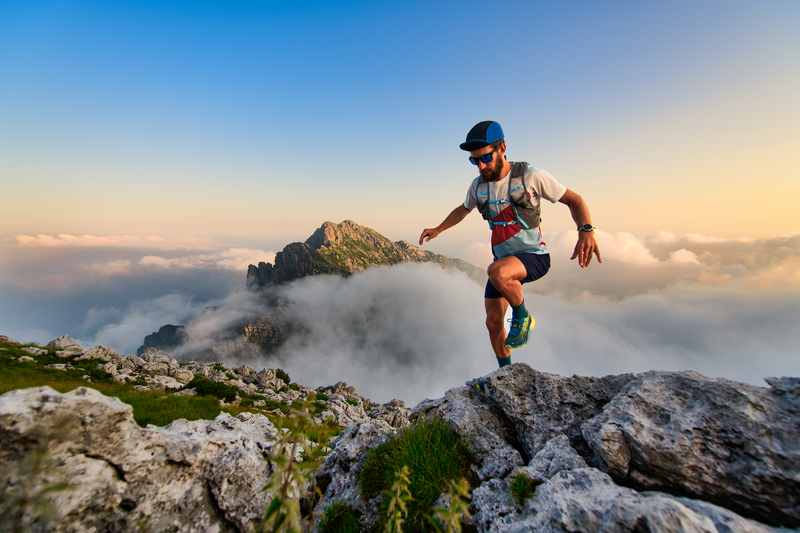
95% of researchers rate our articles as excellent or good
Learn more about the work of our research integrity team to safeguard the quality of each article we publish.
Find out more
ORIGINAL RESEARCH article
Front. Ecol. Evol. , 21 November 2022
Sec. Biogeography and Macroecology
Volume 10 - 2022 | https://doi.org/10.3389/fevo.2022.1032083
This article is part of the Research Topic Modeling Large-Scale Ecological and Evolutionary Dynamics View all 5 articles
Environmental and intrinsic factors interact to determine energy requirements in vertebrates. Glucocorticoid hormones (GCs) are key mediators of this interaction, as they fluctuate with energetic demands and regulate physiological and behavioral responses to environmental challenges. While a great body of research has focused on GC variation among individuals, the mechanisms driving GC variation across species and at broad spatial scales remain largely unexplored. Here, we adopted a macrophysiological approach to investigate the environmental factors and life-history traits driving variation in baseline GCs across lizard species. We tested three hypotheses: (1) If GCs increase with body temperature to meet higher metabolic demand, we expect an association between average baseline GCs and the mean species’ body temperature in the field (GC-temperature dependence hypothesis); (2) If GCs mediate behavioral responses to avoid thermal extremes, we expect that individuals frequently exposed to extreme conditions exhibit higher baseline GC levels (Behavioral thermoregulation hypothesis); (3) If GCs increase to support higher energy demands in active foragers during their period of activity, we expect that active foraging species have higher baseline GCs than sit-and-wait foragers, and that GC levels increase in relation to the duration of daily activity windows (Activity hypothesis). We used biophysical models to calculate operative temperatures and the activity patterns of lizards in sun-exposed and shaded microenvironments. Then, we tested the association between baseline GCs, body temperature, operative temperatures, foraging mode, and activity windows across 37 lizard species, using data from HormoneBase. Our comparative analyses showed that variation in baseline GCs was primarily related to the mean field body temperature and foraging mode, with higher baseline GCs in active foragers with higher body temperatures. Our results suggest that body temperature and foraging mode drive GC variation through their effects on energy requirements across lizard species.
Understanding the responses of organisms to environmental variation is crucial in the current context of global change. In order to make predictions on how organisms will respond and adapt to everchanging environments, we need to gain insights into the mechanisms driving organism-environment interactions. In vertebrates, environmentally-driven responses are primarily mediated by glucocorticoids (GCs, i.e., corticosterone and cortisol). GCs are metabolic hormones that orchestrate physiological and behavioral responses to internal and external factors, helping organisms to overcome challenges and maintain homeostasis. Therefore, they have a pivotal role linking environmental changes and vertebrate coping mechanisms (Sapolsky et al., 2000; Koolhaas et al., 2001; Landys et al., 2006; Romero et al., 2009). GC levels fluctuate in daily and seasonal patterns, and show acute increases in response to unpredicted perturbations or environmental changes. Through the synthesis and release of GCs, organisms mobilize body reserves (i.e., glucose, fatty acids, and proteins; Sapolsky et al., 2000; Remage-Healey and Romero, 2001; Romero and Beattie, 2022) to provide the resources needed to cope with a current or anticipated increase in energy expenditure (McEwen and Wingfield, 2003; Landys et al., 2006; Romero et al., 2009; Herman et al., 2016). Thus, GCs are implicated in the physiological regulation of organismal tolerances to variation in environmental conditions challenging homeostasis, including, for instance, unpredictable and anticipated weather fluctuations (Wingfield et al., 1988; Wingfield, 2005, 2013; de Bruijn and Romero, 2013; Romero and Wingfield, 2015; Dupoué et al., 2018; Crino et al., 2020). Although different studies have described associations between GC levels and environmental variables, especially temperature, these relationships are not ubiquitous as they often differ among taxa or between sexes (Lendvai et al., 2009; Telemeco and Addis, 2014; Jessop et al., 2016; Jimeno et al., 2017; Dupoué et al., 2018; Hudson et al., 2020), and their mechanistic underpinnings remain poorly understood. Additionally, existing literature testing for the associations between ambient temperature and GC concentrations shows a strong bias toward studies in captivity and endothermic species, indicating a need for field studies, especially in ectotherms such as reptiles and amphibians, which remain underrepresented (reviewed in de Bruijn and Romero, 2018).
Ectotherms depend on external heat sources to control their body temperature, and thus show a tight association among physiology, behavior, and ambient temperature. However, the link between ambient temperature and GC levels is complex as it involves both direct and indirect effects of temperature on energy requirements, behavior, and activity patterns (e.g., Romero, 2002; Telemeco and Addis, 2014; Jessop et al., 2016 Dupoué et al., 2018; Megía-Palma et al., 2022). Body temperature affects multiple physiological processes such as metabolic rates, digestion, locomotion, or reproduction (Hamilton, 1973; Angilletta, 2001; Gillooly et al., 2001), and thus weather conditions might affect energy requirements and GC levels directly (Racic et al., 2020). Furthermore, temporal variation and spatial variation in ambient temperature affect both the patterns of activity and the use of different microenvironments for behavioral thermoregulation, which may indirectly determine behavioral patterns, energy requirements, and GC levels (Dupoué et al., 2018; Megía-Palma et al., 2022). For instance, frequent exposure to temperature extremes requires the activation of physiological and behavioral responses to maintain homeostasis or avoid thermal stress (Telemeco and Addis, 2014; Jessop et al., 2016). GCs may be implicated in this activation, as they release required nutrients to activate behavioral responses such as selection of microhabitat to avoid overheating or cooling risk (Jessop et al., 2016). In addition to short-term responses to environmental changes, energy requirements and GC levels might differ among species with different life-history traits, reproductive modes, or foraging strategies (e.g., active predators vs. sit-and-wait foragers; Huey and Pianka, 1981; Vitt et al., 2003). These among-species differences in energy expenditure must be sustained by either increased energy uptake rates or a differential allocation into activity or self-maintenance likely determining life-history evolution (Shine, 2005). To understand the extent to which GCs vary in response to either short-term variation in environmental conditions or interspecific strategies, GC levels need to be investigated across species on a broad spatial scale through macrophysiology (Chown et al., 2004).
The broad-scale analyses of physiological traits in relation to environmental variables entail different challenges, such as the need to take into consideration the heterogeneity of microenvironments at the individual scale, e.g., sun-exposed and shaded conditions used for behavioral thermoregulation (e.g., Kearney et al., 2009). To incorporate the microclimatic heterogeneity into broad-scale analysis of physiological traits, microclimate and biophysical models arise as a powerful tool since they predict how key physiological traits such as body temperature or metabolic rate respond to environmental conditions at the scale of individuals (Kearney et al., 2020; Anderson et al., 2022; Rubalcaba and Jimeno, 2022). Considering the effects of microclimates on heat exchange of individuals greatly improves the model’s capacity to unravel the effects of temperature on physiological parameters such as body temperature and GC levels (Rubalcaba and Jimeno, 2022).
Here we adopt a macrophysiological approach to investigate the role of ambient temperature and life-history traits driving variation in baseline GC concentrations across lizard species. We tested the following, non-mutually exclusive hypotheses:
1. GC-temperature dependence hypothesis. Body temperature determines metabolic rates and GC levels play a fundamental role in mobilizing reserves required to fuel metabolism (Jessop et al. 2016; Francis et al., 2018). Therefore, we expect that species experiencing higher body temperatures in the field exhibit higher mean baseline GC concentrations.
2. Behavioral thermoregulation hypothesis. GCs regulate physiology and behavior to maintain homeostasis and avoid thermal extremes (Telemeco and Addis, 2014; Jessop et al., 2016; Dupoué et al., 2018). Therefore, when individuals experience extreme thermal conditions in their available microhabitats, they might require to behaviorally control body temperature, which will increase energy requirements. Here, we expect that individuals experiencing extreme conditions will exhibit higher baseline GC levels.
3. Activity hypothesis. Maintaining activities such as foraging or predator avoidance requires increasing metabolic rate with a commensurate elevation in baseline GCs (Hamann et al., 2007; Jessop et al., 2015). Therefore, species that forage actively and invest a greater proportion of their activity period seeking for food might require higher baseline GC concentrations to support their greater energy requirements. Foraging modes of lizards have traditionally been characterized as either active or sit-and-wait foraging and, although simplistic, this bimodal approach allows analyzing relationships between foraging strategies with environmental or physiological variables (Cooper, 2005). Here, we expect that GC concentrations will be higher in active than in sit-and-wait foragers and that they increase in relation to the duration of activity window since active foraging in reptiles is restricted to the period in which individuals can maintain body temperature within their operational temperature range (Nelson and Gregory, 2000; Kerr et al., 2008).
By testing the role of body temperature and life-history traits related to energy expenditure in GC levels across lizard species, we aim at improving our mechanistic understanding of GC variation in ectotherms across broad spatial scales.
We collected baseline GC concentrations from HormoneBase (Vitousek et al., 2018), a published database of circulating steroid hormone levels from free-living vertebrate populations. HormoneBase contains mean baseline GC concentrations of male and female adults including information on the time and location of hormone collection, as well as the method and latency of sampling. We then combined these GC data with species-level information from the lizard species database by Meiri (2018). First, to test the GC-temperature dependence hypothesis (species experiencing higher field body temperatures display higher baseline GC levels due to their higher energy requirements), we used mean field body temperature data at the species level. Second, to test the Activity hypothesis (species with higher energy requirements for foraging display higher GC levels), we used foraging mode categorized as either active or sit-and-wait foragers. Finally, we used mean species body mass estimated using family-specific allometric relationships in relation to body length following Meiri (2018). Our final database included 223 GC records from 37 lizard species to investigate the effect of body temperature on GC levels, and 143 records across 23 species for the effect of activity level and foraging mode.
To investigate the Behavioral thermoregulation hypothesis (GC levels increase to trigger thermoregulatory responses when individuals experience extreme temperatures), we modeled operative temperatures (i.e., the equilibrium temperature that individuals experience in their microenvironments; Bakken, 1992). Operative temperatures (Te) provide a measure of the thermal conditions available in the environment considering both properties of microenvironments (such as temperature, solar radiation, and wind speed) and the organism (size, shape, or skin absorbance to solar radiation, e.g., Clusella-Trullas et al., 2021). Here, we used Te to characterize the thermal extremes (i.e., sun-exposed vs shaded conditions) and thereby the thermal constraints that individuals experienced in the environment at the time and location at which GC samples were collected. We modeled Te combining models of heat exchange via absorption of short-wave solar radiation, thermal radiation, convection, and conduction with the ground (Porter et al., 1973; Rubalcaba and Olalla-Tárraga, 2020). These heat transfer mechanisms depend on total incoming solar radiation, S (Wm−2), air temperature, Ta (°C), soil temperature, Tg, and sky radiant temperature, Tsky (°C), as well as the dorsal, Ad (m2), and ventral, Ag, skin surface areas, and the skin absorbance to short-wave radiation, a:
Here, Ra and Rg are radiative transfer coefficients representing the exchange of heat via thermal radiation with the air and the ground, respectively, and were estimated as and where ε and σ are, respectively, the lizard’s emissivity of infrared radiation (set here at 0.95; Campbell and Norman, 1998) and the Stefan–Boltzmann constant (5.67 × 10−8 Wm−2 K−4). The convection, hc, and conduction, hg, transfer coefficients (Wm−2°C−1), were used to model heat exchange via convection and conduction to the ground surface. We calculated the convection coefficient using the Reynolds-Nusselt numbers (Re-Nu) expression , which is a function of wind velocity (v, ms−1), kinematic viscosity of the air (υ, m2s−1), and the characteristic length (L, m) over which the fluid flows in contact with the animal’s surface, estimated here as L = 3.3 × (M × 10−6)1/3 (Mitchell, 1976). Finally, hg was estimated following Stevenson (1985) as the ratio between the thermal conductivity (set here to 0.027 Wm−1°C−1) and skin thickness .
We modeled microclimatic conditions (i.e., solar radiation, wind velocity, air, soil, and sky temperature) using the function micro_ncep in NicheMapR (Kearney et al., 2020). This function uses a microclimate model that integrates the RNCEP 6-hourly weather and the downscaling functions from the microclima package (Maclean et al., 2019) to compute hourly estimations of historical weather data in either sun-exposed or shaded conditions. Here, we computed hourly estimations of the microclimatic variables in the sun and in the shade (90% excluded solar radiation) for a period of 1 month preceding the time at which GC samples were collected and specifying the location of each population in HormoneBase. We then computed operative temperatures using the equation 1 and estimated: (1) the maximum and minimum operative temperatures, respective as the 95% (Te upp) and 5% (Te low) percentiles; and (2) the activity window (i.e., the number of hours that the animal is predicted to be active) by counting the number of hours in which the species’ mean field body temperature was within the operative temperatures in the shade and in the sun (Te, shade ≤ Tb ≤ Te, sun).
Based upon the Behavioral thermoregulation hypothesis, we expect that individuals increase their baseline GC concentrations when operative temperatures are either high or low when compared with the thermal preference of the species. To characterize this species’ thermal preference, we used the mean species’ field body temperature (see Data collection). Thus, we expect that baseline GCs are negatively related to the difference (Tb − Te upper) and positively to the difference (Tb − Te lower), indicating that GC levels increase when individuals are frequently exposed to operative temperatures that are either higher or lower than the average field body temperature of each species.
We used phylogenetically controlled mixed-effect models to investigate the relationship between GC concentration and field body temperature (GC-temperature dependence hypothesis), the extremes of the operative temperature range (Behavioral thermoregulation hypothesis), and the activity window and foraging mode (Activity hypothesis). The models included the species identity and the laboratory that performed GC analyses as random intercepts to control for the non-independence of intraspecific hormone concentrations and the non-independence of samples from the same laboratory (Francis et al., 2018). Models including random intercepts were better supported than those including both random intercepts and slopes based on their lower DIC values.
To test the GC-temperature dependence hypothesis, we included log-transformed GC concentrations as a response variable, and the species’ mean field body temperature, sex, and log-body mass as fixed factors. In a different model, we tested the Activity-foraging mode hypothesis including activity window, foraging mode, and the interaction between them (in addition to body mass and sex) as fixed factors. Finally, to test the Behavioral thermoregulation hypothesis, we analyzed the relationship between log-GCs and the differences (Tb − Te upper) and (Tb − Te lower) including log-body mass and sex as additional fixed factors. Phylogenetic models were fitted using the R package MCMCglmm (Hadfield, 2010) in R v4.2.0 (R Core Team, 2022) and using the phylogeny by Johnson et al. (2018) available for HormoneBase.
Baseline GC concentrations were positively and significantly related to the species’ mean field body temperature (Figure 1A). The model also included a significant effect of sex (Table 1A), with males displaying significantly higher GC levels than females (22.19 ± 0.13 ng mL−1 (mean ± SE) in males and 18.57 ± 0.17 ng mL−1 in females). We did not find a significant interaction between activity window and foraging mode, or a significant effect of activity window on GCs (Table 1B). However, active foragers displayed higher GC levels than sit-and-wait foragers (56.27 ± 4.94 ng mL−1 in active foragers and 19.48 ± 0.20 ng mL−1 in sit-and-wait foragers; Figure 1B). Finally, the model testing the effect of deviations between field body temperature and operative temperatures on baseline GCs showed no significant effects of either exposure to high operative temperature (Tb − Te upper; Figure 2A), or exposure to cold temperature (Tb − Te lower; Figure 2B; Table 1C).
Figure 1. Baseline glucocorticoid (GC) concentrations in relation to field body temperature (A) and foraging mode (B) across lizard species. Boxes and whiskers in (A) represent GC levels for each species where horizontal lines within boxes are median GC concentration, boxes capture the interquartile range, and whiskers represent scores outside the lower an upper quartile. The smooth line was generated using loess curve with span parameter of 2.
Table 1. Results of the three phylogenetic linear mixed-effect models testing the effect of body temperature (A), foraging mode and activity (B), and experienced temperature extremes (C) of glucocorticoid concentrations across lizard species.
Figure 2. Baseline glucocorticoid (GC) concentrations in relation to the difference between field body temperature (Tb) and upper range of operative temperatures (Te upper; 95% percentile, A), and difference between field body temperature and lower range of operative temperatures (Te lower; 5%, B). Boxes represent variation in GCs and operative temperatures for each species, where horizontal lines within boxes are median GC concentrations, box width captures the interquartile range in operative temperature, box height represents the interquartile range in GC concentrations, and whiskers show the range outside the lower an upper quartile. Smooth lines (dashed denotes nonsignificant relationships) are loess curves with span parameter of 2.
Body temperature and foraging mode were the two most important factors determining variation in baseline GC concentrations across lizard species. The phylogenetic analyses showed that GC levels were higher in species displaying elevated mean body temperatures (Figure 1A). Body temperature fundamentally affects the kinetic energy of cellular components, increasing reaction rates of biochemical processes comprising metabolism (Hamilton, 1973; Gillooly et al., 2001). Because GCs play a fundamental role in mobilizing reserves required to fuel metabolic processes (Sapolsky et al., 2000; Remage-Healey and Romero, 2001; Landys et al., 2006; Jimeno et al., 2018; Romero and Beattie, 2022), GC levels are predicted to increase in relation to body temperature (Francis et al., 2018). Supporting this notion, a previous interspecific analysis found that baseline GCs are related to ambient temperature across reptile species (Jessop et al., 2016); however, the mechanistic underpinnings of this relationship remain elusive. Ambient temperature is related to a variety of ecological factors and life-history traits that may affect GC levels (Eikenaar et al., 2012; Meiri et al., 2013). For example, ambient temperature is a good predictor of variation in life-history traits such as clutch frequency, longevity, or age at first reproduction (Meiri et al., 2013). In contrast, body temperatures are unrelated to environmental temperatures across lizard species (Meiri et al., 2013). Therefore, interspecific relationships between baseline GCs and ambient temperature in lizards might be primarily driven by variation in life-history traits rather than changes in body temperature and metabolism. However, the positive effect of mean species’ body temperature on baseline GCs described here across lizard species supports the hypothesis that interspecific GC variation is influenced by the temperature dependence of metabolism (GC-temperature dependence hypothesis), providing insights into the mechanisms underpinnings of broad-scale variation in GCs.
Previous studies have found positive relationships between GCs and field body temperature or ambient temperature at the intraspecific level (e.g., Dunlap and Wingfield, 1995; Tyrrell and Cree, 1998; Jessop et al., 2000; Telemeco and Addis, 2014; Jessop et al., 2016; Megía-Palma et al., 2020, but see Racic et al., 2020). This temperature dependence of GCs might be driven by multiple factors including direct influence of temperature on metabolism, indirect effects via GC increases in response to suboptimal temperatures that trigger behavioral avoidance (Preest and Cree, 2008), or temporal correlations between daily patterns of GC and ambient temperature fluctuations (Romero, 2002; Eikenaar et al., 2012). Here, we found that species experiencing higher mean field body temperatures displayed higher baseline GC levels. However, baseline GCs did not increase when operative temperatures (at the time of hormone sampling) exceeded the average body temperature of the species (i.e., when the difference Tb − Te upper was more negative). Therefore, our results suggest that species that frequently experience higher body temperature have higher energy requirements and hence higher baseline GCs, and we did not find support for the hypothesis that higher thermal constraints trigger thermoregulatory behavior to avoid overheating in exposed conditions. Although baseline GCs were not related to thermal constraints, GC levels could have showed acute increases in response to sudden fluctuations in body temperature (Jessop et al., 2016), but gone back to baseline concentrations soon after the exposure to these suboptimal temperatures. If this is so, baseline GC levels would not be appropriate to detect short-term exposure to extreme operative temperatures, as they reflect GC secretion at one time-point. In addition, GC responses to thermal extremes are complex in lizards as they involve other environmental factors that we did not consider here such as food (Cote et al., 2010) or water availability (Dupoué et al., 2018). Therefore, we cannot discard that GC levels fluctuated more, nor that the accumulated exposure to GCs was higher, in populations experiencing thermal extremes (Behavioral thermoregulation hypothesis). More integrative GC measurements in feces or keratinized tissues, which capture GC secretion during longer time periods (i.e., hours to weeks), may be useful to detect these effects.
Lizard species displaying active foraging had higher average baseline GC levels than sit-and-wait foragers. Active foragers invest a significant amount of energy in locomotion resulting in a two- to ten-fold increase in energy requirements when compared to sit-and-wait foragers (Taylor, 1973; Anderson and Karasov, 1981). Therefore, higher baseline GCs in active than in sit-and-wait foragers suggest that active species require higher GC concentrations to sustain higher daily energy demands. Although foraging activity influences energy requirements and GC levels, we did not find a relationship between GCs and the activity window in either active or sit-and-wait foragers. The relationship between energy requirements, GC concentrations, and activity patterns in ectotherms is complex and might lead to context-specific covariation between GC levels and activity windows. Thus, GC levels might be higher among species experiencing longer activity windows as individuals need to maintain elevated metabolic rates during longer periods. However, the opposite pattern (i.e., shorter activity windows increase GC levels) might also be expected if reducing the activity window leads to an increase in intraspecific competition for breeding resources and mates (Eikenaar et al., 2012). As a result, the activity window does not seem to be a strong predictor of energy requirements and GC variation across lizard species, unless perhaps combined with other factors influencing conspecific competition such as population density or resource availability. Our analysis combining activity window and foraging mode was nonetheless restricted to a relatively small number of active species in relation to those exhibiting the sit-and-wait foraging strategy that might impede detecting a significant interaction between foraging mode and activity window. In addition, the use of discrete foraging modes has been criticized since the variables that ultimately reflect energy requirements (e.g., movements per minute, or percent time moving) vary continuously (Cooper, 2005). Our approach thus uses a surrogate of activity and hence further empirical support is required to confirm the Activity-foraging mode hypothesis.
Lizard species experiencing higher body temperatures and active foraging exhibited higher baseline GC levels, whereas the effect of ambient temperature on baseline GCs was not significant when analyzed across species. These results suggest that among-species differences in mean body temperature determine interspecific variation in baseline GC levels, probably as a result of the temperature dependence of metabolism. This points at species that consistently display higher energy demands (either due to their higher experienced body temperatures or active foraging mode) having to sustain these requirements either via increasing their energy uptake rates, or else prioritizing energy allocation into activity or self-maintenance, potentially at the expense of reducing investment in other life-history traits.
Our work adds up to the increasing body of research emphasizing the need to account for variation in energy demands and metabolic processes toward an accurate ecological interpretation of GCs, in light of their metabolic function. More specifically, we demonstrate the role of energy metabolism and life-history traits related to energy expenditure in determining GC variation across ectotherm species, which illustrates the capacity of biophysical models to reveal patterns that are difficult to assess in the field. These models thus emerge as powerful tools to gain insights and elaborate predictions on physiological processes taking place at broad spatial scales, and on their expected variation in response to changes in environmental conditions.
The raw data and code supporting the conclusions of this article are available in the GitHub repository: github.com/JRubalcaba/CORT_vs_Tb.
JR and BJ conceived the idea, designed research, and wrote the paper. JR performed the microclimate and biophysical simulations and analyzed the data. All authors contributed to the article and approved the submitted version.
This project has received funding from the European Union’s Horizon 2020 research and innovation program under the Marie Skłodowska-Curie grant agreement no. 843094 to JR. BJ was funded by grant FJC2019-039748-I of MCIN/AEI/10.130 39/501100011033.
The authors declare that the research was conducted in the absence of any commercial or financial relationships that could be construed as a potential conflict of interest.
All claims expressed in this article are solely those of the authors and do not necessarily represent those of their affiliated organizations, or those of the publisher, the editors and the reviewers. Any product that may be evaluated in this article, or claim that may be made by its manufacturer, is not guaranteed or endorsed by the publisher.
Anderson, R. A., and Karasov, W. H. (1981). Contrasts in energy intake and expenditure in sit-and-wait and widely foraging lizards. Oecologia 49, 67–72. doi: 10.1007/BF00376899
Anderson, R. O., White, C. R., Chapple, D. G., and Kearney, M. R. (2022). A hierarchical approach to understanding physiological associations with climate. Glob. Ecol. Biogeogr. 31, 332–346. doi: 10.1111/geb.13431
Angilletta, M. J. Jr. (2001). Thermal and physiological constraints on energy assimilation in a widespread lizard (Sceloporus undulatus). Ecology 82, 3044–3056. doi: 10.1890/0012-9658(2001)082[3044:TAPCOE]2.0.CO;2
Bakken, G. S. (1992). Measurement and application of operative and standard operative temperatures in ecology. Am. Zool. 32, 194–216. doi: 10.1093/icb/32.2.194
Campbell, G. S., and Norman, J. M. (1998). An introduction to environmental biophysics. Springer Verlag, New York.
Chown, S. L., Gaston, K. J., and Robinson, D. (2004). Macrophysiology: large-scale patterns in physiological traits and their ecological implications. Funct. Ecol. 18, 159–167. doi: 10.1111/j.0269-8463.2004.00825.x
Clusella-Trullas, S., Garcia, R. A., Terblanche, J. S., and Hoffmann, A. A. (2021). How useful are thermal vulnerability indices? Trends Ecol. Evol. 36, 1000–1010. doi: 10.1016/j.tree.2021.07.001
Cooper, W. E. (2005). The foraging mode controversy: both continuous variation and clustering of foraging movements occur. J. Zool. 267, 179–190. doi: 10.1017/S0952836905007375
Cote, J., Clobert, J., Poloni, L. M., Haussy, C., and Meylan, S. (2010). Food deprivation modifies corticosterone-dependent behavioural shifts in the common lizard. Gen. Comp. Endocrinol. 166, 142–151. doi: 10.1016/j.ygcen.2009.11.008
Crino, O. L., Driscoll, S. C., Brandl, H. B., Buchanan, K. L., and Griffith, S. C. (2020). Under the weather: corticosterone levels in wild nestlings are associated with ambient temperature and wind. Gen. Comp. Endocrinol. 285:113247. doi: 10.1016/j.ygcen.2019.113247
de Bruijn, R., and Romero, L. M. (2013). Artificial rain and cold wind act as stressors to captive molting and non-molting European starlings (Sturnus vulgaris). Comp. Biochem. Physiol. A Mol. Integr. Physiol. 164, 512–519. doi: 10.1016/j.cbpa.2012.12.017
de Bruijn, R., and Romero, L. M. (2018). The role of glucocorticoids in the vertebrate response to weather. Gen. Comp. Endocrinol. 269, 11–32. doi: 10.1016/j.ygcen.2018.07.007
Dunlap, K. D., and Wingfield, J. C. (1995). External and internal influences on indices of physiological stress. I. Seasonal and population variation in adrenocortical secretion of free-living lizards, Sceloporus occidentalis. J. Exp. Zool. 271, 36–46. doi: 10.1002/jez.1402710105
Dupoué, A., Rutschmann, A., Le Galliard, J. F., Clobert, J., Blaimont, P., Sinervo, B., et al. (2018). Reduction in baseline corticosterone secretion correlates with climate warming and drying across wild lizard populations. J. Anim. Ecol. 87, 1331–1341. doi: 10.1111/1365-2656.12843
Eikenaar, C., Husak, J., Escallon, C., and Moore, I. T. (2012). Variation in testosterone and corticosterone in amphibians and reptiles: relationships with latitude, elevation, and breeding season length. Am. Nat. 180, 642–654. doi: 10.1086/667891
Francis, C. D., Donald, J. W., Fuxjager, M. J., Goymann, W., Hau, M., Husak, J. F., et al. (2018). Metabolic scaling of stress hormones in vertebrates. Integr. Comp. Biol. 58, 729–738. doi: 10.1093/icb/icy063
Gillooly, J. F., Brown, J. H., West, G. B., Savage, V. M., and Charnov, E. L. (2001). Effects of size and temperature on metabolic rate. Science 293, 2248–2251. doi: 10.1126/science.1061967
Hadfield, J. D. (2010). MCMC methods for multi-response generalized linear mixed models: the MCMCglmm R package. J. Stat. Softw. 33, 1–22. doi: 10.18637/jss.v033.i02
Hamann, M., Jessop, T. S., and Schauble, C. S. (2007). Fuel use and corticosterone dynamics in hatchling green sea turtles (Chelonia mydas) during natal dispersal. J. Exp. Mar. Biol. Ecol. 353, 13–21. doi: 10.1016/j.jembe.2007.08.017
Herman, J. P., McKlveen, J. M., Ghosal, S., Kopp, B., Wulsin, A., Makinson, R., et al. (2016). Regulation of the hypothalamic-pituitary-adrenocortical stress response. Compr. Physiol. 6, 603–621. doi: 10.1002/cphy.c150015
Hudson, S. B., Lidgard, A. D., and French, S. S. (2020). Glucocorticoids, energy metabolites, and immunity vary across allostatic states for plateau side-blotched lizards (Uta stansburiana uniformis) residing in a heterogeneous thermal environment. J. Exp. Zool. A Ecol. Integr. Physiol. 333, 732–743. doi: 10.1002/jez.2415
Huey, R. B., and Pianka, E. R. (1981). Ecological consequences of foraging mode. Ecology 62, 991–999. doi: 10.2307/1936998
Jessop, T. S., Hamann, M., Read, M. A., and Limpus, C. J. (2000). Evidence for a hormonal tactic maximizing green turtle reproduction in response to a pervasive ecological stressor. Gen. Comp. Endocrinol. 118, 407–417. doi: 10.1006/gcen.2000.7473
Jessop, T. S., Anson, J. R., Narayan, E., and Lockwood, T. (2015). An introduced competitor elevates corticosterone responses of a native lizard (Varanus varius). Physiol. Biochem. Zool. 88, 237–245. doi: 10.1086/680689
Jessop, T. S., Lane, M. L., Teasdale, L., Stuart-Fox, D., Wilson, R. S., Careau, V., et al. (2016). Multiscale evaluation of thermal dependence in the glucocorticoid response of vertebrates. Am. Nat. 188, 342–356. doi: 10.1086/687588
Jimeno, B., Briga, M., Hau, M., and Verhulst, S. (2018). Male but not female zebra finches with high plasma corticosterone have lower survival. Funct. Ecol. 32, 713–721. doi: 10.1111/1365-2435.13021
Jimeno, B., Hau, M., and Verhulst, S. (2017). Strong association between corticosterone levels and temperature-dependent metabolic rate in individual zebra finches. J. Exp. Biol. 220, 4426–4431. doi: 10.1242/jeb.166124
Johnson, M. A., Francis, C. D., Miller, E. T., Downs, C. J., and Vitousek, M. N. (2018). Detecting bias in large-scale comparative analyses: methods for expanding the scope of hypothesis-testing with Hormone Base. Integr. Comp. Biol. 58, 720–728. doi: 10.1093/icb/icy045
Kearney, M. R., Gillingham, P. K., Bramer, I., Duffy, J. P., and Maclean, I. M. (2020). A method for computing hourly, historical, terrain-corrected microclimate anywhere on earth. Methods Ecol. Evol. 11, 38–43. doi: 10.1111/2041-210X.13330
Kearney, M., Shine, R., and Porter, W. P. (2009). The potential for behavioral thermoregulation to buffer “cold-blooded” animals against climate warming. Proc. Natl. Acad. Sci. 106, 3835–3840. doi: 10.1073/pnas.0808913106
Kerr, G. D., Bottema, M. J., and Bull, C. M. (2008). Lizards with rhythm? Multi-day patterns in total daily movement. J. Zool. 275, 79–88. doi: 10.1111/j.1469-7998.2008.00413.x
Koolhaas, J. M., Baumans, V., Blom, H. J. M., Von Holst, D., Timmermans, P. J. A., Wiepkema, P. R., et al. (2001). “Behaviour, stress and well-being,” in Principles of laboratory animal science: A contribution to the humane use and Care of Animals and to the quality of experimental results. 2nd Edn. eds. L. F. van Zutphen, V. Baumans, and A. C. Beynen (Amsterdam: Elsevier Science BV), 77–102.
Landys, M. M., Ramenofsky, M., and Wingfield, J. C. (2006). Actions of glucocorticoids at a seasonal baseline as compared to stress-related levels in the regulation of periodic life processes. Gen. Comp. Endocrinol. 148, 132–149. doi: 10.1016/j.ygcen.2006.02.013
Lendvai, Á. Z., Loiseau, C., Sorci, G., and Chastel, O. (2009). Early developmental conditions affect stress response in juvenile but not in adult house sparrows (Passer domesticus). Gen. Comp. Endocrinol. 160, 30–35. doi: 10.1016/j.ygcen.2008.10.004
Maclean, I. M., Mosedale, J. R., and Bennie, J. J. (2019). Microclima: an r package for modelling meso-and microclimate. Methods Ecol. Evol. 10, 280–290. doi: 10.1111/2041-210X.13093
McEwen, B. S., and Wingfield, J. C. (2003). The concept of allostasis in biology and biomedicine. Horm. Behav. 43, 2–15. doi: 10.1016/S0018-506X(02)00024-7
Megía-Palma, R., Arregui, L., Pozo, I., Žagar, A., Serén, N., Carretero, M. A., et al. (2020). Geographic patterns of stress in insular lizards reveal anthropogenic and climatic signatures. Sci. Total Environ. 749:141655. doi: 10.1016/j.scitotenv.2020.141655
Megía-Palma, R., Barja, I., and Barrientos, R. (2022). Fecal glucocorticoid metabolites and ectoparasites as biomarkers of heat stress close to roads in a Mediterranean lizard. Sci. Total Environ. 802:149919. doi: 10.1016/j.scitotenv.2021.149919
Meiri, S. (2018). Traits of lizards of the world: variation around a successful evolutionary design. Glob. Ecol. Biogeogr. 27, 1168–1172. doi: 10.1111/geb.12773
Meiri, S., Bauer, A. M., Chirio, L., Colli, G. R., Das, I., Doan, T. M., et al. (2013). Are lizards feeling the heat? A tale of ecology and evolution under two temperatures. Glob. Ecol. Biogeogr. 22, 834–845. doi: 10.1111/geb.12053
Mitchell, J. W. (1976). Heat transfer from spheres and other animal forms. Biophys. J. 16, 561–569. doi: 10.1016/S0006-3495(76)85711-6
Nelson, K. J., and Gregory, P. T. (2000). Activity patterns of garter snakes, Thamnophis sirtalis, in relation to weather conditions at a fish hatchery on Vancouver Island, British Columbia. J. Herpetol. 34, 32–40. doi: 10.2307/1565235
Porter, W. P., Mitchell, J. W., Beckman, W. A., and DeWitt, C. B. (1973). Behavioral implications of mechanistic ecology. Oecologia 13, 1–54. doi: 10.1007/BF00379617
Preest, M. R., and Cree, A. (2008). Corticosterone treatment has subtle effects on thermoregulatory behavior and raises metabolic rate in the New Zealand common gecko, Hoplodactylus maculatus. Physiol. Biochem. Zool. 81, 641–650. doi: 10.1086/590371
R Core Team. (2022). R: A language and environment for statistical computing. R Foundation for Statistical Computing, Vienna, Austria.
Racic, A., Tylan, C., and Langkilde, T. (2020). Effects of temperature on plasma corticosterone in a native lizard. Sci. Rep. 10, 1–8. doi: 10.1038/s41598-020-73354-z
Remage-Healey, L., and Romero, L. M. (2001). Corticosterone and insulin interact to regulate glucose and triglyceride levels during stress in a bird. Am. J. Physiol. Regul. Integr. Comp. Physiol. 281, R994–R1003. doi: 10.1152/ajpregu.2001.281.3.R994
Romero, L. M. (2002). Seasonal changes in plasma glucocorticoid concentrations in free-living vertebrates. Gen. Comp. Endocrinol. 128, 1–24. doi: 10.1016/S0016-6480(02)00064-3
Romero, L. M., and Beattie, U. K. (2022). Common myths of glucocorticoid function in ecology and conservation. J. Exp. Zool. A Ecol. Integr. Physiol. 337, 7–14. doi: 10.1002/jez.2459
Romero, L. M., Dickens, M. J., and Cyr, N. E. (2009). The reactive scope model—a new model integrating homeostasis, allostasis, and stress. Horm. Behav. 55, 375–389. doi: 10.1016/j.yhbeh.2008.12.009
Romero, L. M., and Wingfield, J. C. (2015). Tempests, poxes, predators, and people: Stress in wild animals and how they cope. New York, NY: Oxford University Press.
Rubalcaba, J. G., and Jimeno, B. (2022). Biophysical models unravel associations between glucocorticoids and thermoregulatory costs across avian species. Funct. Ecol. 36, 64–72. doi: 10.1111/1365-2435.13946
Rubalcaba, J. G., and Olalla-Tárraga, M. Á. (2020). The biogeography of thermal risk for terrestrial ectotherms: scaling of thermal tolerance with body size and latitude. J. Anim. Ecol. 89, 1277–1285. doi: 10.1111/1365-2656.13181
Sapolsky, R. M., Romero, L. M., and Munck, A. U. (2000). How do glucocorticoids influence stress responses? Integrating permissive, suppressive, stimulatory, and preparative actions. Endocr. Rev. 21, 55–89. doi: 10.1210/edrv.21.1.0389
Shine, R. (2005). Life-history evolution in reptiles. Annu. Rev. Ecol. Evol. Syst. 36, 23–46. doi: 10.1146/annurev.ecolsys.36.102003.152631
Stevenson, R. D. (1985). The relative importance of behavioral and physiological adjustments controlling body temperature in terrestrial ectotherms. Am. Nat. 126, 362–386. doi: 10.1086/284423
Taylor, C. R. (1973). “Energy cost of locomotion,” in Comparative physiology: Locomotion, respiration, transport, and blood. eds. L. Bolis, K. Schmidt-Nielsen, and S. H. P. Maddrell (Amsterdam, The Netherlands: North-Holland Publishing Co p), 23–42.
Telemeco, R. S., and Addis, E. A. (2014). Temperature has species-specific effects on corticosterone in alligator lizards. Gen. Comp. Endocrinol. 206, 184–192. doi: 10.1016/j.ygcen.2014.07.004
Tyrrell, C. L., and Cree, A. (1998). Relationships between corticosterone concentration and season, time of day and confinement in a wild reptile (tuatara, Sphenodon punctatus). Gen. Comp. Endocrinol. 110, 97–108. doi: 10.1006/gcen.1997.7051
Vitousek, M. N., Johnson, M. A., Donald, J. W., Francis, C. D., Fuxjager, M. J., Goymann, W., et al. (2018). Hormone Base, a population-level database of steroid hormone levels across vertebrates. Sci. Data 5, 1–7. doi: 10.1038/sdata.2018.97
Vitt, L. J., Pianka, E. R., Cooper, W. E. Jr., and Schwenk, K. (2003). History and the global ecology of squamate reptiles. Am. Nat. 162, 44–60. doi: 10.1086/375172
Wingfield, J. C. (2005). The concept of allostasis: coping with a capricious environment. J. Mammal. 86, 248–254. doi: 10.1644/BHE-004.1
Wingfield, J. C. (2013). Ecological processes and the ecology of stress: the impacts of abiotic environmental factors. Funct. Ecol. 27, 37–44. doi: 10.1111/1365-2435.12039
Keywords: corticosterone, ectotherms, temperature dependence, metabolism, energy demand
Citation: Rubalcaba JG and Jimeno B (2022) Body temperature and activity patterns modulate glucocorticoid levels across lizard species: A macrophysiological approach. Front. Ecol. Evol. 10:1032083. doi: 10.3389/fevo.2022.1032083
Received: 30 August 2022; Accepted: 25 October 2022;
Published: 21 November 2022.
Edited by:
Shan Huang, Senckenberg Biodiversity and Climate Research Centre, GermanyReviewed by:
Rodrigo Megía Palma, University of Alcalá, SpainCopyright © 2022 Rubalcaba and Jimeno. This is an open-access article distributed under the terms of the Creative Commons Attribution License (CC BY). The use, distribution or reproduction in other forums is permitted, provided the original author(s) and the copyright owner(s) are credited and that the original publication in this journal is cited, in accordance with accepted academic practice. No use, distribution or reproduction is permitted which does not comply with these terms.
*Correspondence: Juan G. Rubalcaba, amcucnViYWxjYWJhQGdtYWlsLmNvbQ==
Disclaimer: All claims expressed in this article are solely those of the authors and do not necessarily represent those of their affiliated organizations, or those of the publisher, the editors and the reviewers. Any product that may be evaluated in this article or claim that may be made by its manufacturer is not guaranteed or endorsed by the publisher.
Research integrity at Frontiers
Learn more about the work of our research integrity team to safeguard the quality of each article we publish.