- 1South African Environmental Observation Network (SAEON), Elwandle Coastal Node, Gqeberha, South Africa
- 2Department of Botany and the Institute for Coastal and Marine Research, Nelson Mandela University, Gqeberha, South Africa
Benthic diatoms form an important component of the microphytobenthos and have long been utilised as suitable bioindicators in aquatic systems. However, knowledge on benthic diatom community succession on hard substrata (biofilm) remains understudied in austral marine coastal systems. In this study, we investigated benthic diatom colonisation on artificial substrates (Plexiglass) over a period of 5 weeks at two locations with different physical environments along the warm temperate coast of South Africa. Results revealed relatively similar physico-chemical conditions but highly contrasting diatom community development were observed between the two sites. While there were some shared taxa, site-specific dynamics resulted in significantly different diatom species diversity and richness, facilitated by common (e.g., Nitzschia ventricosa and Cocconeis scutellum) and a large percentage of rarely observed species such as Cocconeis testudo and Lyrella lyra. A total of 134 species belonging to 44 genera were observed during the study. The overall diatom composition differed spatio-temporally during the experimental period, with the fluctuating species occurrences and abundances highlighting the rapid microalgal species turnover within days, under natural conditions. Environmental variables were shown to have varying influences as drivers of the diatom community descriptors. Multivariate modelling confirmed that study site and the interaction between site and sampling occasion were important predictors of diatom abundances, and the overall observed community composition. The current results suggest that benthic diatoms on artificial substrata could be incorporated as suitable indicators of change along the coastline subject to further investigations, taking into account site-specific differences driven by habitat complexity and environmental variability. The experimental method proved to be efficient and can be implemented to study the response of benthic diatoms to localised nutrient enrichment around the coastline.
Introduction
Increasing human populations around the world’s coastal regions have amplified anthropogenic impacts such as increased pollution (e.g., excess nutrient inputs) (Islam and Tanaka, 2004). These human-driven impacts affect the coastal oceans’ biogeochemistry (Doney, 2010), thus influencing the ecosystem health and ultimately the public health (Islam and Tanaka, 2004; Fleming et al., 2006). The monitoring of coastal and nearshore marine waters became increasingly popular, particularly in Europe, to assess and monitor their ecological status (see Borja and Dauer, 2008; Borja et al., 2009 and reviews Beiras, 2016; Borja et al., 2016; Danovaro et al., 2016; Cocquempot et al., 2019). There is an urgent need for similar monitoring of the coastal waters of southern Africa, considering the importance of baseline studies for data scarce regions given their increased susceptibility to deteriorating water quality (Wepener and Degger, 2012). The occurrence and dominance of marine benthic diatoms in coastal nearshore waters has recently been documented for this region (Cotiyane-Pondo et al., 2021).
The ecological significance of the microphytobenthos is well known (MacIntyre et al., 1996) and benthic diatoms (Bacillariophyceae) are an important component of the epilithic microphytobenthos (EMPB), succeeding bacterial colonisation (Edyvean et al., 1985; MacLulich, 1986). The initial colonisation by these microalgae facilitates the secondary colonisation of the substrate by other micro-and-macroflora and fauna (Round, 1981). Due to their unique characteristics (e.g., short lifespan, rapid response to change, etc.), benthic diatoms have been employed as suitable indicators in the monitoring of freshwater systems, further resulting in the formulation and implementation of diatom-based indices (Kelly and Whitton, 1995; Kelly et al., 1998; Potapova and Charles, 2007; Taylor et al., 2007; Dalu and Froneman, 2016). Continuous advancements in diatom research have shed more light on the use of diatoms in freshwater biomonitoring (e.g., Rimet and Bouchez, 2012; Dalu et al., 2020; Kelly et al., 2020a,b). Despite the progressive knowledge on the use of diatoms as water quality indicators in freshwater systems, the adoption of similar techniques in coastal marine systems is lacking (Desrosiers et al., 2013), but slowly gaining momentum (Desrosiers et al., 2014). A recent study by Kafouris et al. (2019) also highlighted benthic diatoms from sediment samples as reliable predictors of eutrophication in an oligotrophic coastal ecosystem.
The dynamic nature of coastal ecosystems, driven by their natural cycles and coupled with variable fluctuations, necessitate the use of cost-effective assessment tools (Beiras, 2016). Coastal marine environments such as intertidal rocky shores, have long been ideal environments for experimental ecological studies using artificial structures as surrogates of natural systems. This has advanced our knowledge of intertidal organisms, their biological interactions and response to abrupt intertidal environmental extremes (Hawkins et al., 2020). Hard substrates are preferable in ecological assessments of aquatic systems (e.g., freshwater systems, Kelly et al., 1998). Hence, the understanding of the colonisation dynamics of EMPBs on artificial substrates in different coastal regions is central to the global standardisation and use of epilithic diatoms in coastal bioassessments (Desrosiers et al., 2013, 2014) as well as restoration initiatives (Pennesi and Danovaro, 2017). One of the benefits of artificial substrata, it allows for the standardisation of sampling methods, investigation of diatom assemblages influenced by the water column physico-chemistry rather than the substrate (Desrosiers et al., 2014), and the deployment of experimental substrata at different locations of interest.
Studies of marine diatom colonisation on artificial substrates (e.g., Caribbean, Desrosiers et al., 2014; Antarctica, Zidarova et al., 2020) are non-existent in southern Africa, other than the foundational studies in freshwater (Dalu et al., 2014) and estuarine (Nunes et al., 2019) systems in South Africa. To fill this gap, the aim of this study was to assess and compare diatom colonisation and development on artificial substrata at two coastal locations. This was achieved through quantifying the spatial (i.e., between sites) and temporal (i.e., between weeks) variations in diatom assemblages and the local environmental conditions. The sites were selected based on their contrasting oceanographic conditions, physical environment, and anthropogenic influences but similar climate within the 10 km distance. We hypothesised that: (1) diatom assemblages will differ between the initial colonisation stage (early succession) and the stable community stage (late succession) at both sites; (2) the diatom assemblages will be similar between the two sites, primarily due to their close proximity and the same artificial substrate used. We further aimed to investigate the potential influence of environmental conditions on observed diatom community variations. This research is responsive to the call to evaluate the potential use of benthic diatoms for biomonitoring in coastal ecosystems (Desrosiers et al., 2013); provides evidence and a better understanding of the dynamics of benthic diatoms over a small spatial and short-temporal scale; and is the first baseline assessment of this nature in southern African coastal marine waters.
Materials and Methods
Study Location
This study was conducted at two sites located approximately 10 km apart in the shallow subtidal zone of the coastline near Gqeberha (Port Elizabeth), South Africa (Figure 1). The sites are in the warm-temperate, south-east coast section of the Agulhas bioregion (Griffiths et al., 2010) and the coastline conditions are influenced by the southward-flowing warm Agulhas Current, wind driven upwelling and semi-diurnal tides with a microtidal range of ∼2 m (Schumann et al., 2005; Goschen et al., 2012). Coastal current speeds of <10 cm s–1 have been documented in this region and the currents vary spatio-temporally (Schumann et al., 2005). Site 1 (Cape Recife: 34°01′36″ S, 25°42′03″ E) is located on the far western sector (headland) of Algoa Bay, is part of the Cape Recife Nature Reserve and characterised by an exposed intertidal area composed of a relatively flat sandstone substrate and subjected to low wave action. Wind-driven coastal upwelling occurs at Cape Recife mostly due to north-easterly winds in summer (Beckley, 1983; Goschen and Schumann, 1995), along with sea-surface temperature fluctuations between 12°C and 27°C (Beckley, 1983). There is a wastewater treatment plant (WWTP) situated within the Cape Recife Nature Reserve that discharges into the coastal nearshore environment (Lemley et al., 2019). The WWTP outlet is ∼2 km north of the study site. Site 2 (Willows Resort: 34°02′43″ S, 25°36′24″ E) is located west of Cape Recife, characterised by large rock outcrops of Table Mountain quartzite and is exposed to strong wave action driven by the dominant south-westerly winds. These surface winds create unpredictable high-energy swells along this section of the coast (locally referred to as the Wild Side) and thus sea-surface temperature fluctuations are more pronounced (Beckley, 1983). In situ data collected during this study showed daily temperature fluctuations within the study area, ranging between 16°C and 23°C at Site 1 and 14°C and 20°C at Site 2 (Supplementary Figure S1). Both sites are located in areas with controlled access and this was important in limiting possible vandalism/theft of the experimental setup during the study.
Experimental Design
Benthic diatom colonisation was monitored for a period of 5 weeks following recommendations by Desrosiers et al. (2014) using frosted and roughly hand-sanded Plexiglass® panels (10 × 10 cm, with 5 mm thickness). A total of 15 panels for each site were placed vertically, with a 3.5 cm distance between them, on a steel cage attached to railway line anchors (Supplementary Plate S1). The cages were deployed on the same day, within 20 min of each other, at an average depth of 0.5–0.8 m at spring low tide (and at ∼1.8 m at spring high tide). At this depth, light availability was considered constant at both sites, however, it was expected that hydrographic conditions would create turbid conditions due to the resuspension of the benthic substrate, detritus and detached macroalgae. To assess diatom colonisation, sample collection was conducted on a weekly basis from 14 October to 12 November 2020 (austral Spring season) during low tide (for easy access to the experimental setup) and within the same hour to minimise the temporal differences between the two sites during sampling.
Water Column Conditions and Biomass
In situ physico-chemical variables (temperature - °C, dissolved oxygen: DO - mg l–1, salinity, and turbidity in Formazin Nephelometric Units - FNU) were measured at the sub-surface and near-bottom (next to the cage) using a YSI ProDSS multiprobe (YSI Incorporated, United States). The pH was measured using either the ProDSS multiprobe or an AquaLytic pH meter fitted with the SensoDirect probe. Field conditions such as wind speed and wave action were recorded during each sampling occasion. Small conductivity/temperature data loggers (Star-Oddi, Iceland) were deployed ∼0.5 m from the experimental cage to record hourly temperature data during the study period. Triplicate (500 ml) water samples were collected at the same depth as the cages for inorganic nutrient determinations. The water samples were gravity-filtered through Whatman® GF/F filters (47-mm diameter, 0.7-μm pore size) in the laboratory, the filters and filtrates were immediately frozen at −20°C and the latter were analysed for dissolved inorganic nitrogen (DIN: sum of nitrate [NO3–], nitrite [NO2–] and ammonium [NH4+]), phosphorus [DIP: PO43–], and dissolved silicate [DSi: SiO2] concentrations using a SEAL AA3 AutoAnalyser (SEAL Analytical, Inc.) following Bate and Heelas (1975) and Parsons et al. (1984). Water column chlorophyll-a (Chl-a—used as proxy for phytoplankton biomass) was extracted from the GF/F filters using 10 ml of 95% ethanol (Merck® 4111) for 24 h at ∼2°C in the dark. After extraction, samples were re-filtered and Chl-a concentrations (expressed as μg Chl-a l–1) were determined at 665 nm before and after acidification of the extracts with 1N HCl (Nusch, 1980) using a Hitachi UH5300 Spectrophotometer.
Diatom Sampling, Benthic Biomass and Analyses
At each site, three replicate Plexiglass® panels were chosen at random on a weekly basis, carefully removed from the cages and placed in sterile plastic containers then filled with 250 ml of Milli-Q® Type-1 ultrapure water. The panels were then brushed (on both sites) using a sterile toothbrush to remove the epilithic material, resulting in a homogenised 250 ml sample for each week, that was preserved with 2.5 ml of 25% glutaraldehyde solution (Grade II). Before preservation, the homogenised sample was vigorously shaken and a 50 ml subsample was filtered through the Whatman® GF/F filters, processed and analysed for the estimation of benthic Chl-a biomass (expressed as mg Chl-a m–2) following the spectrophotometer method described above. However, due to a rapid colonisation by green and brown macroalgal spores, causing high Chl-a biomass on the panels at Site 1 (Cape Recife) and the presence of marine intertidal snails (Oxystele sinensis) grazing on the biofilm at Site 2 (Willow Resort), the benthic Chl-a biomass samples were discontinued after Week 3 to avoid measuring the macroalgal biomass at Site 1 and the reduced biomass caused by grazing at Site 2.
To analyse the diatom assemblages, 15 ml subsamples were pre-treated with 10% hydrochloric acid (HCl) and thereafter poured through a 200 μm mesh to remove the remaining macroalgal filaments and calcareous organisms. The subsamples were treated with concentrated nitric acid (HNO3) and potassium dichromate (K2Cr2O7) and left for 24 h on a mini-shaker (100 rpm) at room temperature. The diatom samples were rinsed repeatedly with Milli-Q® Type-1 ultrapure water. Coverslips with cleaned diatom valves were permanently mounted on glass slides using Pleurax. Thereafter, diatom counts and the subsequent species identification to lowest taxonomic level possible (either genus or species) were performed using a Zeiss AxioObserver A1 light microscope with Differential Interference Contrast (DIC) at 630× and 1000× (under oil immersion) magnification. A minimum of 400 valves per sample were counted and presented as relative abundance (RA in %). Taxonomic identifications were made using a range of literature (Giffen, 1966; Witkowski et al., 2000; Al-Handal and Wulff, 2008a,b; Lobban et al., 2012; Álvarez-Blanco and Blanco, 2014; Al-Handal et al., 2016; Park et al., 2018).
Numerical Analyses
All analyses were performed using the R statistical environment (R v4.0.3; R Core Team, 2020). Data normality were evaluated using the Shapiro–Wilk test (α < 0.05). Thereafter, depending on data distribution, the Independent t-test (parametric) and Wilcoxon test (non-parametric) were applied to assess the differences between the two sites.
In assessing diatom assemblages, the diatom dataset was reduced by only considering taxa with ≥1% relative abundance (66 species – sites combined) in statistical analyses. Diatom composition between two sites (β-diversity) was assessed, firstly by checking the multivariate homogeneity of group dispersions (variances) using the betadisper function. The permutest function (999 permutations) was used to test if the variances were homogeneous and the result was visualised as a boxplot. Thereafter, permutational multivariate ANOVA (PERMANOVA) using the adonis2 function was computed to test for compositional differences (by site) and the non-metric multidimensional scaling (nMDS) was then used to visualise the community composition differences between the sites and samples using the metaMDS function (data square-root transformed, Bray–Curtis dissimilarity, 999 permutations). The analyses functions used are from the ‘vegan’ R package (Oksanen et al., 2020).
We determined the alpha diversity (community descriptors: species richness – S, Shannon’s diversity – H′, Simpson’s dominance – D and Pielou’s evenness – J′) for each sample/week using the ‘vegan’ R package (Oksanen et al., 2020). Subsequently, the influence of measured environmental (predictor) variables on the community descriptors during the study period was assessed using the modelling approach. Prior to the analyses, collinearity among possible predictor variables was assessed using variance inflation factors (VIF), with VIFs > 5 deemed collinear (Zuur et al., 2009, 2010). Therefore, salinity, dissolved inorganic phosphorus and wind force were omitted from further analyses. Linear mixed-effects modelling (LMM) using the ‘lme4’ package (Bates et al., 2015) was then used to evaluate the influence of the predictor variables on diatom community descriptors (response variables, i.e., S, H′, D, and J′). For each response variable, the initial model included all predictor variables followed by a stepwise model selection (removal of non-significant terms). The models were fitted under restricted maximum likelihood (REML) estimation and then compared using Akaike Information Criterion (AIC), with the best fitted model resulting in low AIC score (Zuur et al., 2009, 2010). Site and sampling occasion were included in the models as random effects to account for spatial independence (autocorrelation).
Lastly, we used generalised linear modelling (GLMmv) for the multivariate abundance data using the ‘mvabund’ package (Wang et al., 2012), to analyse how the above-mentioned predictor variables affected the observed diatom taxa variations. The GLMmv were fitted to the community data using the manyglm function, jointly predicting abundance across multiple taxa with the error distributions specified as ‘negative binomial’ for count data (Wang et al., 2012). This approach is advantageous by fitting a separate GLM for each taxon, accounts for species relationships and provides a more predictive power compared to the conventional distance-based community analyses (Warton et al., 2012). Diatom taxa with ≥5% in at least one sample were grouped according to genus, to account for low abundances and allow for meaningful comparisons. The model to assess the effects of the categorical predictors (i.e., site and sample ‘sampling occasion’) was computed separately, followed by that for the continuous predictors (i.e., Temperature, DO, pH, Turbidity, DIN, and DSi), with the results presented separately. The effects (or deviance explained) of the predictor variables in the models were assessed using the anova.manyglm function (‘p.uni’ = “adjusted”; ‘nBoot’ = 1000; Wang et al., 2012). All analyses were computed at a significance level of α < 0.05.
Results
Pelagic Environment
Environmental variables revealed similar conditions at Site 1 (Cape Recife) and Site 2 (Willows Resort). From this, temperature (W = 76; p < 0.05; n = 20) and nutrients in the form of DIN (W = 22; p < 0.001; n = 30) and DIP [t(28) = −3.283; p < 0.001; n = 30] were the exception, and varied significantly between the two sites (Table 1 and Supplementary Table S1). Daily averaged in situ temperature measurements (using underwater CT loggers) varied between the sites, with significantly higher values recorded at Cape Recife (W = 67; p < 0.001; n = 1940) (Supplementary Figure S1). Water column biomass (Chl-a) did not show any variation between the two sites (p > 0.05), while benthic Chl-a showed a weekly increasing trend at both sites (Supplementary Table S1, data for Week 1–3), however, the differences in benthic biomass were not significant between the two sites (p > 0.05; Table 1). The weekly variations and field conditions during the experimental period are documented in Supplementary Table S1.
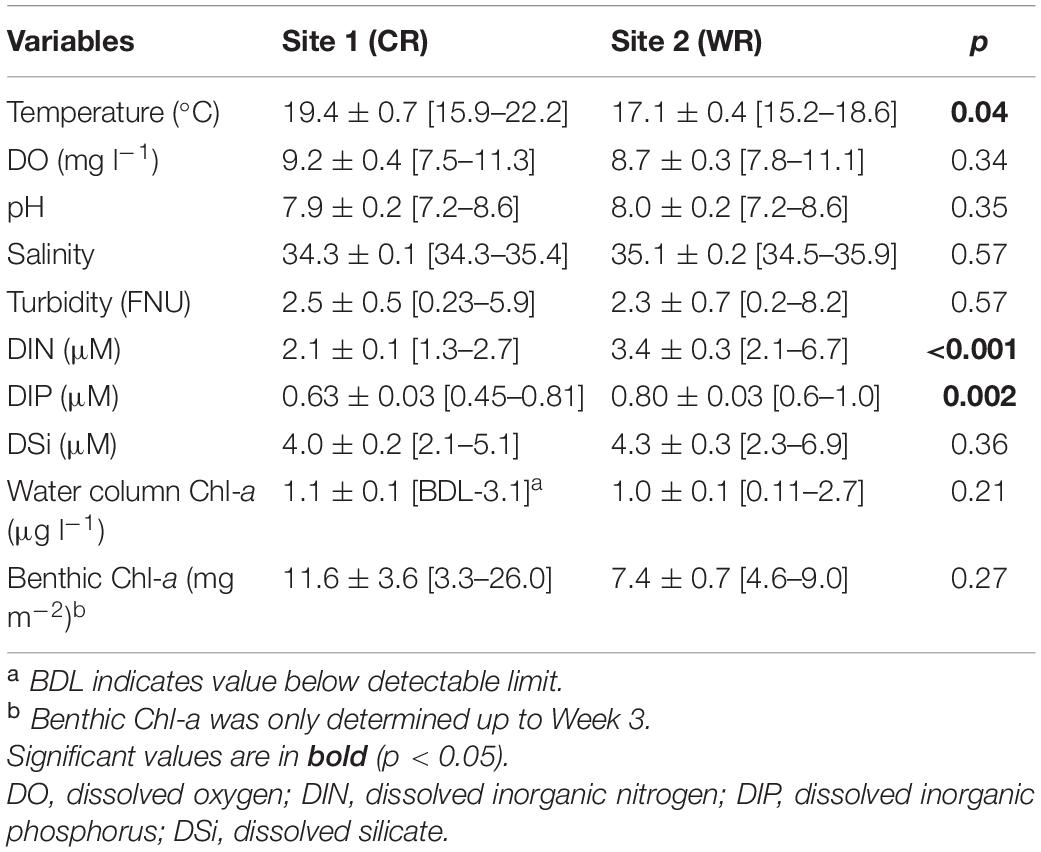
Table 1. Summary statistics of the physicochemical, nutrients and Chl-a data presented as mean ± SD and range [min-max value] recorded during the study at Cape Recife (CR) and Willows Resort (WR).
Spatio-Temporal Patterns of Diatom Assemblages and Drivers
Site-specific data revealed a total of 102 species (38 genera) and 75 species (34 genera) observed at Site 1 (Cape Recife) and Site 2 (Willows Resort), respectively. The dominant species observed, based on abundance and irrespective of site and sampling occasion, included Navicula sp1, Navicula sp3, Actinocyclus subtilis, Licmophora paradoxa, and Nitzschia ventricosa (Figure 2). Cocconeis (13 species) and Nitzschia (9 species) were observed as the dominant genera in this study. While certain species were shared between the two sites (e.g., Grammatophora undulata, Haslea nautica, and Navicula cf. directa), a number of species were observed at either one site or the other (e.g., Site 1 – Diploneis cabro, Mastogloia fimbriata, and Plagiotropis lepidoptera; Site 2 – Lampriscus shadboltianum and Trachyneis velata; Supplementary Figure S2). The overall diatom composition was composed of 134 species (and varieties) from 44 genera (sites data combined). From this, 66 species contributed ≥1% in relative abundance with only 24 species attaining ≥5% abundance in at least one sample (Figure 2 and Supplementary Figure S2). Forty-eight percent (48%) of the diatom composition was composed of rarely observed species such as Cocconeis testudo, Diplomenora cocconeiformis var., Donkia sp., Psammodictyon panduriforme, Lyrella lyra, Navicula perrhombus, and Paralia sulcata contributing <1% in abundance (Supplementary Table S2).
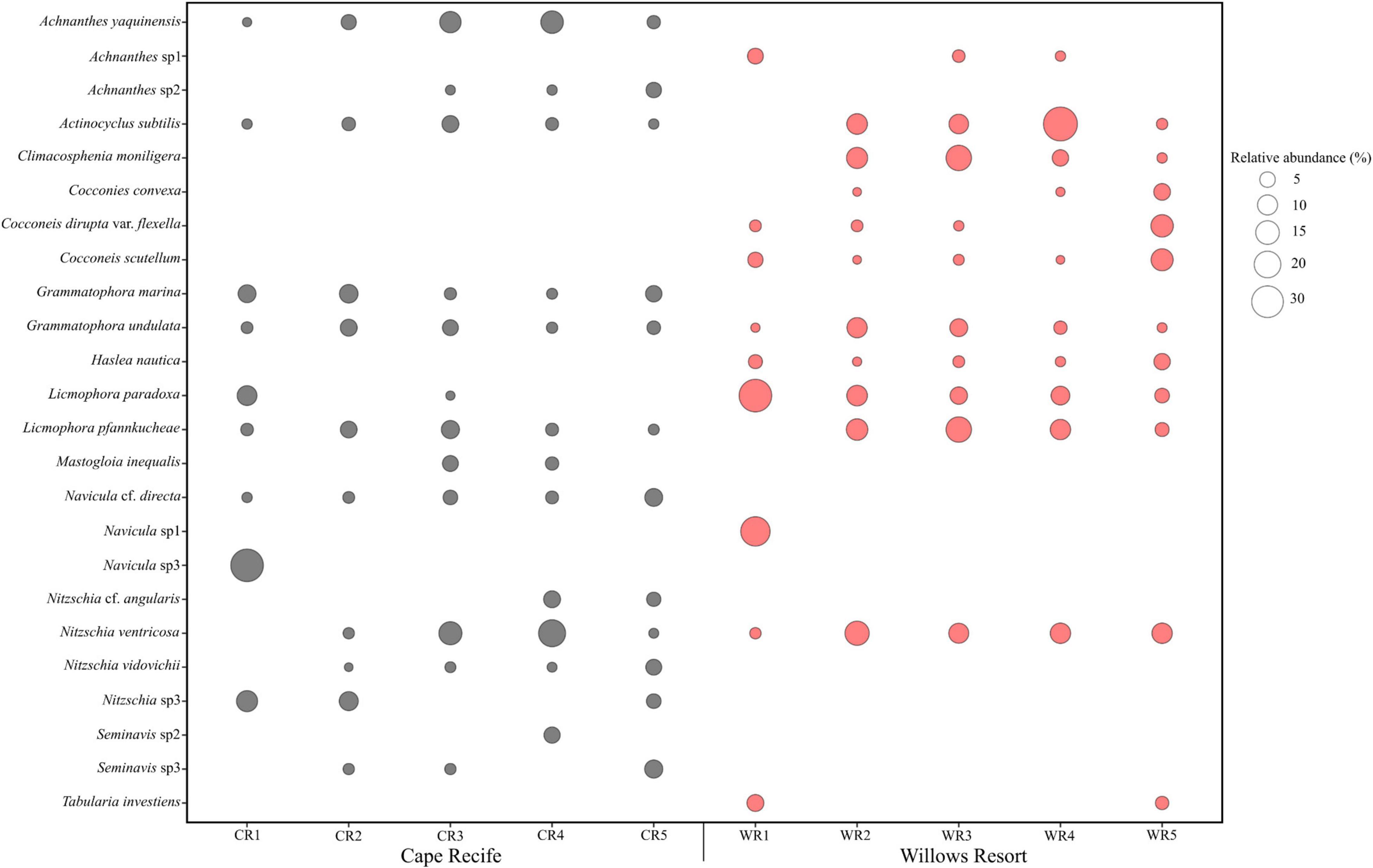
Figure 2. Diatom taxa observed with ≥5% relative abundance in at least one sample during the study period.
The multivariate homogeneity of group dispersion (variances) analyses revealed no significant differences between the two sites (p > 0.05; Supplementary Figure S3). The nMDS ordination based on diatom composition illustrated a distinct separation of the sampling sites (and weekly samples), the ordination was supported by a low stress value of 0.08 (indicating a good representation; Figure 3) and a high correlation between the observed dissimilarity and the ordination distance (non-metric fit, R2 = 0.99). The compositional dissimilarity observed between the two sites was revealed as significant (PERMANOVA, R2 = 0.24, p < 0.01). Temporal compositional differences were strikingly evident when considering the diatom assemblages from Week 1 and Week 5, at both sites (Figure 3). Further, large fluctuations in species presence and abundances, indicated by the weekly changes in the number of species observed, during the experimental period (Supplementary Figures S2, S4).
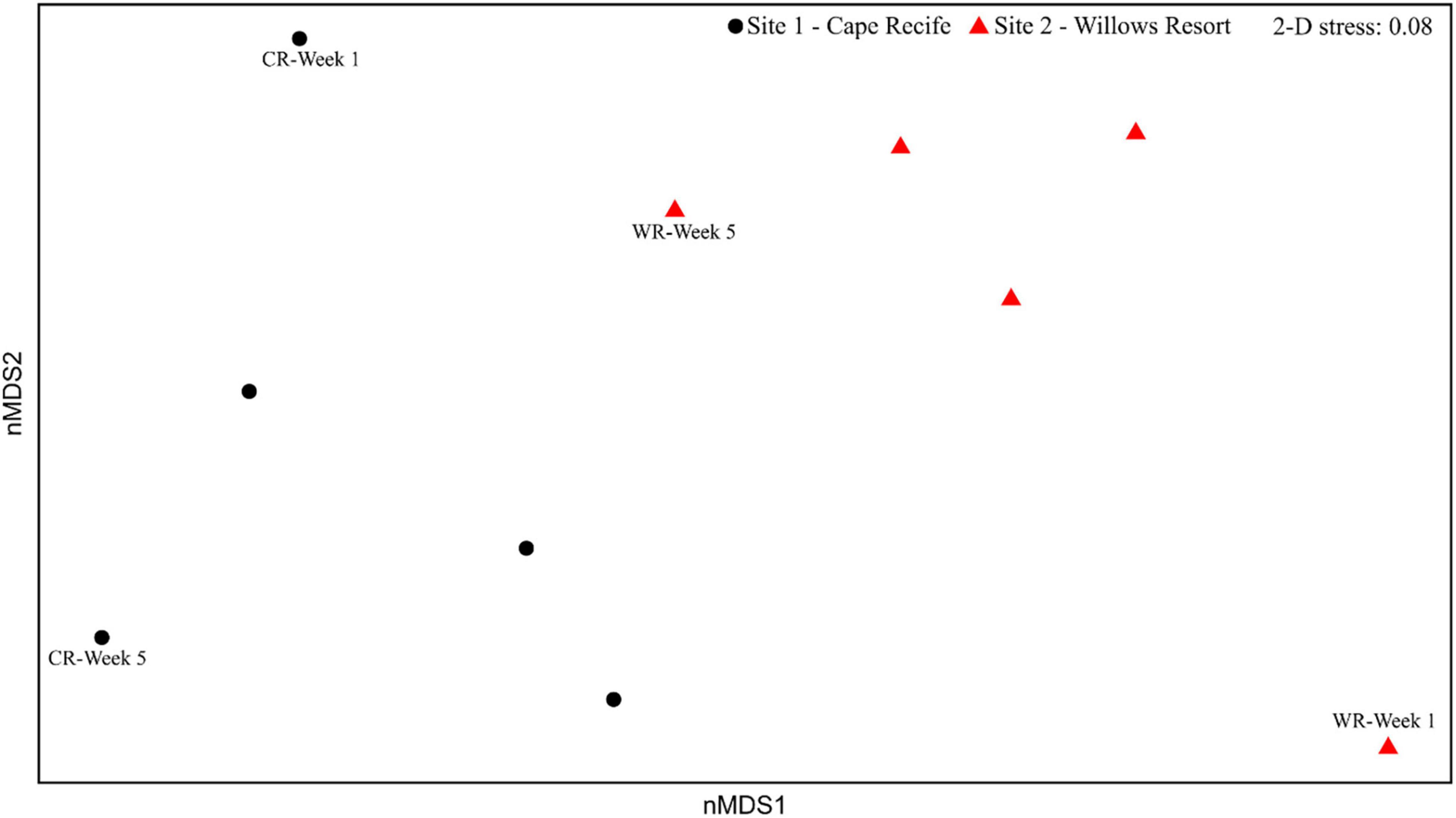
Figure 3. Non-metric multidimensional scaling ordination of diatom assemblages at Cape Recife (black circles) and Willows Resort (red triangles).
The species richness (S) and Shannon diversity (H′) showed a significant variation between the two sites, whereas Simpson dominance (D) and Pielou’s evenness (J′) remained similar during this study (Table 2). Overall, the diatom community descriptors were temporally (between weeks) variable during the experimental period, with a discernible trend of higher values recorded at the Cape Recife site. Noticeably, at both sites, the minimum and maximum values for each of the community descriptors were observed at Week 1 and Week 5, respectively (Supplementary Figure S4).
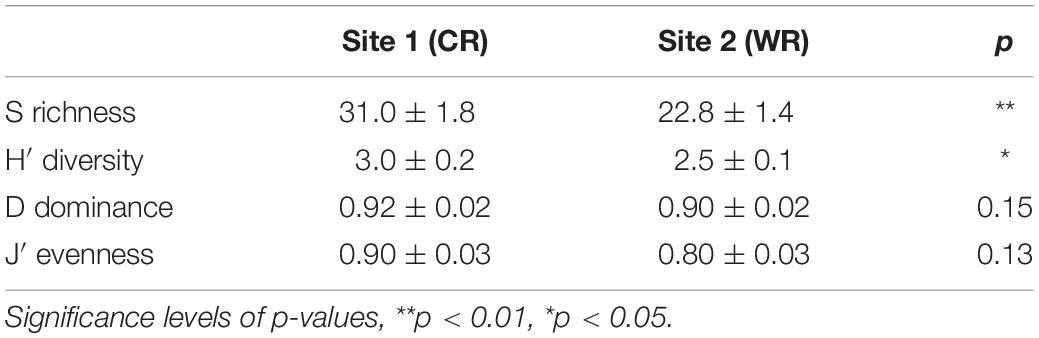
Table 2. Average (± SE) species richness (S), Shannon diversity (H′), Simpson dominance (D) and Pielou’s evenness (J) between Cape Recife (CR) and Willows Resort (WR).
The predictor variables in the most-parsimonious models demonstrated a varied influence on each of the community descriptors (diversity H′, dominance D and evenness J′). On the contrary, none of the predictor variables showed any significant effect on species richness (p > 0.05; Table 3), indicating possible influence of unmeasured variables (e.g., species interactions). Overall, temperature showed significantly positive relationships with H′, D (p < 0.01) and J′ (p < 0.05) while dissolved oxygen demonstrated significant negative relationships with H′ and D (Table 3). Nutrients in the form of DIN and DSi were significant (p < 0.05) predictors for H′, with DIN also showing a positive significant relationship with D (p < 0.01; Table 3). Turbid conditions were negatively associated with the descriptors with a weak significant relationship detected between turbidity and D (p < 0.05; Table 3).
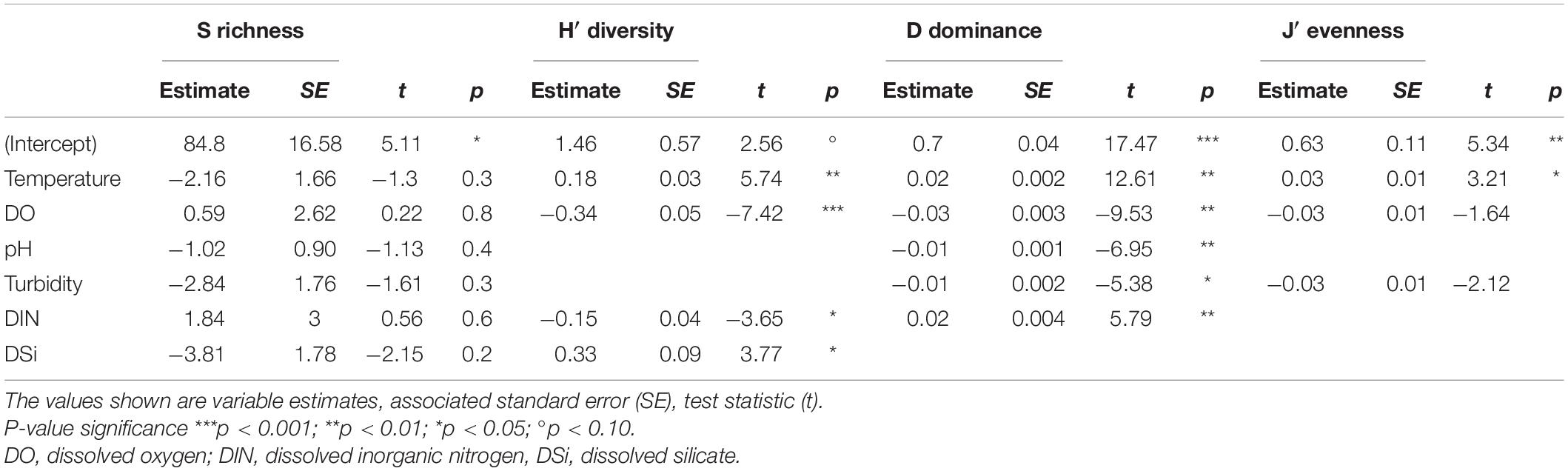
Table 3. Summary of best-fit linear mixed-effects models (LMM) fitted separately by restricted maximum likelihood (REML) estimation to evaluate the effect of the predictor variables on each community descriptors (S, H′, D, and J′).
The observed diatom community abundances, were best explained by the site, sampling occasion and their interaction, with a proportionate contribution to deviance explained in the overall model (25–49% D; GLM, p < 0.05; Table 4). Based on the univariate models nested within the overall model, site location was an important predictor of Cocconeis (46% D) and Haslea (72% D) occurrence and abundances (GLM, p < 0.05), explaining high deviance, including that for Climacosphenia (39% D) and Seminavis (47% D; Table 4). Unexpectedly, sampling occasion was not a significant predictor in the univariate models, however, it was important for the rare observations of Mastogloia and Tabularia genera (Figure 2) based on deviance explained (74% and 75% D, respectively; Table 4). Overall, the interaction between site and sampling occasion (Site: Sample) was significant for a number of taxa abundances across the sites (40–70% D; GLM, p < 0.05; Table 4). Some of the diverse genera, represented by up to three species (Figure 2), provided a high contribution to the overall Site: Sample interaction deviance, e.g., Achnanthes (27% D), Navicula (23% D), and Nitzschia (14% D; Table 4).
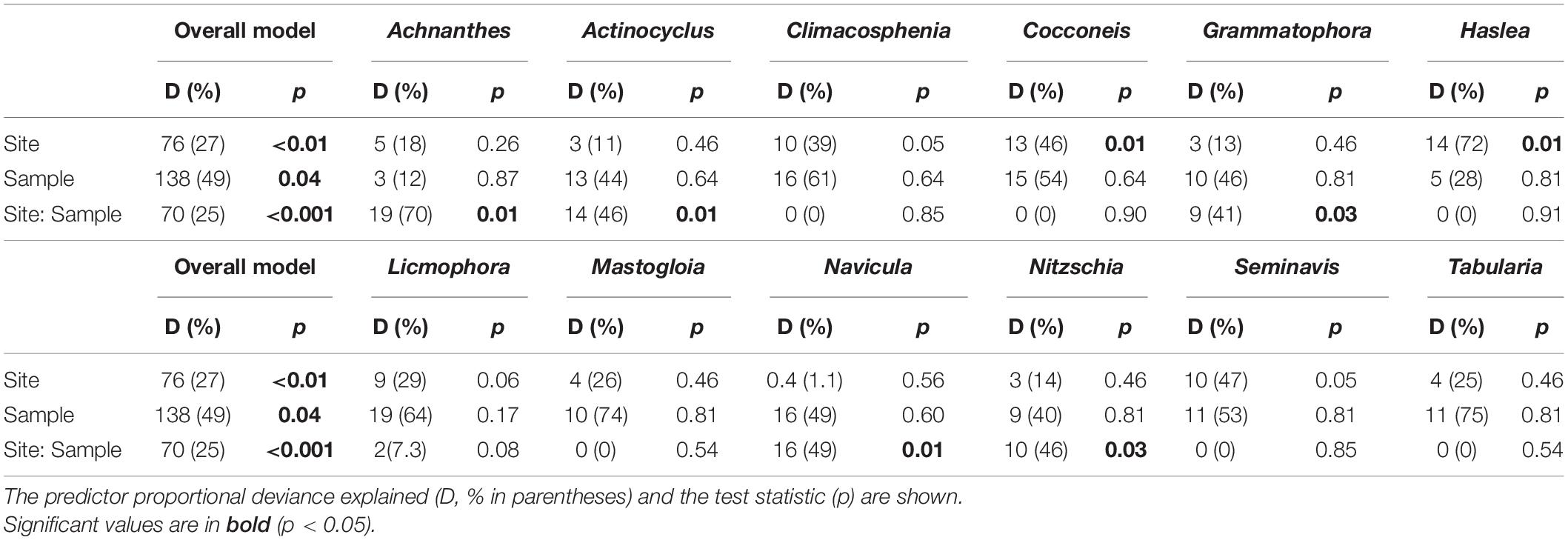
Table 4. Multivariate generalized linear model (GLMmv) result showing the categorical predictors’ (site, sample ‘sampling occasion’ and their interaction) effects on the diatom taxa abundance (grouped by genus).
Considering the continuous variables, DSi was the only significant predictor of the observed multivariate diatom community (25% D; GLM, p < 0.05) in the overall model (Table 5). Despite this, the deviance explained by temperature and turbidity suggested that the warm and turbid conditions observed in this study were important for some taxa, e.g., Licmophora and Nitzschia (temperature: 37% D, 28% D, respectively), Achnanthes and Mastogloia (turbidity: 62% D, 74% D, respectively; Table 5). Dissolved oxygen (DO: ∼8 mg l–1) and pH (∼7) revealed a low deviance contribution in the overall model (<10% D), however, showed that oxygenated and alkaline conditions have considerable varying relation to some of the observed genera such as Cocconeis and Grammatophora (∼48% D; Table 5). In terms of nutrients, DIN and DSi made substantial contributions to the deviance explained in the overall model (24–30% D; Table 5). These nutrients were associated with the temporal variation of some taxa at either one site or both (Figure 2), reflected in the high deviance accounted for, e.g., DIN: >50% D for Climacosphenia, Tabularia, and DSi: >50% D for Actinocyclus and Nitzschia (Table 5).
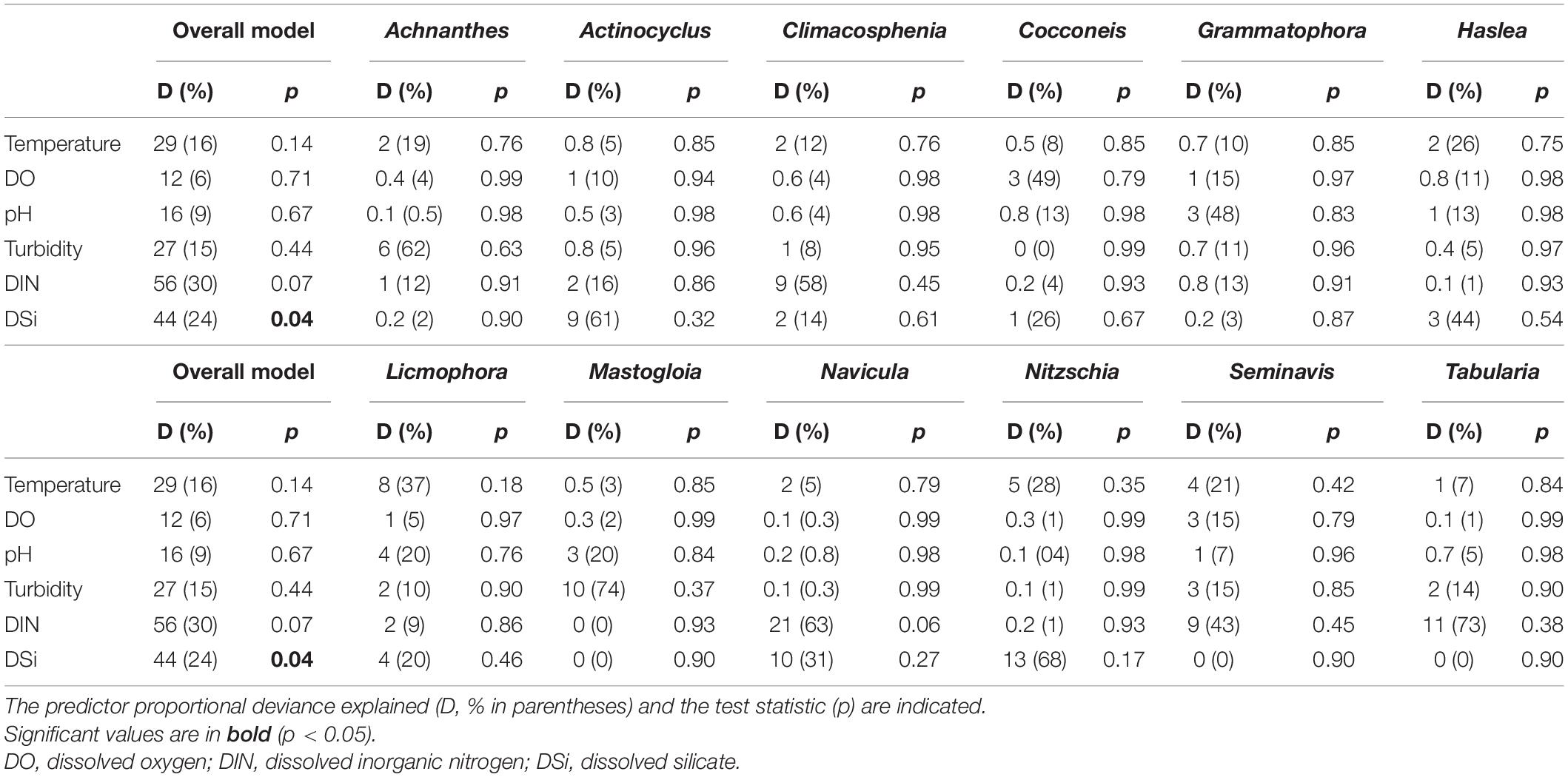
Table 5. Multivariate generalized linear model (GLMmv) results showing the continuous predictors’ effects on the diatom taxa abundance (grouped by genus).
Discussion
Situated at the land-sea interface, the coastal environment is vulnerable to anthropogenic disturbances such as nutrient enrichment. Methods to ascertain the response of biological entities to such disturbances are vital to understand and manage the resultant environmental impacts. It is standard practice to select indicator species to observe changes to the ecosystem. In freshwater environments, diatoms are often ideal bioindicators. However, there is limited knowledge on benthic diatoms as potential bioindicators in coastal ecosystems (Desrosiers et al., 2013). The main objective of this study was to investigate the diatom colonisation dynamics in nearshore waters, to obtain baseline information on compositional variations, drivers thereof, and further assess the applicability of marine benthic diatoms on artificial substrata for routine monitoring in South Africa. Considering that, the coastal waters of Algoa Bay are prone to land-based nutrient loading (Lemley et al., 2019), observing a combination of biological and physico-chemistry variables allows for a comprehensive understanding of the ecological state of the environment.
Field Observations
Biofilm development was evident at both sites from day 3 following the commencement of the experimental period. However, after 7 days (first sampling), site-specific colonisation differences became apparent. At Site 1 (Cape Recife), macroalgal spores (green algal spores) colonised the substrate during Week 2, which was followed by a rapid increase in brown algal spores on one side of the panel and a combination of both green and brown spores on the other side. The presence of the green and brown macroalgae spores dramatically increased thereafter, which resulted in high macroalgal biomass on the panels by Week 3. Macroalgal (seaweed) spores are released into the water column based on their physiological response to local environmental conditions that include temperature and photo-period (Stengenga et al., 1997). Thus, the presence of macroalgal spores is likely seasonal or all-year round depending on the presence of macroalgal species in the study location. Similar to our study, Ryabushko et al. (2021) also recorded “seedlings of macrophytes” on artificial material in an experimental study along the coastal waters of the Black Sea. At Site 2 (Willows Resort), the biofilm development was interrupted by the presence and grazing activity of the marine intertidal gastropod – Oxystele sinensis. The signs of grazing were evident from Week 2, with the panels grazed at random (i.e., some of panels were grazed ∼80% on one side). Some of the ungrazed panels showed similar biofilm development patterns of increased green and brown macroalgal spores as was observed at Site 1. The grazing activity also lead to the colonisation of the panels by patches of coralline algal crusts, seen at the end of the experiment (Week 5). Surprisingly, despite the grazing pressure by O. sinensis, a considerable biomass and diversity of diatom species was still present on the grazed panels. Car et al. (2020) also observed similar consumption of the periphyton by snails on artificial substrates. Overall, the grazing pressure at Site 2 was most likely the cause of the lower number of species observed at Site 2 compared to Site 1.
Diatom Spatio-Temporal Patterns
Although the observed taxonomic composition data was highly variable, distinct spatio-temporal assemblage patterns were evident, thus supporting the hypothesis that the diatom assemblages would differ between weeks (biofilm stages). However, the results did not support to our postulation that the assemblages would be similar between the two study sites. Overall a higher number of diatom taxa was observed at Site 1 (Cape Recife) compared to Site 2 (Willows Resort). While certain taxa were shared, composition analyses revealed distinct taxonomic dissimilarity between the two sites (Figure 3). Besides the expected occurrence of benthic diatoms on the natural substratum at these sites, the shared taxa could also be attributed to dispersal within the study area via shared oceanographic processes (i.e., waves and currents). In line with our observed results, a similar study by Zidarova et al. (2020) along the coastal island waters of South Bay (Antarctica), reported a significant variation between two experimental sites based on diatom composition. Generally, there is high heterogeneity in benthic epilithic diatom communities from coastal habitats due to the environmental variability, geography and complexity of substrate surface microtexture (Cotiyane-Pondo et al., 2020). Such heterogeneity is also the result of environmental gradients such as changes in salinity and coastal climate (Virta et al., 2020). Information on spatial beta diversity is important for our understanding of ecosystem processes such as biogeochemical cycles and trophic interactions. Diverse species assemblages, including that of benthic diatom communities (Virta et al., 2021), impart various ecological roles in an ecosystem, highlighting the crucial role of biodiversity in providing multiple ecosystem functions (see Mori et al., 2018). Therefore, the spatial taxonomic variation observed in this study highlights the diatom biodiversity present at a local scale. The spatial differences in diatom composition are noteworthy and support the results from Cotiyane-Pondo et al. (2020) that showed high spatial heterogeneity along the coast of South Africa. The most commonly observed diatom genera identified (i.e., Cocconeis, Diploneis, Grammatophora, Licmophora, Navicula, Nitzschia, Psammodictyon, and Seminavis) were represented by ≥5 species each. Further, the results also revealed a large number of rare taxa (Supplementary Table S2), that contributed >40% to the overall taxa composition observed during this study. The rarely observed diatoms included taxa such as Cocconeis testudo, Rhaphoneis mirabunda, and Campylodiscus scalaris, first described by Giffen (1966, 1971) during the early taxonomic studies along the South African shore. However, the focus of this study was to understand the short-term temporal changes (succession) in diatom community structure.
Diverse diatom assemblages were observed throughout the experimental period with the weekly compositional variations yielding high diatom taxa abundances and overall, a high number of species. This highlights the temporal community dynamics not captured in the previous spatially focused investigations in this region (Cotiyane-Pondo et al., 2020, 2021). The considerable weekly fluctuations in diatom abundances were characterised by diverse genera. Newly submerged substrates are known to be quickly colonised by bacteria, followed by pioneer diatom species (Pennesi and Danovaro, 2017; Car et al., 2020), depending on the species growth form and their ability to withstand factors such as competition and grazing once attached (Round et al., 1990; Fricke et al., 2016). The colonisation of substrate and the subsequent biofilm development was driven by the presence of the microphytobenthos at the site of interest. The initial colonisation by bacteria enhances the attachment of diatoms on the substrate (Totti et al., 2007; Car et al., 2020). Benthic diatom species attachment is also facilitated by the nature of the substrate (Desrosiers et al., 2014) and the presence of suspended benthic species in the water column. In this study, the pioneer species (initial colonisers) at Week 1 were characterised by numerous genera dominated particularly by Navicula species, followed by Licmophora and Nitzschia, at both sites. The settlement of new taxa during the experimental period (Supplementary Figure S2) further contributed to the diatom community composition and diversity. Colonisation of the substrata by new species can be expected to differ depending on local biofilm dynamics and the influence of the surrounding environment (Desrosiers et al., 2014; Zidarova et al., 2020). The weekly variation in diatom composition was reflected in the community descriptors (diversity metrics), with an initially increasing, then fluctuating trend during the study period at both sites (Supplementary Figure S4). The community descriptors declined during Week 4, which was interpreted as a signal of the first stage towards biofilm maturity. However, both sites showed elevated community descriptor values during Week 5. This variation can be explained by the biofilm dynamics observed at each study site. We postulate that the increased presence of macroalgal spores at Site 1, during Week 5, allowed for the attachment of new taxa (late colonisers), particularly opportunistic taxa found in low abundances (e.g., Mastogloia fimbriata, Lyrella lyra, Psammodictyon panduriforme). Similarly, Fricke et al. (2016) noted that opportunistic species alternating in presence and abundances made an important contribution to the variable species richness and diversity found during their experimental study. Herbivory has a large impact on benthic microalgae, leading to altered abundance, diversity and community structure (Hillebrand et al., 2000; Armitage et al., 2009; Alberti et al., 2017). The grazing activity at Site 2 (this study), facilitated the increase in the presence and diversity of the genera Cocconeis (e.g., C. scutellum, C. capensis, C. convexa, C. cf. pellucida). This highlights the opportunistic nature of some taxa that colonised the panels after the grazing occurred and most importantly, the resilience of some taxa to grazing, which is attributed to their growth forms (e.g., adnate growth). The biofilm dynamics described ultimately lead to the observed fluctuations in the community descriptor values. Despite these site-specific differences, some of the observed taxa were present throughout the experimental period, either at only one site or at both. These included Actinocyclus subtilis, Climacosphenia moniligera, Grammatophora undulata, Haslea nautica, Licmophora pfannkucheae, Navicula cf. directa, and Nitzschia ventricosa.
Drivers of Variation and Implications for Monitoring
Diatom biofilm development occurs in phases to reach a mature diatom community, and this process is facilitated by numerous factors (Desrosiers et al., 2014). Despite the vital role of benthic diatoms in many ecosystem processes, the drivers of community composition, diversity etc., vary and are highly location/region dependent. Studies also investigate different biotic or abiotic factors thereby limiting direct comparison between studies. Our interrogation of the potential drivers identified that some of the local environmental variables (e.g., temperature and nutrients) were significantly correlated to the diatom assemblages observed, particularly species diversity, dominance and evenness (community descriptors). Interestingly, these variables did not have a significant effect on species richness (Table 3). Desrosiers et al. (2014) found that species richness was less informative compared to other diversity indices when studying diatom community development. In this study, sampling site (location) and sampling occasion (weekly sampling) were good predictors of the diatom taxa abundances (Table 4), indicating that the influence of the measured environmental variables on the community descriptors is superseded by the significant effect natural succession at the study location. The less pronounced effects of the environmental variables on the diatom abundances (Table 5) further supported this. However, temperature and nutrients (in particular DIN), which varied between the sites, explained some of the variation in the taxa abundances. The observed influence of these variables in this study is consistent with previous findings along the nearshore waters of a nearby coastal island (Cotiyane-Pondo et al., 2021) and to a larger extent, around the South African coastline (Cotiyane-Pondo et al., 2020). However, being snapshot studies, the limitation of the previous research is that it did not cater for temporal variability of the diatom assemblages, hence the importance of the results presented in this study. The variability induced by the physical environment (i.e., structure of the natural substratum) of each study site and the associated oceanographic conditions (i.e., wave action) dictate the fluctuations in the biogeochemical environment. In line with the recommendations by Desrosiers et al. (2013, 2014), the present study highlighted benthic diatom species abundances and assemblage structure dynamics in relation to the physico-chemistry and site characteristics under natural conditions. Further, our results also share similarity with Pennesi and Danovaro (2017), who supported the use of artificial substrata to study the influence of site-specific environmental conditions on the microphytobenthos. The use of the artificial substrata in this study proved vital in the investigation of the diatom succession, which is not easy to replicate using natural substrata. Mori et al. (2018) noted that natural systems, including intertidal natural rocky substrata, can be found in different developmental stages. The highly variable South African coastline is an ideal environment to investigate the response of nearshore benthic diatoms to environmental change. Our understanding of the local diatom assemblages’ response to potential drivers is central to the subsequent implementation of the experimental method used in this study for routine monitoring, and effective assessment of anthropogenic inputs on biological communities along the coastline. Interestingly, despite the presence of a WWTP in the vicinity of Site 1, no eutrophication of the nearshore environment was observed during the current study. Nutrient concentrations remained low (i.e., below 10 μM) as did the resultant chlorophyll-a biomass (average: <2 μg l–1; see Table 1 and Supplementary Table S1). Low concentrations in the Cape Recife site might be due to nutrient uptake and assimilation by the dense resident macroalgae beds [Plocamium corallorhiza (Turner) J.D. Hooker & Harvey], and the influence of oceanographic conditions such as current and wind-induced water column mixing. Conversely, nutrient concentrations at Site 2 were higher and this is attributed to groundwater influence along that section of the coastline (Perissinotto et al., 2014). Since there was no evident eutrophication in close proximity to our study sites, the recorded nutrient concentrations provide us with reference conditions, upon which the occurrence of elevated nutrient loading and subsequent change in dominance of certain taxa (Hillebrand et al., 2000) can be compared. This is important, because as noted by Lemley et al. (2019), changes in nutrient inputs in the coastal waters of Algoa Bay induce changes in biological communities. We acknowledge that, globally, the microphytobenthic response (i.e., sensitivity) to nutrients has been widely documented (e.g., Hillebrand and Sommer, 1997; Armitage et al., 2009; Car et al., 2020; Clark et al., 2020) and that species sensitivity to nutrient enrichment can vary within a genus (Hillebrand and Sommer, 1997) and within assemblages (Kafouris et al., 2019).
Interlinked with the response of benthic diatoms to changing conditions is species growth forms. Hillebrand et al. (2000) documented that a “trade-off” between access to nutrients and grazing resistance is associated with growth form. Adnate diatoms (e.g., Cocconeis spp.) being highly resistant to grazing compared to erect forms (e.g., Licmophora spp.), and motile diatoms (e.g., Navicula spp.) (Totti et al., 2007). It is likely that the diatom community dynamics based on growth form will produce a different community composition compared to the taxonomic composition, as shown in Totti et al. (2007). We acknowledge that investigating compositional shifts based on diatom functional groups (life forms, ecological guilds) will further offer progressive insights towards diatom-based bioassessments (Riato et al., 2017). Considering the cosmopolitan nature of many diatom taxa, this study and the addition of functional-based information might assist future studies in the selection of indicator taxa. Identification of indicator taxa or pollution resistant taxa in this region is inherently dependent on being able to distinguish between diatom assemblage variation influenced by natural conditions, to that driven by anthropogenic-induced change (e.g., nutrient pollution). In that regard, it is plausible that a follow up study in a heavily impacted site or along a pollution gradient will reveal diatom taxonomic differences and taxa abundances, further revealing pollution tolerant taxa, that will aid in the selection of indicator taxa. Kafouris et al. (2019) identified Cocconeis and Tryblionella genera as good indicators of change along a eutrophication gradient. However, these taxa might not be directly applicable/useful elsewhere due to region-specific climate differences and environmental conditions, including the natural nutrient loading. Thus, further experimental studies are required in South Africa to assess diatom species-specific ecological preferences (environmental tolerances) and sensitivity to nutrient concentrations within this region. This research, conducted across the region will in all likelihood identify species with a specific biogeographic affinity or distribution (i.e., only found in one coastal bioregion, Cotiyane-Pondo et al., 2020), which may hinder the use of specific taxa as the only indicators of change around the coastline.
Coastal ecosystems are highly variable environments, especially around the coast of South Africa, where wind induced upwelling, coastal trapped waves, meanders in the warm oligotrophic Agulhas Current, etc. all contribute to the variable biological response. Recently, Pattrick et al. (2021) showed the role of coastal and nearshore hydrographic variability on larval fish dynamics along the south coast of South Africa. Coastal hydrodynamics in the study area generate and drive the environmental heterogeneity, which leads to rapid fluctuations in species, populations and ultimately, communities, coupled with biological interactions, drives microbial community composition and distribution along nearshore environments. Concerning benthic diatoms, the current study has shown that environmental variability results in the rapid change in diatom species assemblages across space and time. Nonetheless, due to differences in individual species response dynamics to environmental change, understanding and predicting population response or sensitivity to fluctuating environments remains a challenge (Benedetti-Cecchi, 2009). To fully comprehend the influence of environmental heterogeneity of coastal environments (e.g., rocky shores) on organisms and populations, aspects such as organisms’ evolutionary and adaptation traits should be considered (Bitter et al., 2021). This would to allow for better predictability of population or community response to fluctuating environments and climate change (see Bernhardt et al., 2020; Bitter et al., 2021).
Given the dynamic nature of inter-and subtidal ecosystems, artificial substrata can be effectively used as sampling systems in bioassessment surveys around the coastline, contributing to existing national coastal long-term ecological research (LTER) programmes. Understanding microphytobenthos dynamics using traditional taxonomy is a key step in data-limited regions, prior to the implementation of diatom-based indices and advanced methodologies such as DNA metabarcoding (Kelly et al., 2020a) and isotope analysis (Dalu et al., 2020) used in freshwater systems. Although monitoring initiatives are arguably region dependent, our findings make available baseline information on short-temporal benthic diatom colonisation dynamics and offer guidance for future studies pertaining to the use of benthic diatoms as indicators of change in marine monitoring along the data-limited coastal waters of Southern Africa.
Conclusion
The results of this study provide the first observations of marine benthic diatoms associated with artificial substrata, and present a significant contribution to our understanding of microphytobenthos dynamics along the South African coastline. The continuous presence of macroalgal spores at Cape Recife, contrasted by the continuous grazing at Willows Resort, yielded the observed differences in biofilm dynamics in diatom abundance, species diversity, and the dominance of certain taxa. Given the high dissimilarities observed in this study, we propose that for both short and long-term monitoring, an array of equally distanced sites should be considered to fully incorporate a broad range of site conditions, along with monitoring at sites of known eutrophication. In line with monitoring of future changes, this study also presented valuable data pertaining to diatom biodiversity (i.e., taxonomic composition), and the new knowledge on the rate of compositional shifts is crucial when considering the monitoring of local and regional biodiversity trends (Hillebrand et al., 2018). Future research on the bioindication properties of benthic diatoms along the coastal environment should be complemented with other informative approaches such functional groups to better understand biofilm dynamics, and molecular techniques to extend our knowledge on the marine benthic diatoms of Southern Africa.
Data Availability Statement
The original contributions presented in the study are included in the article/Supplementary Material, further inquiries can be directed to the corresponding author.
Author Contributions
PC-P contributed to the methodology, fieldwork, sample and data analyses, and writing of the original manuscript. TB contributed to the edit and review of manuscript. Both authors contributed to the article and approved the submitted version.
Funding
PC-P was the recipient of the Professional Development Programme (PDP) bursary of the National Research Foundation (NRF – Grant UID 100542). We acknowledge the research support provided by the Shallow Marine and Coastal Research Infrastructure (SMCRI) platforms of the Department of Science and Innovation (DSI) hosted by the NRF-SAEON Elwandle Node. This research was partially funded by the South African Research Chairs Initiative through the DSI/NRF, by a Community of Practice grant in Marine Spatial Planning (UID 87583 and 110612). Any opinions, findings, or recommendations expressed in this publication are those of the authors and not necessarily the funding bodies.
Conflict of Interest
The authors declare that the research was conducted in the absence of any commercial or financial relationships that could be construed as a potential conflict of interest.
Publisher’s Note
All claims expressed in this article are solely those of the authors and do not necessarily represent those of their affiliated organizations, or those of the publisher, the editors and the reviewers. Any product that may be evaluated in this article, or claim that may be made by its manufacturer, is not guaranteed or endorsed by the publisher.
Acknowledgments
We are grateful for the assistance from W. Kuntz, M. Pollard, T. Mkhize, I. Malick, and P. Rukuni during this study. We thank The Willows Resort for access to the study site, and Jan Jorna for assistance with the development of the cages used in this study. P. Da̧bek and Prof. A. Witkowski from University of Szczecin are thanked for assisting with the verification of diatom identifications.
Supplementary Material
The Supplementary Material for this article can be found online at: https://www.frontiersin.org/articles/10.3389/fevo.2021.767960/full#supplementary-material
References
Alberti, J., Cebrain, J., Alvarez, F., Escapa, M., Esquius, K. S., Fanjul, E., et al. (2017). Nutrient and herbivore alterations cause uncoupled changes in producer diversity, biomass and ecosystem function, but not in overall multifunctionality. Sci. Rep. 7:2639. doi: 10.1038/s41598-017-02764-3
Al-Handal, A. Y., Compére, P., and Riaux-Gobin, C. (2016). Marine benthic diatoms in the coral reefs of Reunion and Rodrigues Island, West Indian Ocean. Micronesica 3, 1–77.
Al-Handal, A. Y., and Wulff, A. (2008a). Marine benthic diatoms from Potter Cove, King George Island, Antarctica. Bot. Mar. 51, 51–68. doi: 10.1515/BOT.2008.007
Al-Handal, A. Y., and Wulff, A. (2008b). Marine epiphytic diatoms from the shallow sublittoral zone in Potter Cove, King George Island, Antarctica. Bot. Mar. 51, 411–435. doi: 10.1515/BOT.2008.053
Álvarez-Blanco, I., and Blanco, S. (2014). Benthic diatoms from Mediterranean coasts. Bibliotheca Diatomologica 60, 1–409.
Armitage, A. R., Gonzales, V. L., and Fong, P. (2009). Decoupling of nutrient and grazer impacts on a benthic diatom assemblage. Estuar. Coast. Shelf Sci. 84, 375–382. doi: 10.1016/j.ecss.2009.07.001
Bate, G. C., and Heelas, B. V. (1975). Studies on the nitrate nutrition of two indigenous Rhodesian grasses. J. Appl. Ecol. 12, 941–952.
Bates, D., Maechler, M., Bolker, B., and Walker, S. (2015). Fitting linear mixed-effects models using lme4. J. Stat. Softw. 67, 1–48. doi: 10.18637/jss.v067.i01
Beckley, L. (1983). Sea-surface temperature variability around Cape Recife, South Africa. S. Afr. J. Mar. Sci. 79, 436–438.
Beiras, R. (2016). Assessing ecological status of transitional and coastal waters; current difficulties and alternative approaches. Front. Mar. Sci. 3:88. doi: 10.3389/fmars.2016.00088
Benedetti-Cecchi, L. (2009). “Environmental variability: analysis and Ecological Implications,” in Marine Hard Bottom Communities. Ecological Studies 206, ed. M. Wahl (Berlin: Springer-Verlag), 127–141.
Bernhardt, J. R., O’Connor, M. I., Sunday, J. M., and Gonzalez, A. (2020). Life in fluctuating environments. Philos. Trans. R. Soc. B 375:20190454. doi: 10.1098/rstb.2019.0454
Bitter, M. C., Wong, J. M., Dam, H. G., Donelan, S. C., Kenkel, C. D., Komoroske, L. M., et al. (2021). Fluctuating selection and global change: a synthesis and review on disentangling the roles of climate amplitude, predictability and novelty. Proc. R. Soc. B 288:20210727. doi: 10.1098/rspb.2021.0727
Borja, A., and Dauer, D. M. (2008). Assessing the environmental quality status in estuarine and coastal systems: comparing methodologies and indices. Ecol. Indic. 8, 331–337. doi: 10.1016/j.ecolind.2007.05.004
Borja, A., Elliott, M., Anderson, J. H., Berg, T., Carstensen, J., Halpern, B. S., et al. (2016). Overview of integrative assessment of marine systems: the Ecosystem approach in practice. Front. Mar. Sci. 3:20. doi: 10.3389/fmars.2016.00020
Borja, A., Ranasinghe, A., and Weisberg, S. B. (2009). Assessing ecological integrity in marine waters, using multiple indices and ecosystem components: challenges for the future. Mar. Pollut. Bull. 59, 1–4. doi: 10.1016/j.marpolbul.2008.11.006
Car, A., Hafner, D., Ljubimir, S., Radić, I. D., Bobanović-Ćolić, S., and Jasprica, N. (2020). Colonization of bacteria and diatoms on an artificial substrate in a marine lake (eastern Adriatic Sea, NE Mediterranean). Acta Bot. Croat. 79, 212–227. doi: 10.37427/botcro-2020-028
Clark, D. E., Pilditch, C. A., Pearman, J. K., Ellis, J. I., and Zaiko, A. (2020). Environmental DNA metabarcoding reveals estuarine benthic community response to nutrient enrichment – Evidence from an in situ experiment. Environ. Pollut. 267:115472. doi: 10.1016/j.envpol.2020.115472
Cocquempot, L., Delacourt, C., Paillet, J., Riou, P., Aucan, J., and Castelle, B. (2019). Coastal ocean and nearshore observation: a French case study. Front. Mar. Sci. 6:324. doi: 10.3389/fmars.2019.00324
Cotiyane-Pondo, P., Bornman, T. G., and Da̧bek, P. (2021). Insight into nearshore diatom assemblages from an island ecosystem: composition, spatial variation, and benthic contribution to the pelagic community (Bird Island, South Africa). Reg. Stud. Mar. Sci. 44:101762. doi: 10.1016/j.rsma.2021.101762
Cotiyane-Pondo, P., Bornman, T. G., Da̧bek, P., Witkowski, A., and Smit, A. J. (2020). Austral winter marine epilithic diatoms: community composition and distribution on rocky substrate around the coast of South Africa. Estuar. Coast. Shelf Sci. 242:106837. doi: 10.1016/j.ecss.2020.106837
Dalu, T., Cuthbert, R. N., Taylor, J. C., Magoro, M. L., Weyl, O. L. F., Froneman, W. P., et al. (2020). Benthic diatom-based indices and isotopic biomonitoring of nitrogen pollution in a warm temperate Austral river system. Sci. Total. Environ. 748:142452. doi: 10.1016/j.scitotenv.2020.142452
Dalu, T., and Froneman, P. W. (2016). Diatom-based water quality monitoring in southern Africa: challenges and future prospects. Water SA 42, 551–559. doi: 10.4314/wsa.v42i4.05
Dalu, T., Froneman, P. W., Chari, L. D., and Richoux, N. B. (2014). Colonisation and community structure of benthic diatoms on artificial substrates following a major flood event: a case of the Kowie River (Eastern Cape, South Africa). Water SA 40, 471–480. doi: 10.4314/wsa.v40i3.10
Danovaro, R., Carugati, L., Berzano, M., Cahill, A. E., Carvalho, S., Chenuil, A., et al. (2016). Implementing and innovating marine monitoring approaches for assessing marine environmental status. Front. Mar. Sci. 3:213. doi: 10.3389/fmars.2016.00213
Desrosiers, C., Leflaive, J., Eulin, A., and Ten-Hage, L. (2013). Bioindicators in marine waters: benthic diatoms as a tool to assess water quality from eutrophic to oligotrophic ecosystems. Ecol. Indic. 32, 25–34. doi: 10.1016/j.ecolind.2013.02.021
Desrosiers, C., Leflaive, J., Eulin, A., and Ten-Hage, L. (2014). Optimal colonisation and growth of marine benthic diatoms on artificial substrata: protocol for a routine use of bioindication. J. Appl. Phycol. 26, 1759–1771. doi: 10.1007/s10811-013-0204-3
Doney, S. C. (2010). The growing human footprint on coastal and open-ocean biogeochemistry. Science 328:1512. doi: 10.1126/science.1185198
Edyvean, R. G. J., Rands, G. A., and Moss, B. L. (1985). A comparison of diatom colonisation on natural and artificial substrata in seawater. Estuar. Coast. Shelf Sci. 20, 233–238.
Fleming, L. E., Broad, K., Clement, A., Dewailly, E., Elmir, S., Knap, A., et al. (2006). Oceans and human health: emerging public health risks in the marine environment. Mar. Pollut. Bull. 53, 545–560. doi: 10.1016/j.marpolbul.2006.08.012
Fricke, A., Kopprio, A., Alemany, D., Gastaldi, M., Narvarte, M., Parodi, E. R., et al. (2016). Changes in coastal benthic algae succession trajectories and assemblages under contrasting nutrient and grazer loads. Estuar. Coast. 39, 462–477. doi: 10.1007/s12237-015-9999-2
Giffen, M. H. (1966). Contributions to the diatom flora of South Africa III. Diatoms of the marine littoral regions at Kidd’s Beach near east London, Cape Province, South Africa. Nova Hedwigia 13, 245–292.
Giffen, M. H. (1971). Marine littoral diatoms from the Gordon’s Bay, region of False Bay Cape Province, South Africa. Bot. Mar. 14, 1–16.
Goschen, W. S., and Schumann, E. H. (1995). Upwelling and the occurrence of cold water around Cape Recife, Algoa Bay, South Africa. S. Afr. J. Mar. Sci. 16, 57–67. doi: 10.2989/025776195784156520
Goschen, W. S., Schumann, E. H., Bernard, K. S., Bailey, S. E., and Deyzel, S. H. P. (2012). Upwelling and ocean structures off Algoa Bay and the south-east of South Africa. Afr. J. Mar. Sci. 34, 525–536. doi: 10.2989/1814232X.2012.749810
Griffiths, C. L., Robinson, T. B., Lange, L., and Mead, A. (2010). Marine biodiversity in South Africa: an evaluation of current states of knowledge. PLoS One 5:e12008. doi: 10.1371/journal.pone.0012008
Hawkins, S. J., Pack, K. E., Hyder, K., Benedetti-Cecchi, L., and Jenkins, S. R. (2020). Rocky shores as tractable test systems for experimental ecology. J. Mar. Biol. 100, 1–25. doi: 10.1017/S002531542000104
Hillebrand, H., Blasius, B., Borer, E. T., Chase, J. M., Downing, J. A., Eriksson, B. K., et al. (2018). Biodiversity change is uncoupled from species richness trends: consequences for conservation and monitoring. J. Appl. Ecol. 55, 169–184. doi: 10.1111/1365-2664.12959
Hillebrand, H., and Sommer, U. (1997). Response of epilithic microphytobenthos of the Western Baltic Sea to in situ experiments with nutrient enrichment. Mar. Ecol. Prog. Ser. 160, 35–46.
Hillebrand, H., Worm, B., and Lotze, H. K. (2000). Marine microbenthic community structure regulated by nitrogen loading and grazing pressure. Mar. Ecol. Prog. Ser. 204, 27–39.
Islam, M. S., and Tanaka, M. (2004). Impacts of pollution on coastal and marine ecosystems including coastal and marine fisheries and approach for management: a review and synthesis. Mar. Pollut. Bull. 48, 624–649. doi: 10.1016/j.marpolbul.2003.12.004
Kafouris, S., Smeti, E., Spatharis, S., Tsirtis, G., Economou-Amilli, A., and Danielidis, D. B. (2019). Nitrogen as the main driver of benthic diatom composition and diversity in oligotrophic coastal systems. Sci. Total Environ. 694:133733. doi: 10.1016/j.scitotenv.2019.133773
Kelly, M. G., Cazaubon, A., Coring, E., Dell’Uomo, A., Ector, L., Goldsmith, B., et al. (1998). Recommendations for the routine sampling of diatoms for water quality assessments in Europe. J. Appl. Phycol. 10, 215–224. doi: 10.1023/A:1008033201227
Kelly, M. G., Juggins, S., Mann, D. G., Sato, S., Glover, R., Boonham, N., et al. (2020a). Development of a novel metric for evaluating diatom assemblages in rivers using DNA metabarcoding. Ecol. Indic. 118:106725. doi: 10.1016/j.ecolind.2020.106725
Kelly, M. G., Philliphs, G., Juggins, S., and Willby, N. J. (2020b). Re-evaluating expectations for river phytobenthos assessment and understanding the with macrophytes. Ecol. Indic. 117:106582. doi: 10.1016/j.ecolind.2020.106582
Kelly, M. G., and Whitton, B. A. (1995). The trophic diatom index: a new index for monitoring eutrophication in rivers. J. Appl. Phycol. 7, 433–444. doi: 10.1007/BF00003802
Lemley, D., Adams, J. B., Bornman, T. G., Campbell, E. E., and Deyzel, S. H. P. (2019). Land-derived inorganic nutrient loading to coastal waters and potential implications for nearshore plankton dynamics. Cont. Shelf Res. 174, 1–11. doi: 10.1016/j.csr.2019.01.003
Lobban, C. S., Schefter, M., Jordan, R. W., Arai, Y., Sasaki, A., Theriot, E. C., et al. (2012). Coral-reef diatoms (Bacillariophyta) from Guam: new records and preliminary checklist, with emphasis on epiphytic species from farmer-fish territories. Micronesica 43, 237–479.
MacIntyre, H. L., Geider, R. J., and Miller, D. C. (1996). Microphytobenthos: the ecological role of the “Secret Garden” of unvegetated, shallow-water marine habitats. I. Distribution, abundance and primary production. Estuaries 19, 186–201. doi: 10.2307/1352224
MacLulich, J. H. (1986). Colonisation of bare rock surfaces by microflora in a rocky intertidal habitat. Mar. Ecol. Prog. Ser. 32, 91–96.
Mori, A. S., Isbell, F., and Seidi, R. (2018). ß-Diversity, community assembly, and ecosystem functioning. Trends Ecol. Evol. 33, 549–564.
Nunes, M., Adams, J. B., and Bate, G. C. (2019). The use of diatoms grown on artificial substrata to indicate water quality changes in the lower reaches of the St Lucia Estuary, South Africa. Water SA 45, 149–159. doi: 10.4314/wsa.v45i1.17
Nusch, E. A. (1980). Comparison of different methods for chlorophyll and phaeopigment determination. Arch. Hydrobiol. Beiheft Ergebnise Limnol. 14, 14–36.
Oksanen, J., Blanchet, F. G., Friendly, M., Kindt, R., Legendre, P., McGlinn, D., et al. (2020). vegan: Community Ecology Package. R Package Version 2.5-7.
Park, J. S., Lobban, C. S., and Lee, K. W. (2018). Diatoms associated with seaweeds from Moen Island in Chunk Lagoon, Micronesia. Phytotaxa 351, 101–140. doi: 10.11646/phytotaxa.351.2.1
Parsons, T. R., Maita, Y., and Lalli, C. M. (1984). A Manual of Chemical and Biological Methods for Seawater Analysis. New York, NY: Pergamon Press, 173.
Pattrick, P., Weidberg, N., Goschen, W. S., Jackson, J. M., McQuiad, C. D., and Porri, F. (2021). Larval fish assemblage structure at coastal fronts and the influence of environmental variability. Front. Ecol. Evol. 9:684502. doi: 10.3389/fevo.2021.684502
Pennesi, C., and Danovaro, R. (2017). Assessing marine environmental status through microphytobenthos assemblages colonising the Autonomous reef Monitoring Structures (ARMS) and their potential in coastal restoration. Mar. Pollut. Bull. 125, 56–65. doi: 10.1016/j.marpolbul.2017.08.001
Perissinotto, R., Bornman, T. G., Steyn, P.-P., Dorrington, R. A., and Matcher, G. F. (2014). Tufa stromatolite ecosystems on South African south coast. S. Afr. J. Sci. 110, 1–8. doi: 10.1590/sajs.2014/20140011
Potapova, M., and Charles, D. F. (2007). Diatom metrics for monitoring eutrophication in rivers of the United States. Ecol. Indic. 7, 48–70.
R Core Team (2020). R: A Language and Environment for Statistical Computing. Vienna: R Foundation for Statistical Computing.
Riato, L., Bella, V. D., Leira, M., Taylor, J., and Oberholster, P. J. (2017). A diatom functional-based approach to assess changing environmental conditions in temporary depressional wetlands. Ecol. Indic. 78, 205–213. doi: 10.1016/j.ecolind.2017.03.018
Rimet, F., and Bouchez, A. (2012). Biomonitoring river diatoms: implications of taxonomic resolution. Ecol. Indic. 15, 92–99. doi: 10.1016/j.ecolind.2011.09.014
Round, F. E., Crawford, R. M., and Mann, D. G. (1990). The Diatoms: Biology and Morphology of the Genera. Cambridge, MA: Cambridge University Press.
Ryabushko, L., Miroshnichenko, E., Blaginina, A., and Shiroyan, A. (2021). Diatom and cyanobacteria communities on artificial polymer substrates in the Crimean coastal waters of the Black Sea. Mar. Pollut. Bull. 169:112521. doi: 10.1016/j.marpolbul.2021.112521
Schumann, E. H., Churchill, J. R. S., and Zaayman, H. J. (2005). Oceanic variability in the western sector of Algoa Bay. S. Afr. J. Mar. Sci. 27, 65–80.
Stengenga, H., Bolton, J. J., and Anderson, R. J. (1997). Seaweeds of the South African west coast. Contrib. Bolus Herbarium 18:655.
Taylor, J. C., Prygiel, J., Vosloo, A., de la Rey, P. A., and van Rensburg, L. (2007). Can diatom-based pollution indices be used for biomonitoring in South Africa? A case study of the Crocodile West and Marico water management area. Hydrobiologia 592, 455–464. doi: 10.1007/s10750-007-0788-1
Totti, C., Cucchiari, E., De Stefano, M., Pennesi, C., Romagnoli, T., and Bavestrello, G. (2007). Seasonal variations of epilithic diatoms on different hard substrates, in the northern Adriatic Sea. J. Mar. Biol. Ass. U.K. 87, 649–658. doi: 10.1017/S0025315407054665
Virta, L., Soininen, J., and Norkko, A. (2020). Diversity and distribution across a large environmental and special gradient: evaluating the taxonomic and functional turnover, transitions and environmental drivers of benthic diatom communities. Glob. Ecol. Biogeogr. 29, 2214–2228. doi: 10.1111/geb.13190
Virta, L., Soininen, J., and Norkko, A. (2021). Biodiversity loss threatens the current functional similarity of beta diversity in benthic diatom communities. Microb. Ecol. 81, 290–303. doi: 10.1007/s00248-020-01576-9
Wang, Y., Naumann, U., Wright, S. T., and Warton, D. I. (2012). mvabund – an R package for model-based analysis of multivariate abundance data. Methods Ecol. Evol. 3, 471–474. doi: 10.1111/j.2041-210X.2012.00190.x
Warton, D. I., Wright, S. T., and Wang, Y. (2012). Distance-based multivariate analyses confound location and dispersion effects. Methods Ecol. Evol. 3, 89–101. doi: 10.1111/j.2041-210X.2011.00127.x
Wepener, V., and Degger, N. (2012). Status of marine pollution research in South Africa (1960 – present) Mar. Pollut. Bull. 64, 1508–1512. doi: 10.1016/j.marpolbul.2012.05.037
Witkowski, A., Lange-Bertalot, H., and Metzelin, D. (2000). Diatom Flora of Marine Coasts I Iconographia Diatomologica. Argentina: A.R.G. Gantner Verlag KG.
Zidarova, R., Ivanov, P., and Dzhembekova, N. (2020). Diatom colonisation and community development in Antarctic marine waters – a short-term experiment. Pol. Polar Res. 41, 187–212.
Zuur, A. F., Ieno, E. N., and Elphick, C. S. (2010). A protocol for data exploration to avoid common statistical problems. Methods Ecol. Evol. 1, 3–14. doi: 10.1111/j.2041-210X.2009.00001.x
Keywords: coastal systems, biofilm, diatom composition, succession, diversity, South Africa
Citation: Cotiyane-Pondo P and Bornman TG (2021) Environmental Heterogeneity Determines Diatom Colonisation on Artificial Substrata: Implications for Biomonitoring in Coastal Marine Waters. Front. Ecol. Evol. 9:767960. doi: 10.3389/fevo.2021.767960
Received: 31 August 2021; Accepted: 11 November 2021;
Published: 30 November 2021.
Edited by:
Simone Baldanzi, Universidad de Valparaiso, ChileReviewed by:
Janine Barbara Adams, Nelson Mandela University, South AfricaMarco Fusi, Edinburgh Napier University, United Kingdom
Copyright © 2021 Cotiyane-Pondo and Bornman. This is an open-access article distributed under the terms of the Creative Commons Attribution License (CC BY). The use, distribution or reproduction in other forums is permitted, provided the original author(s) and the copyright owner(s) are credited and that the original publication in this journal is cited, in accordance with accepted academic practice. No use, distribution or reproduction is permitted which does not comply with these terms.
*Correspondence: Phumlile Cotiyane-Pondo, cGwuY290aXlhbmVwb25kb0BzYWVvbi5ucmYuYWMuemE=