentative identifications of active compounds in (B) and (C) that were not common to the blend of synthetic standards shown in (A) were confirmed with comparison of linear n-alkane Kováts retention indices with published values.
Chromatograms obtained simultaneously from the FID and the MS were comparable, although absolute retention times were later on the total ion chromatogram (TIC) from the MS (Figure 3). In runs with the injection of synthetic standards, the retention time for the first eluting peak on the TIC from the MS was 44.6 s later than the corresponding peak on the FID chromatogram. This retention shift decreased to 41.2 s for the last eluting peak in the chromatograms. Although retention times on the MS were not shifted by a constant relative to the FID retention times (i.e., the shift was slightly non-linear), the two chromatograms were sufficiently similar that manual alignment of the chromatograms along the x-axis (to crudely correct for the difference in retention time) allowed peaks in the FID chromatogram to be relatively easily translated to peaks in the TIC, with comparison of Kováts retention indices on each of the FID and MS chromatograms providing additional accuracy, especially for assignment of minor components that may not yield clear peaks on both chromatograms. Because Kováts retention indices for the FID and MS chromatograms are derived from a shared analytical column, they are less affected by slight differences in polarity that can arise between columns in separate instruments (especially noticeable between polar columns of different ages), and are thus more similar than Kováts retention indices derived from injections on separate instruments.
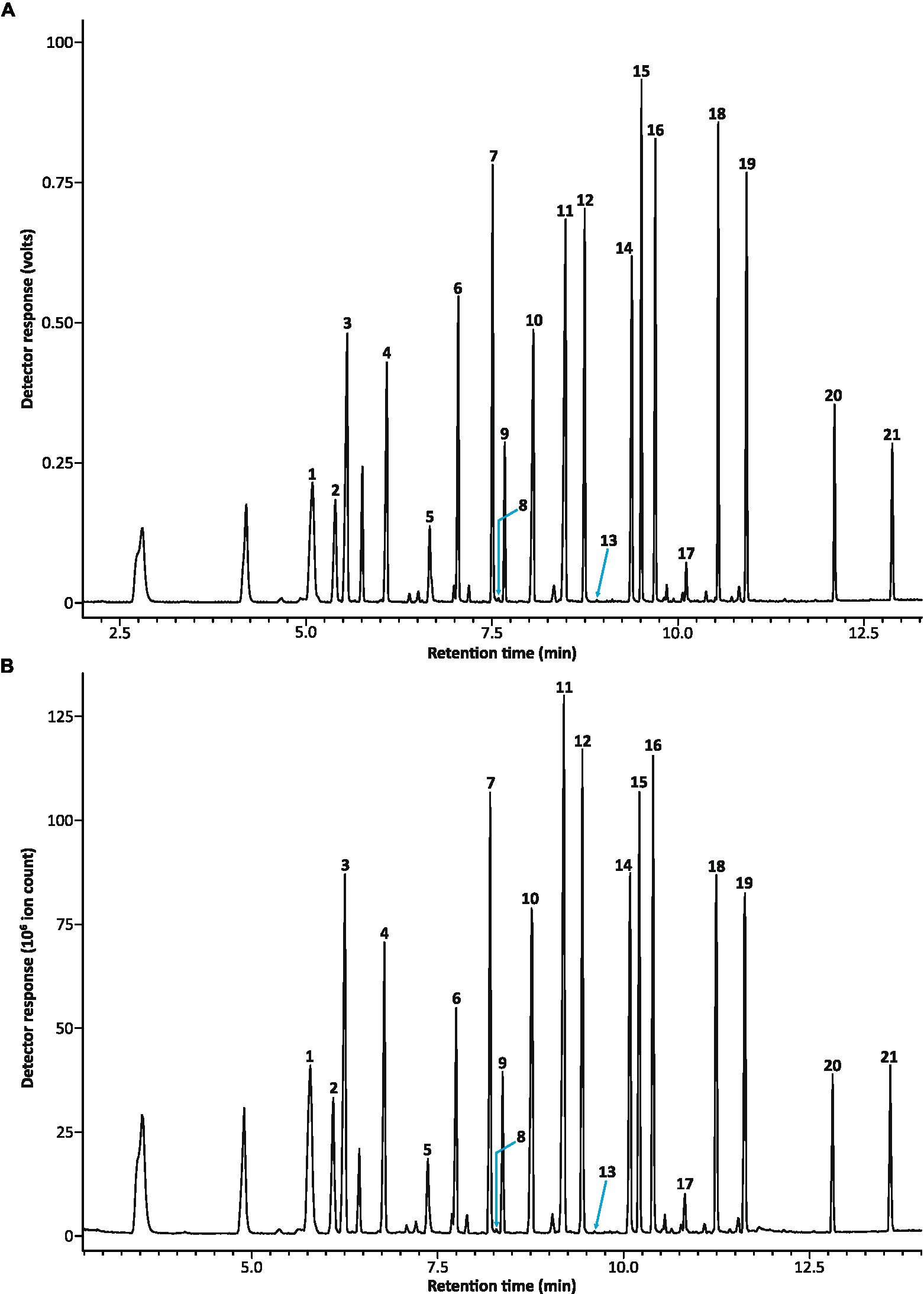
Figure 3. Chromatogram obtained from the flame ionization detector (FID) (A) and the total ion chromatogram (TIC) obtained from the mass spectrometer (MS) (B) for the synthetic standard injection used in the EAD run shown in Figure 2A. Note the difference in absolute retention times between the two chromatograms which have been manually aligned in this figure for ease of reference. Peaks eliciting antennal responses are labeled (numbering as per Figure 2).
Discussion
The GC-MS/FID/EAD apparatus described here offers a simple method for incorporating an MS into a GC-EAD system and resolves some of the challenges resulting from the effect of introducing an outlet under vacuum into the system. Test runs of the system described in this study using Temnora pseudopylas (Sphingidae) antennae and floral volatiles yielded EAD runs that were comparable to results we have obtained on a more conventional GC-FID/EAD system (Figure 2; Johnson et al., 2020; Shuttleworth and Johnson, 2020). By maintaining the FID in the system and removing a portion of effluent for the MS before the split between the FID and the EAD, the effect of the MS on relative elution times no longer affects the assignment of physiologically active peaks, as these are based on the FID signal. A further advantage of this method is that there is no longer a need for an analog output from the MS (analog outputs are not available on many modern MS instruments), since the analog signal for the EAD apparatus is derived from the FID as in any conventional GC-FID/EAD system. Combining two detectors (FID and MS) with varying sensitivities to different types of compounds broadens the range of compound types that can be detected, and this system allows for EAD, FID and MS data to be obtained from a single injection. This is particularly valuable for analysis of thermally desorbed and solid-phase microextraction (SPME) samples where repeat injections of a sample are not possible. The only disadvantage of this method is that effluent is divided between three instead of two detectors, which reduces the amount of effluent reaching the antenna. This is not a concern when using thermally desorbed samples of floral volatiles that usually contain relatively large amounts of volatiles, but may be a consideration for analyses of samples that contain only trace amounts of analyte.
Previously described GC-EAD systems that incorporated an MS have all replaced the FID with an MS (Weissbecker et al., 2004; Schott et al., 2013; Bohman and Peakall, 2014; Li et al., 2021). Balancing the vacuum at the outlet for the MS with the EAD outlet at atmospheric pressure has usually been achieved by reducing the column bore leading to the MS. Other authors have not reported whether they observed a non-linear relationship between the elution times of individual compounds at the MS outlet relative to the EAD outlet, although Li et al. (2021) report using a GC calculator to work out the precise lengths of column after the effluent split that were required to obtain simultaneous elution at the antenna and MS. We found this effect to be extremely challenging to overcome in a previously tested configuration using a single Y-splitter to divide effluent between EAD and an MS, since antennal depolarizations drifted apart from corresponding peaks in the total ion chromatogram (TIC) obtained from the MS as the run progressed. Because the difference between the antennal depolarization and the corresponding peak in the chromatogram changed throughout the run (it was not simply shifted by a constant time), it was very challenging to accurately assign active compounds in complex odor blends with closely eluting peaks. In the GC-MS/FID/EAD system described here, the difference in retention times between the MS and FID chromatograms (the latter would be representative of the timing of EAD responses) decreased by 3.4 s between the first and last eluting peaks (Figure 3). This non-linear shift in retention times is no longer a problem, however, because active peaks are assigned by comparing antennal responses to the FID chromatogram instead of the TIC from the MS.
In comparison to other published systems utilizing an MS with GC-EAD, the non-linear relationship between retention times on the MS versus the FID chromatograms observed in our runs may relate to our use of columns after the split which have a thicker-bore than those utilized by other practitioners (0.25 mm ID on both sides of the split in the case of our previous single Y-splitter configuration, 0.32 ID and 0.15 mm ID leading to the FID/EAD and MS, respectively, in the system described here, as opposed to combinations of 0.1–0.2 mm ID in other systems, Weissbecker et al., 2004; Li et al., 2021). Using thicker-bore columns also requires longer column lengths after the split in order to maintain the vacuum on the MS, which may further exacerbate the problem. Although using shorter lengths of thinner-bore column after the split may ameliorate the problem to a degree, thicker-bore columns are desirable for GC-EAD as they allow higher column flow rates to allow effluent to be flushed from the column more rapidly which is beneficial for the electroantennographic response (Marion-Poll and Thiéry, 1996). By maintaining an FID in the system and removing effluent for the MS before the split between EAD and FID, it is possible to use relatively thick columns (0.32 mm ID on our case) leading to the FID and EAD. The length and bore of the column leading to the MS can be optimized as needed, because the absolute retention times in the TIC from the MS no longer need to be identical to those of antennal depolarizations. We installed a relatively long length (11.8 m) of column between the first splitter and the MS as this has the practical advantage of maintaining the vacuum on the MS when the gas flow is interrupted to replace one of the analytical columns.
Although the configuration described in this study resolves the practical challenges associated with the effects of the MS on the relative retention times, we still experienced a slight accumulating delay in the antennal depolarizations relative to associated peaks in the FID chromatograms (Figure 2A). The delay reached a maximum of ca. 2.5 s for the last eluting peak, but was less prominent for the middle region of the chromatogram and would thus not greatly impact the ability to assign active compounds. The cause of this delay is not clear, but may relate to the effect of a portion of the column leading to the antenna passing through a relatively long transfer line held at a fixed temperature (or possibly a cold spot within the transfer line). In contrast, the column leading to the FID remains inside the GC oven and thus follows the oven temperature program. Shortening the column lengths from the splitter to the EAD and FID outlets or increasing the temperature of the transfer line would possibly reduce the delay. These will be tested in future experiments with this system.
The GC–MS/EAD system described by Li et al. (2021) includes a pneumatic control module that allows precise control over the effluent split, although altering the split ratio affected the column lengths downstream of the splitter that were required to optimize elution at the MS and the antenna. Our system described here would result in a 1:1 effluent split between the FID and the EAD. The proportion of effluent diverted to the MS before this split is difficult to estimate and was optimized empirically by testing different bore columns to both maintain the vacuum on the MS and obtain a split ratio that yielded clear chromatograms from the MS without depleting the effluent remaining for the antenna and FID.
Maintaining an FID in the GC-EAD system, and introducing an MS as well, necessitates a splitter configuration that allows two inputs (assuming make-up gas is introduced at the split) and three outlets. We achieved this by using two splitters in series (Figure 1A). An alternative, though not tested, configuration to achieve the same result could be to use a 5-way effluent splitter instead of two separate splitters in series (Figure 1B). In principle this should function in a similar way to the configuration described here (Figure 1A), as the influence of the vacuum from the MS would be the same for both the FID and EAD outlets. The Valco 4-inlet, 1 outlet manifold (part no: Z4M1) supplied by VICI AG International could possibly be used for this purpose. Adopting a 5-way splitter would also affect split ratios which may need to be optimized by adjusting the diameter of the column leading to the MS. The configuration described here is also contingent on the use of confluent analytical columns as a means to introduce make-up gas (see Shuttleworth and Johnson, 2020 for discussion of this approach). Alternative plumbing (also not tested) for a GC-EAD system using a single analytical column with make-up gas introduced at the split for EAD is illustrated in Figure 1C. This could equally be achieved with the 5-way effluent splitter illustrated in Figure 1B by replacing one of the analytical column inlets with the make-up gas inlet.
There are several practical challenges that result from incorporating an MS into a GC-EAD system, but these were relatively easily overcome. The second transfer-line needed to deliver effluent to the antenna was added through a special request for factory modification by Bruker, but this could equally be achieved with independently sourced components (transfer lines and associated temperature controllers are available from Ockenfels Syntech GmbH) and in-house technicians. Adding an additional transfer-line is, however, contingent on the flow compartment of the GC having enough free space to accommodate a transfer-line passing through it to exit the GC. The addition of the MS on the bench beside the EAD apparatus introduced bench vibration which affected antennal stability. This was countered by installing a bench-top vibration isolator and dividing the (wooden) bench in two so that the bench on which the EAD apparatus is placed is physically separated from the bench with the GC and MS. This successfully resolved the vibration effects. Subsequent experiments with the vibration isolator removed suggest that the physical separation of the benches alone was sufficient to resolve this issue.
Incorporating an MS into a GC-EAD system is a desirable technique but remains remarkably under-utilized given the widespread application of GC-EAD in research examining insect semiochemicals. The configuration we have described here represents a relatively simple method of combining GC-EAD and MS, and solves some of the challenges associated with introducing an outlet under vacuum into a GC-EAD system, as well as eliminating the need to have an analog signal from the MS to the EAD apparatus. We hope that demonstration of the effectiveness of this system, along with discussion of the challenges and solutions to implementing GC-MS/FID/EAD will precipitate greater uptake of this technique.
Data availability statement
The original contributions presented in the study are included in the article, further inquiries can be directed to the corresponding author.
Author contributions
AS conceptualized the study in discussion with SJ. AS collected the data and wrote the manuscript with comments from SJ. All authors contributed to the article and approved the submitted version.
Funding
This study was funded by the National Research Foundation of South Africa (grant numbers 46372, 90691, and 127037).
Acknowledgments
We would like to thank Annemarie Heiduk for discussion of GC-EAD and useful suggestions for optimizing the system described in this study. We also thank Doug Gibbs (Gibbs Technology) for technical support and assistance setting up this system.
Conflict of interest
The authors declare that the research was conducted in the absence of any commercial or financial relationships that could be construed as a potential conflict of interest.
The handling editor declared a past co-authorship with the author SJ.
Publisher’s note
All claims expressed in this article are solely those of the authors and do not necessarily represent those of their affiliated organizations, or those of the publisher, the editors and the reviewers. Any product that may be evaluated in this article, or claim that may be made by its manufacturer, is not guaranteed or endorsed by the publisher.
References
Amirav, A., and Dagan, S. (1997). A direct sample introduction device for mass spectrometry studies and GC-MS analysis. Eur. J. Mass Spectrom. 3, 105–111. doi: 10.1255/ejms.27
Arn, H., Städler, E., and Rauscher, S. (1975). The electroantennographic detector—a selective and sensitive tool in the gas chromatographic analysis of insect pheromones. Z. Naturforsch. C 30, 722–725.
Bohman, B., and Peakall, R. (2014). Pyrazines attract Catocheilus thynnine wasps. Insects 5, 474–487. doi: 10.3390/insects5020474
Cork, A., Beevor, P. S., Gough, A. J. E., and Hall, D. R. (1990). “Gas chromatography linked to electroantennography: a versatile technique for identifying insect semiochemicals” in Chromatography and isolation of insect hormones and pheromones. eds. A. R. McCaffery and I. D. Wilson (New York: Plenum Press), 271–279.
Dötterl, S., Wolfe, L. M., and Jürgens, A. (2005). Qualitative and quantitative analyses of flower scent in Silene latifolia. Phytochemistry 66, 203–213. doi: 10.1016/j.phytochem.2004.12.002
Hoballah, M. E., Stuurman, J., Turlings, T. C. J., Guerin, P. M., Connetable, S., and Kuhlemeier, C. (2005). The composition and timing of flower odour emission by wild Petunia axillaris coincide with the antennal perception and nocturnal activity of the pollinator Manduca sexta. Planta 222, 141–150. doi: 10.1007/s00425-005-1506-8
Jennings, W., Mittlefehldt, E., and Stremple, P. (1997). Analytical gas chromatography. 2nd ed. New York and London: Academic Press.
Johnson, S. D., Balducci, M. G., and Shuttleworth, A. (2020). Hawkmoth pollination of the orchid Habenaria clavata: mechanical wing guides, floral scent and electroantennography. Biol. J. Linn. Soc. 129, 213–226. doi: 10.1093/biolinnean/blz165
Li, C., Cao, J., Wang, X., Xu, P., Wang, X., and Ren, G. (2021). Efficacy of an improved method to screen semiochemicals of insect. PeerJ 9:e11510. doi: 10.7717/peerj.11510
Marion-Poll, F., and Thiéry, D. (1996). Dynamics of EAG responses to host-plant volatiles delivered by a gas chromatograph. Entomol. Exp. Appl. 80, 120–123. doi: 10.1111/j.1570-7458.1996.tb00901.x
Moorhouse, J. E., Yeadon, R., Beevor, P. S., and Nesbitt, B. F. (1969). Method for use in studies of insect chemical communication. Nature 223, 1174–1175. doi: 10.1038/2231174a0
Raguso, R. A., and Light, D. M. (1998). Electroantennogram responses of male Sphinx perelegans hawkmoths to floral and ‘green-leaf volatiles’. Entomol. Exp. Appl. 86, 287–293. doi: 10.1046/j.1570-7458.1998.00291.x
Schiestl, F. P., and Marion-Poll, F. (2002). “Detection of physiologically active flower volatiles using gas chromatography coupled with electroantennography” in Analysis of taste and aroma. eds. J. F. Jackson and H. F. Linskens (Berlin, Heidelberg, New York, Barcelona, Hong Kong, London, Milan, Paris, Tokyo: Springer), 173–198.
Schneider, D. (1957). Elektrophysiologische untersuchungen von chemo-und mechanorezeptoren der antenne des seidenspinners Bombyx mori L. Zeit. Vergl. Physiol. 40, 8–41. doi: 10.1007/BF00298148
Schott, M., Wehrenfennig, C., Gasch, T., Düring, R. A., and Vilcinskas, A. (2013). A portable gas chromatograph with simultaneous detection by mass spectrometry and electroantennography for the highly sensitive in situ measurement of volatiles. Anal. Bioanal. Chem. 405, 7457–7467. doi: 10.1007/s00216-013-7196-3
Shuttleworth, A., and Johnson, S. D. (2009). The importance of scent and nectar filters in a specialized wasp-pollination system. Funct. Ecol. 23, 931–940. doi: 10.1111/j.1365-2435.2009.01573.x
Shuttleworth, A., and Johnson, S. D. (2020). Using two confluent capillary columns for improved gas chromatography-electroantennographic detection (GC-EAD). Entomol. Exp. Appl. 168, 191–197. doi: 10.1111/eea.12873
Struble, D. L., and Arn, H. (1984). “Combined gas chromatography and electroantennogram recording of insect olfactory responses” in Techniques in pheromone research. eds. H. E. Hummel and T. A. Miller (New York: Springer-Verlag), 161–178.
Van den Dool, H., and Kratz, P. D. (1963). A generalization of the retention index system including linear temperature programmed gas-liquid partition chromatography. J. Chromatogr. 11, 463–471. doi: 10.1016/S0021-9673(01)80947-X
Keywords: Electroantennography, floral volatile, GC-MS-EAD, GC-MS/EAD, GC-FID-EAD, GC-FID/EAD, Sphingidae
Citation: Shuttleworth A and Johnson SD (2022) GC-MS/FID/EAD: A method for combining mass spectrometry with gas chromatography-electroantennographic detection. Front. Ecol. Evol. 10:1042732. doi: 10.3389/fevo.2022.1042732
Edited by:
Li Chen, Hebei University, ChinaReviewed by:
Gerhard Johannes Gries, Simon Fraser University, CanadaZsolt Kárpáti, Julius Maximilian University of Würzburg, Germany
Copyright © 2022 Shuttleworth and Johnson. This is an open-access article distributed under the terms of the Creative Commons Attribution License (CC BY). The use, distribution or reproduction in other forums is permitted, provided the original author(s) and the copyright owner(s) are credited and that the original publication in this journal is cited, in accordance with accepted academic practice. No use, distribution or reproduction is permitted which does not comply with these terms.
*Correspondence: Adam Shuttleworth, c2h1dHRsZXdvcnRoQHVrem4uYWMuemE=