- 1Department of Biological Sciences, Vanderbilt University, Nashville, TN, United States
- 2Department of Earth and Environmental Sciences, Vanderbilt University, Nashville, TN, United States
- 3La Brea Tar Pits, Los Angeles, CA, United States
- 4Research and Collections, New York State Museum, Albany, NY, United States
- 5Department of Earth System Science, University of California, Irvine, Irvine, CA, United States
- 6Department of Geology and Geophysics, University of Utah, Salt Lake City, UT, United States
- 7Department of Biology, Loyola Marymount University, Los Angeles, CA, United States
- 8Department of Biology, Pace University, New York City, NY, United States
- 9Institute of the Environment and Sustainability, University of California, Los Angeles, Los Angeles, CA, United States
- 10Department of Earth Sciences, University of Southern California, Los Angeles, CA, United States
- 11Department of Anatomy, Des Moines University, Des Moines, IA, United States
- 12Department of Biological Sciences, Marshall University, Huntington, WV, United States
Studies of Rancho La Brea predators have yielded disparate dietary interpretations when analyzing bone collagen vs. enamel carbonate—requiring a better understanding of the relationship between stable carbon isotopes in these tissues. Stable carbon isotope spacing between collagen and carbonate (Δca-co) has also been used as a proxy for inferring the trophic level of mammals, with higher Δca-co values indicative of high carbohydrate consumption. To clarify the stable isotope ecology of carnivorans, past and present, we analyzed bone collagen (carbon and nitrogen) and enamel carbonate (carbon) of extinct and extant North American felids and canids, including dire wolves, sabertooth cats, coyotes, and pumas, supplementing these with data from African wild dogs and African lions. Our results reveal that Δca-co values are positively related to enamel carbonate values in secondary consumers and are less predictive of trophic level. Results indicate that the foraging habitat and diet of prey affects Δca-co in carnivores, like herbivores. Average Δca-co values in Pleistocene canids (8.7+/−1‰) and felids (7.0+/−0.7‰) overlap with previously documented extant herbivore Δca-co values suggesting that trophic level estimates may be relative to herbivore Δca-co values in each ecosystem and not directly comparable between disparate ecosystems. Physiological differences between felids and canids, ontogenetic dietary differences, and diagenesis at Rancho La Brea do not appear to be primary drivers of Δca-co offsets. Environmental influences affecting protein and fat consumption in prey and subsequently by predators, and nutrient routing to tissues may instead be driving Δca-co offsets in extant and extinct mammals.
Introduction
Rancho La Brea (RLB) is one of the best preserved and studied fossil localities in the world, providing insight into the ecology of ancient mammals over the past ~50,000 years in southern California (e.g., Merriam and Stock, 1932; Stock and Harris, 1992; Van Valkenburgh and Hertel, 1993; DeSantis et al., 2012, 2019; Meachen and Samuels, 2012). Herbivorous mammals trapped in asphalt seeps (“pits”) attracted carnivores that also became trapped, resulting in one of the world’s best localities for studying ancient predators and their prey (Stock and Harris, 1992). As predators normally occur in lower numbers than their prey in modern ecosystems, they are also typically rare in fossil accumulations. However, because of their abundance in these deposits, Rancho La Brea provides unique insight into predator and prey dynamics in the Late Pleistocene—a time characterized by dramatic climatic change and the arrival of humans in North America (Stock and Harris, 1992; Barnosky et al., 2004). One productive avenue of research has been the inference of diet based on stable isotopes, allowing deep insight into the ecosystem dynamics of Late Pleistocene Rancho La Brea (e.g., Coltrain et al., 2004). However, recent work on isotopic values from RLB carnivores has revealed a clear discrepancy between values derived from collagen vs. enamel carbonate (DeSantis et al., 2019, 2020), complicating dietary inferences from either tissue. The goals of this study are to quantify and test potential drivers responsible for collagen/carbonate offsets generally, and to use these understandings to clarify dietary inference at RLB.
Stable isotopes and dietary inference
Stable isotope analyses of bone and/or tooth enamel have clarified the dietary ecology of numerous animals across the globe, past and present (e.g., Cerling et al., 1997; DeSantis et al., 2009; Secord et al., 2012). While studies of extant animals benefit from the ability to sample different tissues (including hair, feathers, scales, blood, etc.), archeological and paleontological studies of ancient life are limited to the analysis of tissues that preserve original biogenic stable isotope signatures in the fossil record (e.g., bones and teeth). Enamel is primarily inorganic (Teruel et al., 2015; Kendall et al., 2018) and stable isotopes of carbon and oxygen have been used to infer diet and climate, respectively, through time (e.g., DeNiro and Epstein, 1978; Cerling et al., 1997; Levin et al., 2006; DeSantis et al., 2009; Secord et al., 2012). Bone and dentin collagen, which are organic, also provide insights into the diets of ancient animals (e.g., Schoeninger et al., 1983; Bocherens et al., 1994; Finucane et al., 2006; Fuller et al., 2006; Kellner and Schoeninger, 2007; Lee-Thorp, 2008; Tung et al., 2016). Specifically, carbon isotope values measured in collagen (bone or dentin) provide information about the protein component of an animal’s diet (Ambrose and Norr, 1993) and nitrogen isotope values clarify trophic level with higher values indicative of feeding at higher trophic levels and/or more meat or fish consumption (Schoeninger et al., 1983). In archeological studies, both tooth enamel (or bone apatite) and bone collagen are analyzed to infer differences between whole diet (e.g., carbohydrates, fat, and protein) and protein components of diet, respectively (e.g., Ambrose and Norr, 1993). However, paleontological studies often employ only one tool, due to a lack of preserved collagen, interest in only isotopes associated with a particular tissue (e.g., δ18O in carbonate, δ15N in collagen; Bocherens et al., 1994; DeSantis et al., 2009), and/or the need to minimize destructive sampling. Therefore, direct comparisons between data types from the same specimens are rare.
Those studies that have examined both tooth enamel and bone collagen stable isotopes from the same individual specimens (e.g., Krueger and Sullivan, 1984; Lee-Thorp et al., 1989; Ambrose and Norr, 1993; Clementz et al., 2007, 2009; Murphy et al., 2007; Bocherens et al., 2017; Codron et al., 2018) indicate that isotope values from the different tissues record different aspects of their diet. There is broad consensus that δ13Ccollagen reflects the protein component of diet, while δ13Ccarbonate reflects whole diet (e.g., protein, carbohydrates, and fats; Ambrose and Norr, 1993) and that the isotopic spacing between carbonate and collagen (Δca-co, δ13Ccarbonate - δ13Ccollagen) varies depending on trophic category (Krueger and Sullivan, 1984; Lee-Thorp et al., 1989; Clementz et al., 2009). Specifically, the lowest Δca-co values occur in carnivores (averaging 4.3+/−1.0‰ to 4.8+/−0.4‰; Lee-Thorp et al., 1989; Clementz et al., 2009) while herbivores have higher Δca-covalues (6.8+/−1.4‰ to 7.6+/−0.5‰; Lee-Thorp et al., 1989; Clementz et al., 2009). Animals with lower Δca-co values are often attributed as having more meat and/or fat in their diet than animals with higher Δca-co values—Δca-co values are therefore used to infer degree of meat and/or fat consumption in ancient mammals (Lee-Thorp et al., 1989; Clementz et al., 2009). In Canis lupus, Δca-co increases with increased δ13Ccarbonate values (p = 0.016, R = 0.51; per our analysis of the supplemental data in Clementz et al., 2009); however, there is no predictable relationship between Δca-co in bone apatite or bone collagen δ13C values in African carnivores (Codron et al., 2018). Further, the significant positive-relationship between Δca-co values and δ13Ccarbonate values in a sample of dire wolves (Aenocyon dirus, previously known as Canis dirus) at Rancho La Brea is similar to the relationship between Δca-co and δ13Ccollagen values in the African mammals included in Codron et al. (2018; DeSantis et al., 2020, see Figure 1B).
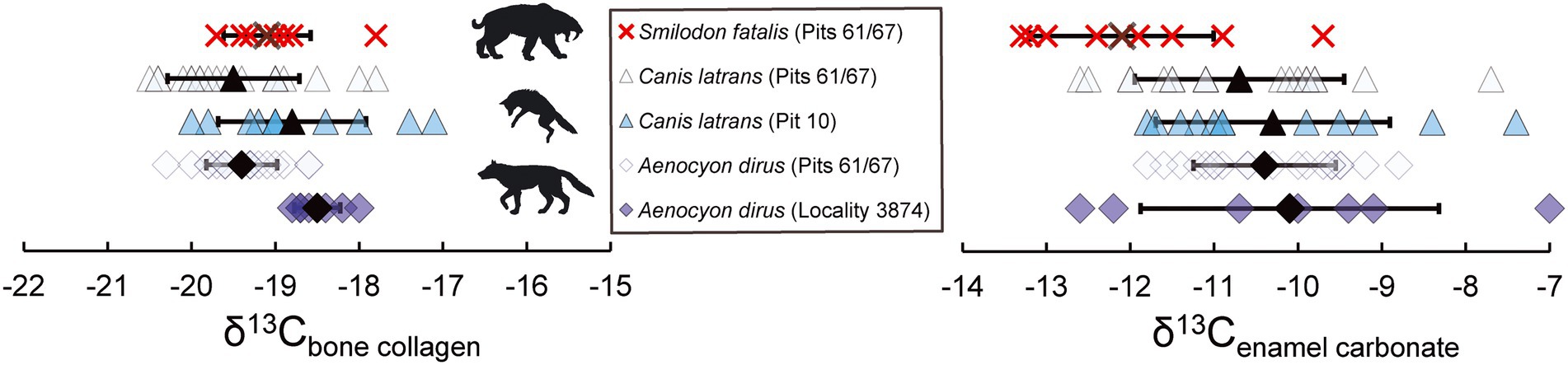
Figure 1. Stable carbon isotope data (collagen and carbonate) for all fossil specimens examined from Rancho La Brea (n = 71). Mean values are denoted in bold (bars indicate 1 standard deviation, n-1). All collagen data are from bone collagen and carbonate data are from tooth enamel (lower first molars), with the exception of A. dirus from Locality 3,874 which are from incisor dentin collagen and incisor enamel, respectively. The felid S. fatalis is noted in red, with all canids noted in blue; the same shade of light blue is used for canids from pits 61/67. The scales on the x-axis are of the same magnitude for the two tissues.
Offset values between carbonate and collagen can be highly variable among herbivores, specifically African mammals (primarily ungulates, especially bovids) and Australian kangaroos (Murphy et al., 2007; Codron et al., 2018). Murphy et al. (2007) demonstrated a significant negative relationship between Δca-co in herbivorous kangaroos and aridity (i.e., water availability index; higher Δca-co values when less water is available). Codron et al. (2018) instead documented higher Δca-co values with increased δ13Ccollagen values in African mammals, which they attribute to both physiological differences between ruminants and non-ruminants and dietary differences between grass and browse (e.g., effects of higher amounts of protein in 13C deplete browse and higher amounts of CH4 produced when consuming grass). In closely related ruminant taxa (i.e., members of the family Bovidae), Δca-co values calculated from teeth (enamel carbonate and dentin collagen) span the full range of values from 3.6 to 14.8‰; the second lowest offset value (4.3‰) and highest offset value (14.8‰) also occur with the same species—springbok (Antidorcas marsupialis). Further, when re-analyzing and comparing just tooth data (enamel carbonate and dentin collagen) from Codron et al. (2018) from ruminants in the family Bovidae and hindgut fermenters (in the families Equidae and Rhinocerotidae), the slopes and R2 values are indistinguishable (slopes are 0.21 and 0.20, R2 values are 0.41 and 0.43, respectively). Thus, it is unlikely that ruminant or non-ruminant physiology is the primary driver of Δca-co values; instead, the proportion of grass and browse may be a larger driver of herbivore Δca-co values. Specifically, higher amounts of protein enriched browse with 13C deplete values yield both lower δ13C carbonate values and smaller Δca-co values, while protein deplete C4 grasses also result in more methane production (that is isotopically depleted in 13C, leaving behind more 13C enriched carbon from which tissues are synthesized, in both ruminants and hind-gut fermenters) and are correlated with higher δ13C carbonate values and higher Δca-co values. Discrepancies between how herbivore and carnivore Δca-co values correlate with stable isotope values of collagen and enamel are apparent and more work on carbonate-collagen relationships is needed to elucidate potential drivers of Δca-co values and the efficacy of using Δca-co values to infer ecological information such as trophic level in extinct and extant mammals.
Stable isotopes and dietary inferences in RLB carnivores
Despite extensive study of Rancho La Brea carnivores, there are differences between the dietary interpretations of Late Pleistocene mammals at Rancho La Brea as inferred from bone collagen versus enamel carbonate stable isotope values (Coltrain et al., 2004; Feranec et al., 2009; Fuller et al., 2014, 2020; DeSantis et al., 2019, 2020; Van Valkenburgh et al., 2020). Seminal work analyzed the carbon and nitrogen isotope ratios of bone collagen from Rancho La Brea carnivores and concluded that sampled individuals of Smilodon fatalis (the sabertooth cat) and Aenocyon dirus (the dire wolf, previously known as Canis dirus) had indistinguishable carbon and nitrogen isotope values and were therefore competing for similar prey (Coltrain et al., 2004). This idea persisted until dietary interpretations based on tooth enamel carbonate indicated the consumption of different prey (DeSantis et al., 2019, 2020). Specifically, DeSantis et al. (2019, 2020) found that A. dirus yielded statistically greater δ13Ccarbonate values than S. fatalis—which was interpreted as S. fatalis primarily having consumed prey that occupied denser forests/shrubland ecosystems, while A. dirus consumed prey from more open grassland environments. The reasons for this discrepancy between the two tissues remain unclear (Fuller et al., 2014, 2020; DeSantis et al., 2019, 2020), and have led some to question the recent dietary interpretations of Rancho La Brea carnivorans (Van Valkenburgh et al., 2020). While DeSantis et al. (2019, 2020) speculate on some of these discrepancies, they concentrated on an enamel-based interpretation of diet.
To clarify the paleobiology of Rancho La Brea carnivores and resolve relationships between enamel carbonate and bone collagen in both extinct and extant mammals, tooth enamel and bone collagen were examined in extant canids and felids from C3 and C4 ecosystems in North America and Africa, in addition to Rancho La Brea carnivorans. Specifically, we examined Late Pleistocene specimens from Rancho La Brea (A. dirus, C. latrans, S. fatalis), modern specimens from predominately C3 ecosystems in southern California (C. latrans and Puma concolor), and modern specimens from predominately C4 ecosystems throughout Africa (Lycaon pictus and Panthera leo). We test the hypotheses that Δca-co values in carnivorous mammals (1) are largely consistent and independent of either δ13Ccarbonate or δ13Ccollagen values (~4.3+/−1.0‰ to 4.8+/−0.4‰; Lee-Thorp et al., 1989; Clementz et al., 2009), and (2) are indicative of trophic level. We also further clarify relationships between carbonate (enamel, bone, and dentin) and collagen (bone and dentin) in extant African canids and felids.
Statistical considerations of isotopic data from different tissues
We recognize that the correlations between Δca-co values and δ13Ccarbonate or δ13Ccollagen, may, in part, be influenced by plotting these values against each other; but these plots and correlations permit comparison to other studies, and provide information regarding the source of these isotopic data. As Auerswald et al. (2010) demonstrated, if pairs of values are drawn randomly from populations of variables A and B of equal variance, the difference A-B will be biased to high values when A is high and to low values when A is low. Hence an A-B vs. A correlation with a slope of +1 is generated; conversely A-B vs. B will yield a slope of-1. In the unrealistic scenario that δ13C variations in the two tissues were completely independent (i.e., if their responses to environmental and physiological changes were totally uncoupled) the slopes of these relationships would approach +1 and-1 respectively, whereas if the isotopic responses of the two tissues were identical the slopes would both be zero. Since Δca-co is defined as δ13Ccarbonate − δ13Ccollagen, all other things being equal one expects to see a positive correlation between Δca-co and δ13Ccarbonate and a negative correlation between Δca-co and δ13Ccollagen. Additionally, if these relationships were a product of random sampling, we would not expect δ13C values of different tissues to yield different relationships with Δca-co values. See Caut et al. (2010) for a detailed reply to Auerswald et al. (2010) and discussion regarding the rationale for these sorts of comparisons that are prevalent in paleobiology and archeology (e.g., Krueger and Sullivan, 1984; Lee-Thorp et al., 1989; Ambrose and Norr, 1993; Murphy et al., 2007; Clementz et al., 2009; Codron et al., 2018).
Differences in isotopic variance of tissue types are evident from our data set and the extensive data sets of Murphy et al. (2007) and Codron et al. (2018). However, this is not necessarily an intrinsic property of these tissues; studies have shown that δ13Ccollagen ranges are greater than those in δ13Ccarbonate in humans when their diets include protein sources with disparate δ13C values (e.g., marine protein enriched in 13C in addition to terrestrial C3 resources that are 13C deplete; Tung et al., 2020).
Materials and methods
To decipher the conflicting data interpretations between the stable isotope values of bone collagen and tooth enamel carbonate in Rancho La Brea carnivores, jawbone specimens containing teeth (n = 71) were obtained for stable isotope analysis of both bone collagen and tooth enamel carbonate in the same specimen. Lower first molars were sampled in all taxa due to the larger size of these carnassial teeth and the need to minimize specimen destruction. In the case of Aenocyon dirus from the University of California Museum of Paleontology (UCMP) Locality 3,874, enamel was from incisors while collagen was from dentin (both near the enamel/dentin junction and down through the root, due to the need for appropriately large amounts of this tissue, as these teeth were isolated and lacked associated bone for bone collagen analysis; as noted in Supplementary Table S1, Supplemental Tables 1, 2). Rancho La Brea specimens comprised Aenocyon dirus (Pits 61/67, n = 21; Locality 3,874, n = 8), Canis latrans (Pits 61/67, n = 19; Pit 10, n = 12), and Smilodon fatalis (Pit 61/67, n = 11; Supplementary Table S1, Supplemental Table 1). Modern predators from southern California (n = 19; Canis latrans, Puma concolor) and from Africa (n = 18; Lycaon pictus, Panthera leo) were also analyzed for both bone collagen and tooth enamel (Supplementary Tables S1, S2, Supplemental Table 2). The African specimens were also analyzed for bone (carbonate) and dentin (carbonate and collagen), in addition to enamel (carbonate) and bone (collagen). When more than one analysis was run on the same tissue, the sample was first homogenized and then split up after drilling to reduce temporal variability that could otherwise occur if tissues were not homogenized after sampling. While we would have liked to have sampled dentin carbonate and collagen from all Rancho La Brea specimens, this was not possible due to the scale and scope of this study and need to minimize specimen destruction on the fossil specimens (collagen analyses require significantly more sample as compared to carbonate). As tooth enamel carbonate and bone collagen are the most commonly analyzed tissues at Rancho La Brea and other Late Pleistocene fossil sites, we concentrated on these tissues for the bulk of our analyses. The addition of dentin carbonate and collagen and bone carbonate of modern African specimens can provide insights that can be followed up with future studies. Rancho La Brea specimens are housed at the University of California, Berkeley (UCMP Locality 3,874) and the La Brea Tar Pits and Museum (LACMHC). Modern specimens from southern California are from the Santa Barbara Museum of Natural History (SBMNH) and modern specimens from Africa are from the American Museum of Natural History (Mammalogy Collections; AMNH).
Carbonate samples from both teeth and bone were drilled with a low-speed dental-style drill and carbide dental burrs (<1 mm burr width). The resulting carbonate powder samples (enamel, dentin, bone) were pretreated with 30% hydrogen peroxide for 24 h, and 0.1 N acetic acid for 18 h, to remove organics and secondary carbonates, respectively (Koch et al., 1997; DeSantis et al., 2009). Sample preparation followed procedures outlined in DeSantis et al. (2019) and included the rinsing of samples with ultra-pure water and subsequently centrifuging them between all treatment steps. Approximately 1 mg of each sample was then analyzed on a VG Prism stable isotope ratio mass spectrometer with an in-line ISOCARB automatic sampler in the Department of Geological Sciences at the University of Florida. All bone or dentin samples analyzed for collagen carbon or nitrogen isotope ratios were cut with a Dremel® UltraSaw ™ cutting wheel or a diamond coring drill (dentin samples also sampled for carbonate were subsequently drilled, i.e., a subset of the sample was removed for carbonate analysis).
Clean collagen was recovered from the asphalt-impregnated bones using a modified procedure based on the protocol developed by Fuller et al. (2014). Samples of crushed bone were sonicated in a solvent sequence of increasing polarity to remove bulk asphalt, demineralized overnight with HCl, rinsed with ultrapure water, and gelatinized overnight at pH 2, at an elevated temperature (75°C) sufficient to break up collagen molecules into fragments of a molecular weight on the order of 10’s of kDa, while leaving massive residual asphalt aggregates intact. The gelatin solution was then purified using two sequential ultrafiltrations to select an intermediate molecular weight fraction (5–100 kDa) which was frozen with liquid nitrogen and lyophilized overnight. Elemental analyses (‰C and ‰N) and stable isotope ratios were determined by flash combustion of ~0.7 mg samples of collagen in tin capsules, and analysis of the gas products using a Fisons NA1500NC elemental analyzer/Finnigan Delta Plus isotope ratio mass spectrometer combination at the Keck AMS laboratory at University of California Irvine. Stable isotope data are reported in conventional delta (δ) notation for carbon (δ13C), oxygen (δ18O), and nitrogen (δ15N), where δ13C (parts per thousand, ‰) = [(Rsample/Rstandard)−1]*1,000, and R = 13C/12C; and, δ15N (parts per mil, ‰) = ((Rsample/Rstandard)-1)*1,000, and R = 18O/16O, R = 15N/14N on the VPDB (for carbon and oxygen; Pee Dee Belemnite, Vienna Convention; Coplen, 1994) and AIR (for nitrogen; Mariotti, 1983) scales. The analytical precision for carbonate δ13C and δ18O is ±0.1‰, based on replicate analyses of samples and standards (NBS-19). The analytical precisions for δ13C and δ15N from bone collagen are ±0.1‰, and ±0.2‰ respectively, based on replicate analyses of samples and standards.
Studies utilizing carbonate-collagen spacing use “capital delta notation” values, calculated as follows: Δca-co = δ13Ccarbonate − δ13Ccollagen (e.g., Krueger and Sullivan, 1984; Lee-Thorp et al., 1989; Ambrose and Norr, 1993; Clementz et al., 2007; Murphy et al., 2007; Clementz et al., 2009; Bocherens et al., 2017; Codron et al., 2018). While we use these calculations to be directly comparable with prior analyses, we also calculated the fractionation factor (α) and isotopic enrichment (ε; Supplemental Table 1) similar to Passey et al. (2005), based on Friedman and O’Neil (1977). Specifically, αcarbonate-collagen = (1,000+ δcarbonate)/ (1,000+ δcollagen), and εcarbonate-collagen = (αcarbonate-collagen – 1)1000 (Friedman and O’Neil, 1977). Differences between Δca-co and εcarbonate-collagen are small within the ~10‰ range here noted (Cerling and Harris, 1999); of all specimens examined here, the average difference is 0.133‰ +/−0.0518 SD, n-1 (ranging from 0.029 to 0.207‰). These differences are minor (approximately the error of the mass spectrometers), do not significantly affect the patterns observed, and the use of Δca-co is important for consistency with published works (e.g., Krueger and Sullivan, 1984; Lee-Thorp et al., 1989; Ambrose and Norr, 1993; Murphy et al., 2007; Clementz et al., 2009; Codron et al., 2018); however, we suggest that α and ε be reported and discussed moving forward, as noted by Passey et al. (2005; all values are reported in the Supplemental information).
Parametric statistical tests were employed to compare stable isotope values among taxa, between tissues, and assess relationships between isotopic values and Δca-co values (e.g., Student’s t-tests, including paired t-tests when comparing tissues in the same individuals, linear regressions). No corrections (including the Suess correction) were made to reported and analyzed data (Supplementary Tables S1, S2, Supplemental Tables 1, 2). While we recognize that δ13C values today are approximately 1.5‰ lower than during the Late Pleistocene (Cerling et al., 1997; Cerling and Harris, 1999; Passey et al., 2005), we are focused on assessing relationships between δ13C values from disparate tissues within individuals (e.g., relationships between δ13Ccarbonate and Δca-co, as opposed to comparing diets of extant and extinct predators) and the majority of comparisons are done within modern or within Late Pleistocene samples. Further, it is possible that the δ13C values of the atmosphere (which are a function of CO2 levels; Keeling, 1979; Keeling et al. 1979) affect how δ13C values are incorporated into various tissues via plants and prey, as we suggest in the discussion. All things being equal, plants exposed to higher atmospheric CO2 levels have lower nutrients and lower nitrogen content/lower protein content but more carbon per unit biomass, and C4 grasses have lower nutritional quality (Conroy, 1992; Barbehenn et al., 2004). This can impact nutrient routing to tissues in consumers and have downstream impacts on carnivores. Further, all studies to date have examined relationships between δ13Ccarbonate and Δca-co in modern taxa with no Suess corrections applied (e.g., Lee-Thorp et al., 1989; Ambrose and Norr, 1993; Murphy et al., 2007; Clementz et al., 2009; Codron et al., 2018), and we aimed to produce data directly comparable to those data sets.
Results
Bone collagen and enamel carbonate δ13C values from Rancho La Brea
Stable carbon isotopes measured in bone collagen of S. fatalis (Pits 61/67) are indistinguishable from A. dirus (Pits 61/67; p = 0.141; Figure 1), consistent with prior work (Coltrain et al., 2004; Fuller et al., 2014, 2020). In contrast, stable carbon isotopes measured in enamel carbonate of S. fatalis (Pits 61/67) are significantly lower than in A. dirus (Pits 61/67; p < 0.0001; Figure 1), which is also consistent with prior analyses of enamel carbonate (DeSantis et al., 2019, 2020). When comparing the three most abundant carnivores at Rancho La Brea from the same deposit (A. dirus, C. latrans, and S. fatalis from Pits 61/67), bone collagen carbon isotope values are indistinguishable (p = 0.187), while enamel carbon isotope values of the canids A. dirus and C. latrans are significantly greater than S. fatalis (p = 0.0002, p = 0.004, respectively). Further, stable nitrogen isotopes ratios measured in the morphologically inferred hypercarnivores A. dirus and S. fatalis are significantly greater than C. latrans (p < 0.0001 for both), while A. dirus and S. fatalis are indistinguishable from one another (p = 0.332). Despite the fact that bone collagen and enamel carbonate integrate information about different portions of animal diets (protein component versus whole diet; Ambrose and Norr, 1993), there is a significant positive relationship between enamel δ13Ccarbonate and δ13Ccollagen from bone in both the fossil Rancho La Brea specimens (p < 0.0001, R2 = 0.25, R = 0.5 in Rancho La Brea taxa, Figure 2A) and modern southern California (p = 0.0002, R2 = 0.57, R = 0.75; Supplemental Table 1) specimens examined here.
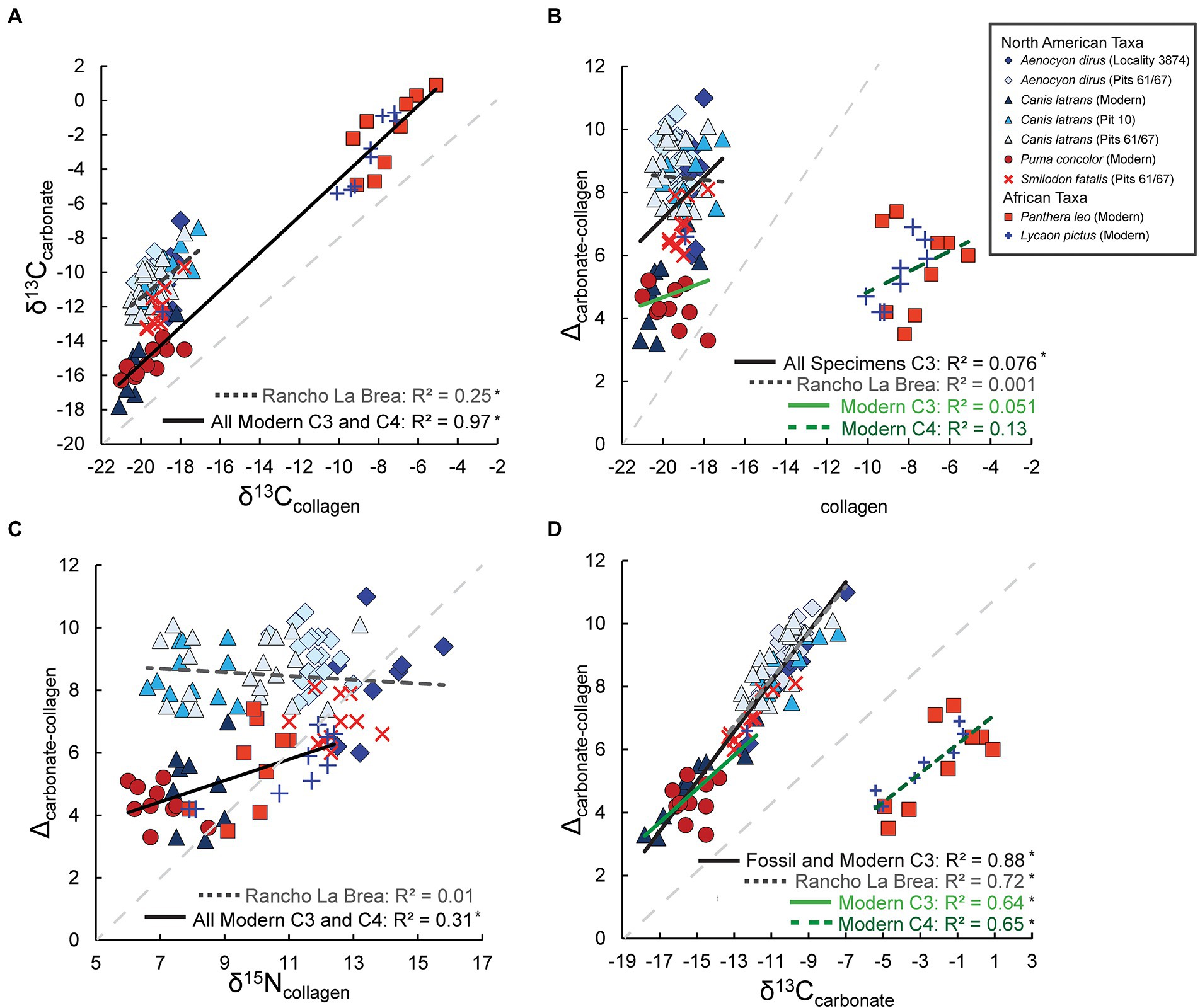
Figure 2. Relationship between δ13Ccarbonate and δ13Ccollagen values (A), and Δca-co and δ13Ccollagen values (B), δ15Ncollagen values (C), and δ13Ccarbonate values (D; n = 108). The regression lines and R2 values correspond to text in the same color and are also noted in Supplementary Table S3. Note, the modern C3 regression lines in (A) and (B) includes modern North American taxa and one African wild dog with a C3 value (Supplementary Tables S3, S4 only include geographically similar taxa). The legend in (B) applies to all (A–D). A light-gray dashed-line with a slope of 1 is present in the background of each panel for reference. *Denotes significant relationships (p < 0.05).
Collagen-carbonate δ13C spacing in carnivorans from southern California
There is a strong and significant positive relationship between Δca-co and δ13Ccarbonate in enamel from both extant and extinct southern California canids and felids (R2 = 0.88, R = 0.94; p < 0.0001; Figure 2D; Supplementary Table S3). This relationship additionally holds true when modern and fossil specimens are evaluated separately (modern: R2 = 0.58, R = 0.76, p = 0.0002; fossil: R2 = 0.72, R = 0.85, p < 0.0001). Because modern specimens of the canid C. latrans and the felid P. concolor have lower δ13Ccarbonate values than the extinct felids and canids from Rancho La Brea, likely due to their consumption of prey from ecosystems with increased canopy cover or denser shrubs (Feranec and DeSantis, 2014; DeSantis et al., 2019) and the Suess effect that accounts for ~1.5‰ lower δ13Ccarbonate values (Cerling et al., 1997; Cerling and Harris, 1999; Passey et al., 2005); we graphically present the results of linear regressions for fossil and modern specimens separately (see Materials and Methods, Figure 2). However, all relationships between enamel δ13Ccarbonate and Δca-co were examined and reported (Supplementary Table S3). Δca-co values and δ13Ccollagen values exhibit no significant correlation when including either Rancho La Brea specimens (R = 0.03, p = 0.767) or modern specimens from southern California (R = 0.14, p = 0.531); however, there is a weak but significant positive relationship when both Rancho La Brea specimens and modern C3 specimens are included (Figure 2B; Supplementary Table S4). In contrast to δ13Ccollagen values, δ13Ccarbonate values can be used to predict Δca-co values; thus, δ13Ccollagen values can be estimated within 0.5‰.
Rancho La Brea specimens have average Δca-co values of 8.5+/−1.2‰ (ranging from 6 to 11‰, n = 71) and modern specimens from southern California have average Δca-co values of 4.6+/−1‰ (ranging from 3.2 to 7‰, n = 19). Rancho La Brea canid and felid Δca-co values (8.7+/−1.0‰, 7.0+/−0.7‰, respectively) overlap with modern herbivore Δca-co values (Lee-Thorp et al., 1989; Murphy et al., 2007; Clementz et al., 2009; Codron et al., 2018) and are higher than modern carnivores based on the literature (averaging 4.3+/−1.0‰ to 4.8+/−0.4‰; Lee-Thorp et al., 1989; Clementz et al., 2009).
Most notably, Δca-co values of both Rancho La Brea carnivores and modern carnivores from southern California are not significantly (negatively) correlated with δ15Ncollagen values as was expected (Figure 3C; Supplementary Table S4), based on prior research that documents higher Δca-co values in more omnivorous or herbivorous taxa (e.g., Lee-Thorp et al., 1989; Clementz et al., 2009). C. latrans from Pits 61/67 at Rancho La Brea exhibit significantly lower δ15Ncollagen values than both A. dirus (p < 0.0001) and S. fatalis (p < 0.0001) from the same location, suggesting that coyotes were more omnivorous than the larger, more abundant, and inferred hypercarnivores (A. dirus and S. fatalis; Merriam and Stock, 1932; Stock and Harris, 1992). Yet, the mean Δca-co value of C. latrans is indistinguishable from A. dirus (p = 0.411, Mann–Whitney U).
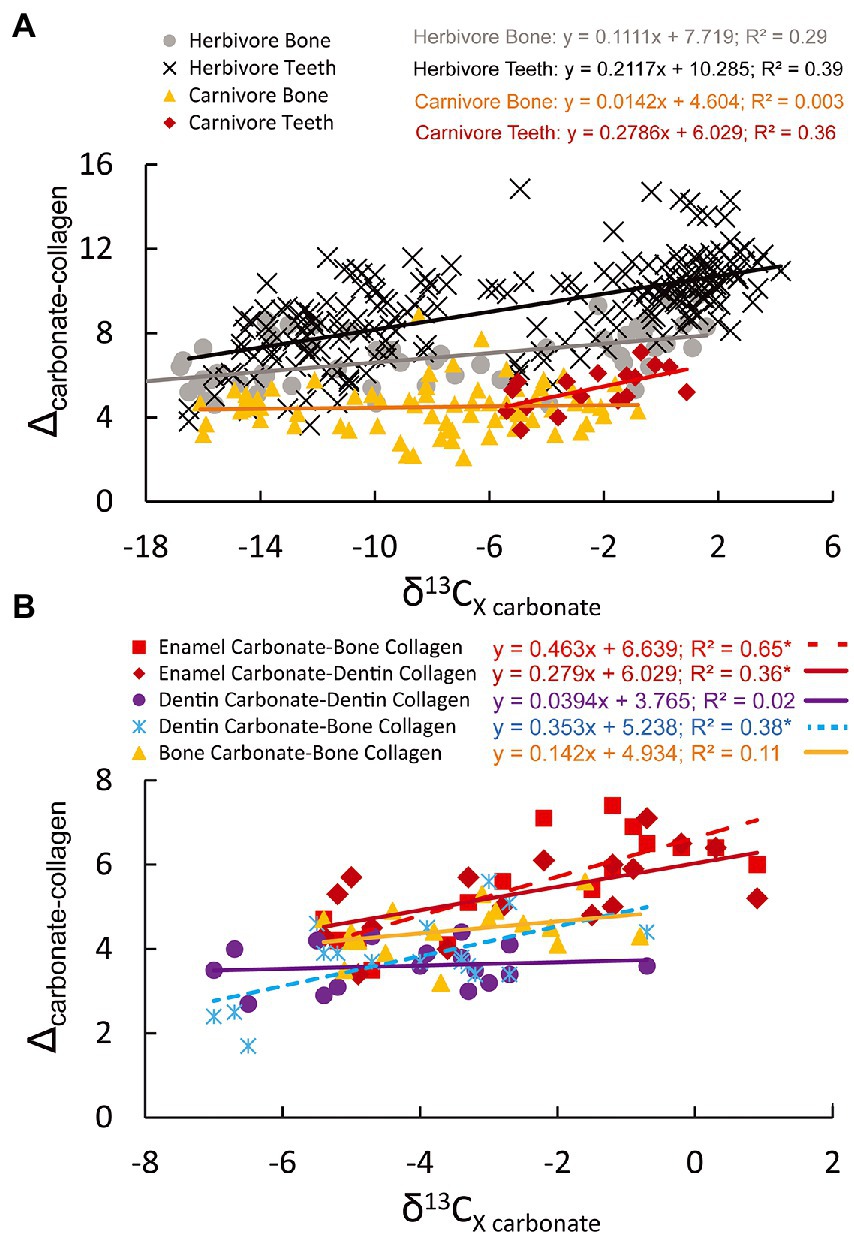
Figure 3. Relationships between δ13Ccarbonate (bone, dentin, or enamel) and Δca-co (calculated from carbonate-collagen from different tissues) in African herbivores (A) and carnivores (A,B). Data from herbivores are from Codron et al. (2018), in addition to some of the carnivore bone samples noted in panel A. The regression lines and R2 values correspond to text in the same color and are also noted in Supplementary Table S3. The dashed red-line in (B) is for enamel carbonate-bone collagen (squares). *Denotes significant relationships (p < 0.05).
Collagen-carbonate δ13C spacing and tissue specific enrichment in carnivorans from Africa
As in southern California, there is a strong (R2 = 0.65, R = 0.81) and significant positive relationship (p = 0.0001; Supplementary Table S3) between δ13Ccarbonate in enamel and Δca-co in African canids and felids from predominantly C4 ecosystems. One individual with a δ13Cenamel carbonate value of −12.3‰, interpreted as consuming significant C3 prey (and ~ 3 standard deviations from the mean) fell on the regression line from southern California extant and extinct taxa (Figure 2A) and was not included in the analysis of exclusively African (C4) carnivores; however, it was included in the regression of C3 taxa in Figures 2B,D, and relevant regressions of all carnivores (or modern carnivores; per Materials and Methods). δ13Ccollagen and Δca-co values are not significantly correlated to one another in exclusively C4 carnivorans (R2 = 0.13, p = 0.158; Figure 2B; Supplementary Table S4), while δ15Ncollagen and Δca-co values exhibit a significant positive relationship (R2 = 0.31, p = 0.021; Supplementary Table S4, all data located in Supplemental Table 2).
There are differences among stable carbon isotope values measured in different tissues (Supplementary Table S5). Collagen consistently has lower δ13C values than carbonate samples by 3.6–5.5‰, which is consistent with prior work (e.g., Lee-Thorp et al., 1989; Ambrose and Norr, 1993). Enamel δ13Ccarbonate values are significantly higher than both dentin δ13Ccarbonate values (p < 0.001) and bone δ13Ccarbonate values (p = 0.004), though mean differences are low (1.7 and 1.1‰, respectively; Supplementary Table S5). Further, dentin δ13Ccarbonate values are significantly lower than bone δ13Ccarbonate values (p = 0.029; Supplementary Table S5). All carbon isotopes signatures from all tissues sampled (enamel, dentin, and bone) yield significantly different values from one another (all p-values≤0.001; Supplementary Table S5). Only dentin and bone δ13Ccollagen values yield mean values indistinguishable from one another (p = 0.504; Supplementary Table S5). Mean δ15Ncollagen values from dentin are significantly higher than bone collagen (p < 0.001; Supplementary Table S5); however, the mean difference is quite small (0.9 +/−0.7‰; Supplementary Table S5). Further, the tissues with the strongest correlations between δ13C values (dentin carbonate-dentin collagen, and bone carbonate-bone collagen) exhibit no significant relationships between apatite (dentin or bone) δ13Ccarbonate values and Δca-co in African carnivores (Supplementary Table S4). Enamel δ13Ccarbonate values are consistently positively correlated with Δca-co values (whether Δca-co is based on dentin or bone collagen; Supplementary Table S3). Dentin δ13Ccarbonate values are also significantly related to Δca-co values when bone collagen is used; however, neither bone nor dentin exhibit any significant relationships between carbonate δ13C values and Δca-co values when calculated from just bone or just dentin tissues (carbonate/collagen; Figure 3). Further, African carnivores from C4 ecosystems have average Δca-co values of 5.5+/−1.2‰ when comparing enamel and bone, however Δca-co values are lower when bone carbonate and bone collagen are used (4.4+/−0.6‰ for C4 carnivores, 4.7+/−0.6‰ for Lycaon pictus, and 4.2+/−0.5‰ for Panthera leo) and closer to values reported in the literature (averaging 4.3+/−1.0‰ to 4.8+/−0.4‰; Lee-Thorp et al., 1989; Clementz et al., 2009).
Collagen-carbonate δ13C and δ18O variances in carnivorans
Carbonate and collagen tissues do vary to different extents, with δ13Ccarbonate values having greater variances than δ13Ccollagen values (p < 0.0001 for both Levene’s Median test and Bartlett’s test) and a standard deviation almost double that of collagen (1.3 vs. 0.7‰) in Rancho La Brea samples. Modern specimens from C3 and C4 ecosystems were equivocal (C3 specimens, p = 0.111 and p = 0.039 for Levene’s Median test and Bartlett’s test; C4 specimens, p = 0.025 and p = 0.081 for Levene’s Median test and Bartlett’s test, respectively), but sample sizes were significantly lower (n = 20, n = 17, respectively) than Rancho La Brea specimens (n = 71).
Stable oxygen isotope ratios were measured and compared among all carbonate samples from southern California to assess if Rancho La Brea fossils had anomalously low δ18O values, potentially due to diagenesis. Modern canid and felid (i.e., Canis latrans and Puma concolor, respectively) enamel δ18O values ranged from-5.0 to-0.6 (mean = −2.6+/−1.3‰, n = 19) and were indistinguishable in both mean value (p = 0.336) and variance (p = 0.955) from δ18O values of fossil specimens that include C. latrans, A. dirus, and S. fatalis from Rancho La Brea (δ18O values ranged from-5.3 to 2.3; mean = −2.3+/−1.3‰, n = 71).
Discussion
Identifying the primary drivers of collagen-carbonate spacing in carnivores requires the testing of numerous hypotheses. Below we evaluate hypotheses pertaining to ontogenetic dietary differences, diagenesis, and physiological differences between canids and felids, eliminating these as potential drivers with data provided from this paper and published studies. We are unable to eliminate hypotheses pertaining to tissue and environmental/dietary differences, and their downstream impacts on Δca-co spacing. Further work is needed to clarify the exact mechanism(s) responsible for Δca-co spacing (including the role of trophic level in different ecosystems); regardless, this work helps to advance our understanding of a challenging problem and cautions the use of static offset values between collagen and carbonates in future work (as has become a more common practice; e.g., Clementz et al., 2009; Smith et al., 2022).
The absence of significant ontogenetic dietary differences and diagenetic effects on collagen-carbonate δ13C spacing
Tissues such as bone collagen and enamel carbonate mineralize at different times and undergo significant to no remodeling, respectively. While it is possible that ontogenetic differences in diet are responsible for the isotopic differences between bone collagen (representing a later in life signal due to continuous remodeling) and enamel carbonate (occurring earlier in life when the teeth mineralized and representing a discrete interval of time) in the carnivores examined, this would require dramatic dietary changes over time that are possible but not probable. The canids studied would need to eat prey from more open grassland environments as young individuals and then shift to eating forest-dwelling prey as adults, in a manner that predictably correlates with δ13Ccarbonate values in all felids and canids studied. This depiction of canid and felid ecology also does not align with morphological interpretations of extinct taxa or our knowledge of extant felid and canid behavior in the wild (Elliot et al., 1977; Bekoff and Wells, 1982). Specifically, the morphology of S. fatalis and A. dirus suggests terrestrial and cursorial hunting in these taxa, respectively (Samuels et al., 2013), while observational data suggests that extant felids typically use vegetation as cover to ambush prey while extant canids rarely utilize cover (Elliot et al., 1977; Bekoff and Wells, 1982). Additionally, both A. dirus and S. fatalis are interpreted as social predators (Carbone et al., 2009; McHorse et al., 2012); in extant social carnivores, juveniles typically consume the same prey as adults (i.e., meat and/or carcasses are shared, as observed in African lions; Schaller, 1972). Published adult δ13Ccollagen values that span multiple pits (Fuller et al., 2014, 2020) prepared via ultrafiltration (as was done here), were indistinguishable from juvenile δ13Ccollagen values from Locality 3,874 (p = 0.378; means of −18.4 and −18.5‰ respectively), indicating that juvenile dire wolves are eating similar prey as adults. Juvenile δ13Ccollagen values are from tooth dentin in A. dirus (Supplemental Table 1) and bone collagen from one juvenile humerus (Fuller et al., 2020). While δ13Ccollagen values from tooth dentin (laid down during the animal’s youth) in A. dirus (Locality 3,874; no published radiocarbon dates, a general locality name for material collected by the University of California Museum of Paleontology; Feranec et al., 2009) are significantly greater than δ13Ccollagen values from bone collagen from A. dirus deposited in Pits 61/67 (~11,581 +/−3,768 years before present, calibrated; O’Keefe et al., 2009), these differences may be a result of different ecologies during different sampling periods (i.e., Locality 3,874 may represent a much broader, narrower, or different span of time than Pits 61/67; Feranec et al., 2009, O’Keefe et al., 2009). Further, extant African canids and felids reveal only minor differences in δ13Ccarbonate values between bone (a more recent diet) and both enamel (1.1‰) or dentin (0.7‰; both enamel and dentin are laid down earlier in life; also see Figure 6 from Bocherens, 2015), suggesting that ‘whole diet’ ontogenetic differences are minor through time, with such minor differences potentially due to weaning. It should also be noted that δ13Ccollagen are indistinguishable between dentin and bone collagen in extant African carnivores (Supplementary Table S5), indicative of similar dietary protein sources through ontogeny in African canids and felids.
A case could be made that the difference in variance at Rancho La Brea is due to bone turnover smoothing out differences that appear in δ13C values of the enamel, the latter of which is laid down over a relatively short period of the animal’s lifespan and is not subject to reworking (as is the case with bone; Hedges et al. 2007). However, this cannot explain the dire wolf data from Locality 3,874, where enamel δ13C is compared with collagen from dentin (which like enamel, does not turn over; Helfman and Bada, 1976) though it is of course possible that the sampling geometry of enamel and dentin represent slightly different times (separated by days to month, not years; Bocherens, 2015). Further, the tooth dentin analyzed from Locality 3,874 has significantly higher δ15Ncollagen values than A. dirus from Pit 61/67 where bone collagen (not dentin collagen) was analyzed; suggesting that the dentin analyzed was laid down early in the life of the animal when it was still consuming a significant portion of its mother’s milk (i.e., 13.7+/−1.1‰ SD in dentin from Locality 3,874, 11.8 +/−0.5‰ in bone collagen from Pits 61/67; p < 0.0001). At locality 3,874, δ13Ccarbonate values range from −12.6 to −7.0‰ while δ13Ccollagen values of the same individuals range from −18.8 to −18‰, in the same teeth. Therefore, differences in δ13C variability likely stems from difference in δ13C variability of protein and whole diet sources, and not the timing or turnover of tissues. As mentioned above, lipids can be extremely 13C depleted relative to protein (Ramsay and Hobson, 1991) and may contribute to larger δ13Ccarbonate ranges as compared to δ13Ccollagen ranges. Hence, understanding relationships between δ13C values from different tissues and Δca-co values derived from those tissues has unique challenges. This highlights the importance of experimental studies and the benefits of obtaining data from multiple tissue types (especially from fossils) when possible—both to best understand protein and whole diet sources while also providing further resolution to understanding relationships between δ13C and Δca-co values.
If a nursing/weaning signal was apparent, we would expect both enamel and dentin to have significantly lower δ13Ccarbonate values than bone δ13Ccarbonate values (due to the consumption of fattier milk with lower δ13C values while nursing and/or weaning earlier in life; e.g. Richards et al., 2002; Fuller et al., 2006; Tsutaya and Yoneda, 2015), and this is not the case. Contributions from weaning are also less likely due to early weaning of canids (by ~5–8 weeks of age; Mech, 1974, Ewer, 1998, Nowak and Walker, 1999) while weaning occurs later in large felids (e.g., African lions, weaning by ~8–9 months; Haas et al., 2005). Further, studies that examined the effect of weaning on δ13C values in tissues, including plasma, hair, and tooth enamel in humans and other mammals, showed its effect was minimal (<1‰; Tsutaya and Yoneda, 2015). Studies of non-primate taxa, including herbivores and carnivores, showed no evidence of 13C enrichment in the plasma of offspring (0.0+/−0.6‰; Jenkins et al., 2001), while 13C enrichment of ~1‰ was observed in the tissues (hair, fingernails, and/or ribs) of exclusively breastfed human infants (Richards et al., 2002; Fuller et al., 2006). Further, serial sampling of the enamel of two adult upper canines from S. fatalis from Rancho La Brea (which developed and mineralized from near birth up to 25 months of age) did not exhibit a weaning signal or any trend in δ13C values over time (Feranec, 2004; Wysocki et al., 2015).
Other possible reasons for the difference between collagen and carbonate δ13C values include differences in tissue formation and/or post-mortem differences in diagenesis between tissues. Bone collagen is organic, while enamel is primarily inorganic (only ~6–10‰ organic content; Teruel et al., 2015; Kendall et al., 2018). It is possible that the bone collagen values were altered in some way that homogenized the isotopic values of the taxa examined, either in situ or during collection and cleaning (which included boiling in kerosene in the historical early twentieth century collections); or conversely that the larger spread in the enamel δ13C values (Figure 1) is due to variable diagenesis or contamination that affected the carbonate. However, the fact that Δca-co values in modern taxa are predictable from δ13Ccarbonate values, like those from Rancho La Brea (Figure 2A), suggests that neither of these processes is likely and that the origin of the greater variability of δ13Ccarbonate values relative to δ13Ccollagen is biogenic. Furthermore, isotopic difference in δ13Ccollagen values is apparent between other taxa that co-occurred in Rancho La Brea (e.g., bison, horses, and camels as compared to A. dirus, C. latrans, and S. fatalis; Coltrain et al., 2004), again suggesting that bone collagen values were not homogenized due to taphonomic factors; and samples with C:N ratios of 3.1–3.4 (well within the range of 2.9–3.6 indicative of biogenic values; Ambrose et al., 1997) were included in this study. Similarly, δ13Ccarbonate values of extinct taxa from Rancho La Brea are similar in both δ13Ccarbonate values and in variability as other Pleistocene sites in North America (e.g., DeSantis et al., 2009, 2019, 2020; Feranec et al., 2009; Feranec and DeSantis, 2014; Jones and DeSantis, 2017). If the δ13Ccarbonate values were diagenetically altered, we might expect anomalously low oxygen isotope values as oxygen isotopes are more susceptible to post-mortem alteration than δ13C values from structural carbonates from bioapatite (Wang and Cerling, 1994). However, oxygen isotopes values measured in enamel carbonate from fossil material are neither more variable nor anomalously lower than δ18O values from extant C. latrans and P. concolor from southern California (collected during the 20th and 21st centuries). Finally, a large set of published literature demonstrates that tooth enamel is inherently less prone to diagenetic alternation than other tissues like bone apatite, bone collagen, dentin apatite, and dentin collagen (e.g., Wang and Cerling, 1994; Collins et al., 2002; Koch, 2007; Lee-Thorp, 2008; MacFadden et al., 2010). Hence while diagenesis is possible at any fossil locality, several lines of evidence suggest that both types of tissues sampled in this study (i.e., enamel and bone) record biogenic isotope signals and that diagenesis is not a likely driver of the carbonate-collagen discrepancies we observe here.
Physiological similarities in collagen-carbonate δ13C spacing between canids and felids and the need for caution in making trophic level assessments
Δca-co spacing has been previously used to identify differential dietary sources as well as trophic level, however data from this study suggest that such determinations should be made with caution. For example, larger values indicate when protein and carbohydrates come from different sources while smaller values indicate that both protein and carbohydrates are from similar sources. The work of Ambrose et al. (1997) documents that when ancient humans have Δca-co spacing >4.4‰ they consumed C4 carbohydrates and C3 protein, while values under this threshold suggest that both carbohydrates and proteins originate from similar sources (e.g., marine protein). In herbivores, it is thought that consumption of C4 vegetation and rumination contribute to higher Δca-co values; 13C deplete methane produced via fermentation results in 13C enriched CO2, which is subsequently incorporated into the body via blood bicarbonate (Hedges, 2003; Clementz et al., 2009; Codron et al., 2018). Thus, herbivorous mammals exhibit variability in Δca-co values, even when consuming only primary productivity—with values ranging from 3.6 to 14.8‰ in African bovids, likely due to differences in the amounts of 13C depleted methane produced via digestion and subsequently removed from the remaining nutrient pool via expulsion of methane gas by the animal (Codron et al., 2018). Further, it should be noted that all bovids are ruminants, so differences in Δca-co values likely have more to do with disparate diets than differences in digestive physiology—especially when one species (Antidorcas marsupialis) has Δca-co values that range more than 10‰ (from 4.3 to 14.8‰; these wide ranging values may be related to stark differences in protein content in C3 and C4 plants consumed as discussed later). In hypercarnivorous mammals, fats, proteins, and carbohydrates (minimal to no consumption) should largely come from the same source, so that larger Δca-co spacing would suggest individuals with more plant biomass in their diets (Clementz et al., 2009; Bocherens et al., 2017). It was presumed that higher lipid consumption by carnivores (especially hypercarnivores) was responsible for lower Δca-co values (Krueger and Sullivan, 1984; Lee-Thorp et al., 1989; O’Connell and Hedges, 2017) and thus Δca-co values would be predictive of trophic level (Clementz et al., 2009; Bocherens et al., 2017). However, the hypercarnivores examined here—including P. concolor, S. fatalis and A. dirus—have average offset values that range from 4.4+/−0.6 in P. concolor to 8.8+/−1.1 in A. dirus (when calculated from enamel carbonate and bone collagen) and are thus unrelated to trophic level (as inferred from δ15Ncollagen values) in Rancho La Brea carnivores and extant carnivores from C3 ecosystems (Supplementary Table S4). Contrary to expectations, extant hypercarnivores from C4 ecosystems exhibit higher (not lower) Δca-co values with higher δ15Ncollagen values (Supplementary Table S4). Data from extant and extinct carnivores here suggest that the C3 or C4 environment the prey are foraging in has an impact on not only the δ13Ccarbonate values, but also the offset between collagen and carbonate when Δca-co is calculated from enamel carbonate and bone collagen (Figure 2)—though this may be related to fat consumption and/or digestive physiology via effects of vegetation on fat accumulation in prey or digestion of those prey in carnivores. Thus, trophic level estimations may be more nuanced and not directly comparable between disparate ecosystems.
Codron et al. (2018) documented a positive relationship between δ13Ccarbonate and Δca-co values in herbivores, combining teeth and bones into one data set and focusing on the relationship between δ13Ccollagen and Δca-co values (R2 = 0.09, p < 0.0001). Our recalculation of their published data also yields significant (and stronger) relationships between δ13Ccarbonate and Δca-co values (R2 = 0.34, R = 0.58, p < 0.0001 when calculated via enamel carbonate and dentin collagen in teeth; R2 = 0.29, R = 0.54, p < 0.0001 when calculated via bone carbonate and bone collagen; and R2 = 0.35, R = 0.59, p < 0.0001 when combined), though these results are weaker than those observed in carnivores (Supplementary Table S3). Within trophic-level difference in herbivores were attributed to physiology or environmental factors, to differences in methane production that result from grass vs. browse consumption, and to differences between ruminants and hindgut fermenters (Cerling and Harris, 1999; Clauss et al., 2020). However, as we noted with the springbok example generated from data by Codron et al. (2018)—its ‘environment’ (i.e., the composition of its diet, as browse or grass) is likely the driving factor of Δca-co values in this bovid as all members of the species (Antidorcas marsupialis) share the same physiology.
Differences in canid and felid physiology are not likely the primary cause of Δca-co variability. Canid and felid Δca-co values are indistinguishable when feeding in similar environments (e.g., individuals of both P. concolor and C. latrans with similar δ13Ccarbonate values have similar Δca-co values in C3 ecosystems, and the same is true for P. leo and L. pictus in C4 ecosystems) but do vary with δ13Ccarbonate values and both within and between C3 or C4 dominated environments. The fact that S. fatalis Δca-co values are significantly lower than both canids at Rancho La Brea may stem from consuming prey that foraged in disparate environments (based on δ13Ccarbonate values). In contrast, the Δca-co values of the modern felid P. concolor and canid C. latrans that consume prey that forage in similar environments (as inferred from indistinguishable δ13Ccarbonate values, p = 0.964) are indistinguishable from each other (p= 0.762). Thus, it does not appear that differences in canid and felid physiology are the primary cause of Δca-co variability (see Figure 2). If physiological differences between canids and felids were a primary driver of Δca-co variability, we would expect similar δ13Ccarbonate values to yield disparate Δca-co values in canids and felids, especially when feeding on similar prey. Accordingly, these data (Figures 2, 4) can help us rule out physiological differences between canids and felids as the primary driver. It therefore appears that the foraging habitats of prey have a substantial influence on Δca-co values in carnivores, as documented here, and in herbivores as documented by Murphy et al. (2007) and Codron et al. (2018).
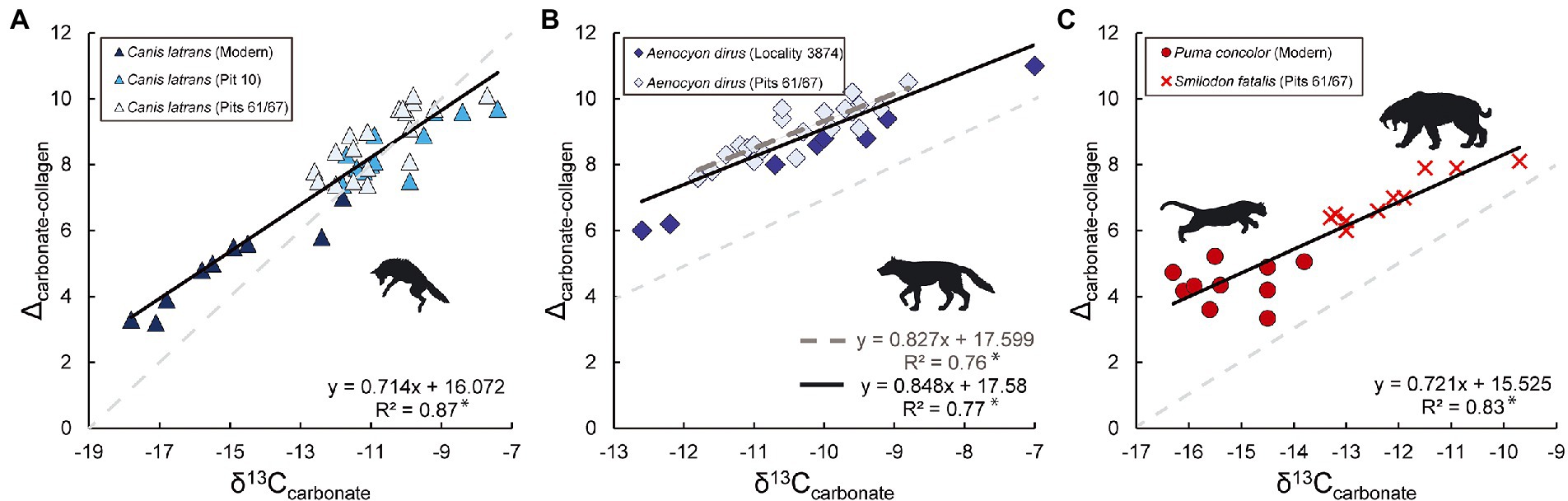
Figure 4. Relationships between δ13Ccarbonate values and Δca-co values for North American canid and felid specimens examined. Relationships between δ13Ccarbonate values and Δca-co values and in Canis latrans (A), Aenocyon dirus (B), and the felids (C; both Puma concolor and Smildon fatalis). Black lines and subsequent regression equations and R2 values reflect the inclusion of all data per taxon. The dark-gray dashed line in panel B denotes the regression line and equations specific to A. dirus based on δ13Ccollagen from bone collagen, while the solid black line includes δ13Ccollagen data from tooth dentin from A. dirus from Locality 3,874. A light-gray dashed-line with a slope of 1 is present in the background of each panel for reference. *denotes significant relationships (p < 0.05).
Tissue and environmental/dietary drivers of collagen-carbonate δ13C spacing
Tissue specific differences, including processes governing how macronutrients such as amino acids and fats (including types and lengths of fatty acids) are incorporated into tooth enamel and bone collagen, may contribute to differences between δ13Ccarbonate and δ13Ccollagen and Δca-co values. Earlier work by Ambrose and Norr (1993) established that δ13Ccarbonate values reflect whole diet (carbohydrates, proteins, and fats), while δ13Ccollagen reflects protein consumption. In carnivores, especially hypercarnivores, it is expected that carbohydrates would be a minor component of their diet and that the ‘whole diet’ most likely reflects proteins and fats, in contrast to primarily protein being routed into bone collagen (Ambrose and Norr, 1993). Further, as fat is depleted in 13C compared to either protein or carbohydrates (Ramsay and Hobson, 1991), one would expect δ13Ccarbonate values to be more 13C depleted when consuming prey with a higher fat content (all else being equal; though research in this area is needed). Fats are 13C depleted compared to muscle to an astonishing degree, differing by as much as 7–8‰ in polar bears and ringed seals, with muscle and fat being even more 13C depleted than bone collagen (Tieszen et al., 1983; Ramsay and Hobson, 1991). The 13C depleted nature of fat is the primary reason why carnivores were thought to have lower Δca-co values (due to the consumption of 13C depleted fat relative to other sources (Lee-Thorp et al., 1989; Clementz et al., 2009). However, an observation of Ambrose and Norr (1993) is often overlooked; when δ13C values of protein are higher than δ13C values of whole diet (regardless of the amount of protein in the diet; i.e., whether the diet consists of 6% protein or 77% protein) then Δca-co values are low, when δ13C values of protein are lower than δ13C values of whole diet then Δca-co values are high (again, regardless of the amount of protein in the diet, i.e., whether the diet consists of 6, 25, or 76% protein). Monoisotopic diets (where protein and whole diet are similar) yield intermediate Δca-co values (i.e., 5.7+/−0.4‰, as compared to a range of 1.2 to 11.3‰; Ambrose and Norr, 1993). Thus, the isotopic composition of carbohydrates and fat relative to protein can influence Δca-co values, and Δca-co values are far from predictable based on trophic level or the amount of protein in one’s diet, alone.
Observed larger offsets in Δca-co values in carnivores could stem from either increased consumption of more 13C enriched carbohydrates, the consumption of leaner prey, and/or different amounts of methane production. One possibility is that the canids consume more terrestrial resources that are not meat, and the higher Δca-co values are a result of more non-meat sources. Alternatively, even limited non-protein sources with higher Δca-co values (including fat) could result in higher Δca-co values. Ambrose and Norr (1993) demonstrated that large Δca-co values occur (i.e., Δca-co values of 10.8+/−0.38, 10.8+/−0.36, and 11.3+/−0.40) when rats were fed protein that was significantly isotopically lighter than whole diet values (by ~10‰); in contrast, much smaller Δca-co values occur (i.e., Δca-co values of 1.2+/−0.10, 2.1+/ 0.24) when rats were fed protein that was isotopically heavier than whole diet values. As the canids have the highest Δca-co values, as compared to the felids (from North America), increased C4 whole diet values (which can include protein, carbohydrates, and fats) in canids could also cause this pattern. Aenocyon dirus has been interpreted as a hypercarnivore (Van Valkenburgh, 1991), making the source of the non-protein components less likely to be terrestrial plants and nuts, though even a small amount of supplemental foods that are isotopically heavier could drive this pattern (based on data from Ambrose and Norr, 1993) in addition to δ15Ncollagen values providing only a ‘minimum estimate’ of trophic level (per Bocherens, 2015). Alternatively, the consumption of fat in prey from more open grassland ecosystems could also result in larger Δca-co values that correlate with δ13Ccarbonate values (per the discussion of Ambrose and Norr, 1993, above). Literature from the livestock industry demonstrates that free-ranging elk and bison can yield disparate fat and fatty acid compositions (Rule et al., 2002; e.g., elk have higher n-6 fatty acids than bison or beef cattle), which can have downstream impacts on the isotopic values observed in predators. Further, carnivores are known to increase fat oxidation when consuming a high-fat diet (Lester et al., 1999), which may also influence resulting Δca-co values, though this has not yet been documented. While methane production is more pronounced in herbivores than carnivores (and most pronounced in grass-rich diets; Cerling and Harris, 1999), methane production could also play a role in carnivores if greater methane production occurs when digesting leaner prey (this could also yield higher Δca-co values in carnivores that correlate with the foraging habitat of prey); however, this is not well understood.
Environmental differences, including water deficits may also contribute to isotopic offsets, as suggested by Murphy et al. (2007). Water deficits are positively correlated with Δca-co values in kangaroos (Murphy et al., 2007); thus, as it gets wetter Δca-co values decrease. While plants need adequate water to grow (zero rainfall results in no growth), excess rainfall beyond a certain threshold can reduce the nutritional quality of foliage (increased rainfall results in increased growth, and subsequently lower nitrogen per unit biomass, which is less nutritious; Austin and Vitousek, 1998). Similar effects are observed when growing plants under higher atmospheric CO2 conditions. Plants grown under higher atmospheric CO2 levels (which also have lower δ13C values when grown in growth chambers) also yield more growth (structural carbon, and often associated tissues like lignin and cellulose) while per unit biomass is subsequently less nutritious (nitrogen content is lower; Conroy, 1992, Barbehenn et al., 2004). All things being equal, herbivores that consume plants with relatively lower nitrogen/protein content may utilize protein differently and have different amounts of fat stores that are subsequently transferred to predators. More work is needed to clarify if and how environmental factors (e.g., precipitation, atmospheric CO2) play a role in affecting herbivore and carnivore Δca-co values.
Future research directions
It is not yet clear why Δca-co and δ13Ccarbonate from bone apatite are unrelated in African carnivores collectively (when combining canids and felids), both here and in Codron et al. (2018), in contrast to enamel apatite. However, there is clear evidence that bone apatite and enamel apatite tissues are not equal and vary quite dramatically in their mineralogy and crystal size (Wopenka and Pasteris, 2005). Bone apatite crystals typically lack the hydroxyl ion (OH−) and are about 10 to 100 times smaller than in enamel, both of which can affect carbonate concentration (with bone apatite having higher carbonate concentration and thus also greater solubility; Wopenka and Pasteris, 2005). Therefore, bone is well suited for being resorbed/re-precipitated and interacting with organic material, while enamel is well suited to resisting dissolution (Wopenka and Pasteris, 2005). While herbivores do exhibit positive relationships between δ13Ccarbonate and Δca-co in both bone apatite and enamel apatite, bone apatite has significantly lower Δca-co values and δ13Ccarbonate values than enamel (p < 0.0001, based on the analysis of data from Codron et al., 2018; mean Δca-co in bone and enamel is 6.7+/-1.4‰ and 9.3+/-2.1‰, respectively; mean δ13Ccarbonate values of-8.5+/-6.5‰, and-5.1+/-6.2‰, respectively). Further, our analysis of African carnivores also demonstrates no relationship between δ13Ccarbonate in bone and Δca-co and no relationship between δ13Ccarbonate in dentin and Δca-co when calculated from dentin carbonate and dentin collagen (Supplementary Table S3). The lack of a relationship between dentin δ13Ccarbonate and dentin δ13Ccollagen, similar to bone yet different from enamel, indicates that while differences in tissue routing may be at play, they likely do not stem from differences in routing due to ontogeny (e.g., using more amino acids for bone growth while young but not while older). Instead, these differences may have more to do with differences in the inorganic content of enamel versus both dentin and bone (and dentin vs. bone) and/or the mineralogy of these tissues (Wang and Cerling, 1994; Wopenka and Pasteris, 2005). Further, wolves (Canis lupus) document a significant positive relationship between δ13Ccarbonate in bone and Δca-co (based on data from Clementz et al., 2009), as do African wild dogs noted here, but in contrast to African lions (Supplementary Table S3). Thus, future work is needed to examine mechanisms behind these tissue specific discrepancies in Δca-co values in carnivores, including differences between specific taxa.
More work is also needed to determine the precise mechanism responsible for the correlation between increased Δca-co values and higher δ13Ccarbonate values, and why this pattern is not apparent in bone apatite and dentin apatite of African carnivores (Codron et al., 2018, and noted here). Further, a re-evaluation of the potential drivers of relationships between herbivore Δca-co and δ13Ccarbonate values may be necessary. Codron et al. (2018) outlined two potential drivers of the positive relationship between Δca-co values and δ13Ccarbonate values in herbivores: environmental differences in food sources; and physiological differences between hindgut fermenters and ruminants. As carnivores did not exhibit the above relationship in their data set (which was primarily composed of bone and not teeth), it was assumed that environmental factors were not at play, as it was expected that environmental influences would similarly affect relationships between both herbivore and carnivore Δca-co values and δ13Ccarbonate values. Considering data presented here, carnivores clearly demonstrate a significant positive relationship between Δca-co values and δ13Ccarbonate values in tooth enamel, but not bone apatite (weakly in some; see Clementz et al., 2009). Thus, macronutrient routing in these disparate tissues (biochemically and structurally) may also play a role. These drivers are not mutually exclusive and may both contribute to the isotopic disparities outlined here. Further, we clearly document that carnivores in both C3 and C4 ecosystems demonstrate positive relationships between Δca-co and δ13Ccarbonate values (Figure 2), yet the reasons for ecosystem-associated differences between these lines and their intercepts is unclear—though may stem from differences in rainfall and/or CO2 over time (and effects on protein and fat content in plants and prey). Thus, until the precise mechanisms driving the highly predictable Δca-co values are identified, the examination of multiple tissues and isotopes may reveal a more complete understanding of the dietary ecology of both extinct and extant mammals.
Advances and challenges of relevance to paleobiology and archeology
The Δca-co spacing in extinct and extant carnivores is predictable based on δ13Ccarbonate values—indicating that the foraging behavior of prey consumed by predators is the best predictor of Δca-co spacing. This positive relationship negates the idea that within trophic-level Δca-co spacing is constant in carnivores and calls into question our ability to interpret degrees of carnivory, omnivory, or herbivory from Δca-co values, alone. In the case of carnivores, felids and canids have similar Δca-co values when consuming similar prey today (in both C3 and C4 environments), while felids and canids only differ in the past when consuming prey that foraged in different habitats. Thus, physiological differences between canids and felids do not appear to be drivers of Δca-co spacing. While either ontogenetic differences in diet or diagenesis have been invoked as potential reasons for differences in δ13Ccarbonate and δ13Ccollagen values, neither of these hypotheses have much support. These data allow us to rule out many hypotheses and indicates that Δca-co spacing is likely driven by the foraging habitat of prey, environmental influences, and/or tissue specific differences that affect how macronutrients are allocated and incorporated into mineralized tissues.
More work is needed to better understand the drivers of increased Δca-co spacing in individuals with higher δ13Ccarbonate values and why these relationships are weaker or absent when comparing certain tissues. Further, not all tissues can be treated similarly. For example, bone apatite and enamel apatite were combined in Codron et al. (2018) as δ13C values were similar; however, the resulting Δca-co values are significantly higher when enamel carbonate and bone collagen were used to calculate Δca-co (p < 0.0001, y-intercepts/mean differences of 2.6‰; Figure 3). While sampling from multiple tissues from the same specimens can be costly and potentially more destructive, more work that examines these relationships in different ecosystems and through time is necessary to better understand if and how a taxon’s habitat contributes to nutrient routing to different tissues.
Our understanding of Rancho La Brea carnivores has benefited from sampling both enamel and bone collagen. Isotopes from different tissues are not “right” or “wrong” but rather provide different insights into the dietary ecology of carnivores. Nitrogen isotope ratios demonstrate that C. latrans likely consumed less meat than either A. dirus or S. fatalis. Stable carbon isotope values measured in bone collagen from Coltrain et al. (2004), Fuller et al. (2020), and here indicate that the protein component of Rancho La Brea carnivore diets are similar, while carbon isotopes ratios from carbonate enamel indicate stark differences in the whole diet of S. fatalis as compared to A. dirus (DeSantis et al., 2019, 2020, and here) and C. latrans (at Pits 61/67). Differences in the stable carbon isotope signatures from whole diet indicate that A. dirus and S. fatalis are not consuming similar proportions of the same prey species and are instead consuming prey with disparate isotopic signatures—suggesting that these prey may forage in more open grasslands and more wooded areas, respectively; and/or may exhibit differences in fat content which can influence whole diet. As C3 grasses have higher amounts of protein than C4 grasses (Barbehenn et al., 2004); protein in prey-species may be biased toward C3 protein sources, which may be responsible for similar carbon isotope values of bone collagen in herbivores and carnivores. The dominance of protein from C3 plants can be further amplified in drier ecosystems, where C3 plants can have even more protein per unit biomass than in higher rainfall regions (Austin and Vitousek, 1998). Fat is an important component of hypercarnivore diets (e.g., polar bears; Ramsay and Hobson, 1991), and many hypercarnivores preferentially consume fat (Nowak and Walker, 1999). However, the isotopic source of fat is primarily reflected in the carbonate portion of tooth enamel, dentin, or bone, but not in collagen (Ambrose and Norr, 1993). Thus, whole diet differences are apparent between S. fatalis and A. dirus (DeSantis et al., 2019, 2020, and here), and C. latrans from Pits 61/67. Protein component of one’s diet does not necessarily equal prey source, and we argue here that whole diet indicators (e.g., carbonate) are necessary to elucidating diets of extinct and extant carnivores. Our work here suggests potential drivers of the differences between stable carbon isotopes in enamel and collagen in Rancho La Brea taxa that include tissue and/or environmental differences (while challenging others including trophic level, ontogenetic dietary differences, physiological differences between canids and felids, and diagenesis), and outlines areas of future research that can bring further clarity to our understanding of the dietary ecology of predators and prey, today and in the past.
Data availability statement
The original contributions presented in the study are included in the article/Supplementary material, further inquiries can be directed to the corresponding author.
Ethics statement
Ethical review and approval was not required for the animal study because all of the animals studied were from museum collections. They were killed previously and not for the purpose of this study.
Author contributions
LD and RF conceptualized the study. All co-authors contributed to study design. LD, JS, JC, RF, and GT collected, prepared, and produced specimen/isotope data. LD analyzed stable isotope data. LD wrote the paper with significant editorial input from RF, JS, and JH, and additional feedback from all co-authors. All authors contributed to the article and approved the submitted version.
Funding
This work was supported by the National Science Foundation (1757545, 1758110, 1758116, 1758117, 1757236, and 1758108), Vanderbilt University, and the NY State Museum.
Acknowledgments
We thank L. Chiappe, P. Collins, P. Holroyd, E. Holt, and K. Fahy for access to materials, KF-D for discussions about stable isotopes and Rancho La Brea, J. Curtis for isotopic analysis, J. Olsson for the shadow drawings in Figures 1, 4, S. DeSantis for assistance with Figures 2, 4, and D. Fox, H. Bocherens, V. M. Bravo-Cuevas and additional reviewers for detailed feedback on earlier versions of this manuscript.
Conflict of interest
The authors declare that the research was conducted in the absence of any commercial or financial relationships that could be construed as a potential conflict of interest.
Publisher’s note
All claims expressed in this article are solely those of the authors and do not necessarily represent those of their affiliated organizations, or those of the publisher, the editors and the reviewers. Any product that may be evaluated in this article, or claim that may be made by its manufacturer, is not guaranteed or endorsed by the publisher.
Supplementary material
The Supplementary material for this article can be found online at: https://www.frontiersin.org/articles/10.3389/fevo.2022.1031383/full#supplementary-material
SUPPLEMENTARY TABLE S1 | Descriptive statistics of stable carbon and nitrogen isotope ratios from bone collagen and enamel carbonate of all taxa examined from North America.
SUPPLEMENTARY TABLE S2 | Descriptive statistics of stable carbon and nitrogen isotope ratios from bone, dentin, and enamel in African carnivores.
SUPPLEMENTARY TABLE S3 | Descriptive statistics of differences between carbon isotope values in carbonate and collagen (carbonate-collagen, Δca-co) in all North American and African carnivores examined in this study.
SUPPLEMENTARY TABLE S4 | Descriptive statistics of relationships between isotope values in carbonate and collagen and offset values in North American and African carnivores.
SUPPLEMENTARY TABLE S5 | Descriptive statistics of relationships between isotope ratios in carbonate and collagen from enamel, dentin, and bone in African carnivores from C4 ecosystems (n = 17).
SUPPLEMENTAL TABLE 1 | Stable isotope values and associated calculations for all individual samples (modern and fossil) from Rancho La Brea, southern California, and throughout Africa.
SUPPLEMENTAL TABLE 2 | Stable isotope data and associated calculations for all tissues (enamel, dentin, bone) from individual samples (modern) from Africa, housed in the American Museum of Natural History (AMNH).
References
Ambrose, S. H., Butler, B. M., Hanson, D. B., Hunter-Anderson, R. L., and Krueger, H. W. (1997). Stable isotopic analysis of human diet in the Marianas archipelago, Western Pacific. Am. J. Phys. Anthropol. 104, 343–361. doi: 10.1002/(SICI)1096-8644(199711)104:3<343::AID-AJPA5>3.0.CO;2-W
Ambrose, S. H., and Norr, L. (1993). “Experimental evidence for the relationship of the carbon isotope ratios of whole diet and dietary protein to those of bone collagen and carbonate,” in Prehistoric human bone: Archaeology at the molecular level. eds. J. B. Lambert and G. Grupe (Heidelberg, German: Springer), 1–37.
Auerswald, K., Wittmer, M. H. O. M., Zazzo, A., Schaufele, R., and Schnyder, H. (2010). Biases in the analyses if stable isotope discrimination in food webs. J. Appl. Ecol. 47, 936–941. doi: 10.1111/j.1365-2664.2009.01764.x
Austin, A. T., and Vitousek, P. M. (1998). Nutrient dynamics on a precipitation gradient in Hawai'i. Oecologia 113, 519–529. doi: 10.1007/s004420050405
Barbehenn, R. V., Chen, Z., Karowe, D. N., and Spickard, A. (2004). C3 grasses have higher nutritional quality than C4 grasses under ambient and elevated atmospheric CO2. Glob. Chang. Biol. 10, 1565–1575. doi: 10.1111/j.1365-2486.2004.00833.x
Barnosky, A. D., Koch, P. L., Feranec, R. S., Wing, S. L., and Shabel, A. B. (2004). Assessing the causes of late Pleistocene extinctions on the continents. Science 306, 70–75. doi: 10.1126/science.1101476
Bekoff, M., and Wells, M. C. (1982). Behavioral ecology of coyotes: social organization, rearing patterns, space use, and resource defense. Ethology 60, 281–305.
Bocherens, H. (2015). Isotopic tracking of large carnivore palaeoecology in the mammoth steppe. Quat. Sci. Rev. 117, 42–71. doi: 10.1016/j.quascirev.2015.03.018
Bocherens, H., Cotte, M., Bonini, R. A., Straccia, P., Scian, D., Soibelzon, L., et al. (2017). Isotopic insight on paleodiet of extinct Pleistocene megafaunal Xenarthrans from Argentina. Gondwana Res. 48, 7–14. doi: 10.1016/j.gr.2017.04.003
Bocherens, H., Fizet, M., and Mariotti, A. (1994). Diet, physiology and ecology of fossil mammals as inferred from stable carbon and nitrogen isotope biogeochemistry: implications for Pleistocene bears. Palaeogeogr. Palaeoclimatol. Palaeoecol. 107, 213–225. doi: 10.1016/0031-0182(94)90095-7
Carbone, C., Maddox, T., Funston, P. J., Mills, M. G., Grether, G. F., and Van Valkenburgh, B. (2009). Parallels between playbacks and Pleistocene tar seeps suggest sociality in an extinct sabretooth cat, Smilodon. Biol. Lett. 5, 81–85. doi: 10.1098/rsbl.2008.0526
Caut, S., Angulo, E., Courchamp, F., and Figuerola, J. (2010). Trophic experiments to estimate isotope discrimination factors. J. Appl. Ecol. 47, 948–954. doi: 10.1111/j.1365-2664.2010.01832.x
Cerling, T. E., and Harris, J. M. (1999). Carbon isotope fractionation between diet and bioapatite in ungulate mammals and implications for ecological and paleoecological studies. Oecologia 120, 347–363. doi: 10.1007/s004420050868
Cerling, T. E., Harris, J. M., MacFadden, B. J., Leakey, M. G., Quade, J., Eisenmann, V., et al. (1997). Global vegetation change through the Miocene/Pliocene boundary. Nature 389, 153–158. doi: 10.1038/38229
Clauss, M., Dittmann, M. T., Vendl, C., Hagen, K. B., Frei, S., Ortmann, S., et al. (2020). Comparative methane production in mammalian herbivores. Animal 14, s113–s123. doi: 10.1017/S1751731119003161
Clementz, M. T., Fox-Dobbs, K., Wheatley, P. V., Koch, P. L., and Doak, D. F. (2009). Revisiting old bones: coupled carbon isotope analysis of bioapatite and collagen as an ecological and palaeoecological tool. Geol. J. 44, 605–620. doi: 10.1002/gj.1173
Clementz, M. T., Koch, P. L., and Beck, C. A. (2007). Diet induced differences in carbon isotope fractionation between sirenians and terrestrial ungulates. Mar. Biol. 151, 1773–1784. doi: 10.1007/s00227-007-0616-1
Codron, D., Clauss, M., Codron, J., and Tütken, T. (2018). Within trophic level shifts in collagen–carbonate stable carbon isotope spacing are propagated by diet and digestive physiology in large mammal herbivores. Ecol. Evol. 8, 3983–3995. doi: 10.1002/ece3.3786
Collins, M. J., Nielsen-Marsh, C. M., Hiller, J., Smith, C. I., Roberts, J. P., Prigodich, R. V., et al. (2002). The survival of organic matter in bone: a review. Archaeometry 44, 383–394. doi: 10.1111/1475-4754.t01-1-00071
Coltrain, J. B., Harris, J. M., Cerling, T. E., Ehleringer, J. R., Dearing, M.-D., Ward, J., et al. (2004). Rancho La Brea stable isotope biogeochemistry and its implications for the paleoecology of the late Pleistocene coastal southern California. Palaeogeogr. Palaeoclimatol. Palaeoecol. 205, 199–219. doi: 10.1016/j.palaeo.2003.12.008
Conroy, J. P. (1992). Influence of elevated atmospheric CO2 concentrations on plant nutrition. Aust. J. Bot. 40, 445–456. doi: 10.1071/BT9920445
Coplen, T. B. (1994). Reporting of stable hydrogen carbon and oxygen isotopic abundances. Pure Appl. Chem. 66, 273–276. doi: 10.1351/pac199466020273
DeNiro, M. J., and Epstein, S. (1978). Influence of diet on the distribution of carbon isotopes in animals. Geochim. Cosmochim. Acta 42, 495–506. doi: 10.1016/0016-7037(78)90199-0
DeSantis, L. R. G., Crites, J. M., Feranec, R. S., Fox-Dobbs, K., Farrell, A. B., Harris, J. M., et al. (2019). Causes and consequences of Pleistocene megafaunal extinctions as revealed from rancho La Brea mammals. Curr. Biol. 29, 2488–2495.e2. doi: 10.1016/j.cub.2019.06.059
DeSantis, L. R., Feranec, R. S., Fox-Dobbs, K., Harris, J. M., Cerling, T. E., Crites, J. M., Farrell, A. B., and Takeuchi, G. T.. 2020. Reply to Van Valkenburgh, et al. Curr. Biol. 30:R151–R152, doi: 10.1016/j.cub.2020.01.011
DeSantis, L. R. G., Feranec, R. S., and MacFadden, B. J. (2009). Effects of global warming on ancient mammalian communities and their environments. PLoS One 4:e5750. doi: 10.1371/journal.pone.0005750
DeSantis, L. R. G., Schubert, B. W., Scott, J. R., and Ungar, P. S. (2012). Implications of diet for the extinction of saber-toothed cats and American lions. PLoS One 7:e5245. doi: 10.1371/journal.pone.0052453
Elliot, J. P., Cowan, I. M., and Holling, C. S. (1977). Prey capture by the African lion. Can. J. Zool. 55, 1811–1828. doi: 10.1139/z77-235
Feranec, R. S. (2004). Isotopic evidence of saber-tooth development, growth rate, and diet from the adult canine of Smilodon fatalis from rancho La Brea. Palaeogeogr. Palaeoclimatol. Palaeoecol. 206, 303–310. doi: 10.1016/j.palaeo.2004.01.009
Feranec, R. S., and DeSantis, L. R. G. (2014). Understanding specifics in generalist diets of carnivorans by analyzing stable carbon isotope values in Pleistocene mammals of Florida. Paleobiology 40, 477–493. doi: 10.1666/13055
Feranec, R. S., Hadly, E. A., and Paytan, A. (2009). Stable isotopes reveal seasonal competition for resources between late Pleistocene bison (Bison) and horse (Equus) from rancho La Brea, southern California. Palaeogeogr. Palaeoclimatol. Palaeoecol. 271, 153–160. doi: 10.1016/j.palaeo.2008.10.005
Finucane, B., Agurto, P. M., and Isbell, W. H. (2006). Human and animal diet at Conchopata, Peru: stable isotope evidence for maize agriculture and animal management practices during the middle horizon. J. Archaeol. Sci. 33, 1766–1776. doi: 10.1016/j.jas.2006.03.012
Friedman, I., and O'Neil, J. R. (1977). Compilation of stable isotope fractionation factors of geochemical interest, vol. 440 US Government Printing Office.
Fuller, B. T., Fahrni, S. M., Harris, J. M., Farrell, A. B., Coltrain, J. B., Gerhart, L. M., et al. (2014). Ultrafiltration for asphalt removal from bone collagen for radiocarbon dating and isotopic analysis of Pleistocene fauna at the tar pits of rancho La Brea, Los Angeles, California. Quat. Geochronol. 22, 85–98. doi: 10.1016/j.quageo.2014.03.002
Fuller, B. T., Fuller, J. L., Harris, D. A., and Hedges, R. E. M. (2006). Detection of breastfeeding and weaning in modern human infants with carbon and nitrogen stable isotope ratios. Am. J. Phys. Anthropol. 129, 279–293. doi: 10.1002/ajpa.20249
Fuller, B. T., Southon, J. R., Fahrni, S. M., Farrell, A. B., Takeuchi, G. T., Nehlich, O., et al. (2020). Pleistocene paleoecology and feeding behavior of terrestrial vertebrates recorded in a pre-LGM asphaltic deposit at rancho La Brea, California. Palaeogeogr. Palaeoclimatol. Palaeoecol. 537:109383. doi: 10.1016/j.palaeo.2019.109383
Haas, S. K., Hayssen, B., and Krausman, P. R. (2005). Panthera leo. Mamm. Species 762, 1–11. doi: 10.1644/1545-1410(2005)762[0001:PL]2.0.CO;2
Hedges, R. E. (2003). On bone collagen—apatite-carbonate isotopic relationships. Int. J. Osteoarchaeol. 13, 66–79. doi: 10.1002/oa.660
Hedges, R. E., Clement, J. G., Thomas, C. D., and O’Connell, T. C. (2007). Collagen turnover in the adult femoral mid-shaft: modelled from anthropogenic radiocarbon tracer measurements. Am. J. Phys. Anthropol. 133, 808–816. doi: 10.1002/ajpa.20598
Helfman, P. M., and Bada, J. L. (1976). Aspartic acid racemisation in dentine as a measure of ageing. Nature 262, 279–281. doi: 10.1038/262279b0
Jenkins, S. G., Partridge, S. T., Stephenson, T. R., Farley, S. D., and Robbins, C. T. (2001). Nitrogen and carbon isotope fractionation between mothers, neonates, and nursing offspring. Oecologia 129, 336–341. doi: 10.1007/s004420100755
Jones, D. B., and DeSantis, L. R. G. (2017). Dietary ecology of ungulates from the La Brea tar pits in southern California: a multi-proxy approach. Palaeogeogr. Palaeoclimatol. Palaeoecol. 466, 110–127. doi: 10.1016/j.palaeo.2016.11.019
Keeling, C. D. (1979). The Suess effect: 13Carbon-14Carbon interrelations. Environ. Int. 2, 229–300. doi: 10.1016/0160-4120(79)90005-9
Keeling, C. D., Mook, W. G., and Tans, P. P. (1979). Recent trends in the 13C/12C ratio of atmospheric carbon dioxide. Nature 277, 121–123. doi: 10.1038/277121a0
Kellner, C. M., and Schoeninger, M. J. (2007). A simple carbon isotope model for reconstructing prehistoric human diet. Am. J. Phys. Anthropol. 133, 1112–1127. doi: 10.1002/ajpa.20618
Kendall, C., Eriksen, A. M., Kontopoulos, I., Collins, M. J., and Gordon, T.-W. (2018). Diagenesis of archaeological bone and tooth. Palaeogeogr. Palaeoclimatol. Palaeoecol. 491, 21–37. doi: 10.1016/j.palaeo.2017.11.041
Koch, P. L. (2007). “Isotopic study of the biology of modern and fossil vertebrates,” in Stable isotopes in ecology and environmental science. eds. R. Michener and K. Lajtha. Second ed (Malden, MA: Blackwell), 99–154.
Koch, P. L., Tuross, N., and Fogel, M. L. (1997). The effects of sample treatment and diagenesis on the isotopic integrity of carbonate in biogenic hydroxylapatite. J. Archaeol. Sci. 24, 417–429. doi: 10.1006/jasc.1996.0126
Krueger, H. W., and Sullivan, C. H. (1984). “Models for carbon isotope fractionation between diet and bone,” in Stable isotopes in nutrition. ACS symposium series 258. eds. J. F. Turnland and P. E. Johnson (Washington D.C: American Chemical Society), 205–222.
Lee-Thorp, J. A. (2008). On isotopes and old bones. Archaeometry 50, 925–950. doi: 10.1111/j.1475-4754.2008.00441.x
Lee-Thorp, J. A., Sealy, J. C., and van der Merwe, N. J. (1989). Stable carbon isotope ratio differences between bone collagen and bone apatite, and their relationship to diet. J. Archaeol. Sci. 16, 585–599. doi: 10.1016/0305-4403(89)90024-1
Lester, T., Czarnecki-Maulden, G., and Lewis, D. (1999). Cats increase fatty acid oxidation when isocalorically fed meat-based diets with increasing fat content. Regul. Integr. Physiol. 277, R878–R886. doi: 10.1152/ajpregu.1999.277.3.R878
Levin, N. E., Cerling, T. E., Passey, B. H., Harris, J. M., and Ehleringer, J. R. (2006). A stable isotope aridity index for terrestrial environments. Proc. Natl. Acad. Sci. 103, 11201–11205. doi: 10.1073/pnas.0604719103
MacFadden, B. J., DeSantis, L. R. G., Hochstein, J. L., and Kamenov, G. D. (2010). Physical properties, geochemistry, and diagenesis of xenarthran teeth: prospects for interpreting the paleoecology of extinct species. Palaeogeogr. Palaeoclimatol. Palaeoecol. 291, 180–189. doi: 10.1016/j.palaeo.2010.02.021
Mariotti, A. (1983). Atmospheric nitrogen is a reliable standard for natural 15N measurements. Nature 303, 685–687. doi: 10.1038/303685a0
McHorse, B. K., Orcutt, J. D., and Davis, E. B. (2012). The carnivoran fauna of rancho La Brea: average or aberrant? Palaeogeogr. Palaeoclimatol. Palaeoecol. 329-330, 118–123. doi: 10.1016/j.palaeo.2012.02.022
Meachen, J. A., and Samuels, J. X. (2012). Evolution in coyotes (Canis latrans) in response to the megafaunal extinctions. Proc. Natl. Acad. Sci. U.S.A. 109, 4191–4196. doi: 10.1073/pnas.1113788109
Merriam, J. C., and Stock, C.. 1932. The Felidae of rancho la Brea. No. 422. Carnegie Institute, Washington.
Murphy, B. P., Bowman, D. M. J. S., and Gagan, M. K. (2007). Sources of carbon isotope variation in kangaroo bone collagen and tooth enamel. Geochim. Cosmochim. Acta 71, 3847–3858. doi: 10.1016/j.gca.2007.05.012
Nowak, R. M., and Walker, E. P. (1999). Walker's mammals of the world Baltimore, MD: John Hopkins University Press.
O’Keefe, F. R., Fet, E. V., and Harris, J. M. (2009). Compilation, calibration, and synthesis of faunal and floral radiocarbon dates, Rancho La Brea, California. Cont. Sci. 518, 1–16. doi: 10.5962/p.226783
O’Connell, T. C., and Hedges, R. E. M. (2017). Chicken and egg: testing the carbon isotopic effects of carnivory and herbivory. Archaeometry 59, 302–315. doi: 10.1111/arcm.12253
Passey, B. H., Robinson, T. F., Ayliffe, L. K., Cerling, T. E., Sponheimer, M., Dearing, M. D., et al. (2005). Carbon isotope fractionation between diet, breath CO2, and bioapatite in different mammals. J. Archaeol. Sci. 32, 1459–1470. doi: 10.1016/j.jas.2005.03.015
Ramsay, M. A., and Hobson, K. A. (1991). Polar bears make little use of terrestrial food webs: evidence from stable-carbon isotope analysis. Oecologia 86, 598–600. doi: 10.1007/BF00318328
Richards, M. P., Mays, S., and Fuller, B. T. (2002). Stable carbon and nitrogen isotope values of bone and teeth reflect weaning age at the Medieval Wharram Percy site, Yorkshire, UK. Am. J. Phys. Anthropol. 119, 205–210. doi: 10.1002/ajpa.10124
Rule, D. C., Broughton, K. S., Shellito, S. M., and Maiorano, G. (2002). Comparison of muscle fatty acid profiles and cholesterol concentrations of bison, beef cattle, elk, and chicken. J. Anim. Sci. 80, 1202–1211. doi: 10.2527/2002.8051202x
Samuels, J. X., Meachen, J. A., and Sakai, S. A. (2013). Postcranial morphology and the locomotor habits of living and extinct carnivorans. J. Morphol. 274, 121–146. doi: 10.1002/jmor.20077
Schaller, G. B. 1972. The Serengeti lion, a study of predator-prey relationships. Chicago; University of Chicago Press.
Schoeninger, M. J., DeNiro, M. J., and Tauber, H. (1983). Stable nitrogen isotope ratios of bone collagen reflect marine and terrestrial components of prehistoric human diet. Science 220, 1381–1383. doi: 10.1126/science.6344217
Secord, R., Bloch, J. I., Chester, S. G., Boyer, D. M., Wood, A. R., Wing, S. L., et al. (2012). Evolution of the earliest horses driven by climate change in the Paleocene-Eocene thermal maximum. Science 335, 959–962. doi: 10.1126/science.1213859
Smith, F. A., Elliott Smith, E. A., Villaseñor, A., Tomé, C. P., Lyons, S. K., and Newsome, S. D. (2022). Late Pleistocene megafauna extinction leads to missing pieces of ecological space in a north American mammal community. Proc. Natl. Acad. Sci. 119:e2115015119. doi: 10.1073/pnas.2115015119
Stock, C., and Harris, J. M.. 1992. Rancho La Brea: A record of Pleistocene life in California Los Angeles: Natural History Museum of Los Angeles. Natural History Museum of Los Angeles County, Los Angeles.
Teruel, J. D., Alcolea, A., Hernández, A., and Ruiz, A. J. O. (2015). Comparison of chemical composition of enamel and dentine in human, bovine, porcine and ovine teeth. Arch. Oral Biol. 60, 768–775. doi: 10.1016/j.archoralbio.2015.01.014
Tieszen, L. L., Boutton, T. W., Tesdahl, K. G., and Slade, N. A. (1983). Fractionation and turnover of stable carbon isotopes in animal tissues: implications for δ13C analysis of diet. Oecologia 57, 32–37. doi: 10.1007/BF00379558
Tsutaya, T., and Yoneda, M. (2015). Reconstruction of breastfeeding and weaning practices using stable isotope and trace element analyses: a review. Am. J. Phys. Anthropol. 156, 2–21. doi: 10.1002/ajpa.22657
Tung, T. A., Dillehay, T. D., Feranec, R. S., and DeSantis, L. R. G. (2020). Early specialized maritime and maize economies of the north coast of Peru. Proc. Natl. Acad. Sci. U.S.A. 117, 32308–32319. doi: 10.1073/pnas.2009121117
Tung, T. A., Miller, M., DeSantis, L., Sharp, E. A., and Kelly, J. (2016). “Patterns of violence and diet among children during a time of imperial decline and climate change in the ancient Peruvian Andes,” in The archaeology of food and warfare, eds. E. Amber, M. VanDerwarker and G. D. Wilson (Cham, Switzerland: Springer), 193–228.
Van Valkenburgh, B. (1991). Iterative evolution of hypercarnivory in canids (Mammalia: Carnivora): evolutionary interactions among sympatric predators. Paleobiology 17, 340–362. doi: 10.1017/S0094837300010691
Van Valkenburgh, B., Clementz, M. T., and Ben-David, M. (2020). Problems with inferring a lack of competition between rancho La Brea dire wolves and sabertooth cats based on dental enamel. Curr. Biol. 30, R149–R150. doi: 10.1016/j.cub.2020.01.009
Van Valkenburgh, B., and Hertel, F. (1993). Tough times at La Brea: tooth breakage in large carnivores of the late Pleistocene. Science 261, 456–459. doi: 10.1126/science.261.5120.456
Wang, Y., and Cerling, T. E. (1994). A model of fossil tooth and bone diagenesis: implications for paleodiet reconstruction from stable isotopes. Palaeogeogr. Palaeoclimatol. Palaeoecol. 107, 281–289. doi: 10.1016/0031-0182(94)90100-7
Wopenka, B., and Pasteris, J. D. (2005). A mineralogical perspective on the apatite in bone. Mater. Sci. Eng. C 25, 131–143. doi: 10.1016/j.msec.2005.01.008
Keywords: bone, carbon isotopes, carnivora, carnivores, enamel, nitrogen isotopes, Rancho La Brea
Citation: DeSantis LRG, Feranec RS, Southon J, Cerling TE, Harris J, Binder WJ, Cohen JE, Farrell AB, Lindsey EL, Meachen J, Robin O'Keefe F and Takeuchi GT (2022) On the relationship between collagen- and carbonate-derived carbon isotopes with implications for the inference of carnivore dietary behavior. Front. Ecol. Evol. 10:1031383. doi: 10.3389/fevo.2022.1031383
Edited by:
Florent Rivals, Catalan Institution for Research and Advanced Studies (ICREA), SpainReviewed by:
Hervé Bocherens, University of Tübingen, GermanyRachel E. B. Reid, Virginia Tech, United States
Victor Manuel Bravo-Cuevas, Autonomous University of the State of Hidalgo, Mexico
Copyright © 2022 DeSantis, Feranec, Southon, Cerling, Harris, Binder, Cohen, Farrell, Lindsey, Meachen, O'Keefe and Takeuchi. This is an open-access article distributed under the terms of the Creative Commons Attribution License (CC BY). The use, distribution or reproduction in other forums is permitted, provided the original author(s) and the copyright owner(s) are credited and that the original publication in this journal is cited, in accordance with accepted academic practice. No use, distribution or reproduction is permitted which does not comply with these terms.
*Correspondence: Larisa R. G. DeSantis, bGFyaXNhLmRlc2FudGlzQHZhbmRlcmJpbHQuZWR1