- 1School of Resource and Environmental Management, Simon Fraser University, Burnaby, BC, Canada
- 2Parks Canada, Office of the Chief Ecosystem Scientist, Protected Areas Establishment and Conservation Directorate, Vancouver, BC, Canada
- 3School of Environmental Science, Simon Fraser University, Burnaby, BC, Canada
Paleoecological investigation of two montane lakes in the Kootenay region of southeast British Columbia, Canada, reveal changes in vegetation in response to climate and fire throughout the Holocene. Pollen, charcoal, and lake sediment carbon accumulation rate analyses show seven distinct zones at Marion Lake, presently in the subalpine Engelmann Spruce-Subalpine Fir (ESSF) biogeoclimatic (BEC) zone of Kootenay Valley, British Columbia. Comparison of these records to nearby Dog Lake of Kootenay National Park of Canada in the Montane Spruce (MS) BEC zone of Kootenay Valley, British Columbia reveals unique responses of ecosystems in topographically complex regions. The two most dramatic shifts in vegetation at Marion Lake occur firstly in the early Holocene/late Pleistocene in ML Zone 3 (11,010–10,180 cal. yr. B.P.) possibly reflecting Younger Dryas Chronozone cooling followed by early Holocene xerothermic warming noted by the increased presence of the dry adapted conifer, Douglas-fir (Pseudotsuga menziesii) and increasing fire frequency. The second most prominent change occurred at the transition from ML Zone 5 through 6a (∼2,500 cal. yr. B.P.). This zone transitions from a warmer to a cooler/wetter climate as indicated by the increase in western hemlock (Tsuga heterophylla) and subsequent drop in fire frequency. The overall cooling trend and reduction in fire frequency appears to have occurred ∼700 years later than at Dog Lake (∼43 km to the south and 80 m lower in elevation), resulting in a closed montane spruce forest, whereas Marion Lake developed into a subalpine ecosystem. The temporal and ecological differences between the two study sites likely reflects the particular climate threshold needed to move these ecosystems from developed forests to subalpine conditions, as well as local site climate and fire conditions. These paleoecological records indicate future warming may result in the MS transitioning into an Interior Douglas Fir (IDF) dominated landscape, while the ESSF may become more forested, similar to the modern MS, or develop into a grassland-like landscape dependent on fire frequency. These results indicate that climate and disturbance over a regional area can dictate very different localized vegetative states. Local management implications of these dynamic landscapes will need to understand how ecosystems respond to climate and disturbance at the local or ecosystem/habitat scale.
Introduction
National Parks and other protected areas are commonly established to conserve biodiversity and ecological integrity (Parks Canada Agency, 2000; Parks Canada and the Canadian Parks Council, 2008). Even though these parks are protected from most forms of resource extraction, climate change impacts are expected to affect the structure and function of ecosystems at a rate that exceeds natural ranges of variability (Canadian Parks Council Climate Change Working Group, 2013). Land managers are faced with the challenge in understanding how park ecosystems will respond to future change. Paleoecological proxies offer information on long-term climate, vegetation, and disturbances (e.g., fire) and their relationship with present ecological states.
One approach to understanding the ecological trajectories at the local to landscape level is to use paleoecological methods such as pollen and charcoal analyses of radiometrically dated lake sediments. These methods allow researchers and managers to see how ecosystems have responded to environmental change such as climate and fire over millennia. In topographically complex regions such as the North American Cordillera it is important to look at more than one study site using multiple proxies to understand the local to regional variation in ecosystem response to climate, fire, and other land uses that are likely not represented by single study sites meant to represent broad geographic regions (Davis et al., 2020; Trant et al., 2020; Collison, 2021). This study examines two study sites in the adjacent Kootenay and Columbia Valleys in order to understand the temporal and spatial variation that climate and disturbance had in the southeast Kootenay region of British Columbia, Canada.
Previous Holocene paleoecological research in southeastern British Columbia and the northwestern United States focused on vegetation dynamics and paleolimnological responses to climate changes occurring at the treeline at alpine sites (Beaudoin, 1986; Luckman and Kearney, 1986; Pellatt et al., 1998; Smith et al., 1998; Pellatt et al., 2000; Heinrichs et al., 2002; Davis et al., 2018; Peteet et al., 2019; Wang et al., 2020) and forest cover changes at mid and lower elevations (Mack et al., 1983; Hebda, 1995; Hallett and Walker, 2000). All of these studies propose an approximation of the following climate history. The early Holocene period (∼11,400–10,500 cal yr. B.P.) was warm and dry. The early Holocene Xerothermic period (∼10,500–8,000 cal yr. B.P.) was hot and dry. The mesothermic period (∼8,000–4,500 cal yr. B.P.) was warm and wet, except on the Pacific Coast where near modern (cool and wet) conditions existed (Pellatt and Mathewes, 1997). The Neoglacial period (∼4,500 cal yr. B.P. to present) was cool and wet (Hansen, 1955; Mathewes, 1973; Mathewes and Rouse, 1975; Hebda, 1982; Mathewes, 1985; Hebda, 1995; Pellatt and Mathewes, 1997; Walker and Pellatt, 2008). More recent works have taken a site-specific look at vegetation dynamics and disturbance regimes in the Columbia Mountains and found that fire is the dominant disturbance over the Holocene along with more localized disturbances like insect outbreaks and windthrow (Mustaphi and Pisaric, 2014; Mustaphi and Pisaric, 2017) as well as a regional approach to interpreting large scale trends in changing fire frequency during the Holocene (Walsh et al., 2015).
In this study, we examine the vegetation, fire, climate, and lake sediment carbon accumulation rates of a catchment area within Kootenay National Park of Canada and in an adjacent non-protected catchment area, both in the Kootenay Region of southeast British Columbia (Figure 1). This work differs from previous studies by comparing similar catchment areas in and outside a protected area providing insight into the causal mechanisms of ecosystem responses to disturbance during the late-Holocene as well as examining local vegetation and fire responses of these watersheds to climate in a topographically complex region. By analyzing the paleoecological proxies of pollen, carbon, and charcoal over the Holocene, we can characterize ecosystems responding to disturbance and infer what the causal mechanisms may be. When experiencing a disturbance, resilience is the ability of an ecosystem to retain its current state rather than rearranging into an alternative, functionally and structurally different state (Gunderson and Holling, 2002). A crucial need for assessing resilience in an ecosystem is detecting thresholds and changes that provide early warning signs of impending regime shifts (Kéfi et al., 2014; Dakos et al., 2015; Dominguez-Garcia et al., 2019). The large changes in climate and disturbance during the Holocene provide an excellent example of changes in ecosystem resilience, thresholds, and trajectories. For the purposes of this study, a regime shift would be a change in biogeoclimatic zone. A biogeoclimatic zone (BEC) is a geographic area that has a broadly homogenous macroclimate and similar vegetation (Ministry of Forests and Range, 2008). The goal of this study is to use paleoecological data to infer vegetation response to fire disturbance and long-term climate change in two adjacent catchment areas currently representing two adjacent BEC zones within the Kootenay Valley of British Columbia.
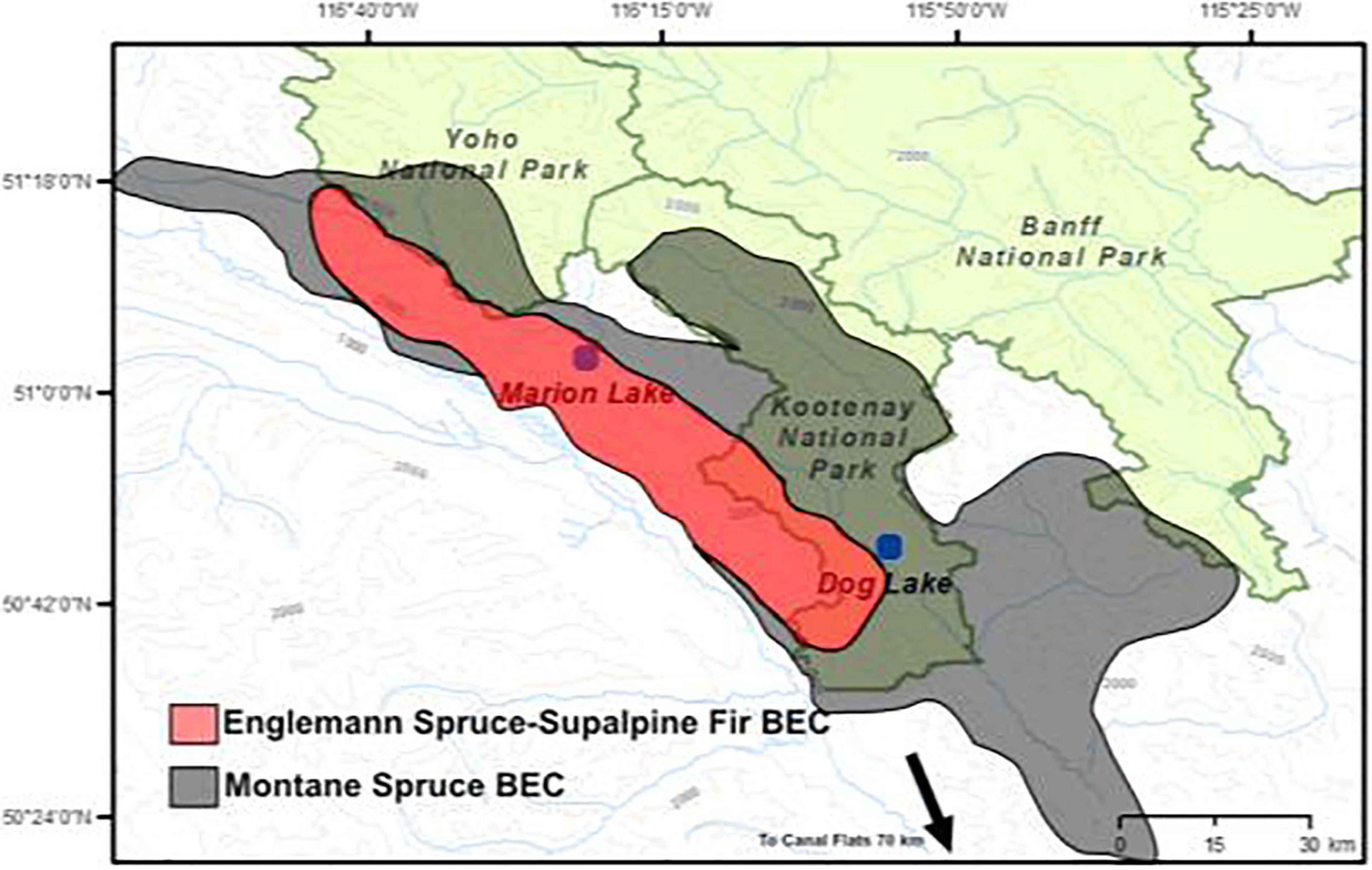
Figure 1. Study area of Marion and Dog Lake. Shaded green areas represent National Parks. Blue lines represent waterways. BEC, Biogeoclimatic Zone.
Materials and Methods
Study Area
Marion and Dog lakes are situated in the northwest-southeast trending Kootenay Valley (Figure 1), just west of the Continental Divide in the Rocky Mountains of southeastern British Columbia. The Kootenay Valley is the drainage basin of the upper Kootenay River and part of the Columbia River watershed. Marion Lake sits near the headwaters of the Kootenay River in a sub-region of the Kootenay Valley known as the Beaverfoot Valley. Dog Lake sits near the Kootenay River downstream of Marion Lake in the Kootenay Valley inside Kootenay National Park.
Marion Lake was chosen as a companion lake to Dog Lake on the basis of proximity outside the national park boundaries, surrounding catchment characteristics, lake morphology, and biogeoclimatic properties and the existing pollen and charcoal data that was undertaken by Hallett and Hills (2006) (Table 1). Dog and Marion Lakes both possess small outlets that are active during spring snowmelt. Both lakes have a flat rectangular bathymetric basin with steep sides and a broad littoral zone.
The lakes are situated in different BEC zones and thus experience different seasonal temperature ranges and vegetation distributions. Specifically, Dog Lake is in the Montane Spruce (MS) BEC zone and Marion is in the Engelmann Spruce-Subalpine Fir (ESSF) BEC zone (Table 1; Meidinger and Pojar, 1991). The ESSF zone comprises closed stands of Picea and Abies cf. lasiocarpa. Dog Lake is located at a lower elevation in the MS zone (<500 m), with mixed stands of Pinus, Pseudotsuga, and Betula.
Field Methods and Chronology
A 216 cm sediment core was retrieved from the deepest part of Marion Lake (13.7 m) using both a Glew gravity corer (upper 54 cm) (Glew et al., 2001) and a modified Livingstone corer (Wright, 1967) in July, 2012. A 420 cm sediment core was retrieved from the deepest part of Dog Lake (4.7 m) to examine carbon to compare to pollen, and charcoal analysis undertaken by Hallett and Hills (2006) Cores taken using the Livingstone corer were matched using a Bartington MS2 Magnetic Susceptibility reader. Sediment cores were subsampled at 1 cm intervals and placed in Whirlpak® bags and transported to the Climate Oceans and Paleoenvironments (COPE) laboratory at Simon Fraser University in Burnaby, Canada where they subsequently were preserved at 4°C.
The chronologies were developed using accelerator mass-spectrometry (AMS) 14C dating on pollen samples (Brown et al., 1989) from the lower Livingstone sections and using 210Pb dating of bulk sediment from the upper sediment recovered with the Glew corer for both Marion and Dog Lakes. AMS 14C ages from pollen from the lower Livingstone sections of the Marion and Dog Lake cores were processed at the Center for Accelerator Mass Spectrometry at the Lawrence Livermore National Laboratory following AMS recent developments (Southon et al., 2016). The 210Pb ages were determined from bulk sediment samples in the upper Glew section of both the Marion and Dog Lake cores. MyCore Scientific Inc. processed all the 210Pb samples for Marion Lake, and Core Scientific International processed all the 210Pb samples for Dog Lake. 210Pb and 14C ages were then used to create an age-vs.-depth model for Marion and Dog lakes (Figures 2A,B) using the Bayesian age-depth model Bacon (v2.3.7) (Blaauw and Christen, 2011). 14C ages in the Bacon model were calibrated using the CALIB program (Stuiver et al., 2021).
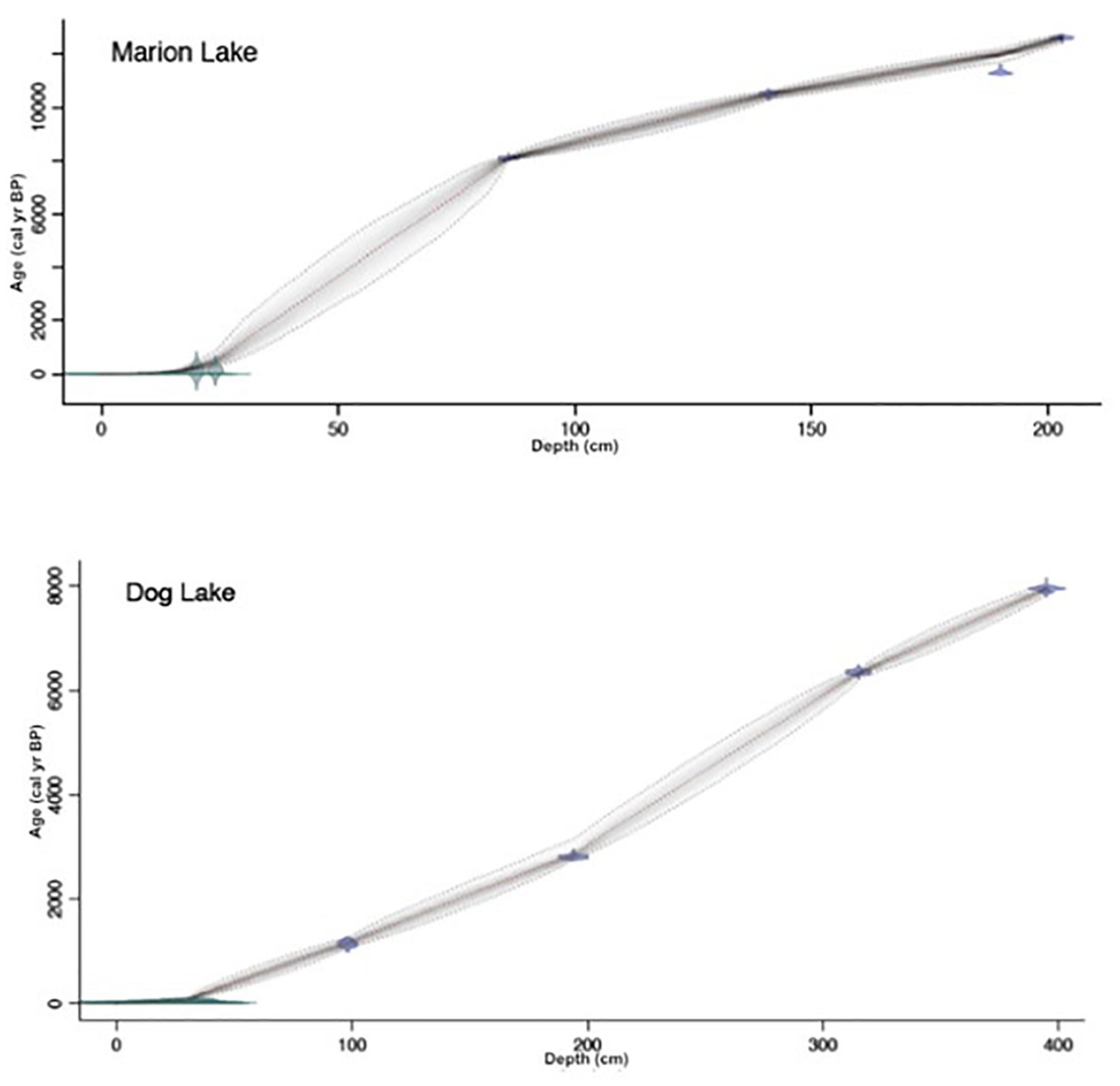
Figure 2. Marion Lake age-vs.-depth relationship (A) and Dog Lake age-vs.-depth relationship (B). The likelihood the model runs through a sample (points with errors) in grayscale, where the darker the gray, the more likely the model runs through that sample.
Pollen Analysis
We collected 50 (1 cm3) samples at selected intervals throughout the Marion Lake core. Pollen preparation followed the standard pollen recovery methods using acetolysis and HF as outlined in Faegri and Iverson (1989) at the Parks Canada Vancouver Ecology Laboratory and the Geological Survey of Canada Vancouver Laboratory. A sodium polytungstate process (van den Bos et al., 2020) was attempted, but was found to be inadequate at the concentration needed for analysis. Exotic Lycopodium tablets (Batch # 938934) were added to calculate pollen concentration and subsequent accumulation rates. Pollen and spores were identified at 400× magnification and difficult identifications were made at 1000× under oil immersion. Pollen and spores were identified using in-house reference keys at the Parks Canada Vancouver Ecology Laboratory and the Mathewes Laboratory at Simon Fraser University. A minimum of 500 pollen grains were counted per sample. A percentage diagram based on the terrestrial pollen sum was produced using TILIA v1.7.16 (Grimm, 1993). Pollen assemblage zones were established using the application of CONISS in the TILIA program, using square root transformed percent data (Figure 3). A pollen influx diagram using linear interpolation of calibrated dates was also prepared using TILIA. Dr. Hallett conducted pollen analysis using the same methods for Dog Lake (Hallett and Hills, 2006).
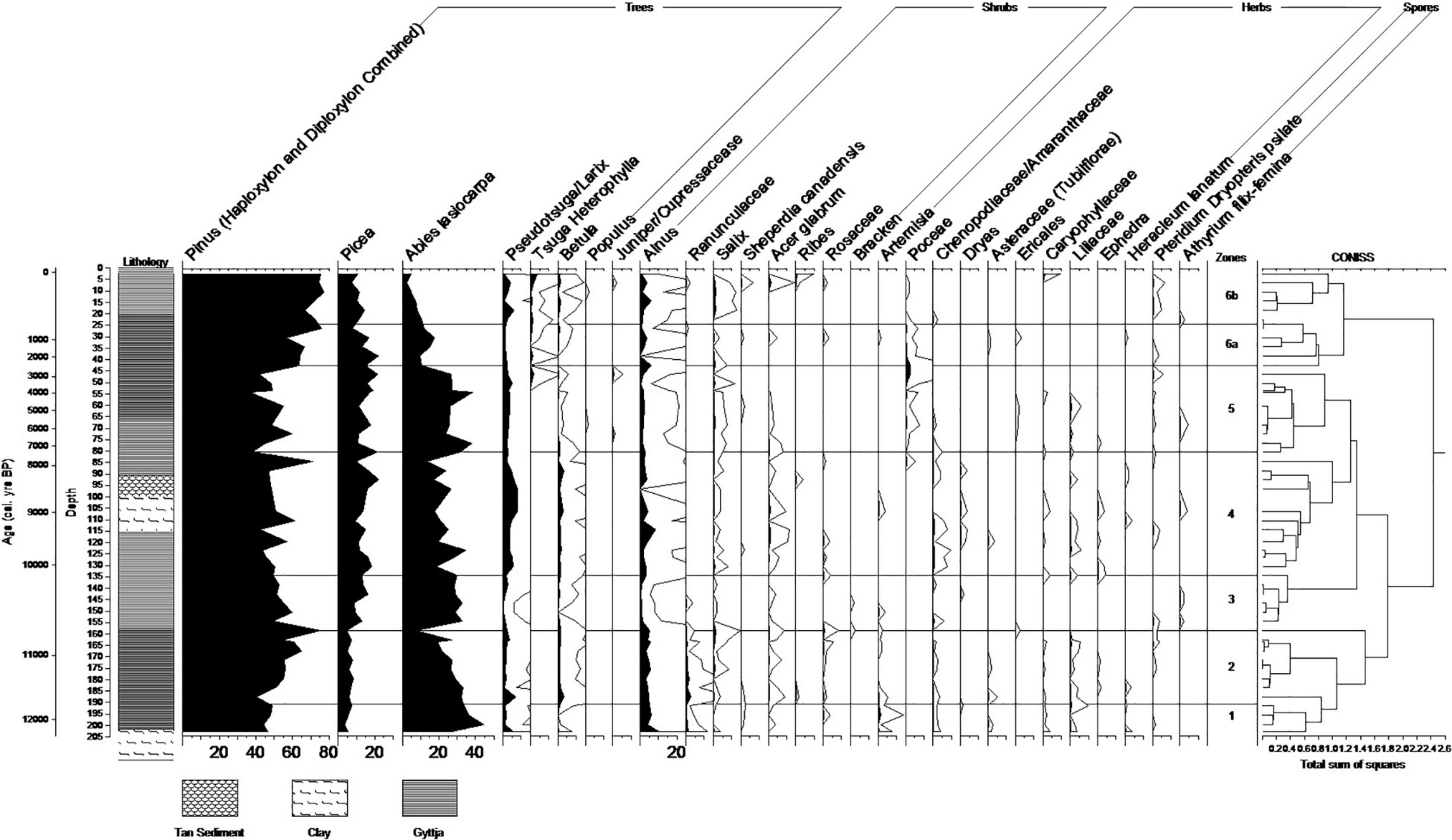
Figure 3. Percent pollen diagram for Marion Lake, BC, including age depth, lithology, and constrained cluster analysis for zonation. Hollow lines represent 10X exaggeration of pollen percentages.
Charcoal (Fire) Analysis
Reconstructing western North American fire histories on century-to-millennial scales is often done through analysis of macroscopic charcoal (Long et al., 1998; Higuera et al., 2011). Macroscopic charcoal (>125 μm) analysis can be used to differentiate between background and peak fire events. Peak events in the charcoal history are an indication of fire episodes localized near the lake basin. Because charcoal can enter a catchment area and ultimately the lake basin in multiple ways [e.g., wind (Whitlock and Millspaugh, 1996), landslides, or anthropogenic fires], interpretation of charcoal peaks as fire episodes needs to be done cautiously (Lynch et al., 2004). Records in lake sediments often can be distinguished between fires burning within the catchment area and those at a distance. Macroscopic charcoal remains the best tool for reconstructing long-term Holocene fire history and can be paired with other independent paleoecological proxies.
Contiguous 1 cm3 samples were taken at 1 cm intervals in both the Glew (upper) and Livingstone (lower) Marion Lake cores for charcoal analysis. Samples were soaked in 10% KOH and placed in a hot water bath for 15 min. After shaking, centrifuging, and decanting, 6% H2O2 was added to the remaining sample and soaked at 50°C for 24 h. Samples were washed on a 125 μm mesh sieve and the residue was transferred into gridded petri dishes and counted. Only charcoal particles >125 μm in minimum diameter were counted as previous studies indicate that large particles are not transported far from the source and thereby indicate local fire activity (Murphy et al., 2019).
Charcoal analysis for the Marion Lake core followed methods outlined in Higuera et al. (2010) using the program CharAnalysis. Number of peak fires represent inferred “fire episodes,” or one or more fires occurring in the duration of a peak (Long et al., 1998). Fire frequency is the sum of the total number of fires within a 1000 year period. Mean fire return interval (mFRI) is the average number of years between fire episodes. Sensitivity analysis showed that the signal-to-noise ratio (i.e., the measure of the separation between peak and non-peak values) was maximized at 500 years. Charcoal analysis of Dog Lake was done by Hallett and Walker (2000) following the methods of Long et al. (1998).
Geochemical Analysis
Samples were taken at contiguous 1 cm intervals in both the Glew (upper) and Livingstone (lower) Marion and Dog Lake cores for carbon and nitrogen analysis. Samples were dried at 60°C for 24 h and crushed. A dried subsample was used to measure the weight percent of total carbon (TC) and nitrogen using a Costech ECS 4010 elemental analyzer. Another dried subsample was used to measure weight percent of total inorganic carbon (TIC) using a UIC CM5130 acidification module connected to a UIC CM5014 CO2 coulometer. The weight percentage of total organic carbon (TOC) was then estimated by taking the difference between TC and TIC (Meyers and Teranes, 2001). Estimates of the organic C/N (atomic) ratios were also calculated based on TOC and nitrogen weight percentage from each sample.
Mass accumulation rate (MAR) was determined by the following equation:
Where DBD is the measured dry bulk density of a one-cm3 volume of sample, and LSR represents the linear sedimentation rate. Dry bulk densities DBD were measured after 24 h in a drying oven set at 60°C. The linear sedimentation rate (LSR) was based on the age-depth model determined for each core. Total organic carbon accumulation rate (TOC MAR) was then determined using the following equation:
Results
Each analysis section will be presented using vegetation zones. Vegetation zones were determined using constrained cluster analysis from the CONISS program (Grimm, 1993) for Marion Lake (Figure 3). Separate results and discussion are reported using the Marion Lake zones. The Dog Lake pollen diagram (Hallett and Hills, 2006) is reproduced for reference in Supplementary Material 1.
Pollen Analysis
As with most pollen studies in boreal and sub-boreal ecosystems, conifer pollen (especially Pinus) is overrepresented (Pellatt et al., 1997). This is true for this study as well with pollen assemblages being dominated by Pinus, Abies, and Picea (Figure 3).
Zone ML-1 Abies-Picea-Artemisia 12,580–12,060 cal. yr. B.P.
Abies has its highest pollen percentage (44%) at 12,438 cal. yr. B.P. and steadily decreases throughout the zone. This decrease is accompanied by a corresponding increase in Pinus that continues into zone ML-2. Pinus was not differentiated as it is highly over-represented, Picea has its lowest value (3.5%). Artemesia has its highest percentage (1.4%) at 12,244 cal. yr. B.P. (Figure 3).
Zone ML-2 Pinus-Abies-Ranunculaceae, 12,060–11,010 cal. yr. B.P.
Pinus spikes to its second highest value (75%) while Abies steadily drops to one of its lowest values (7%). Ranunculaceae pollen has its highest percentage in this zone (2.5%) (Figure 3).
Zone ML-3 Picea, 11,010–10,180 cal. yr. B.P.
Alnus cf. incana, Betula, and Pseudotsuga/Larix have their lowest values (3, 0, and 3%, respectively). Picea increases and Pinus decreases (Figure 3).
Zone ML-4 Pseudotsuga/Larix-Alnus cf. incana-Picea, 10,180–7,342 cal. yr. B.P.
Abies and Pinus have similar values to ML-1. Pseudotsuga/Larix and Alnus cf. incana have high percentages. Betula has similar values to ML-1. Picea has its highest percentage (22%). Poaceae appears at the end of this zone (Figure 3).
Zone ML-4 contains a tan sediment layer between 92.5 and 98.5 cm above the clay layer between 98.5 and 114.5 cm. There is core break between Livingstone drives at 105.5 cm. in the clay layer.
Zone ML-5 Tsuga-Poaceae, 7,342–2,685 cal. yr. B.P.
Tsuga heterophylla appears at the end of this zone. Poaceae increases and reaches its maximum value (2.5%) at the end of this zone. Abies has relatively high values. Alnus cf. incana and Betula have relatively low percentages in this zone (Figure 3).
Zone ML-6a Pinus-Poaceae-Psedudotsuga/Larix, 2,685–400 cal. yr. B.P.
Pinus is increasing throughout the zone. Poaceae starts at its second highest value (2.4%) and then decreases. Pseudotsuga/Larix is low throughout the zone (Figure 3).
Zone ML-6b Abies-Picea-Pinus-Tsuga-Poaceae, 400–0 cal. yr. B.P.
This transitional zone shows abrupt changes in pollen ratios. This zone has lower Abies values. Abies and Picea are both decreasing. The largest percentages of Pinus (undiff.) (77%) and Tsuga (4%) pollen occurs in the last 100 cal. yr. B.P. Poaceae is present, but is decreasing (Figure 3).
Charcoal (Fire) and Geochemical Analyses
Zone ML-1
Fire frequency in this zone is high with 2.1–2.4 (average 2.3) fire events per 1,000 years. The mFRI is 301 years (n = 2) (Figure 4A). Carbon accumulation rate in this zone is low with 3.1–3.4 (average 3.0) g/m2/yr (Figure 4A).
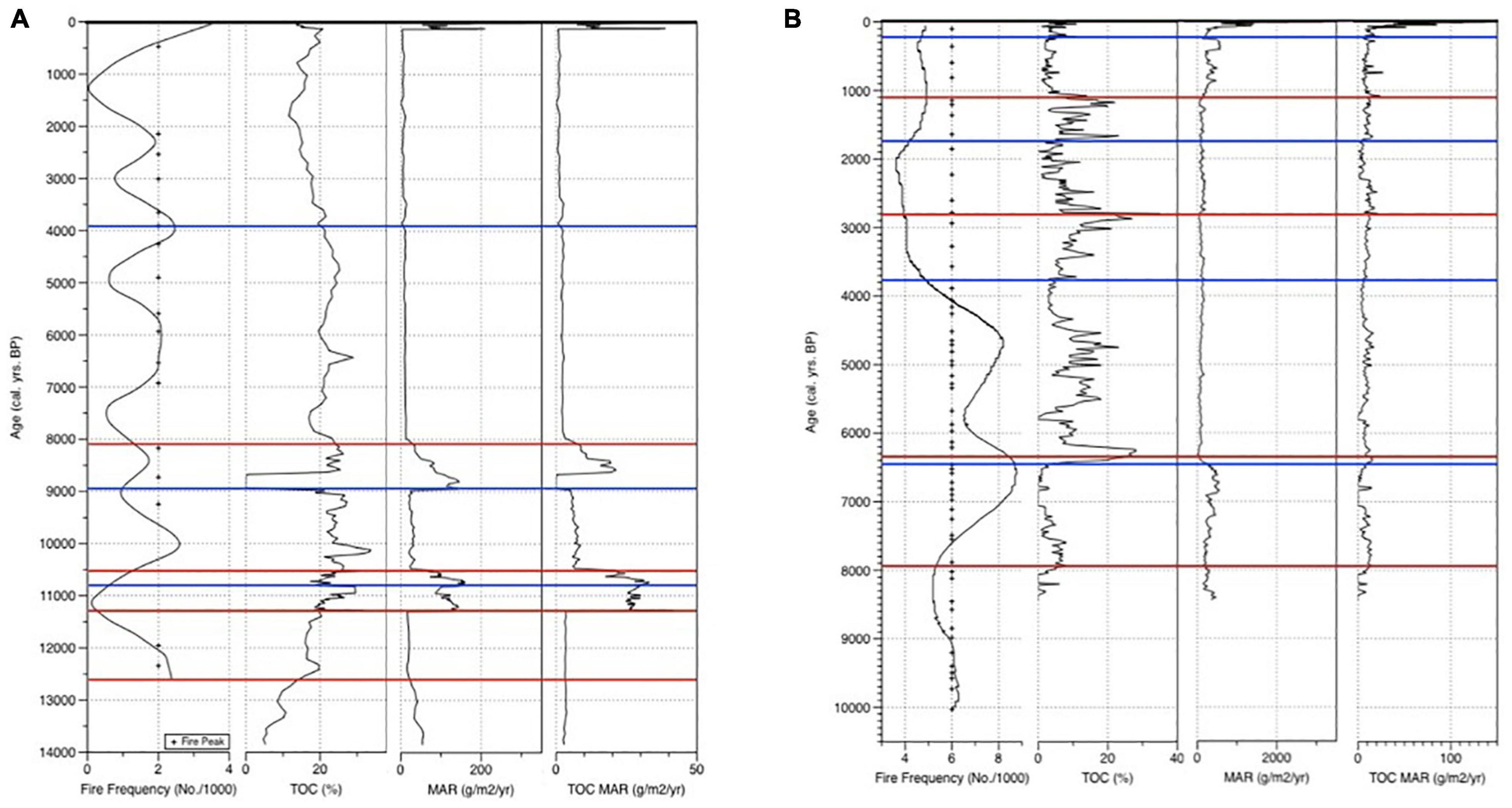
Figure 4. TOC MAR, MAR, TOC, inferred fire frequency, and fire peaks of Marion (A) and Dog Lake (B). Blue lines represent core breaks. Red lines represent tie points. Dog Lake charcoal data is interpolated from Hallett and Walker (2000).
Zone ML-2
Fire frequency in this zone is low with 0.1–2.0 (average 0.9) fire events per 1,000 years. The mFRI is 774 years (n = 2). Carbon accumulation in this zone is at its highest rate with 3.3–41 (average 26) g/m2/yr (Figure 4A).
Zone ML-3
Fire frequency in this zone is low with 0.2–2.4 (average 1.1) fire events per 1,000 years. The mFRI is 473 years (n = 1). Carbon accumulation rate in this zone is high with 5.7–33 (average 21) g/m2/yr (Figure 4A).
Zone ML-4
Fire frequency in this zone is low with 0.58–2.5 (average 1.4) fire events per 1,000 years. The mFRI is 502 years (n = 6). Carbon accumulation is moderate with 0.052–21 (average 7.2) g/m2/yr with high fluctuation (20 g/m2/yr) toward zone ML-4 (Figure 4A).
Zone ML-5
Fire frequency in this zone is low with 0.6–2.5 (average 1.5) fire events per 1,000 years. The mFRI is 439 years (n = 10). Carbon accumulation is low throughout this zone with 0.7–2.7 (average 2.0) g/m2/yr (Figure 4A).
Zone ML-6a
Fire frequency in this zone is low with 0.043–1.9 (average 1.0) fire events per 1,000 years. The mFRI is 846 years (n = 3). Carbon accumulation is low in this zone with 0.1–37 (average 2.7) g/m2/yr (Figure 4A).
Zone ML-6b
Fire frequency in this zone is high with 2.0–3.5 (average 2.8) fire events per 1,000 years. Carbon accumulation is high in this zone with 7.42–46 (average 21) g/m2/yr (Figure 4A).
Discussion
Pollen Analysis
Zone ML-1 Abies-Picea-Artemisia, 12,580–12,060 cal. yr. B.P.
The peak abundance of Artemisia in Marion Lake occurs in this zone, corresponding with the timing of the Younger Dryas Chronozone. The high abundance of Artemisia during the Younger Dryas has also been noted in Waits Lake, northeastern Washington in the nearby Columbia River basin (Mack et al., 1978; Walker and Pellatt, 2008). The Younger Dryas was a period of rapid cooling approximately 12,800–11, 500 cal yr. B.P. that was first considered to be restricted to Europe. However, it has since been corroborated in coastal British Columbia (Mathewes, 1993; Mathewes et al., 1993), the American Rockies (Reasoner and Jodry, 2000), the Canadian Rockies (Reasoner et al., 1994), and the Canadian Maritimes (Levesque et al., 1993).
At Marion Lake, the abundance of Artemisia, Alnus cf. incana, Cyperaceae, Asteraceae, and Ranunculaceae are indicative of an alpine/tundra like landscape. High pollen percentages of nitrogen-fixing shrubs such as Alnus cf. incana and Shepherdia canadensis indicate a pioneering vegetation assemblage. At high enough elevation, the pioneering tree species, Picea and Abies likely grew as krummholz, or stunted trees, as the relatively high percentages of these taxa resemble those of modern subalpine assemblages. The Abies and Picea pollen around the lower elevation Dog Lake might have been derived from upslope subalpine sites akin to Marion Lake. The relatively low Pinus (undiff.) pollen around Marion Lake is likely transported from distant stands (Pellatt et al., 1998, 2000; Heinrichs et al., 2002).
Zone ML-2 Pinus (undiff.)-Abies-Ranunculaceae, 12,060–11,010 Ca. yr. B.P.
Regional studies indicate that following the Younger Dryas (∼11,500 cal yr. B.P.), the early Holocene was marked by sudden warming into the relatively hot and dry xerothermic period between 11,500 and 8,000 cal yr. B.P. (Mathewes, 1993; Walker and Pellatt, 2003). Fossil midge paleotemperature reconstructions for south central interior BC also support a warmer temperature (∼2–3°C warmer than present) during the xerothermic period (Pellatt et al., 2000; Palmer et al., 2002; Walker and Pellatt, 2003).
Zone ML-3 Alnus cf. incana, Betula, Pseudotsuga/Larix, 11,010–10,180 cal. yr. B.P.
With years of soil accumulation in the Kootenay valley, tree species began to establish into new areas. The reduction in Betula and Alnus cf. incana percentages relative to those found in Zone ML-2 indicate that these pioneering species were being outcompeted by species like Picea, adapted to lower levels of disturbance (Figure 3; Hallett and Hills, 2006). Noting the high fire frequency in this zone, this disturbance is likely fire.
Zone ML-4 Pseudotsuga/Larix-Alnus cf. incana-Picea, 10,180–7,342 cal. yr. B.P.
Supporting studies indicate that this zone had the highest summer air temperatures, +3°C higher than today, as indicated by fossil chironomid head capsule records in southern British Columbia (Palmer et al., 2002). Inferred climate records from other southern BC studies concur that this was the beginning of the early Holocene warm and dry xerothermic interval (Hebda, 1995; Pellatt et al., 1998; Smith et al., 1998; Heinrichs, 1999; Heinrichs et al., 2001).
At lower-elevations in the Dog Lake sediment core, Pseudotsuga/Larix appears at around 9,500 cal yr. B.P., likely migrating from lower elevations (Hazell, 1979; Hebda, 1995; Hallett and Hills, 2006). Three hundred km north of Dog Lake in Jasper National Park, oxygen-isotope analysis of fossil wood indicate temperatures became warmer at higher elevations in the region by 8,800 cal yr. B.P. (Luckman and Kearney, 1986). The composite midge paleotemperature reconstruction for southern British Columbia confirms that the period between 9,000 and 6,600 cal yr. B.P. was warmer for southern British Columbia (Rosenberg et al., 2004).
At Dog Lake, high rates of charophyte accumulation, with high percentages of aquatic pollen or spores or other microremains and Abies pollen, indicate a moist climate (Hallett and Hills, 2006). The increase of Picea throughout this zone signals a closing forest around Marion Lake.
Zone ML-5 Tsuga-Poaceae, 7,342–2,685 cal. yr. B.P.
Tsuga heterophylla pollen first appears at around 3,100 cal. yr. B.P. and continues to increase to present. This arrival is in agreement with other fossil pollen studies from southern British Columbia (Rosenberg et al., 2004) and indicates the continuation of a wet moisture regime and less seasonably extreme winter climate (Bartlein et al., 1998; Walker and Pellatt, 2003; Hallett and Hills, 2006).
Zone ML-6a Pinus (undiff.)-Poaceae-Pseudotsuga/Larix, 2,685–400 cal. yr. B.P.
Zone ML-6a is characterized by the continued decrease in Picea and the presence of fire-adapted taxa such as Pinus, Pseudotsuga/Larix, Alnus cf. incana, and Pteridium, which indicate that forests around the lake were being disturbed and moving toward a more open landscape. Other studies suggest that modern forest types were being established at this time in southern BC as the climate continued in the cool and moist state that first began in the mid-Holocene (Kearney and Luckman, 1983; Mathewes, 1985; Luckman and Kearney, 1986; Hebda, 1995; Pellatt et al., 1998; Pellatt et al., 2000; Heinrichs et al., 2002; Hallett and Hills, 2006). The increased pollen accumulation rates in this zone from shrubs and herbs support the idea that subalpine meadows established during Zone ML-5 were maturing. The mix of meadows and forest points toward establishment of the modern ESSF forest as early as 2,500 cal yr. B.P.
Zone ML-6b Abies cf. lasiocarpa-Picea-Pinus-Tsuga-Poaceae, 400–0 cal. yr. B.P.
Of specific note, Abies cf. lasiocarpa pollen is especially low (3–8%) in the last 100 cal yr. B.P. This is likely an artifact of large counts of Pinus (undiff.), which produces abundant pollen. Pinus abundance is likely a result of extensive logging, increased fire frequency, or a more open landscape in general in the immediate area (Pellatt et al., 1997; Goring et al., 2008).
Charcoal (Fire) Analysis
Fire frequencies increase sharply starting at 8,000 cal yr. B.P. and peak at ∼6,700 cal yr. B.P. around Dog Lake (Hallett and Hills, 2006). The Marion Lake sediment record shows similar increases in fire frequency but peaks earlier at 9,800 cal yr. Alnus and Betula can adapt in response to fire, and the high percentages of both around Marion Lake indicate high fire activity in the catchment area during this zone. It should be noted that Alnus might also grow in response to other disturbances such as landslides and avalanches. However, a similar pattern of high fire frequency is seen during this zone in the subalpine conifer forests in the northern United States Rocky Mountains (Huerta et al., 2009). This increase in fire activity coincided with the interval of high summer insolation forcing and also a stronger-than-present subtropical high pressure (Brunelle and Whitlock, 2003). This climate would also have manifested itself as the wet winters akin to the western United States at this time (Bartlein et al., 1998; Kutzbach et al., 1998). The increase in Alnus pollen in this zone is likely from Alnus virdis, a shrub that favors moist conditions and indicates heavy winter snowpack (Thilenius, 1990; Kershaw et al., 1998). Broad-scale climatic controls are interpreted as the major influence on fire frequency in the nearby Engelmann Spruce-Subalpine Fir (ESSF) zone as indicated by charcoal sediment records in the Columbia Mountains (Gavin et al., 2006; Mustaphi and Pisaric, 2014; Mustaphi and Pisaric, 2017) and United States Rockies (Morris et al., 2013).
Geochemical Analysis
The high C accumulation rate (>25 g/m2/yr; Figure 4A) in Marion Lake (zone ML-4) could be caused by (1) high runoff and input of allochthonous matter from the surrounding catchment area, (2) increased in-lake productivity of autochthonous matter, (3) dating artifacts, or (4) a combination thereof. C/N ratios during this time indicate that the organic material is derived from mixed allochthonous and autochthonous sources (Supplementary Material 2). Two possible interpretations exist: (1) a complex shrub layer of nitrogen-fixing taxa was established (Alnus cf. incana, Shepherdia, and Rosaceae) in the catchment area, and/or (2) in-lake productivity of nitrogen-fixing taxa increased (e.g., cyanobacteria). Every effort was made by the authors to constrain the potential for dating artifacts through the use of core imagery and magnetic susceptibility to assemble the sediment core sections (see section “Materials and Methods”) and choosing material to date that were not near (<5 cm) a visual complex change (Figure 3).
At Marion Lake, N (%) is at its highest level in zone ML-4, and the C/N ratio indicates a shift to exclusively autochthonous deposition (Supplementary Material 2). This is supported by the near absence of nitrogen-fixing taxa (Alnus cf. incana, Shepherdia, and Rosaceae) and presence of a variety of aquatic pollen taxa (Figure 3). For this zone, in-lake productivity was the main contributor to the fluctuating C accumulation rate.
Around, 8,500 cal yr. B.P. (zone ML-4) the C/N ratio switches from mixed sourcing {∼8 [C/N (atomic)]} to terrestrial sourcing levels {∼13 [C/N (atomic)]} that carries on into zone ML-6b ([dataset] Supplementary Material 3).
Zone ML-4 at Marion Lake contains a clay layer from 9,331 to 8,631 cal yr. B.P. that is coupled with a sharp reduction in organic carbon, inorganic carbon, and nitrogen (Figure 3). It is important to note that this clay layer creates a large break that may not be representative of actual carbon deposition because of an abrupt increase in sediment deposition. This large sediment deposition could have been caused by a number of natural disturbances. Given the current geomorphology of the area, this abrupt change was likely due to the Kootenay River changing course in the Beaverfoot Valley. Further work would need to be conducted to conclude that a river shift would manifest itself in this way in the sediment.
At Marion Lake, replacement of Pseudotsuga by Abies indicates establishment of a cooler, moister climate during Zone 4. These cooler and moister conditions are consistent with midge-inferred summer temperature reconstructions for southern British Columbia, which reveal an abrupt end to the xerothermic period at approximately 6,700 cal yr. B.P. (Rosenberg et al., 2004). In the northern United States Rockies, the termination of the seasonal cycle of insolation led to Pseudotsuga being replaced by Pinus and Abies indicating a return to cooler and moister conditions (Brunelle and Whitlock, 2003). Corresponding increases in Abies and Picea indicate similar transitions in subalpine forests of southern BC (Pellatt et al., 1998; Pellatt et al., 2000; Heinrichs et al., 2001, 2002).
Around Dog Lake, the Picea pollen increases represent a shift of the ESSF zone to lower elevations into the surrounding MS zone (Hallett and Hills, 2006). Around Marion Lake, the presence of Juniperus/Cupressaceae pollen, which grows today in alpine sites of southern British Columbia (Heinrichs et al., 2002), indicates that the Alpine Tundra (AT) zone also shifted to lower elevations, consistent with cooler conditions during Zone 5. In addition, subalpine meadows may have been established in the ESSF at Marion Lake. The presence of Poaceae in the Marion Lake core at this time indicates an open landscape and the low total pollen accumulation rate (Ritchie and Lichti-Fedorovich, 1967) corroborates the suggestion of a more open landscape.
At Dog Lake, charophyte (freshwater algae) values rise consistently after 5,400 cal yr. B.P., indicating higher lake levels (Hallett et al., 2003). This is concurrent with atmospheric circulation changes and glacial advance (Mayewski et al., 2004). Cool and wet winters during 5,400 cal yr. B.P. to 3,000 cal yr. B.P. years ago (Ramiadantsoa et al., 2019) likely supplied Dog and Marion lakes with a cool water supply from snowpacks melting long into the summer months. These wetter summers account for the low fire frequency and low carbon accumulation rates observed throughout this zone. In Dog Lake, wetter summers increase OC content by 10–20% during this zone compared to the previous Zone 4. This increase is spurred by in-lake activity (Hallett and Hills, 2006). The C/N data indicate that Marion Lake derived its OC content primarily from terrestrial sources during this time period, and wetter summers provided only a small foray into mixed sourcing (Supplementary Material 2).
Marion and Dog Lake Comparison
Marion and Dog lakes illustrate the interaction of climate on the development of subalpine and montane systems, with precipitation or site soil moisture strongly influencing plant communities and rates of change. These site-specific conditions can lead to a decoupling of localized ecosystems from the broader regional climate as localized climate conditions exceed species tolerance (Berauer et al., 2019). For example, the beginning of the cool and moist Neoglacial is around 4,700–4,500 cal yr. B.P. at the lower-elevation Dog Lake (Hallett and Hills, 2006; Walker and Pellatt, 2008). However, Marion Lake appears to experience increasing moisture in zone ML-5 as early as 7,300 cal yr. B.P. with decreasing Douglas-fir and increasing subalpine fir. At Marion Lake, inferred increases in precipitation are most prevalent around 3000 cal. yr. B.P. with increasing open conditions and the presence of western hemlock pollen. This is similar, but prior to, the expansion of western hemlock and the Interior Cedar Hemlock zone noted by Gavin et al. (2009) throughout British Columbia.
A second example of this type of decoupling between local and regional climate transitions is seen during the last 1000 years. However, in this case, the warming signal at the lower-elevation Dog Lake precedes that at Marion Lake. Marion Lake’s warming climate signal only begins in the last 700 cal yr. B.P., while Dog Lake begins to warm as early as 1,000 cal yr. B.P. This temporal difference is likely the same elevation effect working in reverse, with higher elevation sites experiencing growth limitations due to low temperature sooner than lower elevation sites.
After the xerothermic period (11,500–8,000 cal yr. B.P.), Marion Lake switches from a dry to a wetter landscape while Dog Lake stays relatively dry until the onset of the Neoglacial (4,500 cal yr. B.P.). This may be due to increased snowpack and subsequent snow melt allowing trees to establish in sites that accumulate snow, hence supply increased growing season soil moisture (BC Ministry of Forests, 1991).
In the wetter Neoglacial (4,500 cal yr. B.P. to present), Dog Lake switches from an open to a closed landscape while Marion Lake has a continued open landscape to present day. This is likely because continued Neoglacial cool conditions maintained subalpine conditions at Marion Lake. The vegetation shift around Dog Lake corresponds to an increase in biomass, which is reflected in C accumulation. Marion Lake’s shift toward a more open landscape is reflected in a decrease in C accumulation.
At Marion Lake, the higher disturbance rate at the end of zone ML6b is a product of increased fire frequency. This coincides with an increased C accumulation rate (Figure 5) and a spike in terrestrial sourcing material (Supplementary Material 2). The general trend toward a more mixed sourcing material is likely explained by the establishment of the current littoral zone, today supporting a fringe of Equisetum and Cyperaceae and increase in autochthonous productivity as marked by increased Pediastrum counts. Dog Lake is marked with high charophyte growth in this zone.
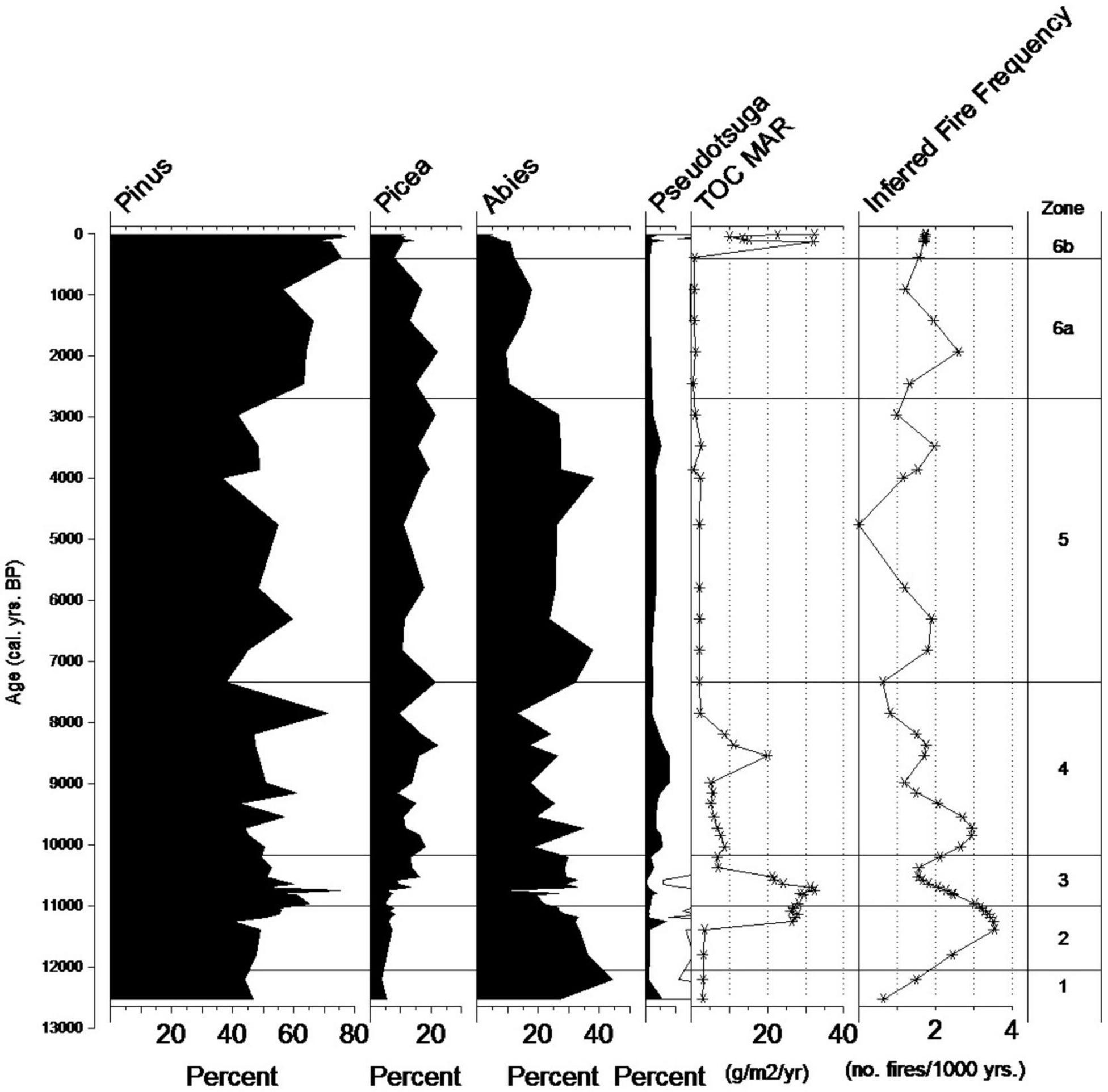
Figure 5. Marion Lake: Comparison of pollen zones and percentage data for Pinus, Picea, Abies, and Pseudotsuga with TOC MAR and inferred fire frequency.
Pollen ratios have been used to interpret changes in treeline throughout sites in the Canadian Cordillera (Beaudoin, 1986; Luckman and Kearney, 1986; Pellatt et al., 1998). In the last 700 cal yr. BP of zone ML-6a, pollen ratios of Pseudotsuga-Larix + Poaceae divided by Picea + Abies at Dog Lake decrease, indicating a return to the wet-closed conditions of the MS zone at the onset of the Neoglacial (Hallett and Hills, 2006). This change is synchronous with the Little Ice Age glacial advances in British Columbia (Luckman, 1995). Fire Frequency is low to moderate at both Dog and Marion Lakes. This late Holocene low to moderate fire frequency in the Kootenay Valley indicates that more complex local controls play more of an influence on fire activity than in the early and middle Holocene climate-driven high fire frequency (Gavin et al., 2006; Mustaphi et al., 2015; Table 2).
Local controls on recent fire regimes appear different between Marion and Dog Lakes. The recent mFRI of Dog Lake indicates a stand replacing fire regime akin to wetter areas (Hallett and Hills, 2006). A longer fire-free interval would allow the recent development of a closed forest and increase fuel conditions allowing for a stand replacing fire regime. An ecological succession model (Keane et al., 1990) demonstrates that with a longer mFRI, Pinus and Pseudostuga/Larix can be better established. At present a closed MS forest exists around Dog Lake and an open ESSF forest around Marion Lake. Looking to the local site factors we can also see how vegetation and disturbance influences carbon accumulation in lake sediments.
In both Marion and Dog Lakes carbon accumulation is synchronous with inferred fire frequency (IFF) (Figures 4A,B, 5; Hallett and Hills, 2006). The Columbia Valley and southern United States Rockies have a similar early Holocene vegetation with composition of Pinus and Alnus cf. incana (likely near the lakeshore) dominating, but have are replaced by the late Holocene with Picea, Abies, and Pseudotsuga/Larix (Gavin et al., 2006; Morris et al., 2013; Mustaphi and Pisaric, 2014). Pseudotsuga/Larix and Alnus cf. incana have their peaks in the mid-Holocene. This mid-Holocene difference between the Kootenay Valley versus the Columbia Valleys and Southern United States Rockies is highlighted by the IFF maximum exhibited by the Kootenay Valley and IFF minima of the Columbia Valley and Southern United States Rockies. With the exception of modern times, C accumulation is high during this unique vegetation period and high IFF of the mid Holocene. Local site factors of fire regime and vegetation composition seem to control carbon accumulation in the Kootenay Valley, however, additional factors need to be considered.
Additional local site factors in the MS and ESSF zones that can control fire-vegetation-carbon accumulation dynamics may include humans (Johnson and Larsen, 1991), aspect and erosion (Mustaphi and Pisaric, 2017), and disturbance (e.g., bark beetle outbreaks and lightning).
The two most dramatic shifts in vegetation at Marion Lake occur firstly in the early Holocene/late Pleistocene in ML Zone 3 (11,010–10,180 cal. yr. B.P.), likely reflecting the Younger Dryas cooling followed by early Holocene xerothermic warming noted by the increased presence of the dry adapted conifer, Douglas-fir (Pseudotsuga menziesii) and increasing fire frequency. The second most prominent change occurred at the transition from ML Zone 5 through 6a (∼2,500 cal. yr. B.P.). This zone transitions from a warmer to a cooler/wetter climate as indicated by the increase in western hemlock (T. heterophylla) and subsequent drop in fire frequency.
Management Implications
Fire and vegetation management decisions are based on the assessment of current vegetation, fire activity and their long-term range of natural variability (Hallett and Walker, 2000). Climate projections for the Kootenay Valley expect a +1°C increase in mean annual temperature by 2100 (Fraser Basin Council, 2019). These temperature changes would be analogous to conditions inferred to have occurred in Southeastern British Columbia during the xerothermic period. If climate was to become similar to xerothermic conditions, vegetation and fire regimes may look similar to what we have interpreted here for the xerothermic. This would indicate Dog Lake would move from the warm, wet –closed modern MS landscape to a warm, dry, open landscape typified by the current Interior Douglas Fir (IDF) zone (Hallett and Hills, 2006). The response around Marion Lake would likely be moving from the cool, wet-open landscape of the ESSF today to a warmer wet landscape that might resemble the modern MS landscape with vanishing meadows determinate on whether Abies lasiocarpa continues its most recent increase and establishes closed stands around these meadows. If fire frequency were to increase and drier conditions prevail, a dry meadow-laden and later ESSF forest might give way to grasslands (Heinrichs et al., 2001) or a landscape typified by the current IDF. Dog Lake’s probable return to a dry-open IDF forest type is within the natural climatic, vegetation, and fire conditions of the Kootenay Valley. The ESSF landscape in the Kootenay Valley around Marion Lake has only been established since the Neoglacial (last 4,500 cal yr. B.P.) and markedly subject to future fire regime variability.
Understanding a local disturbance regime within an Ecosystem-based Management (EBM) framework has become a more holistic approach to ecological management within conservation agencies like Parks Canada (Parks Canada and the Canadian Parks Council, 2008). EBM is place-based and employs ecological knowledge to inform planning decisions (Caldwell, 1970; Grumbine, 1994; Slocombe, 1998). Engelmann spruce and subalpine fir are the climax tree species in the ESSF (BC Ministry of Forests, 1991), and both species live a relatively long time (>100 years). Subalpine fir pollen percentages have steadily decreased from ∼1,000 years toward the present, indicating a shift toward a drier climate within the ESSF around Marion Lake. Over the same time period, the sediment core at Marion Lake shows fire frequency indicating a moderate fire regime and a vegetation signal representing a potential seral shift. If drier conditions persist and the climate continues to warm, then a complete BEC regime change around Marion Lake change might occur as MS expands into the area. Climate Envelope models indicate BEC level changes in ecosystems at the study sites by 2025 (Hamann and Wang, 2006). As of today this change has not been observed supporting the need to examine empirically based information such as paleoecological research that documents vegetation change in response to climate and disturbance. Without the consideration of disturbance such as fire, the needed mechanisms of ecosystem change are lacking and caution must be used when interpreting these models. This paleoecological research provides land managers with an understanding of how ecosystems have responded to climate change and changes in disturbance regimes at the locations they are interested in managing. This will give better insight in how and what to monitor, restore, adapt to, or to direct in terms of ecosystem management (Pellatt, 2002; Schuurman et al., 2021). Thinking about an area’s ecological trajectory alongside a management strategy, a manager can use paleoecological data to influence decisions with respect to more locally controlled human-controlled disturbances (e.g., fire) and any potential restoration efforts (Cote and Darling, 2010).
Management priorities in Kootenay National Park seek to improve the condition of native biodiversity through active management programs, increase understanding of the impact of climate change, and develop adaptation strategies (Parks Canada, 2010). Our paleoecological analysis of Holocene vegetation from Marion Lake, when coupled with climate projections for the end of the century, indicate that the warmer, drier IDF biogeoclimatic zone will most likely expand in the future. The ecological trajectory around Dog Lake will likely fit this pattern moving from the MS to the IDF zone as the climate continues to become drier (Wang et al., 2016) and if forest fires become more prevalent. Characteristic of the IDF is Pseudotsuga. Pseudotsuga has been identified as a tree that may become maladapted if climate changes too rapidly (St. Clair and Howe, 2007). One ecological restoration adaptation strategy that addresses this problem is assisted migration. Assisted migration can include planting seedlings adapted to future climates either through breeding or genetic manipulation and transfer from one perceived climatic setting to another (O’Neill et al., 2008).
Limitations
Making natural resource decisions based on the response of vegetation using BEC zones has its limitations. Most notably, the spatial scale of the BEC system reduces complexity to a level that ignores a host of species-level responses to drivers that are often the focus of restorative efforts. This loss of site complexity may improperly assign goals for making resiliency-focused decisions.
This study focused on two sites within the Kootenay Valley. However, when considering implications for management, spatial complexity among sites, the focus needs to be on the watershed and site level. In the example of fire history reconstruction our two sites show significant asynchrony. Asynchrony between sites close in proximity has been documented on the coast of British Columbia coast (Murphy et al., 2019). This asynchrony between sites that are in close proximity highlights the need to create site-specific fire-related management decisions around the natural range of variability produced by fire reconstructions (Whitlock et al., 2003).
Conclusion
Marion and Dog lake provide evidence for long-term climate variation for southern interior BC. Wetter summers are likely the driver for decreased fire frequency and low C accumulation rate. The Marion Lake pollen record begins over 12,000 cal yr. BP and reflects changes in herbaceous pollen such as Artemisia corresponding with the Younger Dryas period. This earlier signal is likely due to higher exposure and maintains a modern landscape due to early establishment of subalpine meadows. Dog lake switches to a more closed landscape in the Neoglacial likely due to wetter conditions reflected in increase in C accumulation due to increased biomass on the landscape. Cool/wet conditions become well established at Marion Lake ∼2500 cal yr. BP highlighting how asynchronous responses of vegetation to climate can be driven by elevation, site exposure, as well as edaphic factors.
Future climate predictions for the Kootenay Valley are expected to be relatively dry and hot, analogous to the xerothermic period. This would move the MS landscape around Dog Lake to a dry-open landscape typified by the current Interior Douglas Fir (IDF) zone. Around Marion Lake, the ESSF would likely transition to a MS-like landscape if a sufficient moisture regime (i.e., winter snowpack at higher elevations) is sufficient. If fire frequencies were to increase and drier grasslands were to prevail, then an IDF-like landscape may occur.
When considering management implications, spatial complexity between Marion and Dog Lakes needs to be highlighted. Identifying key local ecosystem characteristics should be assessed in terms of their ecological thresholds and addressed alongside any larger management directives.
Data Availability Statement
The raw data supporting the conclusions of this article will be made available by the authors, without undue reservation.
Author Contributions
All authors conceived and designed the research and contributed materials and analysis tools. TR and MP conducted sample collection. TR processed and analyzed samples. All authors wrote and edited the manuscript.
Funding
Parks Canada provided funding to MP. This work was supported by the Natural Sciences and Engineering Research Council (Discovery Grant RGPIN342251) to KK and Natural Sciences and Engineering Research Council Canada Research Chair Award to KK. TR was supported by SFU President’s Ph.D. Scholarship and International Student Travel Grant.
Conflict of Interest
The authors declare that the research was conducted in the absence of any commercial or financial relationships that could be construed as a potential conflict of interest.
Publisher’s Note
All claims expressed in this article are solely those of the authors and do not necessarily represent those of their affiliated organizations, or those of the publisher, the editors and the reviewers. Any product that may be evaluated in this article, or claim that may be made by its manufacturer, is not guaranteed or endorsed by the publisher.
Acknowledgments
We thank C. Barlow, M. Huntley, S. Goring, and S. Murphy for assistance in the field and lab. We also thank T. A. Minckley and D. Willard for constructive comments that improved the manuscript.
Supplementary Material
The Supplementary Material for this article can be found online at: https://www.frontiersin.org/articles/10.3389/fevo.2021.768785/full#supplementary-material
References
Bartlein, P., Anderson, K., Anderson, P., Edwards, M., Mock, C., Thompson, R., et al. (1998). Paleoclimate simulations for North America over the past 21,000 years: features of the simulation climate and comparisons with paleoenviornmental data. Quat. Sci. Rev. 17, 549–585.
BC Ministry of Forests (1991). Ecoystems of British Columbia. Engelmann Spruce –Subalpine Fir Zone. Victoria, BC: Research Branch in the Ministry of Forests.
Beaudoin, A. (1986). Using Picea/Pinus ratios from the Wilcox Pass core, Jasper National Park, Alberta, to investigate Holocene timberline fluctuations. Geogr. Phys. Q. 40, 145–152. doi: 10.7202/032634ar
Berauer, B. J., Wilfahrt, P. A., Arfin-Khan, M. A. S., Eibes, P., Von Heßberg, A., Ingrisch, J., et al. (2019). Low resistance of montane and alpine grasslands to abrupt changes in temperature and precipitation regimes. Arct. Antarct. Alp. Res. 51, 215–231.
Blaauw, M., and Christen, J. (2011). Bacon Manual v2.3.7. Avaialable online at: http://chrono.qub.ac.uk/blaauw/manualBacon_2.3.pdf (accessed January 2017).
Brown, T. A., Nelson, D. E., Mathewes, R. W., Vogel, J. S., and Southon, J. R. (1989). Radiocarbon dating of pollen extracted from peat samples by accelerator mass spectrometry. Quat. Res. 32, 205–212.
Brunelle, A., and Whitlock, C. (2003). Sedimentary charcoal as an indicator of late-Holocene drought in the Sierra Nevada, California and its relevance to the future. Holocene 13, 21–28.
Caldwell, L. (1970). The ecosystem as a criterion for public land policy. Nat. Resour. J. 10, 203–221.
Canadian Parks Council Climate Change Working Group (2013). Canadian Parks and Protected Areas: Helping Canada Weather Climate Change. Victoria, BC: Parks Canada Agency, 1–52.
Collison, B. (2021). Private Managed Forest: A Case Study of Logging Exploitation in the Kootenay Region of British Columbia. Ph.D. thesis, Halifax, NS: Dalhousie University.
Cote, I., and Darling, E. (2010). Rethinking ecosystem resilience in the face of climate change. PLoS Biol. 8:e1000438. doi: 10.1371/journal.pbio.1000438
Dakos, V., Carpenter, S., van Nes, E., and Scheffer, M. (2015). Resilience indicators: prospects and limitation for early warning of regime shifts. Philos. Trans. R. Soc. B 370:20130263.
Davis, E., Brown, R., Daniels, L., Kavanagh, T., and Gedalof, Z. (2020). Regional variability in the response of alpine treelines to climate change. Clim. Change 162, 1365–1384.
Davis, E., Mustaphi, C., and Pisaric, M. (2018). Forests, fire histories, and futures of Columbian and Rocky Mountain forests, western Canada. West. Geogr. 23, 3–11.
Dominguez-Garcia, V., Dakos, V., and Kefi, S. (2019). Unveiling dimensions of stability in complex ecological networks. Proc. Natl. Acad. Sci.U.S.A. 116, 25714–25720. doi: 10.1073/pnas.1904470116
Fraser Basin Council (2019). Climate Projections for the BC Northeast Region. Vancouver, BC: Fraser Basin Council, 1–46.
Gavin, D., Hu, F., Lertzman, K., and Corbett, P. J. (2006). Weak climatic control of stand scale fire history during the late Holocene. Ecology 87, 1722–1732. doi: 10.1890/0012-9658(2006)87[1722:wccosf]2.0.co;2
Gavin, D., Hu, F., Walker, I. R., and Westover, K. (2009). The Northern Inland temperate rainforest of British Columbia: old forests with a young history? Northwest Sci. 83, 70–78.
Glew, J., Smol, J., and Last, W. (2001). “Sediment core collection and extrusion,” in Tracking Environmental Changes Using Lake Sediments, Basin Analysis, Coring, and Chronological Techniques, eds W. Last and J. Smol (Dordrecht: Kluwer Academic Publishers), 73–105. doi: 10.3791/54363
Goring, S., Pellatt, M. G., Lacourse, T., Walker, I. R., and Mathewes, R. W. (2008). A new methodology for reconstructing climate and vegetation from modern pollen assemblages: an example from British Columbia. J. Biogeogr. 36, 626–638. doi: 10.1111/j.1365-2699.2008.02021.x
Gunderson, L., and Holling, C. (2002). Panarchy: Understanding Transformations in Human and Natural Systems. Washington DC: Island Press.
Hallett, D., and Hills, L. (2006). Holocene vegetation dynamics, fire history, lake level and climate change in the Kootenay Valley, southeastern British Columbia, Canada. J. Paleolimnol. 35, 351–371.
Hallett, D., Mathewes, R. W., and Walker, R. (2003). A 1000-year record of forest fire, drought and lake level change in southeastern British Columbia, Canada. Holocene 13, 751–761. doi: 10.1191/0959683603hl660rp
Hallett, D., and Walker, R. (2000). Paleoecology and its application to fire and vegetation management in Kootenay National Park, British Columbia. J. Paleolimnol. 24, 401–415.
Hamann, A., and Wang, T. (2006). Potential effects of climate change on ecosystem and tree species distribution in British Columbia. Ecology 87, 2773–2786. doi: 10.1890/0012-9658(2006)87[2773:peocco]2.0.co;2
Hansen, H. (1955). Postglacial forests in south central and central British Columbia. Am. J. Sci. 253, 640–658. doi: 10.1111/mec.13701
Hazell, S. (1979). Late Quaternary Vegetation and Climate of Dunbar Valley, British Columbia, Department of Botany. Ph.D. thesis, Toronto, ON: University of Toronto, 101.
Hebda, R. (1982). “Postglacial history of grasslands of southern British Columbia and adjacent regions,” in Proceeding of the Grassland Ecologic and Classification, eds A. Nicholson, A. McLean, and T. Baker (Victoria, BC: British Columbia Ministry of Forests), 157–191.
Hebda, R. (1995). British Columbia vegetation and climate history with focus on 6 KA BP. Geogr. Phys. Quat. 49, 55–79. doi: 10.7202/033030ar
Heinrichs, M. (1999). A Late Quaternary Paleoecological Analysis in the Engelmann Spruce-Subalpine Fir Biogeoclimatic Zone of the Okanagan/Ashnola Region, British Columbia, Canada, Department of Biology. Ph.D. thesis, Victoria, BC: University of Victoria, 205.
Heinrichs, M., Hebda, R., and Walker, I. (2001). Holocene vegetation and natural disturbance in the Engelmann spruce –Subalpine fir BEC zone at Mount Kobau, British Columbia. Can. J. For. Res. 31, 2183–2199.
Heinrichs, M., Hebda, R., Walker, I., and Palmer, S. (2002). Postglacial paleoecology and inferred paleoclimate in the Engelmann spruce-subalpine fir forest of south-central British Columbia, Canada. Palaeogeogr. Palaeoclimatol. Palaeoecol. 184, 347–369. doi: 10.1016/s0031-0182(02)00274-2
Higuera, P., Gavin, D., Bartlein, P., and Hallett, D. (2010). Peak detection in sediment-charcoal records: Impacts of alternative data analysis methods on fire-history interpretations. Int. J. Wildl. Fire 19, 996–1014. doi: 10.1071/wf09134
Higuera, P., Whitlock, C., and Gage, J. (2011). Linking tree-ring and sediment-charcoal records to reconstruct fire occurrence and area burned in subalpine forest of Yellowstone National Park, USA. Holocene 21, 327–341. doi: 10.1177/0959683610374882
Huerta, M., Whitlock, C., and Yale, J. (2009). Holocene vegetation-fire-climate linkages in northern Yellowstone National Park, USA. Palaeogeogr. Palaeoclimatol. Palaeoecol. 271, 170–181. doi: 10.1016/j.palaeo.2008.10.015
Johnson, E., and Larsen, C. (1991). Climatically induced change in fire frequency in the southern Canadian Rockies. Ecology 72, 194–201. doi: 10.2307/1938914
Keane, R., Arno, S., and Brown, J. (1990). Simulating cumulative fire effects in Ponderosa pine/Douglas-fir forests. Ecology 71, 189–203. doi: 10.2307/1940259
Kearney, M., and Luckman, B. (1983). Holocene timberline fluctuations in Jasper National Park, Alberta. Science 221, 261–263. doi: 10.1126/science.221.4607.261
Kéfi, S., Guttal, V., Brock, W., Carpenter, S., Ellison, A., Livina, V., et al. (2014). Early warning signals of ecological transitions: methods for spatial patterns. PLoS One 9:e92097. doi: 10.1371/journal.pone.0092097
Kershaw, L., MacKinnon, A., and Pojar, J. (1998). Plants of the Rocky Mountains, ed. N. Foulds (Edmonton, AB: Lone Pine Publishing).
Kutzbach, J., Gallimore, R., Harrison, S., Behling, P., Selin, R., and Laarif, F. (1998). Climate and biome simulations for the past 21,000 years. Quat. Sci. Rev. 17, 473–506. doi: 10.1016/s0277-3791(98)00009-2
Levesque, A., Mayle, F., Walker, I., and Cwynar, L. (1993). A previously unrecognized late-glacial cold event in eastern North America. Nature 316, 623–626. doi: 10.1038/361623a0
Long, C., Whitlock, C., Bartlein, P., and Millspaugh, S. (1998). A 9,000-year fire history from the Oregon Coast Range, based on a high-resolution charcoal study. Can. J. For. Res. 28, 774–782. doi: 10.1139/x98-051
Luckman, B. (1995). Calendar-dated, early “Little Ice Age” glacier advance at Robson Glacier, British Columbia, Canada. Holocene 5, 149–159.
Luckman, B., and Kearney, M. (1986). Reconstruction of Holocene changes in alpine vegetation and climate in the Maligne Range, Jasper National Park, Alberta. Quat. Res. 26, 244–261. doi: 10.1016/0033-5894(86)90108-0
Lynch, J., Clark, J., Stocks, B., Lyu, L., and Zhang, Q. (2004). Charcoal production, dispersal, and deposition from the Fort Providence experimental fire: interpreting fire regimes from charcoal records in boreal forests. Can. J. For. Res. 34, 1642–1656. doi: 10.1139/x04-071
Mack, R., Rutter, N., and Valastro, S. (1983). Holocene vegetational history of the Kootenai River Valley, Montana. Quat. Res. 20, 177–193.
Mack, R., Rutter, N., Valastro, S., and Bryant, V. (1978). Lake quaternary vegetation history at Waits Lake, Colville River Valley, Washington. Bot. Gaz. 139, 499–506. doi: 10.1086/337025
Mathewes, R., and Rouse, G. (1975). Palynology and paleoecology of postglacial sediments from the Lower Fraser River Canyon of British Columbia. Can. J. Earth Sci. 12, 745–756.
Mathewes, R. W. (1973). A palynological study of postglacial vegetation changes in the University Research Forest, southern British Columbia. Can. J. Bot. 51, 2085–2103. doi: 10.1139/b73-271
Mathewes, R. W. (1985). “Paleobotanical evidence for climatic change in southern British Columbia during late-glacial and Holocene time,” in Climatic Change in Canada 5: Critical Periods in the Quaternary Climatic History of Northern North America Syllogeus, ed. C. R. Harinton (Ottawa, ON: National Museum of Natural Sciences).
Mathewes, R. W. (1993). Evidence for Younger Dryas-age cooling on the North Pacific coast of America. Quat. Sci. Rev. 12, 321–331. doi: 10.1016/0277-3791(93)90040-s
Mathewes, R. W., Heusser, L., and Patterson, R. (1993). Evidence for a Younger Dryas-like cooling event on the British Columbia coast. Geology 21, 101–104. doi: 10.1130/0091-7613(1993)021<0101:efaydl>2.3.co;2
Mayewski, P., Rohling, E., Stager, C., Karlen, K., Maasch, K., Meeker, L., et al. (2004). Holocene climate variability. Quat. Res. 62, 243–255.
Meidinger, D., and Pojar, J. (1991). Ecosystems of British Columbia. British Columbia Special Report Series 06. Victoria, BC: British Columbia Ministry of Forests.
Meyers, P., and Teranes, J. (2001). “Sediment organic matter,” in Tracking Environmental Changes Using Lake Sediments, Basin Analysis, Coring, and Chronological Techniques, Vol. 2, eds W. Last and J. Smol (Dordrecht: Kluwer Academic Publishers), 239–270.
Ministry of Forests and Range (2008). Glossary of Forestry Terms in British Columbia. Victoria, BC: Ministry of Forests and Range
Morris, J., Brunelle, A., DeRose, R., Seppa, H., Power, M., Carter, V., et al. (2013). Using fire regimes to delineate zones in a high-resolution lake sediment record from the western United States. Quat. Res. 79, 24–36. doi: 10.1016/j.yqres.2012.10.002
Murphy, S., Pellatt, M. G., and Kohfeld, K. (2019). A 5,000-year fire history in the Strait of Georgia Lowlands, British Columbia, Canada. Front. Ecol. Evol. 7:90. doi: 10.3389/fevo.2019.00090
Mustaphi, C., Davis, E., Perreault, J., and Pisaric, M. (2015). Spatial variability of recent macroscopic charcoal deposition in a small montane lake and implications for reconstruction of watershed-scale fire regimes. J. Paleolimnol. 54, 71–86. doi: 10.1007/s10933-015-9838-2
Mustaphi, C., and Pisaric, M. (2014). Holocene climate-fire-vegetation interactions at a subalpine watershed in southeastern British Columbia, Canada. Quat. Res. 81, 228–239. doi: 10.1016/j.yqres.2013.12.002
Mustaphi, C., and Pisaric, M. (2017). Forest vegetation change and disturbance interactions over the past 7500 years at Sasquatch Lake, Columbia Mountains, western Canada. Quat. Int. 488, 95–106. doi: 10.1016/j.quaint.2017.03.045
O’Neill, G., Ukrainetz, N., Carlson, M., Cartwright, C., Jaquish, B., King, J., et al. (2008). Assisted Migration to Address Climate Change in British Columbia: Recommendations for Interim Seed Transfer Standards. Victoria, BC: Ministry of Forests and Range Forest Science Program.
Palmer, S., Walker, I. R., Heinrichs, M., Hebda, R., and Scudder, G. (2002). Postglacial midge community change and Holocene paleotemperature reconstructions near treeline, southern British Columbia Canada. J. Paleolimnol. 28, 469–490.
Parks Canada (2010). Kootenay National Park of Canada management plan. Radium Hot Springs, BC: Parks Canada.
Parks Canada Agency (2000). Unimpaired for Future Generations”? Protecting Ecological Integrity Within Canada’s National Parks. Ottawa, ON: Canada’s National parks.
Parks Canada and the Canadian Parks Council (2008). Principles and Guidelines for Ecological Restoration in Canada’s Protected Natural Area. Gatineau, QC: Parks Canada Agency.
Pellatt, M. G., Smith, M., Mathewes, R., and Walker, I. R. (1998). Palaeoecology of postglacial treeline shifts in the northern Cascade Mountains, Canada. Palaeogeogr. Palaeoclimatol. Palaeoecol. 141, 123–138.
Pellatt, M. G. (2002). “The role of paleoecology in understanding ecological integrity: an example from a highly fragmented landscape in the strait of Georgia Lowlands,” in Managing Protected Areas in a Changing World, eds S. Bondrop-Nielson, N. Munro, G. Nelson, J. H. M. Willison, T. Herman, and P. Eagles. (Wolfville, CA: SAMPAA), 384–397.
Pellatt, M. G., and Mathewes, R. W. (1997). Holocene Tree Line and climate change on the Queen Charlotte Islands, Canada. Quat. Res. 48, 88–99. doi: 10.1006/qres.1997.1903
Pellatt, M. G., Mathewes, R. W., and Walker, I. R. (1997). Pollen analysis and ordination of lake sediment-surface samples from coastal British Columbia, Canada. Can. J. Bot. 75, 799–814. doi: 10.1139/b97-090
Pellatt, M. G., Smith, M., Mathewes, R. W., Walker, I. R., and Palmer, S. (2000). Holocene treeline and climate change in subalpine zone near Stoyma Mountain, Cascade Mountains, southwestern British Columbia, Canada. Arct. Antarct. Alp. Res. 32, 73–83. doi: 10.2307/1552412
Peteet, D., Nichols, J., and Mann, D. (2019). Holocene vegetation, climate, and carbon history on western Kodiak Island, Alaska. Front. Earth Sci. 7:61. doi: 10.3389/feart.2019.00061
Ramiadantsoa, T., Stegner, M., Williams, J., and Ives, A. (2019). The potential role of intrinsic processes in generating abrupt and quasi-synchronous tree declines during the Holocene. Ecology 100, 1–15. doi: 10.1002/ecy.2579
Reasoner, M., and Jodry, M. (2000). Rapid response of alpine timberline vegetation to the Younger Dryas climate oscillation in the Colorado Rocky Mountains, USA. Geology 28, 51–54. doi: 10.1130/0091-7613(2000)028<0051:rroatv>2.3.co;2
Reasoner, M., Osborn, G., and Rutter, N. (1994). Age of the Crowfoot advance in the Canadian rocky mountains; a glacial event coeval with the Younger Dryas oscillation. Geology 22, 439–442. doi: 10.1130/0091-7613(1994)022<0439:aotcai>2.3.co;2
Ritchie, J., and Lichti-Fedorovich, S. (1967). Pollen dispersal phenomena in artic subarctic Canada. Rev. Palaeobot. Palynol. 3, 255–266.
Rosenberg, S., Walker, I., Mathewes, R. W., and Hallett, D. (2004). Midge-inferred Holocene climate history of two subalpine lakes in southern British Columbia, Canada. Holocene 14, 258–271. doi: 10.1191/0959683604hl703rp
Schuurman, G. W., Cole, D. N., Cravens, A. E., Covington, S., Crausbay, S. D., Magness, D. R., et al. (2021). Navigating ecological transformation: resist–accept–direct as a path to a new resource management paradigm. BioScience doi: 10.1093/biosci/biab067
Slocombe, D. (1998). Lessons from experience with ecosystem-based management. Landsc. Urban Plan. 40, 31–39. doi: 10.1002/ieam.1782
Smith, M., Pellatt, M. G., Walker, I., and Mathewes, R. W. (1998). Postglacial changes in chronomid communities and inferred climate near treeline at Mount Stoyoma, Cascade Mountains, southwestern British Columbia. J. Paleolimnol. 20, 277–293.
Southon, J., Vogel, J., Trumbore, S., Davis, J., Roberts, M., Caffee, M., et al. (2016). Progress in AMS measurements at the LLNL Spectrometer. Radiocarbon 34, 473–477. doi: 10.1017/s0033822200063712
St. Clair, J., and Howe, G. (2007). Genetic maladaptation of coastal Douglas-fir seedlings to future climates. Glob. Change Biol. 13, 1441–1454. doi: 10.1111/j.1365-2486.2007.01385.x
Stuiver, M., Reimer, P., and Reimer, R. (2021). CALIB 8.2 [WWW program]. Availble online at: http://calib.org (accessed November 11, 2021).
Thilenius, J. (1990). Woody plant succession on earthquake-uplifted coastal wetlands of the Copper River Delta, Alaska. For. Ecol. Manag. 33-34, 439–462. doi: 10.1016/0378-1127(90)90209-t
Trant, A., Higgs, E., and Starzomski, B. (2020). A century of high elevation ecosystem change in the Canadian rocky mountains. Nat. Sci. Rep. 20:9698.
van den Bos, V., Newham, R., Rees, A., and Woods, L. (2020). Density separation in pollen preparation: how low can you go? J. Paleolimnol. 63, 225–234. doi: 10.1007/s10933-020-00112-6
Walker, I. R., and Pellatt, M. G. (2003). Climate change in coastal British Columbia a paleoenvironmental perspective. Can. Water Resour. J. 28, 531–566.
Walker, I. R., and Pellatt, M. G. (2008). Climate change and ecosystem response in the northern Columbia River basin –a paleoenvironmental perspective. Environ. Rev. 16, 113–140.
Walsh, M. K., Marlon, J. R., Goring, S. J., Brown, K. J., and Gavin, D. G. (2015). A regional perspective on holocene fire-climate-human interactions in the Pacific Northwest of North America. Ann. Am. Assoc. Geogr. 105, 1135–1157. doi: 10.1080/00045608.2015.1064457
Wang, T., Hamann, A., Spittlehouse, D., and Carroll, C. (2016). Locally downscaled and spatially customizable climate data for historical and future periods for North America. PLoS One 11:e0156720. doi: 10.1371/journal.pone.0156720
Wang, Y., Shipley, B., Lauer, D., Pineau, R., and McGuire, J. (2020). Plant biomes demonstrate that landscape resilience today is the lowest it has been since end-Pleistocene megafaunal extinctions. Glob. Change Biol. 15299, 1–14. doi: 10.1111/gcb.15299
Whitlock, C., and Millspaugh, S. (1996). Testing the assumptions of fire-history studies: an examination of modern charcoal accumulation in Yellowstone National Park, USA. Holocene 6, 7–15.
Whitlock, C., Shafer, S., and Marlon, J. (2003). The role of climate and vegetation change in shaping past and future fire regimes in the northwestern US and the implications for ecosystem management. For. Ecol. Manag. 178, 5–21. doi: 10.1111/cobi.12960
Keywords: fire history, National Park, Holocene, pollen analysis, British Columbia, charcoal analysis, climate change, paleoecology
Citation: Rodengen TJ, Pellatt MG and Kohfeld KE (2022) Paleoecological Investigation of Vegetation, Climate and Fire History in, and Adjacent to, Kootenay National Park, Southeastern British Columbia, Canada. Front. Ecol. Evol. 9:768785. doi: 10.3389/fevo.2021.768785
Received: 01 September 2021; Accepted: 30 November 2021;
Published: 12 January 2022.
Edited by:
Pierre Grondin, Ministère des Forêts, de la Faune et des Parcs, CanadaReviewed by:
Thomas A. Minckley, University of Wyoming, United StatesDebra Willard, United States Geological Survey (USGS), United States
Copyright © 2022 Rodengen, Pellatt and Kohfeld. This is an open-access article distributed under the terms of the Creative Commons Attribution License (CC BY). The use, distribution or reproduction in other forums is permitted, provided the original author(s) and the copyright owner(s) are credited and that the original publication in this journal is cited, in accordance with accepted academic practice. No use, distribution or reproduction is permitted which does not comply with these terms.
*Correspondence: Marlow G. Pellatt, bWFybG93LnBlbGxhdHRAcGMuZ2MuY2E=