- 1Institut für Biologie, Freie Universität Berlin, Berlin, Germany
- 2Berlin-Brandenburg Institute of Advanced Biodiversity Research (BBIB), Berlin, Germany
An increasing frequency of heat events can affect key organisms related to ecosystem functions. Soil saprobic fungi have important roles in carbon and nutrient cycling in soils, and they are clearly affected by heat events. When tested individually, saprobic soil fungi showed a variety of growth responses to a series of two heat events. However, in nature, these fungi rarely grow alone. Coexistence theory predicts that diversity in the response to stressors can influence the outcome of species interactions and growth. This means that the co-cultivation of different fungi may affect their growth response to heat events. To test if recurring heat events affect fungal growth in small synthetic communities, we evaluated fungi previously known to respond to recurring heat events in experimental small communities composed of two and three species. For the fungi growing in pairs, surprisingly, most of the responses could not be predicted by how the isolates responded individually. In some cases, facilitation or increased competition was observed. For the three fungi growing together, results were also not predicted by the individual or pair responses. Both the heat events and the small communities influenced the growth of the fungi and growth properties emerged from the interactions among isolates and the heat stress. We show that not only do environmental conditions influence fungal interactions and growth rates but also that the co-cultivation of different fungi affects the fungal response to recurring heat events. These results indicate that more complex experimental designs are needed to better understand the effects of recurring heat events and climate change on soil fungi.
1. Introduction
There is a growing interest in advancing our understanding of the role that soil microbial communities play in the Anthropocene (Geisen et al., 2019). The urgent need to advance research and knowledge comes from two directions, as soil microorganisms are both an important subject and object in the dynamics of global change. On one hand, considering soil as a subject, we need to deepen and refine our knowledge of the role of soil microbial communities in mitigating the impacts of global change on human societies and ecological and climatic systems (Hannula and Morriën, 2022). On the other hand, considering soil as an object, we need to better understand how soil communities themselves are affected by global change (Geisen et al., 2019). Our understanding of the risks and resilience of soil microbial communities is still rudimentary and needs to be rapidly refined.
In recent years, an increasing occurrence of extreme weather has been reported around the world, including heat waves (Perkins-Kirkpatrick and Gibson, 2017). The effects of such heat waves on soil saprobic fungi are understudied, despite the important role of soil fungi on the feedback loops between the soil and atmospheric carbon (Hannula and Morriën, 2022). Soil saprobic fungi may be susceptible to abrupt changes in air temperature, especially in top soils, and soil fungal species have been shown to be affected by heat events (Andrade-Linares et al., 2016a; Szymczak et al., 2020).
A high diversity of soil fungi lives in top soils, mainly by decomposing plant and organic material and cycling nutrients (Baldrian et al., 2012; Hannula and Morriën, 2022). These soil fungi rarely live alone, but in complex microbial communities, with many fungal species interacting (Baldrian et al., 2012). Most biotic interactions are context dependent and interactions outcomes may change according to environmental conditions, including temperature (Hiscox et al., 2016; de Oliveira et al., 2020). Although temperature is a very important factor, its effect has been usually tested in laboratory experiments with the temperature at a constant value, while in ecosystems temperature is a dynamic variable, with diurnal and seasonal variations (de Oliveira et al., 2020). Thus, recurring heat events may be an important factor that influences fungal species interactions and their consequent growth rates. Temporal temperature variability in environmental conditions may shift hierarchical interactions toward intransitive interactions, with important consequences for coexistence (Crowther et al., 2012; Hiscox et al., 2016).
Saprobic soil fungi exhibit a variety of responses to recurring heat events (Szymczak et al., 2020). The effects of recurring heat events can be neutral (no evident effect), antagonistic (the first event reduces the effect of the second event, which is also called a stress priming effect), additive (the total effect is the sum of individual events), or synergistic (when the overall effect is bigger than the individual events) (Szymczak et al., 2020). It has been previously shown that the community composition of fungi can be affected by the trade-offs between stress tolerance and competitive (interference) capacities, possibly acting also as a variation dependent coexistence mechanism at the local scale (Maynard et al., 2019). Furthermore, a trade-off between competitive dominance and stress resistance can enable organisms’ coexistence (Haegeman et al., 2014).
It has been previously proposed that microbial priming responses can be modulated in a community context and conversely, differences in priming capacity together with differences in competition-related traits (e.g., growth rate) could affect species coexistence (Rillig et al., 2015; Wesener et al., 2021). Here, we developed this idea further using an experiment, by co-cultivating a collection of fungal species in pairs or triplets and applying temporally variable temperature regimes (recurring heat events), and evaluating their growth responses (Szymczak et al., 2020). We tested how the co-cultivation of different fungi affected the capacity of individual isolates to respond to heat events either by the direct effect of co-cultivation on the priming ability of the focal species or by the effect of heat events on the strength of competition (Rillig et al., 2015; Wesener et al., 2021).
2. Materials and methods
2.1. Fungal strains
In a previous study, 32 soil fungi isolates (isolated from the same soil environment) were tested for their response to recurring heat events (Szymczak et al., 2020). Fungi were isolated from 0 to 10 cm deep soil collected in grassland in Mallnow Lebus, Brandenburg Germany (Andrade-Linares et al., 2016b).
Based on the previous performance of the isolates in monoculture, we selected a subset of six isolates with contrasting responses and combined them as pairs or three species growing on Petri dishes containing potato dextrose agar medium (PDA). We then subjected them to the same recurring heat events as previously used in monocultures (Szymczak et al., 2020).
We selected three isolates that previously did not show priming ability: RLCS06 (Chaetominum anustispirale), RLCS07 (Amphisphaeriaceae sp.), and RLCS16 (Pleurotus sapidus), and three that showed priming ability: RLCS02 (Mortierella elongate), RLCS05 (Fusarium sp.), and RLCS32 (Fusarium oxysporum) (more details about the isolates in Supplementary Figure 1 and Szymczak et al., 2020). For ease of following the results, we refer to the different isolates with a code that combines their priming response (np, for no priming, and p, for priming) with the strain identity information (isolate numbers). Then, we refer to the isolates that previously did not show priming as np06, npo07, and np16 and the isolates that showed priming as p02, p05, and p32. All the fungal isolates were kept active in PDA at 20°C until used in the experiments.
We ran a test of the isolates growing with themselves in pairs or triplets at control temperature (C, constant temperature of 22°C) and the colonies looked very symmetrical and presented very similar growth patterns (Supplementary Figure 2). Consequently, to reduce experimental units, we did not apply the temperature treatment combinations on isolates growing with themselves, but only in the combinations of different isolates, as described in the following text.
2.2. Experiment I (species pairs)
First, the six selected isolates were co-cultivated in pairs in Petri dishes containing PDA and received different heat treatments. The heat treatments were previously established by simulating hypothetical heat waves near the soil surface and using temperatures and duration tested in previous experiments, as described in Szymczak et al. (2020): control (C, constant temperature of 22°C), mild perturbation (M, 35°C for 2 h), severe perturbation (S, 45°C during 1 h), and sequence of the two perturbations (MS, 35°C during 2 h, followed by 45°C during 1 h). The heat treatments were applied in a 2 × 2 fully factorial design, composed of mild perturbation (35°C per 2 h, yes or no) followed by a severe perturbation (45°C per 1 h, yes or no) (Szymczak et al., 2020).
For the experiment set up, the fungal isolates were first grown on PDA at 22°C and handled as previously described (Szymczak et al., 2020). Later, plugs were taken from the edge of the active colonies and transferred to new PDA plates according to the fungal combinations. The plugs were inoculated equidistantly from the border of the plate and between each other. After the inoculation of fungal combinations, six replicated plates for each treatment (fungal pairs and temperatures) were placed in replicate incubators according to the treatments (plates were placed in pairs in three different incubators). This means that the treatments were applied to two technical replicates (agar plates) nested within each of the three experimental units (three incubators). For the experiment I, we tested 15 combinations of fungal isolates with four heat treatments (2 × 2 factorial design) applied to six replicated plates (two agar plates placed/nested in three different incubators), in a total of 15 × 4 × 6 = 360 plates.
After the heat treatments, the fungal combinations were grown at 22°C for 2 weeks. Finally, the diameter of the colonies was measured and the growth rate of each strain was calculated for the incubation period, both for mycelial pairs that did and did not contact during the experiment period (Szymczak et al., 2020). Thus, the experiment design treated fungal interactions as an aggregate of interaction at distance, interference upon contact, and competition for space.
2.3. Experiment II (three species)
Later, the six selected strains described above were inoculated in 20 random combinations of three species in PDA media and submitted to the treatments previously described (the combinations are stated in Figure 3). The experiment had the same design described earlier and followed all the same laboratory steps but co-cultivated three isolates instead of two. For experiment II, we tested 20 combinations of fungal isolates, with four heat treatments and six replicates, in a total of 20 × 4 × 6 = 480 plates. Both experiments summed, 360 plates + 480 plates, resulting in 840 plates.
2.4. Statistical analysis
Analysis followed a previously proposed factorial design considering the factors as mild perturbation (yes or no) and severe perturbation (yes or no) (Hilker et al., 2016; Szymczak et al., 2020). The experimental design included a nested design, where duplicated cultivation plates were nested within three replicated incubators. Accordingly, the factorial design was fitted to a nested design (plates nested to the replicated incubators) in a linear-mixed effects model (lme). The model was fit using the software R (R Core Team, 2013) and the package nlme (Pinheiro and Bates, 2000). Some data did not fit normality (even though we tested a box-cox optimal transformation), thus, the models were analyzed by robust analysis of variance (rANOVA) using the R package Rfit (Hettmansperger and McKean, 2010). The p-values were adjusted by the Benjamini-Hochberg (BH) false discovery rate procedure to reduce the chance of type I error (Benjamini and Hochberg, 1995).
The individual responses of the isolates for the fungal combinations and thermal treatments were analyzed as univariate data (rANOVA), while the reciprocal effect of one isolate on the companion strains was analyzed as multivariate data (bivariate for the pairs and trivariate for the three-species combinations). The bivariate and trivariate data were analyzed by permutational multivariate analysis of variance (perMANOVA) using the R package vegan (Anderson, 2017; Oksanen et al., 2019). We also analyzed the data as a factorial focusing on each isolate and considering in addition to thermal treatments the company strains as a factor, i.e., mild perturbation (yes or no) and severe perturbation (yes or no) and company strain (combinations). This provided general insight into how the focal isolates’ growth rates overall changed when growing in pairs or triplets. For experiment I, we also plotted a chord diagram to indicate when a company strain changed the response of the focal strain compared to the control (according to the rANOVA result).
The combinations of two species were scatter plotted to visualize the effects of the heating treatments on the pair species simultaneously, while the responses of the individual isolates were visualized by box plots. For the combinations with three species, the data were visualized in ternary graphs plotted using the R package ggternary (Hamilton and Ferry, 2018), as also as box plots focusing on individual isolates, to allow a general comparison of the two experiments (pairs or triplets).
3. Results
3.1. Experiment I (species pairs)
The six fungal isolates were first tested in pairs (Figure 1). The effects of the co-cultivation in response to the thermal treatments were either negative (the growth of one or both strains decreased after the heating treatments), neutral (no effect on the growth of the isolates), or facilitative (one or both isolates had increased growth rates after the heat events), depending on the pairs.
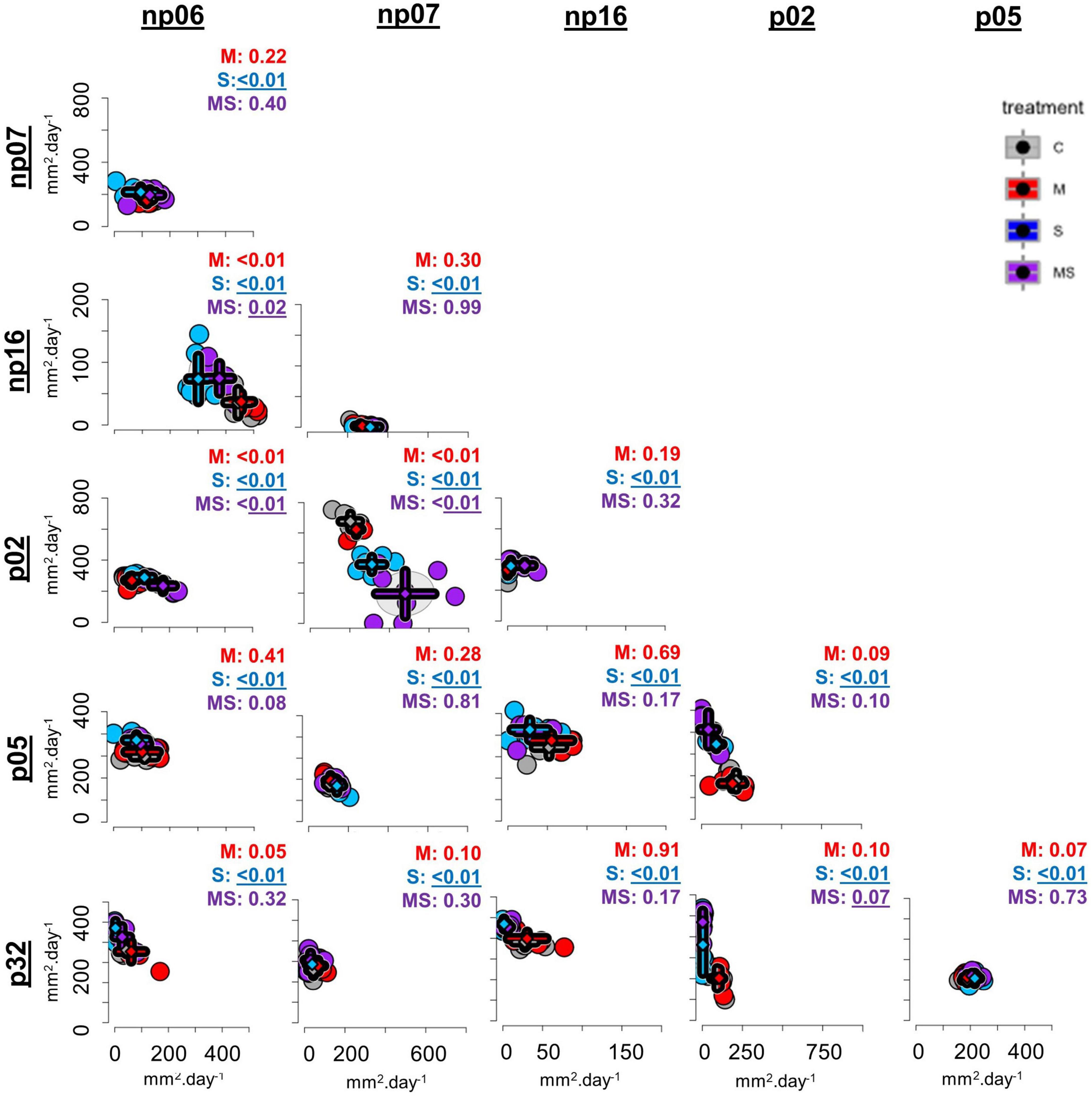
Figure 1. The effects of none (control, C), mild (M), strong (S), and both (MS) heat stress events on fungal isolate growth rates for fungal isolates cultivated in pairs [p-values from permutational multivariate analysis of variance (perMANOVA)]. Each dot represents one petri dish (one replicate for each treatment), while the rhombuses indicate the averages and the error bars the standard deviations for each treatment. The axes were scaled to optimize the comparison across pairs (for comparison of individual pairs refer to Supplementary Figure 2).
Compared to the control of each combination, four pairs had a changed interaction after the mild heating, all 15 combinations responded to the strong heating and three responded simultaneously to the MS heating event (Figure 1, perMANOVA p < 0.05). Observing the scatter plots (Figure 1 and Supplementary Figure 3), the moderate heating event had no evident effect on the co-cultivation growth rate of some isolates (e.g., combination np06-np07). On the other hand, some isolates grew better after the heat event, compared to the control without such a heat event. For example, there was a growth rate shift in the combination np06-np16, since the growth rate of np06 was reduced after the strong and mild-strong heating events in comparison to the control, while the growth rate of np16 increased after the strong and mild-strong heating events in comparison to the control (Figures 1, 2, 3.1). Furthermore, some isolates were severely affected by the strong heat event, resulting in a shift of growth rates in some combinations (Figure 1 and Supplementary Figure 2). For example, the isolate p02 co-cultivated with np07 had a growth rate of around 170 mm2 day–1 in the control, but the rate was reduced to less than 100 mm2 day–1 after the strong heat event. Simultaneously to this reduction, the isolate np07 doubled its growth rate from less than 50 mm2 day–1 in the control to 50–150 mm2 day–1 after the heat treatments (Figures 1, 2, 3.2).
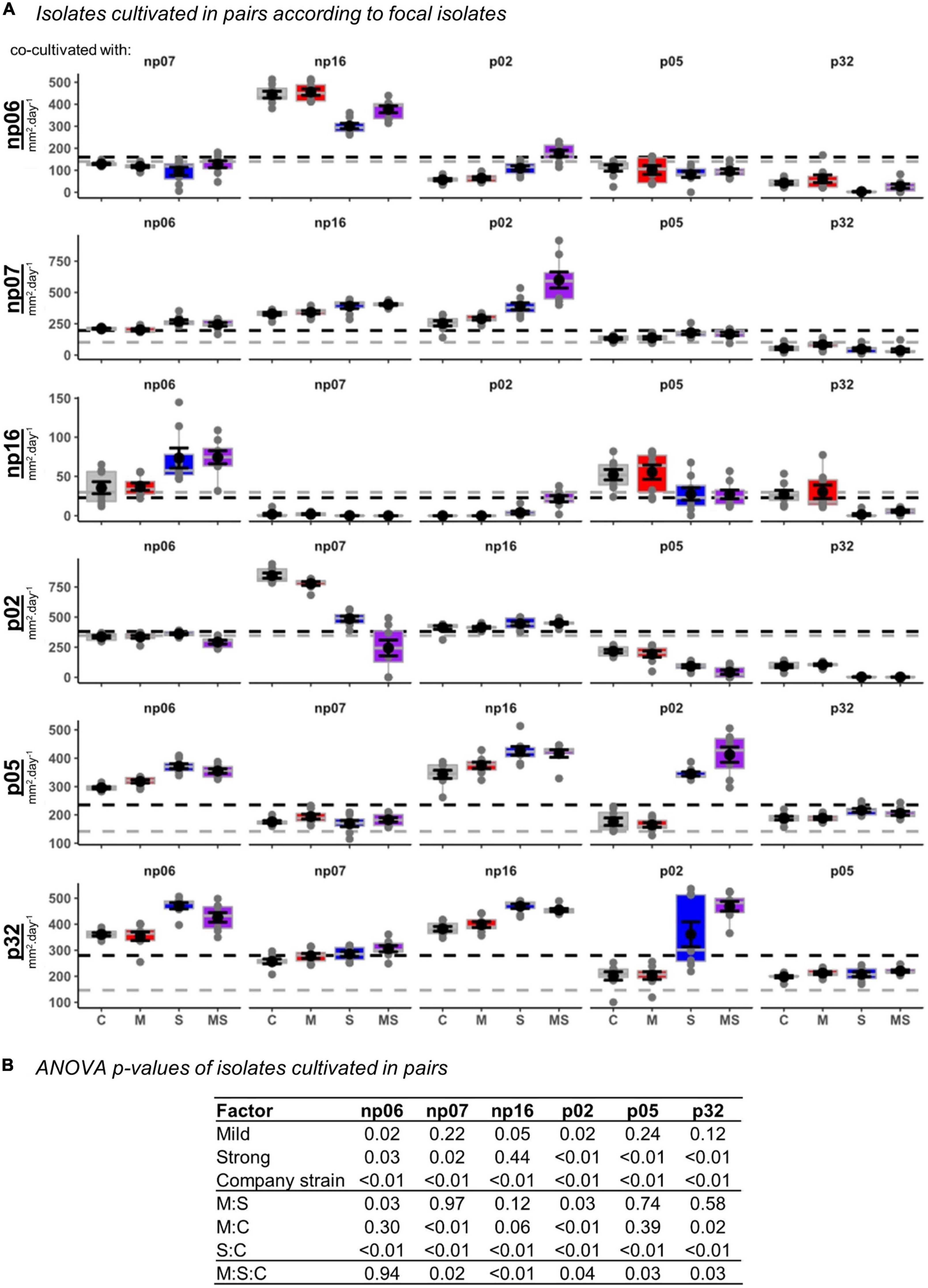
Figure 2. The effects of none (control, C), mild (M), strong (S), and both (MS) heat stress events on fungal isolate growth rates for fungal isolates cultivated in pairs. The panel containing box-plots with average and standard error for each focal isolate according to the pairs (A), and the robust analysis of variance (rANOVA) p-values for the isolates analyzed in a factorial design considering the heat treatments mild (yes or no), strong (yes or no) and company strains combinations (B). For the boxplots, the gray lines are references to the isolates growing alone in control conditions (data from Supplementary Figure 2) and the black lines are the average of all controls for each focal isolate.
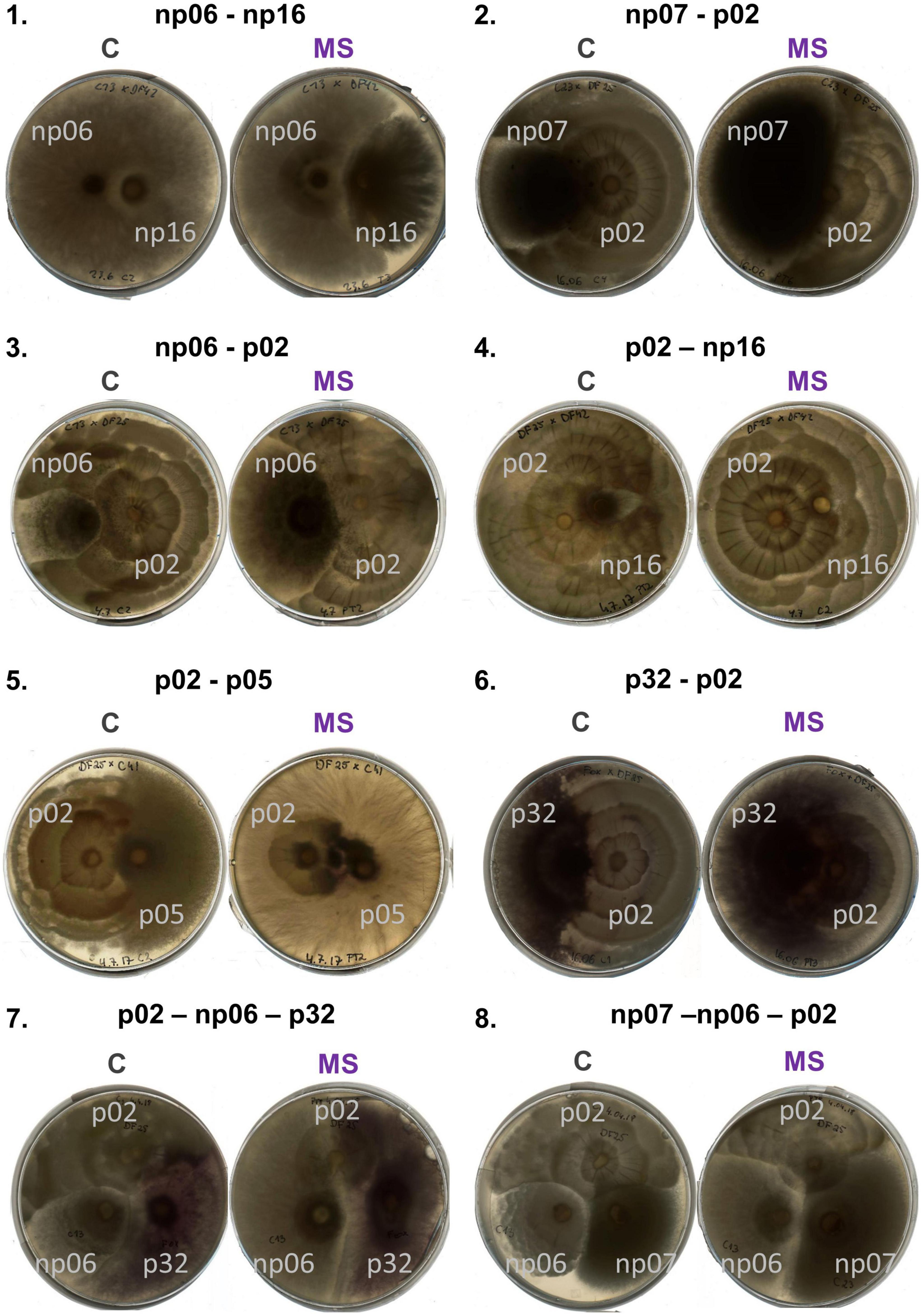
Figure 3. Panel with pictures of plates of isolates discussed in the text grown in pairs (1–6) and triplets (7,8) according to the treatments control (C) and mild strong (MS).
When comparing the isolates individually, these changes were more evident and the growth of some fungi in response to heat events depended on the presence of the accompanying fungi (Figure 1, details in Supplementary Figures 3, 4 and Supplementary Table 1). For example, np06 was not affected by the mild, strong, and mild-strong heat events when this isolate was grown in pairs with the strains np07 or p05; nevertheless, some heat effects were observed when this strain was paired with strains p02, np16, or p32 (Figures 1, 3.3, 3.4). In another example, for p02 paired with np16, isolate p02 had a stable growth independent of the heat treatments. Yet, when paired with isolates p05, np07, or p32 (Figures 1, 3.5), isolate p02 had a strong reduction in growth rates after the strong heat event, compared to its growth in the control.
The positive effect of co-culturing was observed in some cases, e.g., p32 growing in the combinations with np06 and p02 (Figures 1, 3.6), had improved growth after the heat events (mild or strong), compared to the control, which did not occur in any other combination (Figure 1, details in Supplementary Figure 3 and Supplementary Table 1). Surprisingly, isolates that had priming ability in the previous study lost their ability to benefit from it when growing in combinations (Supplementary Table 1). Only the isolate np06, which did not have priming ability in the previous study growing alone (Szymczak et al., 2020), showed priming-like behavior in the present study when paired with isolate np16 (Supplementary Figure 4 and Supplementary Table 1).
Plotting the data for each isolate (Figure 2A) and analyzing also the fungal pair combinations as a factor (Figure 2B), showed that all isolates suffered interference of a company strain, while the thermal effect was variable according to each isolate (Figure 2).
3.2. Experiment II (triplets)
The six fungal isolates were then tested in triplets in 20 random combinations (Figures 4, 5 and Supplementary Figure 5). The heat events changed the growth pattern of the isolates for some combinations. For example, in combination 1, p02-np06-p32, the isolate p02 growth rate in the triplet was largely suppressed by the strong and mild-strong heat event compared to the control (Figures 3.7, 4.1). A similar effect was observed in combination 3, np07-np06-p02 (Figures 3.8, 4.3), however, the suppression was less intense as the example before. In some other combinations, the heat event had no effect on the interaction of the isolates. For example, in combination 14, np07-np06-p05, all the isolates had no change in their growth pattern (Figure 4.14).
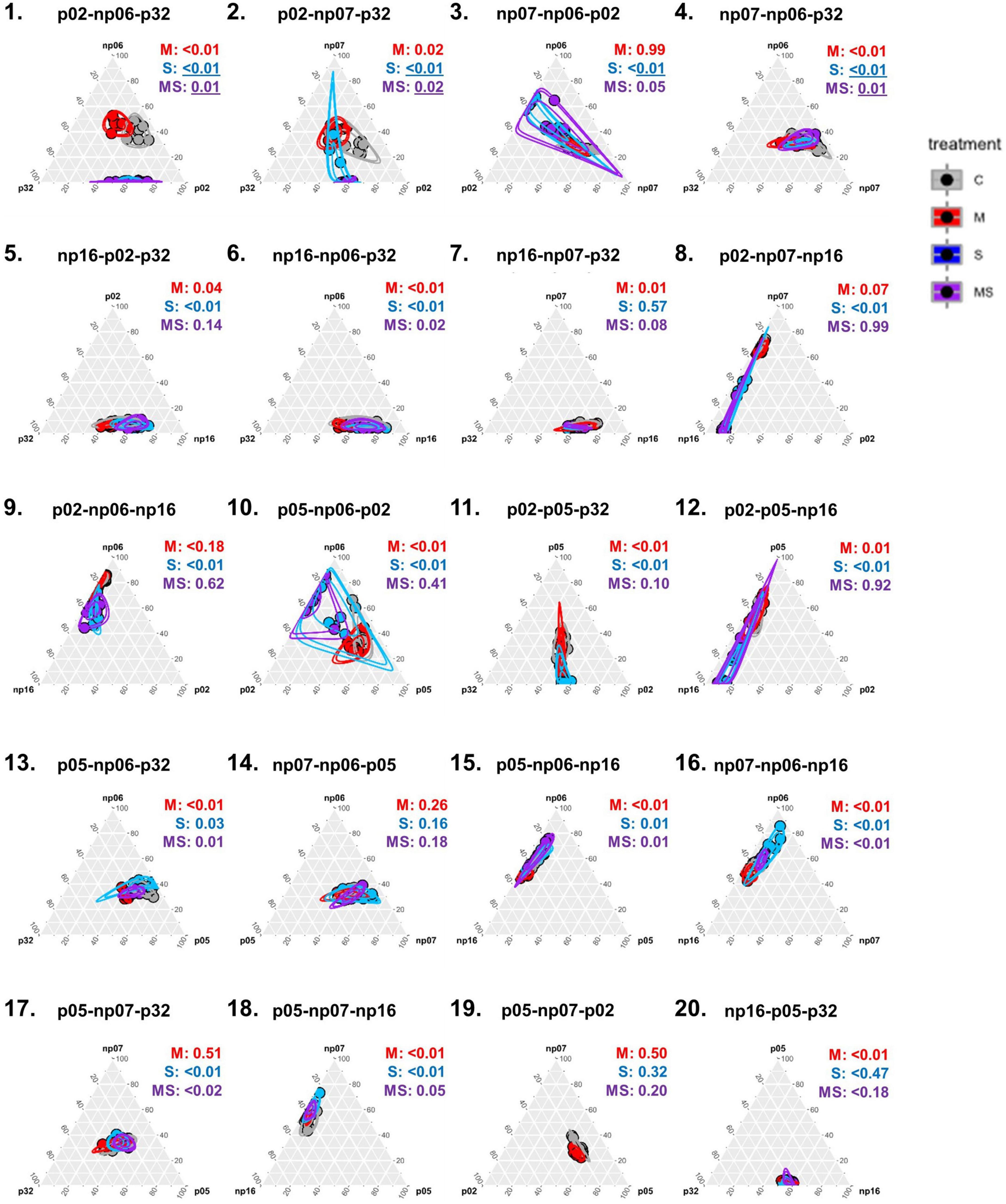
Figure 4. Ternary plots depicting the effects of none (control, C), mild (M), strong (S), and both (MS) heat stress events on fungal isolates cultivated in triplets [p-values from permutational multivariate analysis of variance (perMANOVA)]. Each dot represents one petri dish (one replicate for each treatment) and the circles are the 95% confidence intervals for each treatment. Values were scaled in the percentage of growth of each isolate in a triplet in relation to the maximum growth of that isolate in the full experiment (for information on actual growth rates of each isolate refer to Supplementary Figure 4).
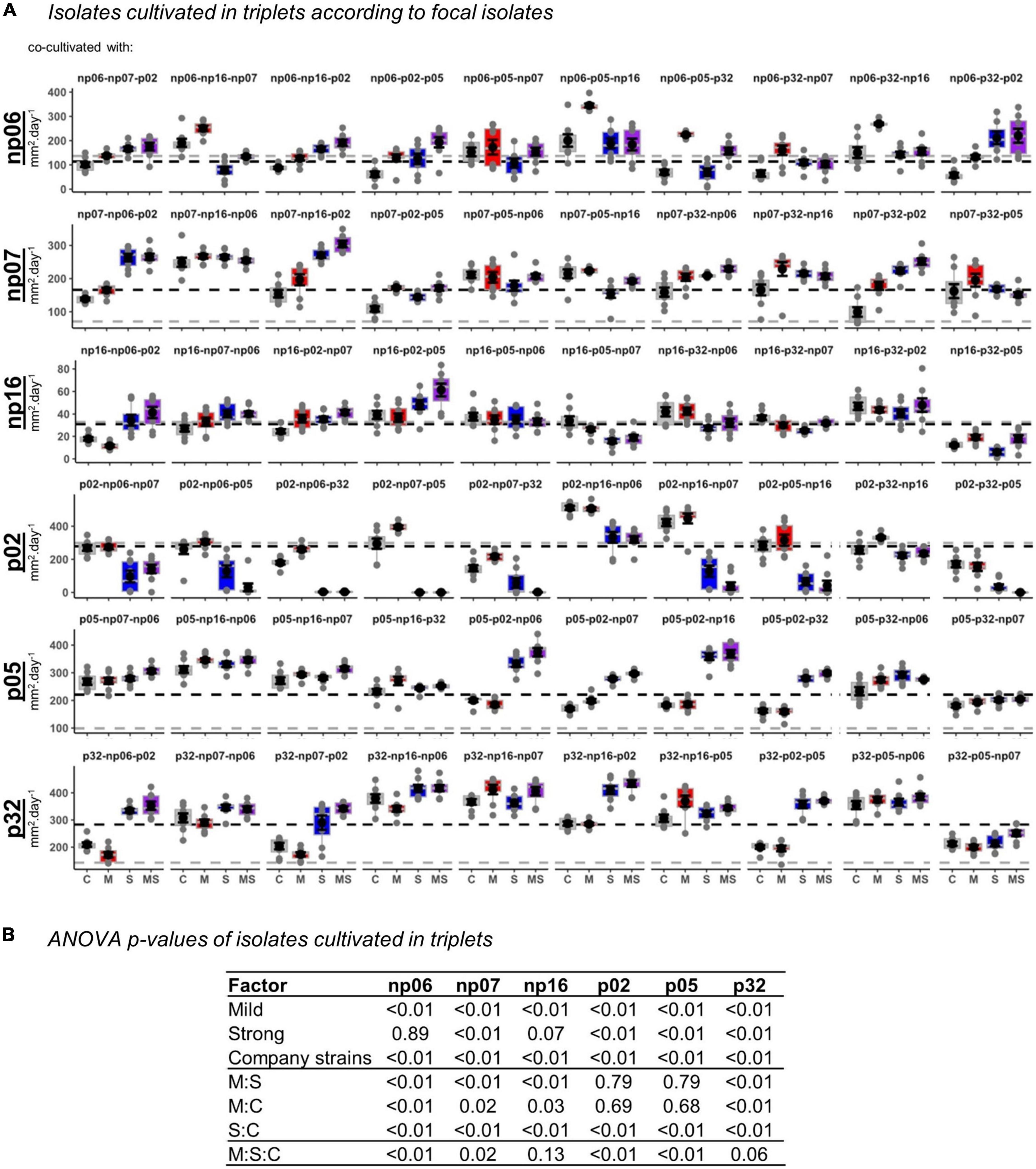
Figure 5. The effects of none (control, C), mild (M), strong (S), and both (MS) heat stress events on fungal isolate growth rates for fungal isolates cultivated in triplets. The panel containing box-plots with average and standard error of each focal isolate according to the triplets (A), and the robust analysis of variance (rANOVA) p-values for the isolates analyzed in a factorial design considering the heat treatments mild (yes or no), strong (yes or no) and company strains combinations (B). For the boxplots, the gray lines are references to the isolates growing alone in control conditions (data from Supplementary Figure 2) and the black lines are the average of all controls for each focal isolate.
Surprisingly, the occurrence of the interaction of recurring heating events (mild-strong) was more frequently observed in the triplets than in the pairs (Figure 4), where 10 significant events were observed out of 20 combinations, 50% (perMANOVA p < 0.05). In the pairs (Figure 1), only five significant events were observed out of 15 combinations, 33% (perMANOVA p < 0.05).
When analyzing the data considering the fungal triplet combinations as a factor (Figure 5), the result was slightly different from when the strains grew in pairs (Figure 2). For example, for triplets, the mild treatment was statistically significant across all the isolates, while for pairs it was only for half of the isolates (rANOVA p < 0.05). However, in both, pairs and triplets, it was evident that there was always an effect of the fungal combinations on the focal isolate growth rate (rANOVA p < 0.01), while the thermal effects were variable according to isolates (Figures 2, 5 and Supplementary Figures 4, 5). Among all isolates, p02 was the one that had the most interactions with other isolates. This isolate influenced the heat response of other isolates and it was also influenced by the other isolates (Figures 2, 5 and Supplementary Table 1). This isolate is the one with the highest growth rate (Supplementary Figure 2), and consequently, it has potentially the best advantage in the competition for space. However, our data showed that this isolate potential advantage was challenged by the isolate responses to the different temperature treatments, which was modulated by the pair and triplet combinations.
4. Discussion
We experimentally tested the hypothesis that species interactions can modulate the response capacity of fungal species, e.g., by affecting their response to heating and priming (Rillig et al., 2015; Wesener et al., 2021). We found support for this hypothesis since several fungal isolates had a different response to the heat events when co-cultivated with different isolates in pairs or triples.
Here, we tested this hypothesis by co-cultivating fungal isolates grown in combinations of mild and strong temperature stress treatments in pairs and triplets. In the previous study, the isolates we used here were shown to have a diverse response to heat stress events when cultivated in monocultures and some of the isolates presented a heat stress priming response (Szymczak et al., 2020). In our new study, the fungal response to recurring heat stress was shown to be modulated also by co-cultivation and fungal isolates interactions (Figures 2, 3). However, surprisingly, the isolates that previously demonstrated priming ability to the same heating treatments (i.e., p02, p05, and p32 in Szymczak et al., 2020) did not have a special growth advantage after the mild-strong temperature treatments when co-cultivated with other strains. Additionally, the co-cultivation made the thermal effects more complex and difficult to predict, since the isolates showed different responses to the temperature treatments according to the different company fungi or when growing in pairs or triplets (Figures 2, 4).
The variability of species in response to environmental stressors, such as mild and strong temperature stress, can alter fungal interactions in at least four ways. First, temperature changes can alter competitive hierarchies between fungal competitors (Crowther et al., 2012; Hiscox et al., 2016). Second, tolerance to changing temperature can be traded for competitive ability, with resulting trade-offs structuring the community and possibly leading to species coexistence (Kneitel and Chase, 2004; Maynard et al., 2019). Furthermore, the trade-off between stress tolerance and competitiveness can be further modulated by species specific responses, e.g., priming. For instance, in the third case, the presence of competing species can alter the focal species’ ability to express priming (Rillig et al., 2015; Wesener et al., 2021). Additionally, in the fourth case, the presence of a competitor can alter the ability of focal species to benefit from its priming ability, even if the priming ability remains unchanged. The latter two possibilities are harder to disentangle experimentally, even though previous research has indicated their potential importance (Rillig et al., 2015; Wesener et al., 2021).
While the present experiment cannot disentangle between community-level mechanisms modulating the heat response, we clearly showed the limits of making predictions on temperature stress effects, for example, heat waves, by studying responses of fungal species grown as monocultures (Szymczak et al., 2020). In fact, in natural soil fungi grow in communities, thus, it is of high relevance that our results clearly showed that a focal fungal isolate growth response to temperature stress is modulated by a company fungal isolate.
Some mechanisms modulating the heat response can be speculated. A focal isolate could have improved growth under heat treatment either by having relatively higher heat-related fitness than its competitor (e.g., heat generates an antagonist release), or the exudates and interference mechanisms of the co-cultivated competitor can increase the priming ability and thermal tolerance of the focal isolate. In the opposite direction (i.e., reduced growth rates in co-cultivation and heat stress), it is plausible that the adverse effects of competition and heat stress simply summed up, reducing their growth rate. Additionally, the metabolism of focal isolates could be affected by the presence of a co-cultivated fungus in such a way that prevented the focal fungus from optimally reacting to the heat event. In the previous study (Szymczak et al., 2020), the authors suggested that metabolic changes caused by the heating stress could include reduced growth after the stress because of the use of energy and resources to adjust membrane and carbon fluxes or an altered state in a transition to dormancy or sporulation (Morano et al., 2012; Bérard et al., 2015). In our present study, the co-cultivation of the isolates could induce competition of the isolates for resources and also induce other species interactions, such as facilitation, potentially inducing further metabolism changes to the heating stress response.
Regardless of the directions and mechanisms by which other community members influence the performance of focal species under abiotic stress, this interaction adds a new layer of complexity to fungal community assembly and ecology under climate change. These findings are important for ecological models that consider fungal growth and diversity outputs based on data produced at constant temperatures and based on monocultures. The experimental design and modeling of further works should take into consideration not only the heat pulses but also simultaneously possible biotic interactions of organisms growing in communities (Rillig et al., 2015; Wesener et al., 2021).
5. Conclusion
In conclusion, both the recurring heat events and the species interactions influenced the growth of focal fungal isolates and the interactions of the fungal pairs and triplets. We found that not only do environmental conditions influence fungal interactions and growth rates, but the co-cultivation of different fungi can affect the fungal response to recurring heat events. These results indicate that more complex experimental designs are needed to better understand the effects of recurring heat events and climate change on soil fungi.
Data availability statement
The raw data supporting the conclusions of this article is available in the Supplementary material. Further inquiries should be directed to the corresponding author.
Author contributions
AS and MR designed the study. AS conducted the experiments. DL analyzed the data. DL and MB drafted the manuscript. All authors reviewed and contributed to the final version.
Funding
This study was funded by the German Research Foundation (DFG), project number: 190578797. We acknowledged the support from the Open Access Publication Initiative of Freie Universität Berlin.
Acknowledgments
The authors are thankful for the participation in the Collaborative Research Centre (CRC) 973 “Priming and Memory of Organismic Responses to Stress “(https://www.sfb973.de, DFG project number: 190578797). The authors thank members of the Rillig lab for suggestions on the experiment, including Julien Roy and Masahiro Ryo and also Mehmet Akif Celiker for technical support.
Conflict of interest
The authors declare that the research was conducted in the absence of any commercial or financial relationships that could be construed as a potential conflict of interest.
Publisher’s note
All claims expressed in this article are solely those of the authors and do not necessarily represent those of their affiliated organizations, or those of the publisher, the editors and the reviewers. Any product that may be evaluated in this article, or claim that may be made by its manufacturer, is not guaranteed or endorsed by the publisher.
Supplementary material
The Supplementary Material for this article can be found online at: https://www.frontiersin.org/articles/10.3389/fevo.2022.1028136/full#supplementary-material
References
Anderson, M. J. (2017). “Permutational Multivariate Analysis of Variance (PERMANOVA),” in Wiley StatsRef: Statistics Reference Online, eds N. Balakrishnan, T. Colton, B. Everitt, W. Piegorsch, F. Ruggeri, and J. L. Teugels (Hoboken, NJ: John Wiley & Sons, Ltd), doi: 10.1002/9781118445112.stat07841
Andrade-Linares, D. R., Lehmann, A., and Rillig, M. C. (2016a). Microbial stress priming: a meta-analysis. Environ. Microbiol. 18, 1277–1288. doi: 10.1111/1462-2920.13223
Andrade-Linares, D. R., Veresoglou, S. D., and Rillig, M. C. (2016b). Temperature priming and memory in soil filamentous fungi. Fungal Ecol. 21, 10–15. doi: 10.1016/j.funeco.2016.02.002
Baldrian, P., Kolařík, M., Štursová, M., Kopecký, J., Valášková, V., Vétrovský, T., et al. (2012). Active and total microbial communities in forest soil are largely different and highly stratified during decomposition. ISME J. 6, 248–258. doi: 10.1038/ismej.2011.95
Benjamini, Y., and Hochberg, Y. (1995). Controlling the false discovery rate: a practical and powerful approach to multiple testing. J. R. Stat. Soc. Ser B Methodol. 57, 289–300.
Bérard, A., Sassi, M. B., Kaisermann, A., and Renault, P. (2015). Soil microbial community responses to heat wave components: drought and high temperature. Clim. Res. 66, 243–264. doi: 10.3354/cr01343
Crowther, T. W., Littleboy, A., Jones, T. H., and Boddy, L. (2012). Interactive effects of warming and invertebrate grazing on the outcomes of competitive fungal interactions. FEMS Microbiol. Ecol. 81, 419–426. doi: 10.1111/j.1574-6941.2012.01364.x
de Oliveira, T. B., de Lucas, R. C., Scarcella, A. S., de, A., Contato, A. G., Pasin, T. M., et al. (2020). Fungal communities differentially respond to warming and drought in tropical grassland soil. Mol. Ecol. 29, 1550–1559. doi: 10.1111/mec.15423
Hannula, S. E., and Morriën, E. (2022). Will fungi solve the carbon dilemma? Geoderma 413:115767. doi: 10.1016/j.geoderma.2022.115767
Geisen, S., Wall, D. H., and van der Putten, W. H. (2019). Challenges and opportunities for soil biodiversity in the anthropocene. Curr. Biol. 29, R1036–R1044. doi: 10.1016/j.cub.2019.08.007
Haegeman, B., Sari, T., and Etienne, R. S. (2014). Predicting coexistence of plants subject to a tolerance-competition trade-off. J. Math. Biol. 68, 1815–1847. doi: 10.1007/s00285-013-0692-4
Hamilton, N. E., and Ferry, M. (2018). ggtern: Ternary Diagrams Using ggplot2. J. Stat. Softw. 87, 1–17. doi: 10.18637/jss.v087.c03
Hettmansperger, T. P., and McKean, J. W. (2010). Robust Nonparametric Statistical Methods, 2nd Edn. Boca Raton, FL: CRC Press, doi: 10.1201/b10451
Hilker, M., Schwachtje, J., Baier, M., Balazadeh, S., Bäurle, I., Geiselhardt, S., et al. (2016). Priming and memory of stress responses in organisms lacking a nervous system. Biol. Rev. 91, 1118–1133. doi: 10.1111/brv.12215
Hiscox, J., Clarkson, G., Savoury, M., Powell, G., Savva, I., Lloyd, M., et al. (2016). Effects of pre-colonisation and temperature on interspecific fungal interactions in wood. Fungal Ecol. 21, 32–42. doi: 10.1016/j.funeco.2016.01.011
Kneitel, J. M., and Chase, J. M. (2004). Trade-offs in community ecology: linking spatial scales and species coexistence. Ecol. Lett. 7, 69–80. doi: 10.1046/j.1461-0248.2003.00551.x
Maynard, D. S., Bradford, M. A., Covey, K. R., Lindner, D., Glaeser, J., Talbert, D. A., et al. (2019). Consistent trade-offs in fungal trait expression across broad spatial scales. Nat. Microbiol. 4, 846–853. doi: 10.1038/s41564-019-0361-5
Morano, K. A., Grant, C. M., and Moye-Rowley, W. S. (2012). The response to heat shock and oxidative stress in Saccharomyces cerevisiae. Genetics 190, 1157–1195. doi: 10.1534/genetics.111.128033
Oksanen, J., Blanchet, F. G., Friendly, M., Kindt, R., Legendre, P., McGlinn, D., et al. (2019). vegan: Community Ecology Package. Falmouth, MA: BCO-DMO.
Perkins-Kirkpatrick, S. E., and Gibson, P. B. (2017). Changes in regional heatwave characteristics as a function of increasing global temperature. Sci. Rep. 7:12256. doi: 10.1038/s41598-017-12520-2
Pinheiro, J., and Bates, D. (2000). Mixed-Effects Models in S and S-PLUS. New York, NY: Springer, doi: 10.1007/b98882
Rillig, M. C., Rolff, J., Tietjen, B., Wehner, J., and Andrade-Linares, D. R. (2015). Community priming—effects of sequential stressors on microbial assemblages. FEMS Microbiol. Ecol. 91:fiv040. doi: 10.1093/femsec/fiv040
Szymczak, A., Ryo, M., Roy, J., and Rillig, M. C. (2020). Diversity of growth responses of soil saprobic fungi to recurring heat events. Front. Microbiol. 11:1326. doi: 10.3389/fmicb.2020.01326
Keywords: fungi, temperature stress, heating wave, species interaction, ecology
Citation: Lammel DR, Szymczak A, Bielcik M and Rillig MC (2023) Fungal growth response to recurring heating events is modulated by species interactions. Front. Ecol. Evol. 10:1028136. doi: 10.3389/fevo.2022.1028136
Received: 25 August 2022; Accepted: 28 December 2022;
Published: 09 February 2023.
Edited by:
Andrew Spiers, Abertay University, United KingdomReviewed by:
Andrii Gryganskyi, UES, Inc., United StatesElly Morrien, University of Amsterdam, Netherlands
Copyright © 2023 Lammel, Szymczak, Bielcik and Rillig. This is an open-access article distributed under the terms of the Creative Commons Attribution License (CC BY). The use, distribution or reproduction in other forums is permitted, provided the original author(s) and the copyright owner(s) are credited and that the original publication in this journal is cited, in accordance with accepted academic practice. No use, distribution or reproduction is permitted which does not comply with these terms.
*Correspondence: Matthias C. Rillig, cmlsbGlnQHplZGF0LmZ1LWJlcmxpbi5kZQ==