- 1International Centre of Insect Physiology and Ecology (icipe), Nairobi, Kenya
- 2Department of Chemistry, Faculty of Natural and Agricultural Science, University of Pretoria, Pretoria, South Africa
- 3Unit of Chemical Ecology, Department of Plant Protection Biology, Swedish University of Agricultural Sciences, Alnarp, Sweden
- 4Department of Zoology and Entomology, Faculty of Natural and Agricultural Science, University of Pretoria, Pretoria, South Africa
Bactrocera dorsalis (Hendel) is a major pest of fruits and vegetables worldwide with documented losses of up to 100%. Various management techniques including the use of parasitoids, such as Fopius arisanus (Sonan) and Diachasmimorpha longicaudata (Ashmead) (Hymenoptera: Braconidae) within the context of the Integrated Pest Management (IPM) approach have been deployed for its control. The effectiveness of parasitoids is well understood, but knowledge of the semiochemicals that mediate their behavior, as well as that of the host fruit fly to tree-attached mangoes, is lacking. Here, we first compared the attractiveness of the above-mentioned fruit fly and its parasitoids to volatiles of different treatments (non-infested physiologically mature unripe and ripe mangoes, mangoes newly exposed to ovipositing B. dorsalis, and mangoes on day 7 and day 9 post-oviposition) of tree-attached Kent, Apple, and Haden mango varieties relative to control (clean air). The fruit fly was significantly more attracted to the mango volatiles (up to 93% of responsive insects) compared to the control (clean air). Fopius arisanus was significantly more attracted to mangoes with ovipositing fruit flies (68–76%) while D. longicaudata was significantly more attracted to day 9 post-oviposited mangoes (64–72%) compared to the control. Secondly, we elucidated the headspace volatile profiles of the non-infested and infested tree-attached mangoes using gas chromatography linked to mass spectrometry (GC-MS). The volatiles revealed various types of organic compounds with qualitative and quantitative differences. The majority of the compounds were esters making 33.8% of the total number, followed by sesquiterpenes-16.4%, and monoterpenes-15.4% among others. Most compounds had higher release rates in headspace volatiles of fruit fly-infested mangoes. Lastly, we harvested the infested mangoes and incubated them for puparia recovery. The number of puparia recovered varied according to the mango variety with Apple mango registering 81.7% of the total, while none was recovered from Kent. These results represent the first report of the changes in the headspace components of non-infested and infested tree-attached mangoes and the associated differential responses of the mentioned insects. A follow-up study can reveal whether there is a convergence in olfactomes which is significant when developing baits that selectively attract the fruit fly and not its natural enemies and fill the knowledge gap from an evolutionary ecological perspective.
Introduction
Mango (Mangifera indica L.) is one of the most widely grown fruits, ranking fifth among major fruit crops in terms of production, with global production of over 55.9 million metric tons in 2019 (Shahbandeh, 2021). In sub-Saharan Africa, mango is an important commodity as it has considerable socioeconomic importance, as a source of food and income for millions of mango growers and other actors along the mango value chain. However, its production and utilization have been hampered by a plethora of biotic and abiotic constraints key among them being infestation by tephritid fruit flies. Bactrocera dorsalis (Hendel) (Diptera: Tephritidae) is one of the most destructive fruit flies (Boinahadji et al., 2020) causing losses of up to 100% if control measures are not implemented (Nankinga et al., 2014; Ekesi et al., 2016, and reference therein). Integrated pest management (IPM) strategies used in its control include the use of chemicals (Akotsen-Mensah et al., 2017; Díaz-Fleischer et al., 2017), lure and kill traps (Doorenweerd et al., 2018; Stringer et al., 2019), early fruit harvesting, bagging and netting (Ndlela et al., 2016), orchard sanitation (Verghese et al., 2004), use of sterilized males [Sterile Insect Technique (SIT)] (Enkerlin et al., 2017; Cai et al., 2020), use of semiochemicals (Biasazin et al., 2018, 2019; Cai et al., 2020; Scolari et al., 2021), and the use of fruit fly natural enemies which include pathogens, predators, and parasitoids (Mohamed et al., 2010; Cai et al., 2020). The understanding of the ecological features that influence the interactions between phytophagous insects and their host plant/fruit is of crucial importance in developing sustainable fruit defense strategies. Several studies on herbivore-plant interactions have elucidated the central role of volatile organic compounds that act as host location kairomones for herbivores (Metcalf and Kogan, 1987; Carrasco et al., 2015; Guarino et al., 2018). The importance of these secondary plant substances as cues for host plant selection was emphasized several decades ago by Fraenkel (1969).
Volatile organic compounds emitted by plants and fruits play major roles in attracting or repelling insect pests (Benelli et al., 2014; Binyameen and Anderson, 2014), as well as in attracting their natural enemies including parasitoids (Segura et al., 2012; Harbi et al., 2019). Previous studies have highlighted some semiochemical-mediated interactions between fruits, fruit flies, and parasitoids (Carrasco et al., 2005; Harbi et al., 2019). For example, volatiles from three mango varieties (Amate, Coche, and Ataulfo) were found to be attractive to Anastrepha obliqua (Macquart) (Diptera: Tephritidae) (Malo et al., 2012), and a total of 22 compounds from ‘Chausa’ and ‘Alphonso’ were EAG active in female B. dorsalis antennae (Kamala et al., 2012). Furthermore, γ-octalactone, ethyl tiglate, benzothiazole, and 1-octen-3-ol either singly or as a blend elicited oviposition response in B. dorsalis (Kamala et al., 2014). A blend of common EAG active volatiles from diverse fruits (guava, banana, mango, and orange) increased the attractiveness of a majority of polyphagous fruit fly species in laboratory experiments (Biasazin et al., 2014, 2019).
The Opiinae subfamily of the Braconidae family is made up of over 1,500 koinobiont endoparasitoid species (Copeland et al., 2006; Badii et al., 2016). Fopius arisanus (Sonan) and Diachasmimorpha longicaudata (Ashmead) (both Hymenoptera: Braconidae) are solitary egg-prepupal endoparasitoids that have been used extensively for biological control of B. dorsalis with outstanding success in Hawaii (Flávio et al., 2020) and French Polynesia (Roger et al., 2012). Recently, the two parasitoids have been introduced into Kenya (Mohamed et al., 2008, 2010) and subsequently released in several African countries for classical biological control of B. dorsalis (Mohamed et al., 2016; Ndlela et al., 2020). Gravid F. arisanus females are attracted to their hosts either using volatiles emanating from the fruits during or after oviposition (Cai et al., 2020). Also, female D. longicaudata is known to exploit semiochemicals from the hosts’ fruits and fruit fly larvae and is more attracted to host-infested fruits than non-infested or mechanically damaged fruits (Carrasco et al., 2005; Segura et al., 2012; Harbi et al., 2019).
In most studies on fruit-fruit fly-parasitoid interactions, little effort, if any, has been made to unravel the changes in volatile composition that occur before and after fruit fly infestation, specifically on tree-attached fruits under field conditions, and how these changes affect the behavior of the fruit fly and its natural enemies. Therefore, the current study aimed at investigating the behavioral responses of B. dorsalis, and the parasitoids F. arisanus and D. longicaudata to volatiles of three tree-attached mango varieties (Kent, Apple, and Haden) that were either non-infested or at different stages of infestation by the fruit fly and then elucidating the chemicals profiles of the mentioned mango varieties headspaces under the non-infested and the infested treatments.
Materials and methods
Mango fruits
During the flowering season, in July 2020, three varieties of mango trees (Kent, Apple, and Haden) were identified, in their growing habitat in Gathigiriri (00°41′39.8″S, 037°24′26.7″E, 1,158 m ASL), Mwea East Sub-county, Kirinyaga County, Kenya. The orchard contained 85 mature mango trees comprising the following varieties Kent (13), Apple (36), Haden (6), Van Dyke (4), Ngowe (8), Tommy Atkin (4), Mukarati (4), and 10 local varieties. In this area, Haden mangoes usually ripen in late December, Apples in January, and Kent ripens in April. Two mango trees of each of the three varieties were randomly selected from the orchard. The trees were kept free of insecticides and fungicides during the entire period of the trials. To prevent crawling insects like ants and termites from damaging the flowers and young fruits, duduthrin 1.75 EC (Twiga Chemical Industries Ltd., Nairobi, Kenya) was applied at the base of each tree according to the manufacturer’s guidelines. The mango fruits were allowed to develop for 4 months, from the time of flowering, after which they were secured in situ (Figure 1) using fine white nets that were mounted on 20 cm × 20 cm × 20 cm of 2.5 mm galvanized metallic wire cube frames sourced from the local market.
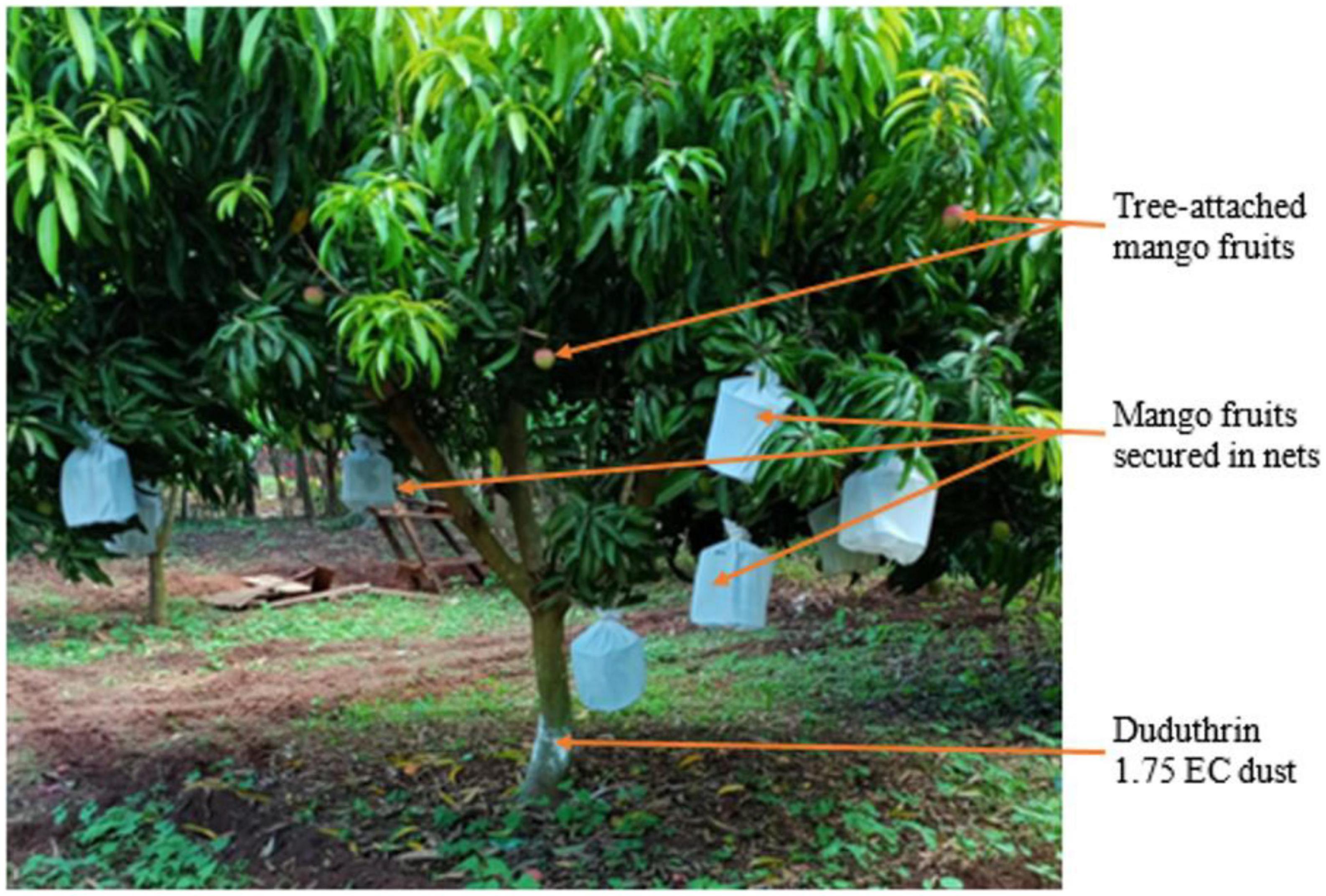
Figure 1. A mango tree with mango fruits some of which were secured using white netted cages to safeguard them from insect attack.
Depending on the mango variety and fruit size, each net cage could hold at least four mangoes. From each mango tree, at least 32 mango fruits were secured. The caged mango fruits were inspected every week until they were physiologically mature and ready for use in the trials.
Fruit flies
Bactrocera dorsalis was reared at the International Centre of Insect Physiology and Ecology (icipe) Duduville campus (01°13′25.3″S, 36°53′49.2″E; 1,600 m ASL) Nairobi Kenya following already established protocols (Ekesi and Mohamed, 2011; Gordello, 2013), where the fruit fly colony was maintained at 26 ± 2°C, 50–60% RH, and a photoperiod of 12:12 h (L: D). Ripe Apple mangoes were purchased from the local market in Nairobi, Kenya, and thoroughly cleaned using liquid soap and tap water to remove surface dirt, rinsed with distilled water which was then wiped out using paper towels. The mangoes were then stored at 4°C for 48 h to kill any residual fruit fly egg/larvae, followed by thawing for 2 h in a laminar flow hood. The six fruits were offered to 12–16 day old B. dorsalis [n = 100 (♂: ♀ = 1:1)] held in a Perspex rearing cages (30 cm × 30 cm × 30 cm) as oviposition substrate for 3 h. The rearing cages had a fine net mounted on two opposite sides to allow for air circulation and a netted window for the provision of food and water to the fruit flies. The adult fruit flies were fed on an artificial diet containing a mixture of finely ground sugar (Mumias Sugar Company, Nairobi Kenya) and enzymatic yeast hydrolyzate (USB Corporation, Cleveland, OH, USA) in a ratio of 3:1. Water was provided ad libitum in glass Petri-dishes (90 mm × 15 mm) with pumice granules to prevent drowning. The infested fruits were then transferred into plastic containers (21 cm × 14 cm × 8 cm; Kenpoly manufacturers limited, Nairobi, Kenya) for eggs to hatch and larvae to develop. The plastic containers were perforated on their bottom side and a sheet of paper towel followed by a fine net laid on the inside. This was done to allow soaking and drainage of any sap that was produced as the larvae developed and the fruit rot and to prevent larvae from escaping. Each plastic container was covered with a fine net and a perforated plastic lid to allow for air circulation. On the onset of pupation, the infested mangoes were put in plastic basins (32 cm diameter × 14 cm depth, Kenpoly manufacturers limited) that were quarter filled with dry, fine (>1.18 mm), and sterilized sand for larvae to pupate. The basins were also perforated at the bottom and a fine net was laid covering the perforations before the sand was added to allow sap drainage. The basins were then covered with a white net to prevent third instar larvae from jumping out. After pupation, the content of the basin was soaked in excess water to separate the puparium from the sand and the remains of the mangoes. The floating puparia were then recovered through sieving (Cheseto et al., 2017), put in petri-dishes, and then transferred into the Perspex rearing cages (30 cm × 30 cm × 30 cm) for eclosion. The adult fruit flies were maintained as aforementioned but at room conditions of temperature (day = 23 ± 4°C, night = 20 ± 4°C), humidity (38–68% RH), and natural photoperiod.
Fruit fly parasitoids
The egg parasitoid Fopius arisanus and the larval parasitoids Diachasmimorpha longicaudata used in this study were also reared at icipe, Duduville campus (Nairobi, Kenya). The host fruit flies were the newly established colony of B. dorsalis explained in section “Fruit flies”.
Fopius arisanus colony was initiated by exposing six Apple mangoes to a colony of 100 adults of B. dorsalis (ratio ♂: ♀ = 1:1) for 3 h (8.00 a.m.–11.00 a.m.). Two sets of three mangoes were then put in cages each containing 100 adults of 8–15 days-old F. arisanus (♂: ♀ ratio = 1:1) for 19 h. For D. longicaudata, mangoes were exposed to B. dorsalis as aforementioned. The infested mangoes were then incubated for 6 days to allow the larvae to develop to the second instar and then transferred into cages containing 100, 8–15 days-old D. longicaudata adults (♂: ♀ = ratio-1:1) for 3 days to maximize parasitization. After eclosion, the parasitoids were separated from B. dorsalis and transferred into their respective cages. Adult parasitoids were fed on 80% honey (Eco Honey, icipe, Nairobi, Kenya) that was spotted on the inside upper surface of the rearing cage, and water was provided ad libitum in glass Petri-dishes with gravel granules and rolled cotton wool (Supplementary Figure 1) after every 4 days (Manoukis et al., 2011). The new parasitoid colony was maintained under room conditions.
Behavioral responses of female fruit flies and parasitoids to tree-attached mango volatiles
Dual-choice olfactometer assays were carried out under the field conditions at Mwea East Sub-county, Kirinyaga County, Kenya (00°41′39.8″S, 037°24′26.7″E, 1,158 m ASL) to evaluate the responses of fruit flies and parasitoids to mango fruit volatiles, in situ, following the methods described by Nyasembe et al. (2012) and Miano et al. (2022) with some modifications. The dual-choice olfactometer and the mango holders were made of Perspex glass (Figure 2).
The temperatures and the humidity during the assays were not regulated since the experiments were conducted in the field. In all the bioassays, the airflow through each of the olfactometer arms was maintained at 350 mL min–1 and evacuated at the center (700 mL min–1) using air-flow meters connected to a portable vacuum field air pump (Analytical Research System Inc., Gainesville, FL, USA). For each bioassay, 10 mated adult females (10–15 days old for B. dorsalis or 8–14 days for parasitoids) were placed in a releasing vial and kept for 10 min for acclimatization. Thereafter, the group of insects was released through a hole at the center of the bottom of the wind tunnel (Figure 2) using a black coated falcon tube and they were allowed 20 min to choose, following the procedure of group release described with modifications by Nyasembe et al. (2012), Biasazin et al. (2014), Njuguna et al. (2018), Binyameen et al. (2021), and Miano et al. (2022) with modifications. The base of the dual-choice olfactometer was marked from 0 to 60 cm on either side of the insect release point to allow scoring. The insects that moved beyond 30 cm from the center on either side of the olfactometer were considered to have made a choice, while those that were in the range of 0–30 cm from the release point were non-responsive. Non-responsive insects were not included in the statistical analysis. For the fruit flies and each species of parasitoids, seven replicates were done (as the day would allow) per choice test. The tested insects were removed through widows marked A (Figure 2) and put in a separate cage. Between two runs, clean air was passed through the apparatus for 20 mins to blow out the odor of the previous test, the air inlets were then changed to avoid positional bias, and -air from odor sources was allowed to pass through the apparatus for 10 mins to stabilize the airflow. For fruit fly infestation, 15 females were randomly selected from a cage containing a 10–15 day old mixture of males and females (♂: ♀ = 1:1) and then released into the mango holder cages (Figure 2) containing four mangoes as an oviposition substrate. Before the assays, the fruit flies and mangoes were kept together for 20 min for fruit flies to probe (the fruit flies remained with the mangoes until the last replicate of that day was done). To ascertain the activity of the fruit flies, mangoes were assessed before and after exposure for punctures and oozing sap using a hand lens (×10). The newly infested mangoes were secured in nets and used for subsequent infested mango assays. After the day tests, the odor containers and the olfactometer were cleaned using hot water and allowed to dry overnight.
Behavioral experiments included the responses of (i) B. dorsalis, F. arisanus, or D. longicaudata to control (clean air); (ii) B. dorsalis or F. arisanus to volatiles of non-infested physiologically mature but unripe mangoes versus control; (iii) B. dorsalis or F. arisanus to volatiles of mangoes with ovipositing B. dorsalis versus control; (iv) responses of B. dorsalis or D. longicaudata to volatiles of mangoes on day 7 or day 9 B. dorsalis post-oviposited mangoes versus control; and (v) responses of B. dorsalis, F. arisanus, or D. longicaudata to volatiles of non-infested ripe mangoes versus control. Each day, the experimental mangoes were secured back into the fine white netted cages to prevent any additional damage. On the tenth day after exposure to fruit flies, the infested mangoes were harvested and incubated for pupation.
Performance of Bactrocera dorsalis in the different mango varieties
To assess the performance of the fruit flies in the three varieties of mangoes, the infested mangoes were harvested on the tenth day and incubated as aforementioned (section “Fruit flies”). Fruit harvesting was informed by the fact that on the tenth day, most of the infested Apple and Haden mangoes had detached from the tree. Kent mango was observed to take longer before detaching. The puparia that were recovered from the infested mangoes were counted and recorded.
In situ collection of mango headspaces
The collection of mango headspaces was carried out simultaneously during the bioassay experiments. Dynamic headspace trapping (DHT) system (Ormeño et al., 2011; Miano et al., 2022) was used with some modifications (Supplementary Figure 2). Ambient air was drawn into the system using portable vacuum field pumps and passed via air flow meters at a rate of 250 mL/min and drawn out at the same rate. Headspace volatiles were trapped in tree-attached mangoes, which were bagged in a polyacetate oven bag (KitchenCraft Ltd., Birmingham, UK) (Supplementary Figure 2). Volatiles were collected onto HayeSep-Q adsorbents [30 mg, copolymers of polydimethylsiloxane-divinylbenzene (PDMS-DVB)] which were pre-cleaned with GC-grade dichloromethane (DCM). The headspace volatiles were trapped in quadruplets from the same tree for each of the subsequent mango treatments. These treatments include (i) non-infested physiologically mature unripe mango fruits; (ii) mango fruits with ovipositing B. dorsalis; (iii) infested mango fruits, a day after oviposition; (iv) infested mango fruits at (a) day 7 and (b) day 9 post-oviposition; (v) non-infested ripe mango fruits; and (vi) clean air as control. Headspace volatiles were collected for 11 h (7.00 a.m. to 6.00 p.m.). For preservation and transportation of the headspace volatiles trapped in HayeSep-Q adsorbents the procedure explained in Miano et al. (2022) was used. The headspace volatiles trapped in HayeSep-Q were then eluted in 200 μL DCM into 250 μL conical point glass inserts contained in clear 1.5 mL glass vials (Supelco, Bellefonte, PA, USA) using high-purity nitrogen gas as the pressurizing gas and immediately stored in a freezer at −81°C until use.
Chemical analysis of mango headspace volatiles
The headspace volatiles were analyzed (1 μL) by GC-MS, a 7890A gas chromatograph linked to a 5975C mass selective detector (Agilent Technologies Inc., Santa Clara, CA, USA). The GC-MS instrument was equipped with an HP-5 MS (5% phenyl-methylpolysiloxane) column (30 m × 0.25 mm ID × 0.25 μm film thickness). The oven temperature program was 35°C for 5 min, then increase to 280°C at the rate of 10°C min–1, and then held at this temperature for 10.5 min. The mass selective detector was retained at 230°C ion source temperature and a quadrupole temperature of 180°C. Electron acceleration energy of 70 eV was used to obtain electron impact (EI) mass spectra while the resulting ions were analyzed over the mass range of 40–550 m/z in the full scan mode. The solvent delay time was set at 3.3 min. High-purity helium gas was used as the carrier gas at a flow rate of 1.2 mL min–1.
The qualitative identification of compounds was done by comparing the mass spectrometric data and retention times to those of reference spectra published by the library–MS databases National Institute of Standards and Technology (NIST 05, 08, and 11), Adams and Chemecol, and also based on their retention indices (RI) while some were confirmed using authentic standard retention times (Supplementary Figure 3). The RI (determined using a homologous series of straight-chain alkanes, C5–C31) was calculated based on the equation of Van den Dool and Kratz and compared with documented values (van Den Dool and Kratz, 1963; Adams, 1995).
For relative quantification of the release rates of volatiles, a serial dilution (2.25–1,000 ηg μL–1) of the authentic standards α-pinene and α-humulene (98% purity, Sigma-Aldrich ® Solutions, St. Luis, MO, USA) were analyzed by GC-MS in full scan mode to generate linear calibration curves (peak area vs. concentration, Supplementary Figure 4; Njuguna et al., 2018; Miano et al., 2022). The linear equations generated were y = 2036653.8x − 5127153.0; R2 = 0.9963 for α-pinene and y = 1127808.7x − 5512234.2; R2 = 0.9991 for α-humulene and were used to quantify volatile compounds that had retention times that were either below or above 16 min, respectively.
Chemicals
All synthetic chemicals used in this study were purchased from Merck, Germany. These compounds included dichloromethane (DCM) for elution, hexanal, (2E)-hexenal, p-xylene, α-pinene, camphene, 1-octen-3-ol, myrcene, δ-3-carene, δ-2-carene, o-cymene, limonene, (Z)-β-ocimene, (E)-β-ocimene, γ-terpinene, terpinolene, linalool, n-non-anal, 1,3,8-p-menthatriene, allo-ocimene, terpinen-4-ol, n-decanal, β-elemene, (E)-caryophyllene, α-humulene, and caryophyllene oxide which had a chemical purity of 90-99.9%, α-phellandrene and sabinene (purity 85 and 75%, respectively) were used for identification of volatiles.
Statistical analysis
The data of the numbers of the responsive fruit flies and parasitoids were analyzed using the R software (RStudio Team, 2021) at a significant level of 5%. The choice of fruit flies and parasitoids between host volatiles and clean air was assessed using the Chi-square goodness of test to confirm whether the responsive insects were in the ratio of 1:1.
The numbers of puparia harvested from the three mango varieties and the numbers of compounds detected from the treatments of each variety of mangoes were compared using Pearson’s Chi-square test followed by Chi-square multi-comparison test in RVAideMemoire (version 0.9-80) (RStudio Team, 2021).
The mango volatile release rates from the three varieties were subjected to the Shapiro-Wilk test and Barlett’s test to check the normality of distribution and homogeneity of variances, respectively. Since the data did not meet these assumptions, non-parametric tests were henceforth performed to analyze the data. The non-parametric Kruskal–Wallis rank-sum test followed by the post hoc Dunn test for pairwise comparison was used to test whether the volatile release rates from the three mango varieties under the different treatments were equal (Dinno, 2015) (where volatiles were present in only two treatments, Mann-Whitney U test was used). The data were then subjected to the non-metric multidimensional scaling (NMDS), similarity percentages (SIMPER) analysis, and one-way analysis of similarities (ANOSIM) using Bray–Curtis dissimilarity matrix in the Past 3 software (Hammer et al., 2001).
The volatile release rate data were then analyzed per mango variety where each data was subjected to one-way analysis of similarities (ANOSIM) to determine whether the headspace composition among treatments was significantly different. Furthermore, the non-metric multidimensional scaling, NMDS and the similarity percentage, SIMPER (Rohart et al., 2017) were performed and the top 30 compounds were visualized graphically. The 30 most discriminant compounds were also used in making NMDS biplots and in the construction of heatmap clusters (Rohart et al., 2017; Ayelo et al., 2021; Miano et al., 2022) using the auto-scaled average of their volatile release rate (y = log10x + 1), where x = average volatile release rates in ηg/mango//h).
The relative release rates of the common compounds present in the headspace of non-infested unripe mangoes or non-infested ripe mangoes were selected from the different treatments of the same mango variety and compared as follows: (i) mango with ovipositing B. dorsalis and mango a day after oviposition relative to those of non-infested physiologically mature unripe mango and (ii) day 7 and day 9 post-oviposited mangoes relative to those of non-infested ripe mangoes. A Kruskal-Wallis rank-sum test was performed to test for the difference in headspace volatile release rates in each of the three treatments followed by the Dunn test for pairwise comparison to test where the differences reported originated from. Furthermore, the averages of the compounds that were common in volatiles as selected in (i) and (ii) were auto-scaled using y = 2 + log10x and their number of fold changes in the quantities relative to either those of non-infested unripe mangoes or non-infested ripe mangoes calculated, where the number of fold changes was given by and then visualized using line graphs.
Results
Behavioral assays of Bactrocera dorsalis and parasitoids to tree-attached non-infested and infested mangoes
In our preliminary assays where control treatments were used, B. dorsalis, F. arisanus, and D. longicaudata showed no significant difference in the number of females that chose either arm of the wind tunnel (P > 0.05; Figure 3). On the other hand, the attraction of the fruit fly and the wasps to mango headspaces differed in magnitude compared to clean air. B. dorsalis was significantly attracted to the volatiles of non-invested ripe and Kent mangoes with ovipositing B. dorsalis (respectively, χ2 = 13.5, P < 0.001; χ2 = 7.02, P < 0.01) but not to the non-infested, 7 and 9 days post-oviposited Kent mangoes compared to the control (Figure 3A). Apple and Haden mango volatiles were more attractive to B. dorsalis (P < 0.001) except for 9 days post-oviposited Apple mango (χ2 = 4.49, P < 0.05) compared to the control (Figures 3B,C). For The egg parasitoid, F. arisanus was not attracted to volatiles from unripe Kent, Apple, or Haden mango, while when ripe only volatiles of ripe Apple mangoes attracted F. arisanus (χ2 = 3.2, P < 0.05). In the presence of ovipositing B. dorsalis, however, all mango varieties significantly attracted F. arisanus (χ2 = 4.45, P < 0.05; χ2 = 7.2, P < 0.01; χ2 = 5.11, P < 0.05, respectively, for Kent, Apple, and Haden with ovipositing B. dorsalis) (Figures 3A–C). Diachasmimorpha longicaudata was significantly attracted to ripe mangoes, regardless of variety (χ2 = 4.17, P < 0.05; χ2 = 4.36, P < 0.05; χ2 = 5.63, P < 0.05, respectively, for Kent, Apple, and Haden ripe mangoes). Except for the Kent mango, D. longicaudata was attracted to day 9 post-oviposited mangoes (χ2 = 12.5, P < 0.001; χ2 = 4.90, P < 0.05, respectively, for Apple and Haden) more than the control (Figures 3A–C). Curiously, mangoes 7-day post-oviposition did not attract D. longicaudata more than the control.
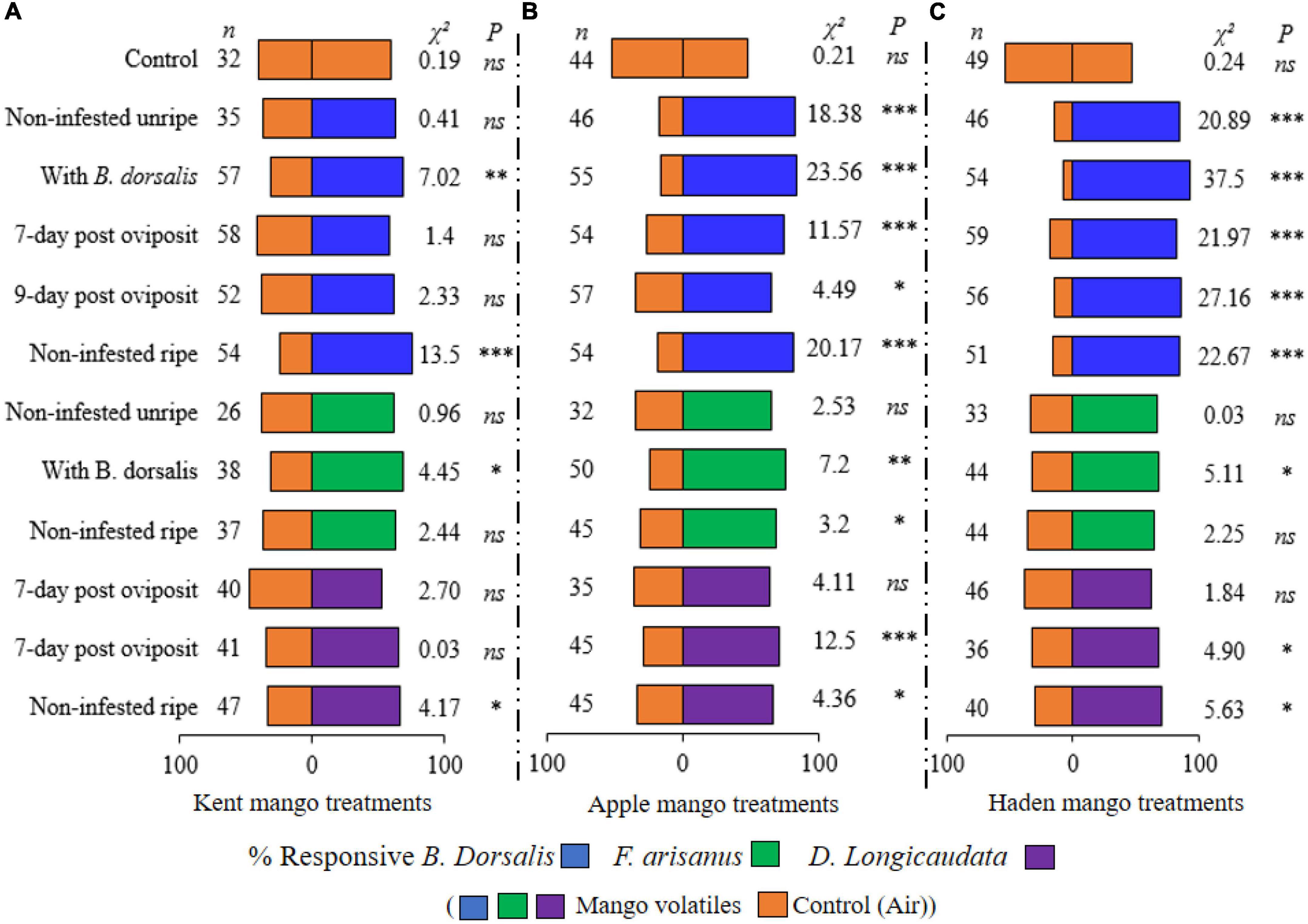
Figure 3. Responses (%) of Bactrocera dorsalis (blue), Fopius arisanus (green), and Diachasmimorpha longicaudata (pink) to different treatments of Kent (A), Apple (B), and Haden (C) mango volatiles. n = numbers of responsive insects out of the 70 tested; χ2, Chi-square; ns, no significant difference, and *, **, *** = significance differences with P < 0.05, 0.01, 0.001, respectively (chi-square goodness of fit test).
Performance of Bactrocera dorsalis on the different mango varieties
The performance of B. dorsalis in the three mango varieties as measured by the number of recovered puparia varied considerably (χ2 = 328.39, df = 2, P < 0.0001) with Apple mango yielding more than 4-fold of the yield from Harden variety (Figure 4). Although punctures and fruit sap were observed on the day of oviposition on Kent mangoes, there were no B. dorsalis puparia recovered from this variety.
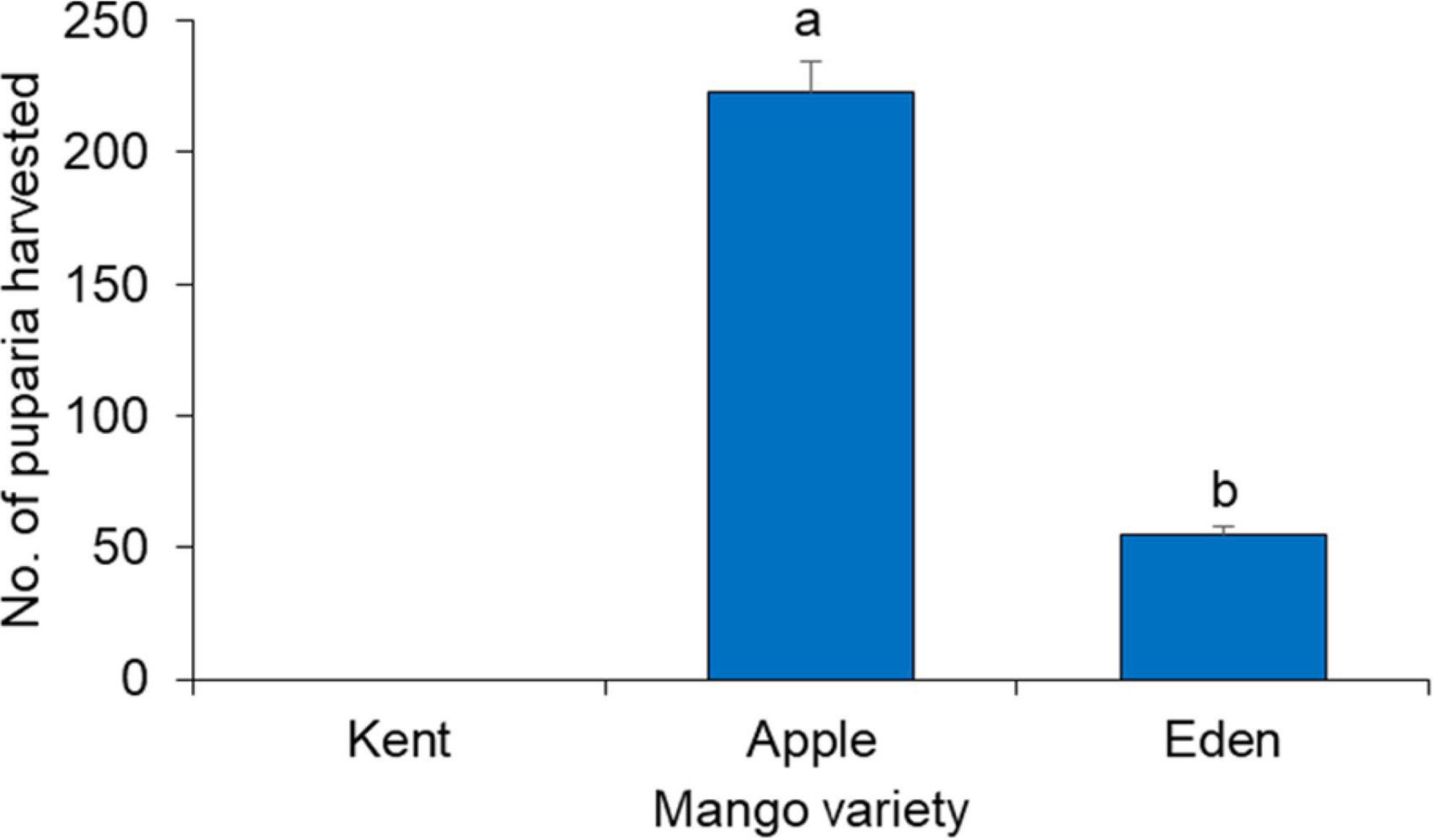
Figure 4. Number of puparia harvested from the different mango varieties. Bars capped with different letters are significantly different (Pearson’s Chi-square test followed by Chi-square multi-comparison test in RVAideMemoire).
Headspace volatiles in treatments of the three varieties of mangoes
A total of 195 volatiles were identified in the mango headspaces, the composition of which varied between the treatments and the mango variety (Supplementary Table 1). Kent mango registered the highest number of compounds (134) followed by Haden (114) while Apple had the least (102) (Supplementary Table 1). Among the compounds detected, 66 were esters, 32 sesquiterpenes, 30 monoterpenes, 14 monoterpenoids, 12 aldehydes, 9 ketones, 10 alcohols, 6 sesquiterpenoids, 5 benzenoids, 3 organic acids, 3 diterpenes, and 5 others (Supplementary Table 1). Among the compounds detected, 9 compounds (α-pinene, β-pinene, myrcene, δ-3-carene, α-gurjunene, (E)-caryophyllene, β-copaene, α-humulene, and δ-cadinene) were present in all treatments of the three varieties of mangoes but with varying release rates (Supplementary Table 1). There were significant differences in the volatile release rates between treatments of the three mangoes varieties (Supplementary Table 1). Infestation affected the volatile released both qualitatively (Figure 5A) and quantitatively (Figure 5B), with variations observed between mango varieties. Except for the Apple mango of which the quantitative change was at its peak on the oviposition day, the aspects of qualitative and quantitative increase peaked on day 9 post-oviposition (Figure 5B and Supplementary Table 1).
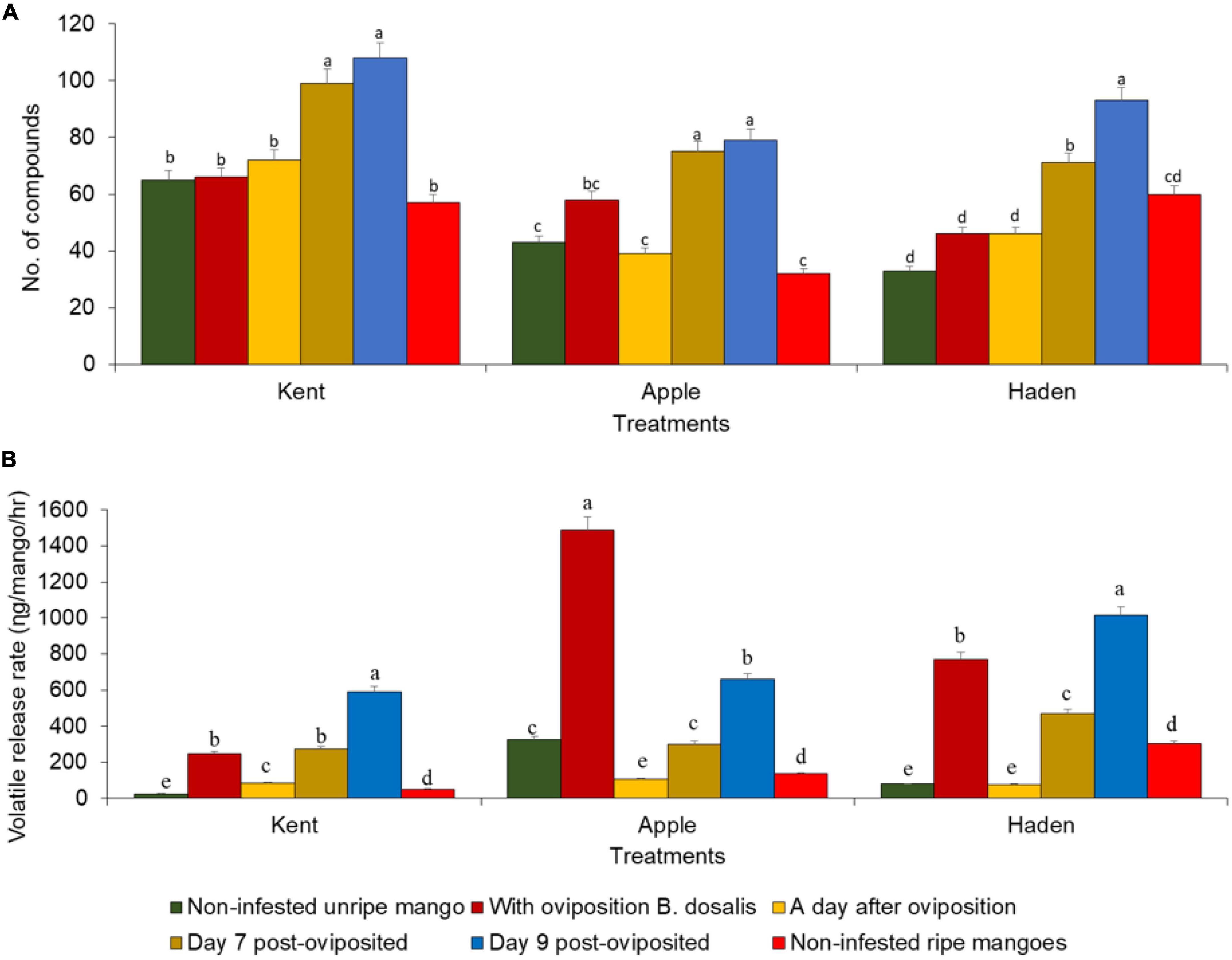
Figure 5. (A) The number of volatile organic compounds detected from the different mango varieties under the six different treatments. Bars capped with different letters, for the same mango variety, are significantly different (Pearson’s Chi-square test followed by Chi-square multi-comparison test in RVAideMemoire). (B) Totals of the average volatile release rates (ηg/mango/h) of the different mango treatments of the three varieties. Bars capped with different letters for the same mango variety are significantly different (Kruskal-Wallis rank-sum test followed by post hoc Dunn test for pairwise comparison).
The non-metric multidimensional scaling (NMDS) shows a significant difference among the treatments across the three mango varieties (k = 2, stress = 0.1218; one-way analysis of similarity, ANOSIM, R = 0.7245, P = 0.0001; Figure 6 and Supplementary Figure 5A).
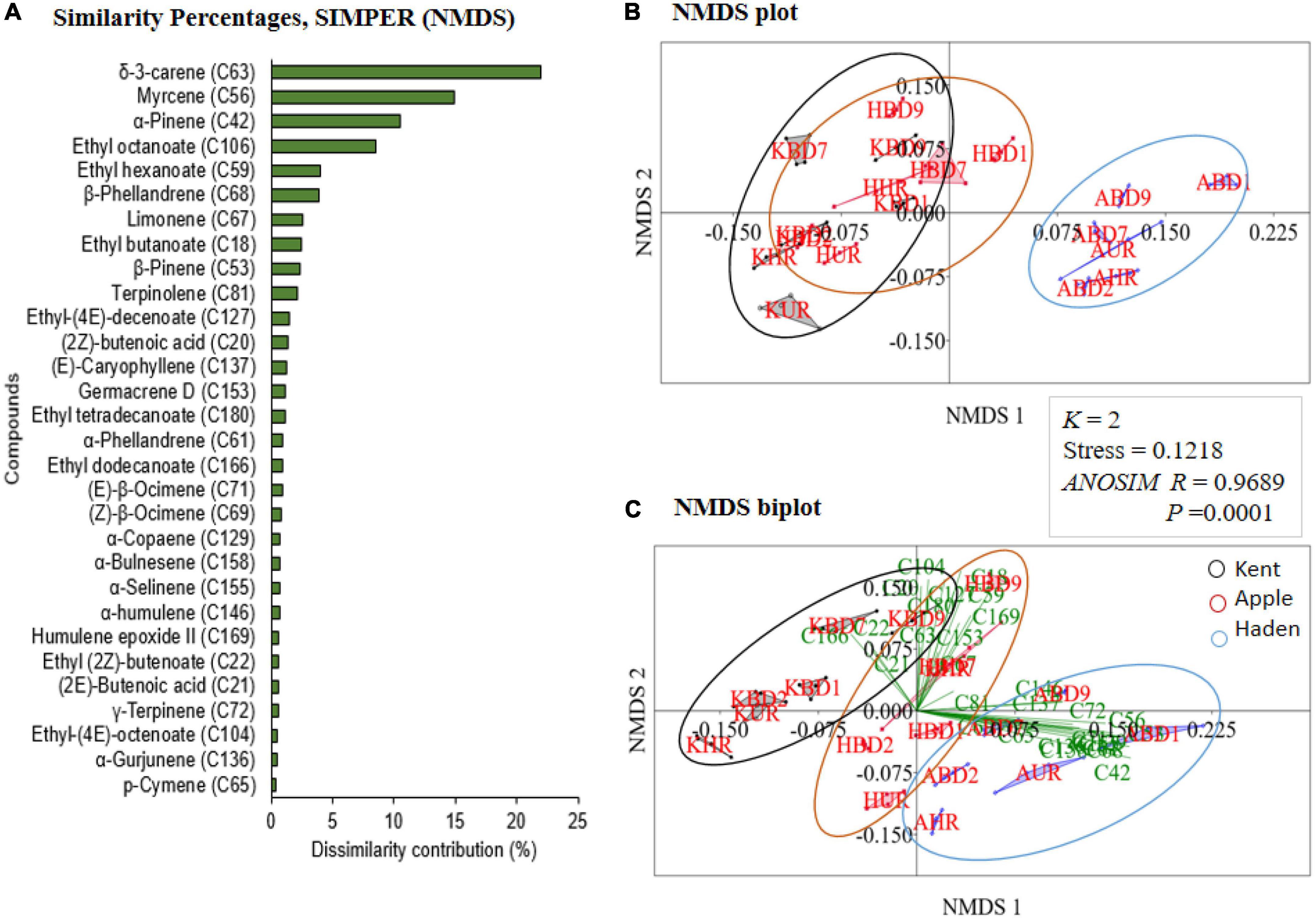
Figure 6. (A) Similarity percentage (SIMPER) of the non-metric multidimensional scaling (NMDS) showing the first 30 top most discriminant volatiles. (B) The NMDS plot shows the scattering of the treatments from the three varieties of mangoes. (C) The NMDS biplots for the differentiation of the discriminant volatiles in the treatments (K, Kent; A, Apple; H, Haden; BD, Bactrocera dorsalis; UR, non-infested unripe mango; HR, non-infested ripe mango; 1, 2, 7, and 9 = number of days from the day of B. dorsalis oviposition).
The 30 top most discriminant volatiles contributed 89.8% of the total dissimilarity contribution. The highest contributors and their percentage dissimilarity contributions were δ-3-carene (C63)-21.9, myrcene (C56)-14.9, α-pinene (C42)-10.6, ethyl octanoate (C106)-8.5, ethyl hexanoate (C59)-4.1, β-phellandrene (C68)-3.9, and limonene (C67)-2.6 (Figure 6A). Volatile compounds from the Apple mangoes were scattered separately unlike those of Kent and Haden mangoes which overlap at some points (Figure 6B). Furthermore, the 30 most discriminating volatiles are more associated with mango volatiles on the day of oviposition, day 7, and day 9 post-oviposited mangoes (Figure 6C).
Considering the treatments per mango variety, the multivariate analytical tool showed different discriminants of the volatile organic compounds (VOCs). The 30 top most discriminant volatiles among Kent mango volatiles as per the non-metric multidimensional scaling’s (NMDS) similarity percentages, SIMPER are graphically presented in Figure 7A where δ-3-carene, ethyl octanoate, ethyl hexanoate, ethyl-(4E)-decenoate, (2Z)-butenoic acid, ethyl dodecanoate, limonene, terpinolene, ethyl (2Z)-butenoate, (2E)-butenoic acid, ethyl butanoate, and myrcene contributed a total of 80.10% of the total dissimilarity.
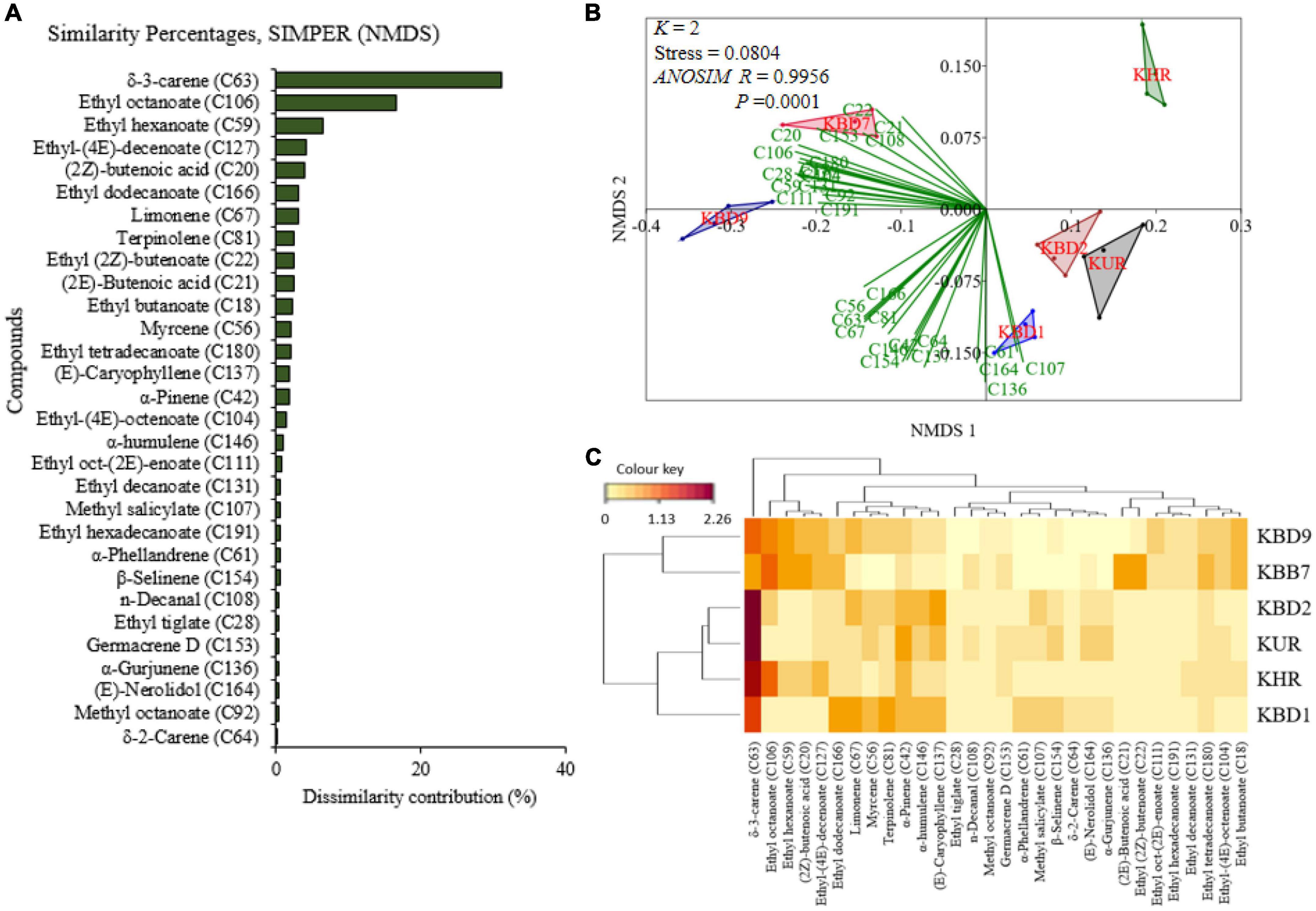
Figure 7. Panel (A) is the graphical representation of the 30 top most discriminant volatiles in their decreasing importance based on the non-metric multidimensional scaling’s (NMDS) similarity percentage (SIMPER). Panel (B) is the NMDS biplots for the differentiation of the 30 selected compounds and how they correlate to the mango treatments, while panel (C) is a heatmap clustering of the auto-scaled volatile release rate (y = log10x + 1) of the 30 compounds. The darker the brown color the higher the release rate (KBD1-Kent mango with ovipositing Bactrocera dorsalis; KHR-non-infested ripe Kent mango; KUR-non-infested unripe Kent mango; KBD2-Kent mango a day after oviposition; KBD7-day 7 post-oviposition KBD9-day 9 post-oviposited mangoes).
The NMDS biplots of the differentiation of the selected volatiles reveal that there were significant differences between the treatment headspaces (k = 2, stress = 0.08304; one-way analysis of similarity, ANOSIM, R = 0.9956, P = 0.0001; Figure 7B and Supplementary Figure 5B). More than 90% of the 30 selected compounds were associated with the volatiles emanating from the day of oviposition (KBD1), day 7 (KBD7), or day 9 (KBD9) post-oviposited mango fruits (Figure 7B). The heatmap clustering (Figure 7C) shows how the discriminating volatiles were spread in the treatments and the dendrograms show how they are correlated. Of the selected compounds, volatiles with dark brown color were released at higher rates. For example, δ-3-carene (C63) was released at a higher rate except on day 9 (Figure 7C).
Furthermore, there was a significant difference in the volatile release rates of non-infested unripe Kent mango (KUR), Kent mangoes on the day of oviposition (KBD1), and Kent mangoes a day after oviposition (KBD2) (χ2 = 27.17, df = 2, P < 0.001). In pairwise comparison, there was a significant difference between volatile release rates of KUR and KBD2 as well as KBD1 and KBD2 (P < 0.001) while there was no significant difference between KBD1 and KUR (P > 0.05). There were several-fold changes in the release rates of common volatiles on the day of oviposition (KBD1) and the day after oviposition (KBD2) compared to those of non-infested unripe Kent mangoes (KUR). The following are examples of some volatiles that were discriminant together with their number of fold changes, i.e., compound [no. of fold change on the day of oviposition (KBD1); no. of fold change a day after oviposition (KBD2)]: δ-3-carene (11.1; 18.8), limonene (19.2; 17.0), terpinolene (55.1; 27.7), ethyl dodecanoate (152.0; 34.0), and β-selinene (6.4; 6.6) (Figure 8A). On the other hand, there was a significant difference in the volatile release rates of non-infested ripe Kent mango (KHR), Kent mangoes on day 7 (KBD7), and day 9 (KBD9) post-oviposition (χ2 = 121.76, df = 2, P < 0.001). The pairwise comparison indicated a significant difference between KBD9 and KHR, KBD7 and KHR (P < 0.001), and KBD7 and KBD9 (P < 0.05; Figure 8B). There were changes in the release rates of common compounds on day 7 and day 9 post-oviposition headspaces compared to those of non-infested ripe mangoes (KHR). Examples of compounds that were discriminating with their quantities of fold change on day 7 and day 9 post-oviposition headspaces, respectively, compared to non-infested ripe mangoes were δ-3-carene (0.9; 6.7), limonene (1.0; 17.5), terpinolene (0.8; 15.4), β-selinene (2.3; 22.4), ethyl dodecanoate (15.4; 14.9), and ethyl hexadecanoate (79.0; 210.6) (Figure 8B). Other than changes in folds, 47 volatiles were only detected in the headspace of B. dorsalis-infested Kent mangoes, among them being pentanal, ethyl propanoate, methyl butanoate, ethyl 2-methyl propanoate, methyl tiglate, n-hexanol, methyl hexanoate, α-fenchene, and methyl (2E)-octenoate.
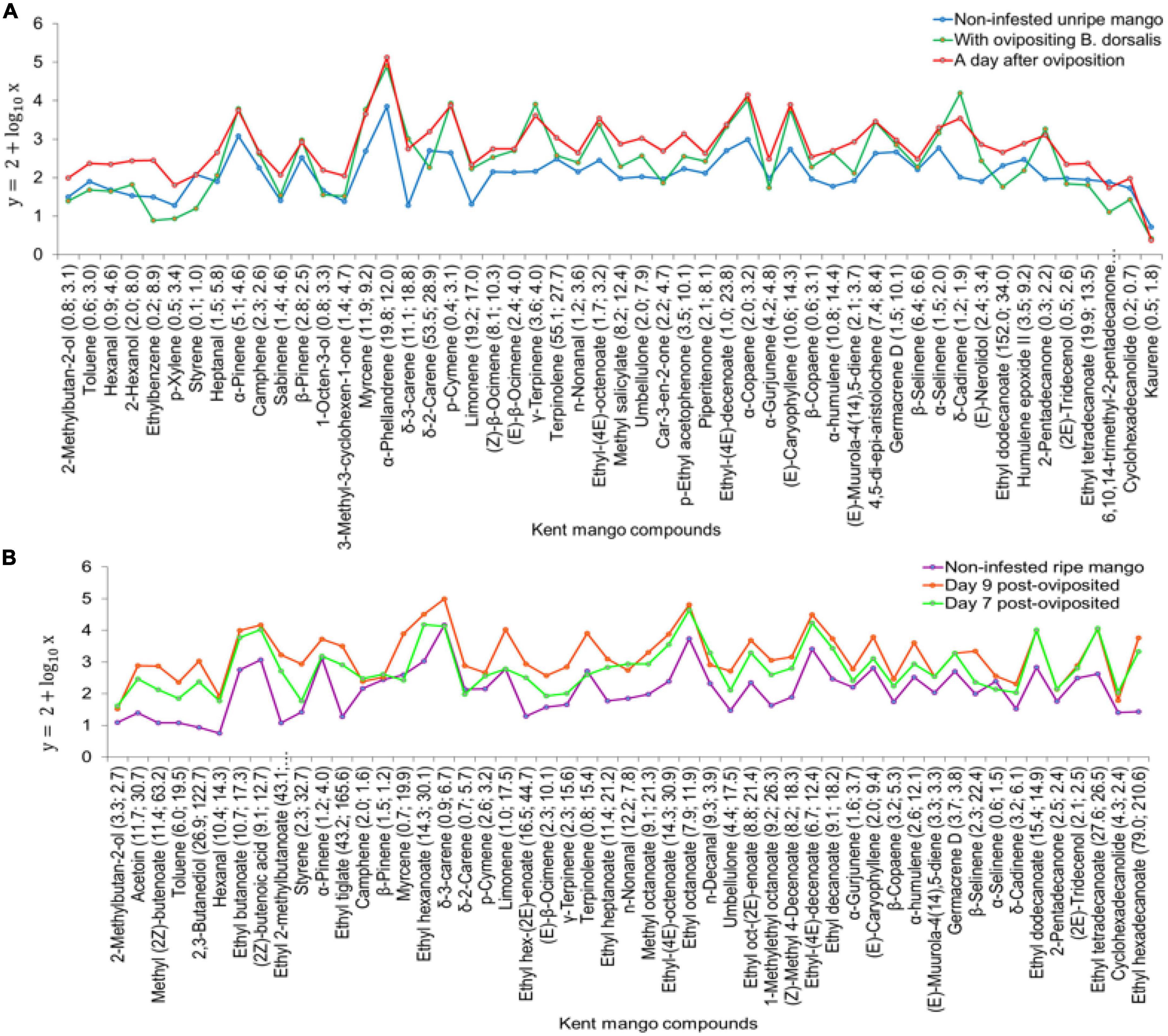
Figure 8. Trends in the change of volatile release rates of the common compounds in headspaces of; (A) non-infested unripe Kent mangoes, Kent mangoes with ovipositing Bactrocera dorsalis, and Kent mangoes a day after oviposition with the number of fold changes of the common compounds relative to those of non-infested unripe Kent mango. (B) Non-infested ripe Kent mangoes and B. dorsalis post-oviposited Kent mangoes on days 7 and 9 with their number of fold changes relative to those of non-infested ripe Kent mango.
For non-infested and B. dorsalis-infested Apple mangoes, the 30 top most discriminant volatiles as selected by the non-metric multidimensional scaling’s (NMDS) similarity percentages, SIMPER are graphically presented in Figure 9A. Of these compounds, myrcene, α-pinene, β-phellandrene, β-pinene, (Z)-β-ocimene, (E)-β-ocimene, α-phellandrene, α-bulnesene, α-selinene, ethyl octanoate, ethyl butanoate, and (E)-caryophyllene contributed 80.81% of the total dissimilarity. The 30 volatiles were used to construct NMDS biplots (Figure 9B) and heatmap (Figure 9C).
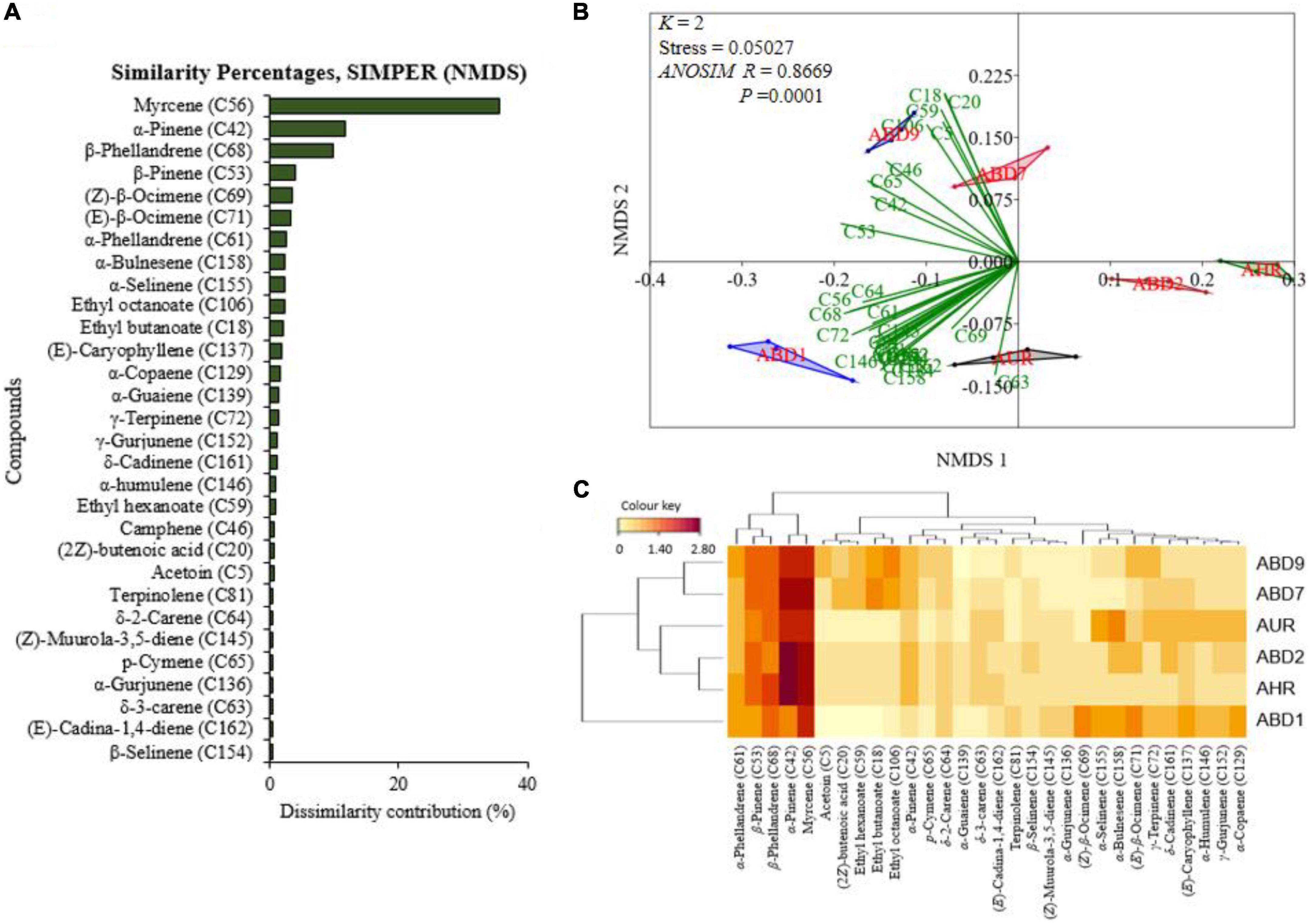
Figure 9. Panel (A) is a graphical representation of the 30 top most discriminant volatiles in their decreasing importance based on the non-metric multidimensional scaling’s (NMDS) similarity percentage (SIMPER). Panel (B) is NMDS biplots for the differentiation of the selected compounds and how they correlate to the mango treatments, while panel (C) is a heatmap clustering of the auto-scaled volatile release rate (y = log10x + 1) of the 30 discriminant compounds. The darker the brown color the higher the release rate (ABD1-Apple mango with ovipositing Bactrocera dorsalis; AHR-non-infested ripe Apple mango; ABD2-Apple mango a day after oviposition; AUR-non-infested unripe Apple mango; ABD7-day 7 post-oviposited; and ABD9-day 9 post-oviposited mangoes).
The NMDS (k = 2, stress = 0.05027) one-way analysis of similarity (ANOSIM, R = 0.8669, P = 0.0001) indicates there is a significant difference among the mango treatments’ volatile release rates (Figure 9B and Supplementary Figure 5C). Of the 30 discriminant compounds used, over 80% were associated with volatiles of mangoes on the day of oviposition (ABD1) or day 9 post-oviposited Apple mangoes (ABD9) (Figure 9B). The heatmap (Figure 9C) shows the distribution of the selected discriminant volatiles among the Apple mango treatments with their release rates corresponding to the intensity of brown color, e.g., the dark brown color of myrcene (C56) and α-pinene (C42) indicates that they had the highest release rates in most treatments (Figure 9C). The dendrograms also show the correlation of the volatiles within and between mango treatments.
There was a significant difference in the volatile release rates of non-infested unripe Apple mango (AUR), Apple mangoes on the day of oviposition (ABD1), and Apple mangoes a day after oviposition (ABD2) (χ2 = 44.5, df = 2, P < 0.001). On pairwise comparison, there were significant differences between ABD1 and ABD2 (P < 0.001), ABD1 and AUR (P < 0.001), and ABD2 and AUR (P < 0.05). There were changes in the quantities of common compounds in the volatiles on the day of oviposition (ABD1) and a day after oviposition (ABD2) relative to those of non-infested unripe mangoes (Figure 10A). Furthermore, there was a significant difference in the volatile release rates of non-infested ripe Apple mango (AHR), Apple mangoes on day 7 (KBD7), and day 9 post-oviposition (χ2 = 103.77, df = 2, P < 0.001). Pairwise comparison indicated significant differences between ABD7 and AHR (P < 0.001), ABD9 and AHR (P < 0.001), and ABD7 and ABD9 (P < 0.05). Most of the common compounds in the volatiles of day 7 and day 9 post-oviposited showed an increase in the number of folds relative to those of non-infested ripe mangoes (Figure 10B). A total of 52 volatiles including acetoin, ethyl propanoate, methyl butanoate, isopentyl formate, 2,3-butanediol, ethyl butanoate, (2Z)-butenoic acid, and ethyl 2-methyl butanoate were detected in headspaces of infested mangoes but not in non-infested.
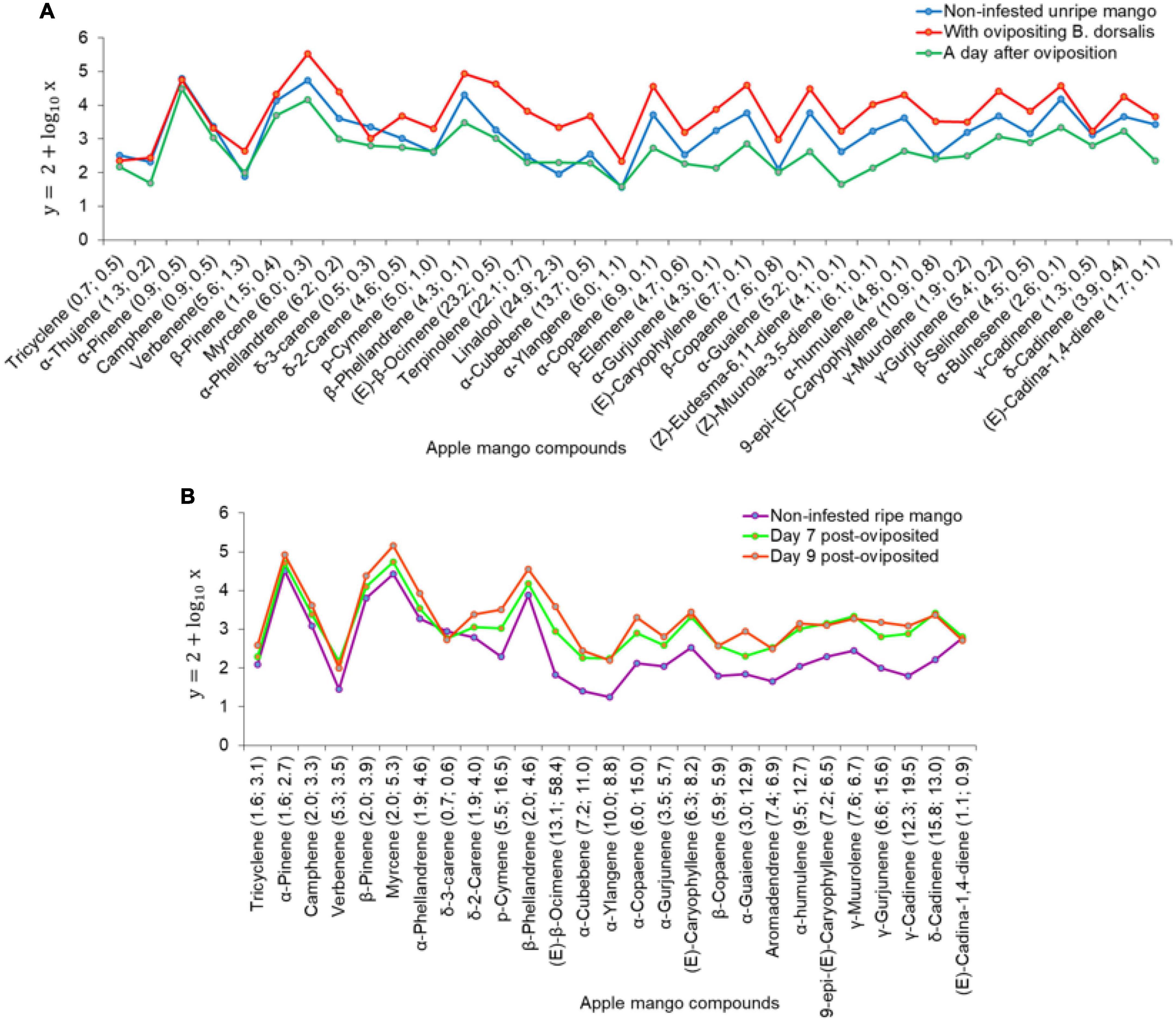
Figure 10. Trends in the change of volatile release rates of the common compounds in headspaces of; (A) non-infested unripe Apple mangoes, Apple mangoes with ovipositing Bactrocera dorsalis, and Apple mangoes a day after oviposition with the number of fold changes of the common compounds relative to those of non-infested unripe Apple mango. (B) Non-infested ripe Apple mangoes, and post-oviposited Apple mangoes on day 7 and day 9 with their number of fold changes relative to those of non-infested ripe Apple mango.
For non-infested and B. dorsalis-infested Haden mangoes, the 30 most discriminating volatiles of the headspaces as per NMDS’s SIMPER are presented in Figure 11A. Out of these compounds, δ-3-carene, ethyl octanoate, ethyl hexanoate, limonene, ethyl butanoate, terpinolene, myrcene, ethyl tetradecanoate, α-pinene, and humulene epoxide II contributed 78.28% of the total dissimilarity. The 30 most discriminant volatiles were used in plotting the NMDS biplots (Figure 11B) and heatmap (Figure 11C) for visualization of their distributions in the treatment headspaces.
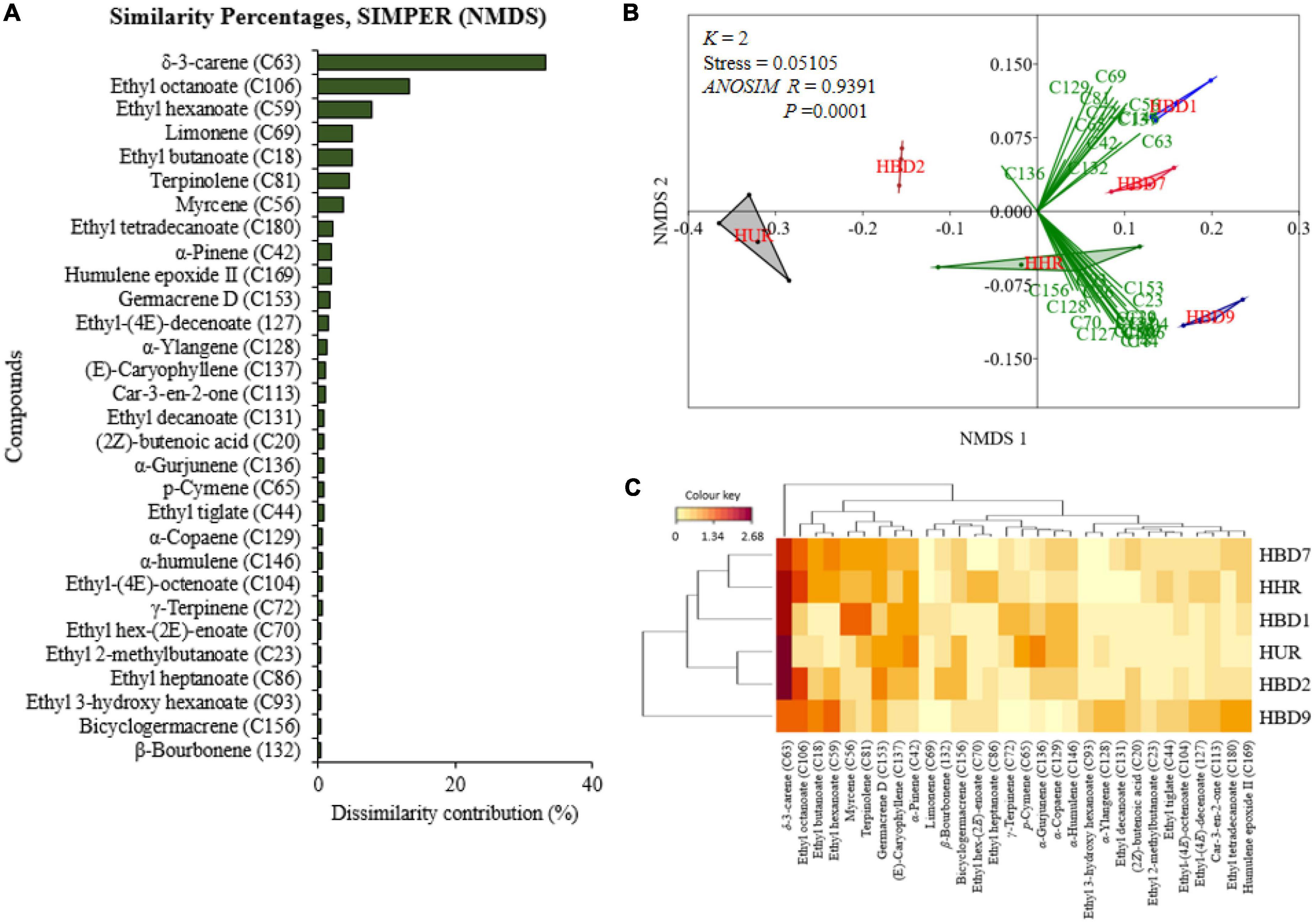
Figure 11. Panel (A) is a graphical representation of the 30 top most discriminant volatiles in their decreasing importance based on the non-metric multidimensional scaling’s (NMDS) similarity percentage (SIMPER). Panel (B) is NMDS biplots for the differentiation of the 30 selected compounds showing how they correlate to the mango treatments while (C) is a heatmap clustering of the auto-scaled volatile release rate(y = log10x + 1), of the compounds. The darker the brown color the higher the release rate (HBD9-day 9 post-oviposition; HBD2-Haden mango a day after oviposition; HUR-non-infested unripe Haden mango; HBD1-Haden mango with ovipositing Bactrocera dorsalis; HHR-non-infested ripe Haden mango, and HBD7-Haden mango on day 7 post-oviposition).
Like in Kent and Apple mangoes, the NMDS indicated a significant difference among the volatile release rates of the different treatments of Haden mangoes (k = 2, stress = 0.05105; one-way analysis of similarity, ANOSIM: R = 0.9391, P = 0.0001; Figure 11B and Supplementary Figure 5D). More than 75% of the selected discriminant volatiles were associated with volatiles of Haden mangoes with ovipositing B. dorsalis (HBD1), day 7 (HBD7), or day 9 (HBD9) post-oviposited Haden mango (Figure 11B). The heatmap clustering (Figure 11C) shows how the selected compounds were distributed in mango treatments while the dendrograms explain their correlation within and between treatments. The volatile whose release rate was high in Haden treatments was δ-3-carene hence having an intense brown color (Figure 11C).
On comparing the volatile release rates of non-infested unripe Haden mango (HUR), Haden mangoes on the day of oviposition (HBD1), and Haden mangoes a day after oviposition (HBD2), there was a significant difference (χ2 = 13.07, df = 2, P < 0.01). The pairwise comparison indicated a significant difference between HBD1 and HUR (P < 0.001) whereas there were no differences between HBD1 and HBD2, and HBD2 and HUR (P > 0.05). There were notable changes in the volatile release rates of common compounds of Haden mangoes with ovipositing B. dorsalis and Haden mangoes on the second day after oviposition relative to those of non-infested unripe Haden mangoes (Figure 12A). A significant difference was also found among volatile release rates of non-infested ripe Haden mango (HHR), Haden mangoes on day 7 (HBD7), and day 9 (KBD9) post-oviposition (χ2 = 21.66, df = 2, P < 0.001). The pairwise comparison revealed significant differences between HBD7 and HBD9 (P < 0.001), HBD9 and HHR (P < 0.001) while there was no significant difference between HBD7 and HHR (P > 0.05). There were changes in the quantities of common compounds detected on day 7 and day 9 of Haden mango volatiles relative to those of non-infested mangoes (Figure 12B). Other than changes in the abundance of common compounds, 46 volatiles among them methyl butanoate, isopentyl formate, 2-methyl-1-butanol, 2,3-butanediol, (2Z)-butenoic acid, 3-methylbutyl ethanoate, methyl hexanoate, α-fenchene, and 3-acetyl-2-octanone were detected only in B. dorsalis infested Haden mangoes.
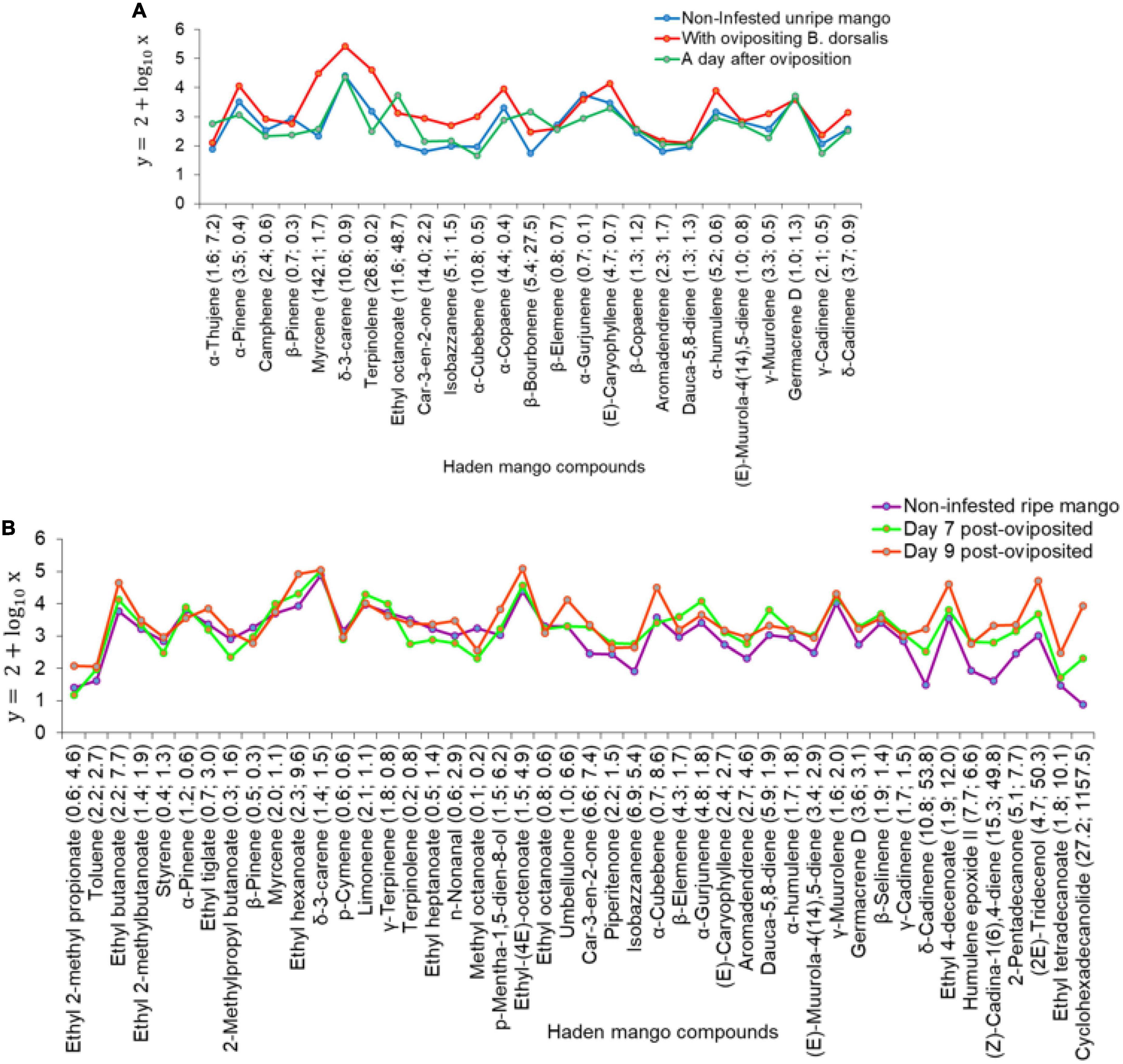
Figure 12. Trends in the change of volatile release rates of the common compounds in headspaces of; (A) non-infested unripe Haden mangoes, Haden mangoes with ovipositing Bactrocera dorsalis, and Haden mangoes a day after oviposition with the number of fold changes of the common compounds relative to those of non-infested unripe Haden mango. (B) Non-infested ripe Haden mangoes, Haden mangoes on day 7 and day 9 post-oviposition volatiles with their number of fold changes relative to those of non-infested ripe Haden mango.
In the first 10 most discriminant volatiles by the three multivariate analyses tools, (Z)-β-ocimene and ethyl octanoate were selected as discriminant volatiles in the three mango varieties while α-pinene, myrcene, ethyl hexanoate, δ-2-carene, (E)-β-ocimene, γ-terpinene, humulene epoxide II, δ-3-carene, limonene, and terpinolene were from two mango varieties.
Discussion
Behavioral assays of Bactrocera dorsalis and parasitoids to tree-attached non-infested and infested mangoes
A lot of emphases has been given to the investigation of volatiles of harvested fruits when trying to understand the behavioral dynamics of insects to their hosts (Milonas et al., 2019; Cai et al., 2020; Silva and Clarke, 2021). In our study, the behaviors of B. dorsalis and its parasitoids were conducted on headspace volatiles of tree-attached mangoes using a dual-choice olfactometer. In all assays, both B. dorsalis and the parasitoids were attracted differentially to the tree-attached mango volatiles compared to the clean air (control). The behavioral responses were highly influenced by the mango variety, the physiological state of the mango fruits, and the infestation status. Bactrocera dorsalis was attracted toward volatiles of mangoes with ovipositing B. dorsalis females and to conspecific infested mangoes relative to control. Possibly, odors from ovipositing conspecific females and/or mangoes signify suitable hosts, and damage that eases oviposition into the mango, respectively, as argued by Nishida (2014) and Masry et al. (2018). Similar findings were reported for the congenic Bactrocera zonata (Saunders) (Diptera: Tephritidae) females which were found to be highly attracted to volatiles of conspecific-infested guavas compared to a blank (control) (Binyameen et al., 2021). Conversely, Bactrocera tryoni (Froggatt) (Diptera: Tephritidae) did not discriminate between conspecific-infested or non-infested fruits (Silva and Clarke, 2021). In this study, except for non-infested unripe Kent mango, female B. dorsalis were attracted to the other non-infested unripe and ripe mangoes volatiles compared to the control. Roh et al. (2021) observed that B. dorsalis females which were ready to oviposit were highly attracted to the host odor. The attraction of B. dorsalis to ripe and unripe mangoes partly disagrees with the findings of Grechi et al. (2021) on B. zonata, Ceratitis capitata (Wiedemann), and C. quilicii (Karsch) (all Diptera: Tephritidae) which were attracted by volatiles of ripe mangoes but not of unripe ones.
As expected, the egg parasitoid F. arisanus was attracted to mangoes with ovipositing B. dorsalis which implies that mangoes with ovipositing fruit flies were emitting volatiles that increased attraction. Fopius arisanus has been reported to exploit the chemical stimuli emitted by the fruits after fruit fly oviposition (Pérez et al., 2013) and those resulting from the presence of the host fruit fly female (Wang and Messing, 2003) as well as the presence of fertile eggs (Pérez et al., 2013; Cai et al., 2020). Furthermore, F. arisanus was reported to prefer parasitizing host eggs that are in tree-attached fruits (Eitam and Vargas, 2007). We also found that volatiles of non-infested ripe mangoes attracted F. arisanus implying that the ripe fruits produce volatile that stimulate attraction. Similar observations were made by Altuzar et al. (2004). This indicates that the olfactory tuning of F. arisanus, and likely many other parasitoids, utilize volatiles emitted by the host of their host, i.e., environmental cues that enhance finding suitable tephritid hosts (Nanga Nanga et al., 2019; Mama Sambo et al., 2020; Ayelo et al., 2021).
The attraction of D. longicaudata to volatiles of B. dorsalis infested Apple and Haden mangoes, at both day 7 and 9 post-oviposited mango volatiles and not to volatiles of B. dorsalis-infested Kent mango signifies that the fruits with developing larvae produce attractive volatiles compared to those that do not have. Diachasmimorpha longicaudata were attracted to volatiles of mango fruits which were infested with larvae of Anastrepha ludens (Loew) (Diptera: Tephritidae), but not to mechanically damaged mangoes, suggesting that the presence of maturing larvae is of paramount importance for the parasitoid to be attracted (Carrasco et al., 2005; García-Medel et al., 2007). Furthermore, we found that D. longicaudata is more attracted to volatiles of infested mango at the older larval stage of B. dorsalis (day 9), which corroborates the finding by Harbi et al. (2019) on the parasitoid responses to volatiles of medfly, C. capitata-infested mango fruits tested at different infestation age, and fits with the ecological niche of the species as a larval parasitoid. Similarly, like F. arisanus, D. longicaudata was attracted to ripe non-infested fruit, indicating that this species’ olfactory circuitry has also evolved a sensitivity to environmental cues, i.e., fruit volatiles, that increases the chances of encountering tephritid hosts (Altuzar et al., 2004; Rousse et al., 2005). It would be interesting to find out how non-infested and infested tree-attached mango headspaces contribute to parasitoids locating their host larvae inside the fruit.
Performance of Bactrocera dorsalis on the different mango varieties
The discrepancy in the numbers of puparia harvested from the three varieties of mangoes indicates that the fruit fly B. dorsalis differs in its performance in the mango varieties as oviposition substrates. This observation is partially in support of the preference-performance hypothesis (PPH) which states, “female insects will evolve to oviposit on hosts on which their offspring fare best” (Gripenberg et al., 2010; Akol et al., 2013). There were no B. dorsalis puparia that were recovered from Kent mangoes though fresh oviposition punctures were observed on the day of oviposition which implies that the Kent variety is more tolerant to B. dorsalis which is further confirmed by its non-preference to volatiles of several treatments of the mango variety. These results corroborate the findings of Akol et al. (2013) who reported minimal preference and offspring survival of B. dorsalis in Kent mangoes compared to other mangoes that included Apple mango. A similar observation was reported for the peach fruit fly, B. zonata, which showed differential attraction and survival in different guava varieties (Binyameen et al., 2021). It has been reported that factors like the variety of fruit (Diatta et al., 2013; Kamala et al., 2014), the stage of fruit maturity (Yashoda et al., 2007), the ease of the fruit fly ovipositor penetrating the pericarp (Balagawi et al., 2005), and the chemical composition of the fruit and its ability to sustain the full development of the fruit fly (Boinahadji et al., 2020) affect the performance and survival of insect offspring. Apple mango constituted a better environment (223 puparia) for the fruit fly larvae development. Okoth et al. (2013) reported differences in pH, total titrable acid, and moisture content among other qualities between Kent and Apple mangoes which may play roles in the preference and performance of the B. dorsalis offspring in the latter variety. A further study on the chemical factors that are associated with the differential performance of B. dorsalis in Kent and apple mango would help in filling the knowledge gap of how the fly assesses the suitability of its hosts.
Headspace volatiles from all treatments of the three varieties of mangoes
In this study, δ-3-carene, myrcene, α-pinene, β-pinene, α-gurjunene, (E)-caryophyllene, β-copaene, α-humulene, and δ-cadinene, among other volatiles, were differentially released by the three mango varieties which were highly dependent on the status of the mango, i.e., unripe, ripe, non-infested, or infested. Some of the volatiles have been reported in earlier findings but not from tree-attached mangoes (Wetungu et al., 2018; Maldonado-Celis et al., 2019; Shimizu et al., 2021) and have been associated with the attraction of various insect pests (Benelli et al., 2014; Biasazin et al., 2014; Biasazin et al., 2019) and their natural enemies (Kamala et al., 2012; Segura et al., 2012; Harbi et al., 2019; Cai et al., 2020).
The stress values from all the two-dimensional NMDS plots indicated a good match between ordination fit and real data of the volatile release rates signifying a good fit of solution (Clarke, 1993). The qualitative and quantitative differences in headspace volatiles among the three mango varieties as revealed by the non-metric multidimensional scaling (NMDS) could be a result of differences in the genetic make-up (Lebrun et al., 2008). The qualitative and quantitative variability in headspace volatiles reported in this study corroborates with findings by other authors (e.g., El Hadi et al., 2013; Wetungu et al., 2018). The compounds selected by the multivariate tools were spread out in all categories of VOCs including the most abundant, common, those with significant quantitative changes, and most importantly the compounds emanating from the treatments that could have contributed to the behavioral responses of B. dorsalis and the two parasitoids.
Non-infested ripe mangoes produced more volatiles, the majority of which were esters, than non-infested unripe mangoes. These results are in agreement with other results from ripe and unripe mango fruits (Pandit et al., 2009; White et al., 2016). The number and release rates of monoterpenes and sesquiterpenes identified from the ripe mango of the three varieties were generally less compared to those of unripe mango. Monoterpenes are associated with the defense mechanisms of plants against herbivorous attack (Singh and Sharma, 2015; Olayemi, 2017), hence their decrease may explain the higher attraction of B. dorsalis to non-infested ripe fruits which appear more suitable for the survival of offspring. A study on the guava attractiveness of the Queensland fruit fly, B. tryoni showed that ripe guavas emitted volatiles that were more attractive than unripe ones (Cunningham et al., 2016). Although there was a minimum change in the number of compounds that were produced on the day of infestation on the three mango varieties, the volatile release rates of most volatiles increased significantly compared to those of non-infested mangoes. An increase in the volatile release rate, especially of terpenes, after an attack by herbivorous insects on any part of a plant, has been associated with defense against the herbivorous pest, and in some cases attraction of the pest’s natural enemies (parasitoids and predators) (War et al., 2011; Olayemi, 2017), but from our study, the increase in the release rate of volatiles lead to increased attraction of conspecific pests and the egg parasitoid F. arisanus. Similar observations for F. arisanus were made by Cai et al. (2020). There was an increase in the number of compounds and the volatile total emission on days 7 and 9 of post-oviposited mangoes relative to those of ripe mangoes. Common knowledge is that fruit ripens in preparation for seed dispersal but the difference in the number of compounds and their release rates of infested mangoes and non-infested ripe mangoes could be attributed to the activities of the mango trying to counter the attacks (Lackus et al., 2018; Sharifi et al., 2018), the activities of the fruit fly larvae in the mangoes, and/or introduction and activity of microbial in the mango (Futagbi et al., 2017). Herbivorous activities may result in the increase or decrease in quantities of compounds produced, formation of new compounds, or disappearance of some compounds as observed from different plant studies (War et al., 2011; Martins et al., 2017; Shivaramu et al., 2017). For example, on day 9 post-oviposited Apple mango headspace, an increase occurred in most common compounds while decreases were only slight for a few compounds. These changes could be responsible for the decrease in the attractiveness of B. dorsalis to the day 9 post-oviposited Apple mango and the generally increased attractiveness of the larva parasitoid D. longicaudata to the day 9 post-oviposited mangoes. Carrasco et al. (2005) reported that infestation of ‘Criollo’ (M. indica) with Anastrepha ludens (Loew) larvae changed the headspace composition and increased the attractiveness of the fruit for D. longicaudata. Similar results were reported by Segura et al. (2012), indicating that D. longicaudata is attracted to Ceratitis capitata (Wiedemann) (Diptera: Tephritidae) infested and non-infested oranges.
Conclusion and further research
The responses of the fruit fly B. dorsalis, the egg parasitoid F. arisanus, and the larval parasitoid D. longicaudata are highly influenced by the mango variety, the physiological, and the infestation status of the mango. This is evident from the behavioral response experiments and the number of puparia harvested from each variety of mangoes. The results indicate that Kent mango is highly tolerant to B. dorsalis hence deterring the fruit fly development while Apple is highly susceptible. The volatile organic compounds in the headspace of non-infested and B. dorsalis-infested mangoes are qualitatively and quantitatively different within and between treatments. This study thus describes the systematic changes which occur in the headspace volatiles of tree-attached mangoes before, during, and after infestation by B. dorsalis, and how this correlates with differential responses of the fruit fly B. dorsalis and its parasitoids, F. arisanus and D. longicaudata. Laboratory experiments have shown that parasitoids can distinguish between infested and non-infested harvested fruits, we, therefore, recommend further studies to assess whether the fruit fly and its parasitoids can distinguish between the headspaces of different treatments of infested and non-infested tree-attached mangoes. In addition, the studies should also determine whether the olfactory convergence of the insects is based on the detection of the same fruit volatile compounds. This is interesting from an evolutionary ecological perspective, but also of significance when developing baits that selectively attract the fly and not its natural enemies.
Data availability statement
The original contributions presented in this study are included in the article/Supplementary material, further inquiries can be directed to the corresponding authors.
Ethics statement
This research was licensed to be conducted in Kirinyaga County, Kenya, by the National Commission for Science, Technology, and Innovation (NACOSTI) under License No.: NACOSTI/P/20/6447. Consent was also sought from the farm owner to use his plantation of mangoes for the study purpose while other local farmers fully participated in the trials.
Author contributions
RM, TD, and SM conceptualized the research idea. RM collected and analyzed the data and drafted the preliminary manuscript. SM, XC, and SN supervised the study. All authors participated in providing intellectual inputs, proofreading, and approving the submission of the manuscript.
Funding
This work received financial support from the Norwegian Agency for Development Cooperation (NORAD), the Section for research, innovation, and higher education for the project “Combatting Arthropod Pests for better Health, Food and Climate Resilience (grant number: RAF-3058 KEN-18/0005)” and International Development Research Centre (IDRC) and the Australian Centre for International Agricultural Research (ACIAR) for the project “Alien invasive fruit flies in Southern Africa: Implementation of a sustainable IPM program to combat their menaces (grant number: 109040)”. Also, the authors gratefully acknowledge the financial support for this research by the following organizations and agencies: The Swedish International Development Cooperation Agency (SIDA); the Swiss Agency for Development and Cooperation (SDC); the Federal Democratic Republic of Ethiopia; and the Government of the Republic of Kenya. RM was sponsored by NORAD (grant number: RAF-3058 KEN-18/0005).
Acknowledgments
The authors gratefully acknowledge Pascal Mahukpe Ayelo for assistance with statistical analysis.
Conflict of interest
The authors declare that the research was conducted in the absence of any commercial or financial relationships that could be construed as a potential conflict of interest.
Publisher’s note
All claims expressed in this article are solely those of the authors and do not necessarily represent those of their affiliated organizations, or those of the publisher, the editors and the reviewers. Any product that may be evaluated in this article, or claim that may be made by its manufacturer, is not guaranteed or endorsed by the publisher.
Supplementary material
The Supplementary Material for this article can be found online at: https://www.frontiersin.org/articles/10.3389/fevo.2022.1021795/full#supplementary-material
References
Adams, R. P. (1995). Identification Of Essential Oil Components By Gas Chromatography/Mass Spectroscopy. Carol Stream: Allured Pub. Corp.
Akol, A. M., Masembe, C., Isabirye, B. E., Kukiriza, C. K., and Rwomushana, I. (2013). Oviposition preference and offspring performance in phytophagous fruit flies (Diptera: Tephritidae): The african invader, Bactrocera invadens. Int. Res. J. Hortic. 1, 1–14. doi: 10.12966/irjh.05.01.2013
Akotsen-Mensah, C., Ativor, I. N., Anderson, R. S., Afreh-Nuamah, K., Brentu, C. F., Osei-Safo, D., et al. (2017). Pest management knowledge and practices of mango farmers in Southeastern Ghana. J. Integr. Pest Manag. 8:13. doi: 10.1093/jipm/pmx008
Altuzar, A., Montoya, P., and Rojas, J. C. (2004). Response of Fopius arisanus (Hymenoptera: Braconidae) to fruit volatiles in a wind tunnel. Fla. Entomol. 87, 616–618.
Ayelo, P. M., Yusuf, A. A., Pirk, W. W., Chailleux, A., Mohamed, S. A., and Deletre, E. (2021). Terpenes from herbivore-induced tomato plant volatiles attract Nesidiocoris tenuis (Hemiptera: Miridae), a predator of major tomato pests. Pest Manag. Sci. 77, 5255–5267. doi: 10.1002/ps.6568
Badii, K. B., Billah, M. K., Afreh-Nuamah, K., Obeng-Ofori, D., and Nyarko, G. (2016). Preliminary inventory of hymenopteran parasitoids associated with fruit-infesting flies (Diptera: Tephritidae) in Northern Ghana. Int. J. Pest Manag. 62, 267–275. doi: 10.1080/09670874.2016.1174318
Balagawi, S., Vijaysegaran, S., Drew, R. A. I., and Raghu, S. (2005). Influence of fruit traits on oviposition preference and offspring performance of Bactrocera tryoni (froggatt) (Diptera: Tephritidae) on three tomato (Lycopersicon lycopersicum) cultivars. Aust. J. Entomol. 44, 97–103.
Benelli, G., Daane, K. M., Canale, A., Niu, C. Y., Messing, R. H., and Vargas, R. I. (2014). Sexual communication and related behaviours in Tephritidae: Current knowledge and potential applications for integrated pest management. J. Pest Sci. 87, 385–405. doi: 10.1007/s10340-014-0577-3
Biasazin, T. D., Chernet, H. T., Herrera, S. L., Bengtsson, M., Karlsson, M. F., Lemmen-Lechelt, J. K., et al. (2018). Detection of volatile constituents from food lures by tephritid fruit flies. Insects 9:119. doi: 10.3390/insects9030119
Biasazin, T. D., Karlsson, M. F., Hillbur, Y., Seyoum, E., and Dekker, T. (2014). Identification of host blends that attract the African invasive fruit fly, Bactrocera invadens. J. Chem. Ecol. 40, 966–976. doi: 10.1007/s10886-014-0501-6
Biasazin, T. D., Larsson Herrera, S., Kimbokota, F., and Dekker, T. (2019). Translating olfactomes into attractants: Shared volatiles provide attractive bridges for polyphagy in fruit flies. Ecol. Lett. 22, 108–118. doi: 10.1111/ele.13172
Binyameen, M., and Anderson, P. (2014). Identification of plant semiochemicals and characterization of new olfactory sensory neuron types in a polyphagous pest moth, Spodoptera littoralis. Chemical 39, 719–733. doi: 10.1093/chemse/bju046
Binyameen, M., Hamid, A., Afzal, I., Sajjad, M., Azeem, M., Muhammad, S., et al. (2021). Role of fruit volatiles of different guava varieties in attraction and oviposition behaviors of peach fruit fly, Bactrocera zonata saunders. Arthropod Plant Interact. 15, 95–106. doi: 10.1007/s11829-020-09796-z
Boinahadji, A. K., Coly, E. V., Diedhiou, C. A., and Sembene, P. M. (2020). Oviposition preference and offspring performance of the oriental fruit fly Bactrocera dorsalis (Diptera, Tephritidae) on eight host plants. Int. J. Adv. Res. 8, 931–937. doi: 10.21474/ijar01/10384
Cai, P., Song, Y., Huo, D., Lin, J., Zhang, H., and Zhang, Z. (2020). Chemical cues induced from fly-oviposition mediate the host-seeking behaviour of an effective egg parasitoid of Bactrocera dorsalis (Diptera: Tephritidae), within a tritrophic context. Insects 11:231. doi: 10.3390/insects11040231
Carrasco, D., Larsson, M. C., and Anderson, P. (2015). Insect host plant selection in complex environments. Curr. Opin. Insect Sci. 8, 1–7.
Carrasco, M., Montoya, P., Cruz-Lopez, L., and Rojas, J. C. (2005). Response of the fruit fly parasitoid Diachasmimorpha longicaudata (Hymenoptera: Braconidae) to mango fruit volatiles. Environ. Entomol. 34, 576–583. doi: 10.1603/0046-225X-34.3.576
Cheseto, X., Kachigamba, D. L., Ekesi, S., Ndung’u, M., Teal, P. E. A., Beck, J. J., et al. (2017). Identification of the ubiquitous antioxidant tripeptide glutathione as a fruit fly semiochemical. J. Agric. Food Chem. 65, 8560–8568. doi: 10.1021/acs.jafc.7b03164
Clarke, K. R. (1993). Non-parametric multivariate analysis of changes in community structure. Austral J. Ecol. 18, 117–143. doi: 10.1111/j.1442-9993.1993.tb00438.x
Copeland, R. S., Wharton, R. A., Luke, Q., De Meyer, M., Lux, S., Zenz, N., et al. (2006). Geographic distribution, host fruit, and parasitoids of African fruit fly pests Ceratitis anonae, Ceratitis cosyra, Ceratitis fasciventris, and Ceratitis rosa (Diptera: Tephritidae) in Kenya. Ann. Entomol. Soc. Am. 99, 261–278.
Cunningham, J. P., Carlsson, M. A., Villa, T. F., Dekker, T., and Clarke, A. R. (2016). Do fruit ripening volatiles enable resource specialism in polyphagous fruit flies? J. Chem. Ecol. 42, 931–940. doi: 10.1007/s10886-016-0752-5
Diatta, P., Rey, J. Y., Vayssieres, J. F., Diarra, K., Coly, E. V., Lechaudel, M., et al. (2013). Fruit phenology of citruses, mangoes and papayas influences egg-laying preferences of Bactrocera invadens (Diptera: Tephritidae). Fruits 68, 507–516. doi: 10.1051/fruits/2013093
Díaz-Fleischer, F., Pérez-Staples, D., Cabrera-Mireles, H., Montoya, P., and Liedo, P. (2017). Novel insecticides and bait stations for the control of Anastrepha fruit flies in mango orchards. J. Pest Sci. 90, 865–872. doi: 10.1007/s10340-017-0834-3
Dinno, A. (2015). Nonparametric pairwise multiple comparisons in independent groups using Dunn’s test. Stata J. 15, 292–300. doi: 10.1177/1536867X1501500117
Doorenweerd, C., Leblanc, L., Norrbom, A. L., Jose, M. S., and Rubinoff, D. (2018). A global checklist of the 932 fruit fly species in the tribe Dacini (Diptera, Tephritidae). ZooKeys 730, 19–56. doi: 10.3897/zookeys.730.21786
Eitam, A., and Vargas, R. I. (2007). Host habitat preference of Fopius arisanus (Hymenoptera: Braconidae), a parasitoid of tephritid fruit flies. Ann. Entomol. Soc. Am. 100, 603–608.
Ekesi, S., and Mohamed, S. A. (2011). “Mass Rearing and Quality Control Parameters for Tephritid Fruit Flies of Economic Importance in Africa,” in Wide Spectra of Quality Control, ed. I. Akyar (Rang-Du-Fliers: InTech), doi: 10.5772/21330
Ekesi, S., Mohamed, S. A., and De Meyer, M. (2016). “Fruit fly research and development in Africa-Towards a sustainable management strategy to improve horticulture,” in Fruit Fly Research and Development in Africa - Towards a Sustainable Management Strategy to Improve Horticulture, eds S. Ekesi, S. A. Mohamed, and M. De Meyer (Berlin: Springer), doi: 10.1007/978-3-319-43226-7
El Hadi, M. A. M., Zhang, F. J., Wu, F. F., Zhou, C. H., and Tao, J. (2013). Advances in fruit aroma volatile research. Molecules 18, 8200–8229. doi: 10.3390/molecules18078200
Flávio, R. M. G., Ovrusk, S. M., Suárez, L., Cancino, J., and Liburd, O. E. (2020). Biological control of tephritid fruit flies in the Americas and Hawaii: A Review of the use of parasitoids and predators. J. Insects 11:662. doi: 10.3390/insects11100662
Fraenkel, G. (1969). Evaluation of our thoughts on secondary plant substances. Entomol. Exper. Appl. 12, 473–486.
Futagbi, G., Koduah, N. A. G., Ampah, B. R., Mattah, P. A. D., Billah, M., Futse, J. E., et al. (2017). Microbial carriage and contamination of mangoes by the Oriental fruit fly. Open Public Health J. 10, 267–275. doi: 10.2174/1874944501710010267
García-Medel, D., Sivinski, J., Díaz-Fleischer, F., Ramirez-Romero, R., and Aluja, M. (2007). Foraging behavior by six fruit fly parasitoids (Hymenoptera: Braconidae) released as single- or multiple-species cohorts in field cages: Influence of fruit location and host density. Biol. Control 43, 12–22. doi: 10.1016/j.biocontrol.2007.06.008
Gripenberg, S., Mayhew, P. J., Parnell, M., and Roslin, T. (2010). A meta-analysis of preference-performance relationships in phytophagous insects. Ecol. Lett. 13, 383–393. doi: 10.1111/j.1461-0248.2009.01433.x
Guarino, S., Arif, M. A., Millar, J. G., Colazza, S., and Peri, E. (2018). Volatile unsaturated hydrocarbons emitted by seedlings of Brassica species provide host location cues to Bagrada hilaris. PLoS One 13:e0209870. doi: 10.1371/journal.pone.0209870
Hammer, D., Harper, D. A. T., and Ryan, P. D. (2001). PAST: Paleontological statistics software package for education and data analysis PAST. Palaeontol. Electron. 4:9.
Harbi, A., De Pedro, L., Ferrara, F. A. A., Tormos, J., Chermiti, B., Beitia, F., et al. (2019). Diachasmimorpha longicaudata parasitism response to medfly host fruit and fruit infestation age. Insects 10:211. doi: 10.3390/insects10070211
Kamala, J. D., Woodcock, P. M. C., Caulfield, J., Birkett, M. A., and Bruce, T. J. A. (2012). Isolation and identification of host cues from mango, Mangifera indica, that attract gravid female oriental fruit fly, Bactrocera dorsalis. J. Chem. Ecol. 38, 361–369. doi: 10.1007/s10886-012-0093-y
Kamala, J. D. P., Kempraj, V., Ravindra, M. A., Venkataramanappa, K. R., Nandagopal, B., Verghese, A., et al. (2014). Specific volatile compounds from mango elicit oviposition in gravid Bactrocera dorsalis females. J. Chem. Ecol. 40, 259–266. doi: 10.1007/s10886-014-0403-7
Lackus, N. D., Lackner, S., Gershenzon, J., Unsicker, S. B., and Köllner, T. G. (2018). The occurrence and formation of monoterpenes in herbivore-damaged poplar roots. Sci. Rep. 8:17936. doi: 10.1038/s41598-018-36302-6
Lebrun, M., Plotto, A., Goodner, K., Ducamp, M. N., and Baldwin, E. (2008). Discrimination of mango fruit maturity by volatiles using the electronic nose and gas chromatography. Postharvest Biol. Technol. 48, 122–131. doi: 10.1016/j.postharvbio.2007.09.010
Maldonado-Celis, M. E., Yahia, E. M., Bedoya, R., Landázuri, P., Loango, N., Aguillón, J., et al. (2019). Chemical composition of mango (Mangifera indica L.) Fruit: Nutritional and phytochemical compounds. Front. Plant Sci. 10:1073. doi: 10.3389/fpls.2019.01073
Malo, E. A., Gallegos-torres, I., Toledo, J., Valle-mora, J., and Rojas, J. C. (2012). Attraction of the West Indian fruit fly to mango fruit volatiles. Entomol. Exp. Appl. 142, 45–52. doi: 10.1111/j.1570-7458.2011.01200.x
Mama Sambo, S., Togbé, D. R., Sinzogan, A. A. C., Adomou, A., Bokonon-Ganta, H. A., and Karlsson, M. F. (2020). Habitat factors associated with Fopius caudatus parasitism and population level of its host, Ceratitis cosyra. Entomol. Exp. Appl. 168, 28–40. doi: 10.1111/eea.12858
Manoukis, N., Geib, S., Seo, D., McKenney, M., Vargas, R., and Jang, E. (2011). An optimized protocol for rearing Fopius arisanus, a parasitoid of Tephritid fruit flies. J. Vis. Exp. 53:2901. doi: 10.3791/2901
Martins, C. B. C., Vidal, D. M., Gomes, S. M. S., and Zarbin, P. H. G. (2017). Volatile organic compounds (VOCs) emitted by Ilex paraguariensis plants are affected by the herbivory of the Lepidopteran Thelosia camina and the Coleopteran Hedypathes betulinus. J. Braz. Chem. Soc. 28, 1204–1211.
Masry, A., Clarke, A. R., and Cunningham, J. P. (2018). Learning influences host versus nonhost discrimination and postalighting searching behavior in the tephritid fruit fly parasitoid Diachasmimorpha kraussii (Hymenoptera: Braconidae). J. Econ. Entomol. 111, 787–794. doi: 10.1093/jee/toy033
Metcalf, R. L., and Kogan, M. (1987). Plant volatiles as insect attractants. Crit. Rev. Plant Sci. 5, 251–301.
Miano, R. N., Ayelo, P. M., Musau, R., Hassanali, A., and Mohamed, S. A. (2022). Electroantennogram and machine learning reveal a volatile blend mediating avoidance behavior by Tuta absoluta females to a wild tomato plant. Sci. Rep. 12:8965. doi: 10.1038/s41598-022-13125-0
Milonas, P. G., Anastasaki, E., and Partsinevelos, G. (2019). Oviposition-induced volatiles affect electrophysiological and behavioral responses of egg parasitoids. Insects 10:437. doi: 10.3390/insects10120437
Mohamed, S. A., Ekesi, S., and Hanna, R. (2008). Evaluation of the impact of Diachasmimorpha longicaudata on Bactrocera invadens and five African fruit fly species. J. Appl. Entomol. 132, 789–797. doi: 10.1111/j.1439-0418.2008.01350.x
Mohamed, S. A., Ekesi, S., and Hanna, R. (2010). Old and new host-parasitoid associations: Parasitism of the invasive fruit fly Bactrocera invadens (Diptera: Tephritidae) and five African fruit fly species by Fopius arisanus, an Asian opiine parasitoid. Biocontrol Sci. Technol. 20, 183–196. doi: 10.1080/09583150903447794
Mohamed, S. A., Ramadan, M. M., and Ekesi, S. (2016). “In and Out of Africa: Parasitoids used for biological control of fruit flies,” in Fruit Fly Research and Development in Africa - Towards a Sustainable Management Strategy to Improve Horticulture, eds S. Ekesi, S. A. Mohamed, and M. De Meyer (Berlin: Springer International Publishing), 325–368. doi: 10.1007/978-3-319-43226-7
Nanga Nanga, S., Hanna, R., Gnanvossou, D., Fotso Kuate, A., Fiaboe, K. K. M., Djieto-Lordon, C., et al. (2019). Fruit preference, parasitism, and offspring fitness of Fopius arisanus (Hymenoptera: Braconidae) exposed to Bactrocera dorsalis’ (Diptera: Tephritidae) infested fruit species. Environ. Entomol. 48, 1286–1296. doi: 10.1093/ee/nvz114
Nankinga, C. M., Isabirye, B. E., Muyinza, H., Rwomushana, I., Stevenson, P. C., and Mayamba, A. (2014). Fruit fly infestation in mango: A threat to the Horticultural sector in Uganda. Uganda J. Agric. Sci. 15, 1–14.
Ndlela, S., Mohamed, S., Ndegwa, P. N., Amo, G. O., and Ekesi, S. (2016). Male annihilation technique using methyl eugenol for field suppression of Bactrocera dorsalis (Hendel) (Diptera: Tephritidae) on mango in Kenya. Afr. Entomol. 24, 437–447.
Ndlela, S., Mohamed, S. A., Azrag, A. G. A., Ndegwa, P. N., Ong’amo, G. O., and Ekesi, S. (2020). Interactions between two parasitoids of Tephritidae: Diachasmimorpha longicaudata (Ashmead) and psyttalia cosyrae (Wilkinson) (Hymenoptera: Braconidae), under laboratory conditions. Insects 11:671. doi: 10.3390/insects11100671
Nishida, R. (2014). Chemical ecology of insect-plant interactions: Ecological significance of plant secondary metabolites. Biosci. Biotechnol. Biochem. 78, 1–13. doi: 10.1080/09168451.2014.877836
Njuguna, P. K., Murungi, L. K., Fombong, A., Teal, P. E. A., Beck, J. J., and Torto, B. (2018). Cucumber and tomato volatiles: Influence on attraction in the melon fly Zeugodacus cucurbitate (Diptera: Tephritidae) [Research-article]. J. Agric. Food Chem. 66, 8504–8513. doi: 10.1021/acs.jafc.8b03452
Nyasembe, V. O., Teal, P. E. A., Mukabana, W. R., Tumlinson, J. H., and Torto, B. (2012). Behavioural response of the malaria vector Anopheles gambiae to host plant volatiles and synthetic blends. Parasit. Vectors 5:234. doi: 10.1186/1756-3305-5-234
Okoth, E., Sila, D., Onyango, C. A., Owino, W., Musyimi, S., and Mathooko, F. M. (2013). Evaluation of chemical and nutritional quality attributes of selected mango varieties at three stages of ripeness, grown in lower Eastern province of Kenya – part 2. J. Anim. Plant Sci. 17, 2619–2630.
Olayemi, R. F. (2017). The role of monoterpenoids and sesqiterpenoids as defense chemicals in plants – a Review. Nigerian Res. J. Chem. Sci. 3, 1–15.
Ormeño, E., Goldstein, A., and Niinemets, Ü (2011). Extracting and trapping biogenic volatile organic compounds stored in plant species. Trends Anal. Chem. 30, 978–989. doi: 10.1016/j.trac.2011.04.006
Pandit, S. S., Kulkarni, R. S., Chidley, H. G., Giri, A. P., Pujari, K. H., Tobias, G. K., et al. (2009). Changes in volatile composition during fruit development and ripening of ‘Alphonso’ mango. J. Sci. Food Agric. 89, 2071–2081. doi: 10.1002/jsfa.3692
Pérez, J., Rojas, J. C., Montoya, P., Liedo, P., and Castillo, A. (2013). Anastrepha egg deposition induces volatiles in fruits that attract the parasitoid Fopius arisanus. Bull. Entomol. Res. 103, 318–325. doi: 10.1017/S0007485312000739
Roger, V. I., Leblanc, L., Harris, E. J., and Manoukis, N. C. (2012). Regional suppression of bactrocera fruit flies (Diptera: Tephritidae) in the pacific through biological control and prospects for future introductions into other areas of the world. Insects 3, 727–742. doi: 10.3390/insects3030727
Roh, G. H., Kendra, P. E., and Cha, D. H. (2021). Preferential attraction of oviposition-ready oriental fruit flies to host fruit odor over protein food odor. Insects 12:909. doi: 10.3390/insects12100909
Rohart, F., Gautier, B., Singh, A., and Lê Cao, K. A. (2017). mixOmics: An R package for ‘omics feature selection and multiple data integration. PLoS Comput. Biol. 13:e1005752. doi: 10.1371/journal.pcbi.1005752
Rousse, P., Harris, E. J., and Quilici, S. (2005). Fopius arisanus, an egg – pupal parasitoid of Tephritidae an overview. Biocontrol N. Inform. 26, 59–69.
Scolari, F., Valerio, F., Benelli, G., Papadopoulos, N. T., and Vaníèková, L. (2021). Tephritid fruit fly semiochemicals: Current knowledge and future perspectives. Insects 12:408. doi: 10.3390/insects12050408
Segura, D. F., Viscarret, M. M., Ovruski, S. M., and Cladera, J. L. (2012). Response of the fruit fly parasitoid Diachasmimorpha longicaudata to host and host-habitat volatile cues. Entomol. Exp. Appl. 143, 164–176. doi: 10.1111/j.1570-7458.2012.01246.x
Shahbandeh, M. (2021). Global Mango Production 2000-2019. Available Online at: https://www.statista.com/statistics/577951/world-mango-production/ (accessed on Sep 27, 2021).
Sharifi, R., Lee, S. M., and Ryu, C. M. (2018). Microbe-induced plant volatiles. N. Phytol. 220, 684–691. doi: 10.1111/nph.14955
Shimizu, K., Matsukawa, T., Kanematsu, R., Itoh, K., Kanzaki, S., Shigeoka, S., et al. (2021). Volatile profiling of fruits of 17 mango cultivars by HS-SPME-GC/MS combined with principal component analysis. Biosci. Biotechnol. Biochem. 85, 1789–1797. doi: 10.1093/bbb/zbab097
Shivaramu, S., Jayanthi, P. D. K., Kempraj, V., Anjinappa, R., Nandagopal, B., and Chakravarty, A. K. (2017). What signals do herbivore-induced plant volatiles provide conspecific herbivores? Arthropod Plant Interact. 11, 815–823. doi: 10.1007/s11829-017-9536-2
Silva, R., and Clarke, A. R. (2021). Aversive responses of Queensland fruit flies towards larval-infested fruits are modified by fruit quality and prior experience. J. Insect Physiol. 131:104231. doi: 10.1016/j.jinsphys.2021.104231
Singh, B., and Sharma, R. A. (2015). Plant terpenes: Defense responses, phylogenetic analysis, regulation and clinical applications. 3 Biotech 5, 129–151. doi: 10.1007/s13205-014-0220-2
Stringer, L. D., Soopaya, R., Butler, R. C., Vargas, R. I., Souder, S. K., Jessup, A. J., et al. (2019). Effect of lure combination on fruit fly surveillance sensitivity. Sci. Rep. 9:2653. doi: 10.1038/s41598-018-37487-6
van Den Dool, H., and Kratz, P. D. (1963). A generalization of the retention index system incruding linear temperature programmed gas-liquid partition chromatography. J. Chromatogr. 11, 463–471. doi: 10.1016/s0021-9673(01)80947-x
Wang, X., and Messing, R. H. (2003). Foraging behavior and patch time allocation by Fopius arisanus (Hymenoptera: Braconidae), an egg-larval parasitoid of tephritid fruit flies. J. Insect Behav. 16, 593–612.
War, A. R., Sharma, H. C., Paulraj, M. G., War, M. Y., and Ignacimuthu, S. (2011). Herbivore induced plant volatiles:Their role in plant defense for pest management. Plant Signal. Behav. 6, 1973–1978. doi: 10.4161/psb.6.12.18053
Wetungu, M. W., Omolo, M. V. O., Tarus, P. K., and Fredrick, K. (2018). Volatile aroma chemical constituents of fruit pulp of some Kenyan varieties of mango (Mangifera indica L .). Am. J. Essent. Oils Nat. Prod. 6, 29–36.
White, I. R., Blake, R. S., Taylor, A. J., and Monks, P. S. (2016). Metabolite profiling of the ripening of mangoes Mangifera indica L. cv. ‘Tommy Atkins’ by real-time measurement of volatile organic compounds. Metabolomics 12:57. doi: 10.1007/s11306-016-0973-1
Keywords: tree-attached mango, Bactrocera dorsalis, Fopius arisanus, Diachasmimorpha longicaudata, headspace, GC-MS
Citation: Miano RN, Mohamed SA, Cheseto X, Ndlela S, Biasazin TD, Yusuf AA, Rohwer E and Dekker T (2022) Differential responses of Bactrocera dorsalis and its parasitoids to headspaces of different varieties of tree-attached mango fruits and the associated chemical profiles. Front. Ecol. Evol. 10:1021795. doi: 10.3389/fevo.2022.1021795
Received: 17 August 2022; Accepted: 24 October 2022;
Published: 14 November 2022.
Edited by:
Michael Wink, Heidelberg University, GermanyReviewed by:
Antonino Cusumano, University of Palermo, ItalySalvatore Guarino, Institute of Bioscience and Bioresources (CNR), Italy
Copyright © 2022 Miano, Mohamed, Cheseto, Ndlela, Biasazin, Yusuf, Rohwer and Dekker. This is an open-access article distributed under the terms of the Creative Commons Attribution License (CC BY). The use, distribution or reproduction in other forums is permitted, provided the original author(s) and the copyright owner(s) are credited and that the original publication in this journal is cited, in accordance with accepted academic practice. No use, distribution or reproduction is permitted which does not comply with these terms.
*Correspondence: Raphael Njurai Miano, cm1pYW5vQGljaXBlLm9yZw==; bWlhbm9ybkBnbWFpbC5jb20=; Samira A. Mohamed, c2ZhcmlzQGljaXBlLm9yZw==
†ORCID: Raphael Njurai Miano, orcid.org/0000-0003-3399-8439; Samira A. Mohamed, orcid.org/0000-0001-6836-7528; Xavier Cheseto, orcid.org/0000-0002-9779-0357; Shepard Ndlela, orcid.org/0000-0003-0015-9946; Tibebe Dejene Biasazin, orcid.org/0000-0001-9269-7236; Abdullahi Ahmed Yusuf, orcid.org/0000-0002-8625-6490; Egmont Rohwer, orcid.org/0000-0002-9123-2338; Teun Dekker, orcid.org/0000-0001-5395-6602