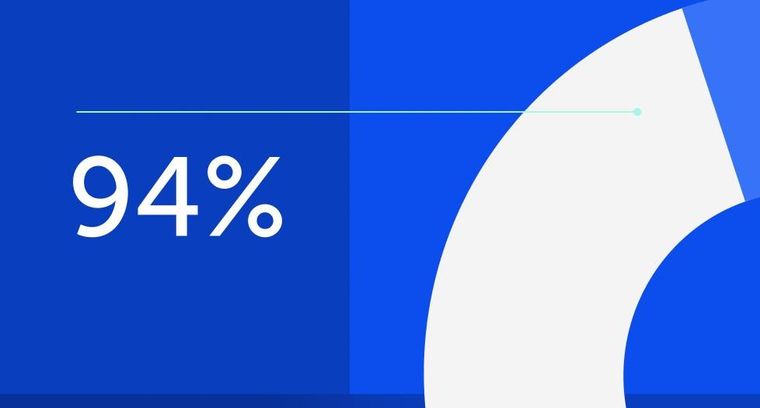
94% of researchers rate our articles as excellent or good
Learn more about the work of our research integrity team to safeguard the quality of each article we publish.
Find out more
REVIEW article
Front. Ecol. Evol., 08 December 2021
Sec. Urban Ecology
Volume 9 - 2021 | https://doi.org/10.3389/fevo.2021.767177
This article is part of the Research TopicEffects of Artificial Light at Night on Organisms: From Mechanisms to FunctionView all 13 articles
Artificial light at night (ALAN) is closely associated with modern societies and is rapidly increasing worldwide. A dynamically growing body of literature shows that ALAN poses a serious threat to all levels of biodiversity—from genes to ecosystems. Many “unknowns” remain to be addressed however, before we fully understand the impact of ALAN on biodiversity and can design effective mitigation measures. Here, we distilled the findings of a workshop on the effects of ALAN on biodiversity at the first World Biodiversity Forum in Davos attended by several major research groups in the field from across the globe. We argue that 11 pressing research questions have to be answered to find ways to reduce the impact of ALAN on biodiversity. The questions address fundamental knowledge gaps, ranging from basic challenges on how to standardize light measurements, through the multi-level impacts on biodiversity, to opportunities and challenges for more sustainable use.
Our planet faces numerous challenges, many of which have direct and indirect connections to biodiversity (Díaz et al., 2020). One such challenge is artificial light at night (ALAN) leading to a fundamental change in the light environment over half of the Earth’s surface—the Earth at night. ALAN has been growing exponentially since the nineteenth century and currently increases by 2–6% per year worldwide (Hölker et al., 2010a; Kyba et al., 2017). ALAN has been introduced in places, at times, spectra and intensities that do not occur naturally (Gaston et al., 2015). Ecosystems are largely organized by natural light-dark cycles, i.e., diurnal, yearly and lunar cycles, which have been stable over geological and hence evolutionary time scales. ALAN-induced disruptions of those cycles affect the structure and function of multiple levels of biodiversity that are again strongly interconnected (Longcore and Rich, 2004; Hölker et al., 2010b; Gaston et al., 2013). Given the growing global pervasiveness of ALAN (Kyba et al., 2017; Gaston et al., 2021), it is important to understand how multiple levels of biodiversity respond to it directly and indirectly (Figure 1).
Figure 1. Impact of artificial light at night (ALAN) on multiple levels of biodiversity. The multiple levels of biodiversity are interlinked, i.e., one level of biodiversity may respond to ALAN and modify processes at other biodiversity levels. For example, ALAN may impact the gene expression of certain clock genes, which results in a reduced fitness of individuals and a population decrease due to a phenological mismatch with other species and finally a changed community composition. This may impact ecosystem processes and nocturnal lightscapes (e.g., forests, coral reefs), which in turn influences all other levels.
Research into the ecological impacts of ALAN has exploded in recent years, mostly focusing on changes in species behavior and physiology (Gaston et al., 2015; Grubisic et al., 2019; Sanders et al., 2021). Yet, many “unknowns” remain that need to be addressed before we can understand and predict the impact of ALAN on multiple levels of biodiversity (genes and cells, individuals, populations, communities, ecosystems and landscapes, Figure 1), and develop effective mitigation measures. Here, we address these “unknowns” by synthesizing the results of a special session and follow-up discussions at the first World Biodiversity Forum in Davos, Switzerland, in 2020, where experts from across the world convened to discuss the impacts of ALAN on multiple levels of biodiversity over a broad spectrum of taxa in multiple biomes (aerial, aquatic, and terrestrial). We capture the complexity of the problem as broadly as possible by considering that different natural light cycles (Figure 2A) are affected by multiple forms of ALAN (e.g., streetlights, advertising lighting, skyglow, Figure 2B) with multiple effects (e.g., lethal attraction of organisms, disruption of circadian rhythms and erosion of ecosystem functions) at multiple levels of biodiversity in multiple realms (Figure 2C). Already at the World Biodiversity Forum it became clear that a transition toward the more sustainable use of ALAN is extremely challenging and requires answers to questions that can only be tackled by broadening the disciplinary perspective to strengthen transdisciplinary approaches.
Figure 2. Artificial light at night: Potential sources, biodiversity impacts and responses are complex. Different natural light cycles (A) are affected by multiple forms of ALAN (B) at multiple levels of biodiversity in multiple realms (e.g. from bottom up: gene expression; phenotypes; population dynamics, e.g. decline; community composition; species dispersal and/or organismic fluxes across ecosystem boundaries and bioms) (C). ALAN can interact with multiple global change stressors (D). Due to the potentially conflicting demands of ALAN a transition to a more sustainable use is extremely challenging and requires multiple levels of regulations (E).
We identify 11 research questions that can be clustered into three main themes. We first outline questions linked to the diverse nature of natural and ALAN. Second, we outline questions related to the effects of ALAN on multiple levels of biodiversity. Finally, we formulate research needs on how to bend the curve of ALAN-induced biodiversity loss.
ALAN research is inherently interdisciplinary, with knowledge of the nighttime being fragmented across multiple subject areas including astronomy, physics, ecology, chronobiology, psychology, and engineering. Each field has different motivations for conducting ALAN research, and draws upon different instrumentation, measurement conventions, and experimental frameworks. Multiple units of measurement for light are encountered across the sciences, and many have little biological relevance. The absence of instruments capable of performing light at night measurements with the required level of detail, and insufficient training of biologists in radiometry and light propagation further compound this problem.
Q1 How to harmonize light measurement methods across disciplinary boundaries?
The interdisciplinary nature of ALAN research has resulted in different measurement approaches, procedures, and the use of various light units (Hänel et al., 2018), which complicates comparison of results (Kalinkat et al., 2021). Lighting engineers measure mainly in human-centric photometric SI units (mostly horizontal illuminance in lx, Figure 3), which some ALAN researchers have adapted for their studies to better facilitate the translation of results into lighting policy (see Q11). Astronomers mainly measure the radiance of the night sky (mostly at zenith) in units of magnitudes/arcsec2 in different astronomical bands (see e.g., Patat, 2008). Some ALAN researchers have adapted the use of a simple radiometer, the Sky Quality Meter (SQM) that has its own spectral band (Hänel et al., 2018), which can be extended to multiple color channels (Kyba et al., 2012; Sánchez de Miguel et al., 2017). The meaningfulness of single point SQM measurements for biodiversity is, however, questionable and can be used in the wrong context (Longcore et al., 2020). Biologists tend to measure either irradiance or radiance in different spectral bands (e.g., photosynthetically active radiation—PAR) and sometimes report W (Watts) in micromole photons per seconds (μmol/s). Visual ecologists prefer wavelength resolved “hyperspectral” measurements, requiring high sensitivity spectrometers to resolve at low nighttime light levels (see Spitschan et al., 2016). Moreover, even within biodiversity research there are large disciplinary differences in the state of the art for measuring light. For example, while an array of measurement systems exists for terrestrial habitats, the attenuation of light in water makes hyperspectral light measurements even more challenging in aquatic ecosystems (Jechow and Hölker, 2019a; Tidau et al., 2021).
Figure 3. Ranges of exposure that animals experience and respond to with natural variation in light and light intensities observed with ALAN (here using human-centric metric lux). Illuminance during day, twilight, and night as a function of elevation angle of sun and moon; yellow solid line—sun illuminance on clear day, gray dashed line—moonlight full moon.
For biodiversity studies, nocturnal light ideally would be measured in biologically relevant ways, based on thresholds and spectral sensitivities of the species under question (see Q2 and Q5), because different light sources interfere differently with the large diversity of sensory systems in nature (Davies et al., 2013; see Q3). Furthermore, it is important to perform and provide ALAN-free natural light reference measurements for different habitats, seasons and weather conditions (Jechow and Hölker, 2019b). One challenge is to break disciplinary boundaries by, for example, connecting photometry of anthropogenic light sources (performed by lighting engineers) and night-sky or night-time radiometry (see e.g., Foster et al., 2021) to visual ecology and species responses (van Grunsven et al., 2014; Longcore et al., 2018; Seymoure et al., 2019). Thus, standardized light measurements that allow comparison across ALAN disciplines are desperately needed. Recent proposals favor spatially resolved multi-spectral night-time radiance measurements of the full light field (not just the upper hemisphere or at zenith) with digital cameras with fisheye lenses in the RGB bands (Jechow et al., 2019; Nilsson and Smolka, 2021), which is further supported by new calibration strategies (Fiorentin et al., 2020; Cardiel et al., 2021) and the proposal of a dark sky unit (Kolláth et al., 2020). This method has radiance and irradiance information in three spectral bands in one image. Additional hyperspectral measurements or species-specific bands, however, are required for visual ecologists, and a translation to photometric units is essential for policy making and the connection to lighting professionals. Thus, a wider and more interdisciplinary harmonization of different needs for a broad application is required to help to establish standardized protocols that are currently lacking. These protocols should match the ecological and biological responses being investigated in terms of spectral band and resolution, directionality (radiance vs. irradiance; scalar vs. planar) and time scale (see Q2). Such consistent and cross-disciplinary standards for measurement are also necessary to formulate thresholds for mitigation and management (Jechow and Hölker, 2019b; Davies et al., 2020; see Q11).
Q2 What are biodiversity-relevant light-measurements and methods?
The multiple realms and levels of biodiversity all have specific photic properties and measurement requirements. At the same time, quantifying ALAN is rather a complex task that requires method development and training. Commercial, off-the-shelf measurement equipment is rarely appropriate because it lacks sensitivity and sufficient spatial and/or spectral resolution. This becomes particularly challenging in aquatic or aerial environments (Jechow and Hölker, 2019a). Furthermore, ecologists often lack sufficient understanding of radiometry in terms of units and measurement approaches, making it hard for ecologists to interpret measurements obtained outside of their field and translate them for their research (see Q1).
In ecological studies, information on ALAN is in most cases derived from single point ground-based measurements with limited spatial, temporal and spectral resolution, very often using human centric devices like lux meters. To be able to understand what an organism perceives, it is important to have the full spatial and spectral information of the light field. This could be acquired either with a spectroradiometer mounted on a rotational head that scans the radiance over the whole sphere (Kocifaj et al., 2018) or with a full-sphere hyperspectral camera that works at night-time, which is not available yet (but see Alamús et al., 2017 for night-time measurements and Shiwen et al., 2021 for full-sphere hyperspectral imaging in a forest during day). Both solutions are not technically mature, and the best current approximation is full-sphere imaging with a fisheye lens digital camera system with limited spectral resolution in the RGB bands (Jechow et al., 2019). Further technological development toward a hyperspectral solution are necessary and interim steps could be adding additional spectral bands to such imaging systems like in the ASTMON system (Aceituno et al., 2011) or by tailoring camera systems to achieve hypercolorimetric multispectral imaging (Colantonio et al., 2018). Ideally, the measurement strategy should also cover temporal variations in light on short time scales but also seasonal variations (Figure 2A). Remaining obstacles of such a holistic approach are the complexity of data and handling as well as potential high costs of a sophisticated device. A combination of multiple measurement devices (multispectral camera, hyperspectral single point, photometric single point) might be a more practical compromise.
Another pressing issue is the extrapolation of single point ground-based measurements to larger areas, which is relevant, for example, for migratory species. Here, challenges are posed by remote sensing approaches that are almost always only proxies for the ecological variable of interest. Night-time satellite data are limited in spatial, spectral and temporal resolution, but color imaging from the international space station ISS, airborne measurements, and particularly UAVs have the potential to fill the existing gaps in ALAN related biodiversity research (Bouroussis and Topalis, 2020; Levin et al., 2020; Sánchez de Miguel et al., 2021). A key component to improving the utility of these data would be research efforts that can translate what remotely sensed values could represent for conditions at ground level, where first steps have been taken but uncertainties remain high (Simons et al., 2020). Furthermore, there is an urgent need for stand-alone satellite missions focused on understanding light pollution and its effects on biodiversity (Barentine et al., 2021).
Focusing on skyglow is becoming a larger component of ALAN research on biodiversity (Kyba and Hölker, 2013). Skyglow occurs when ALAN radiates or reflects toward the sky and the light scatters at atmospheric particles and brightens the night sky (Aubé, 2015). In contrast to direct ALAN, skyglow can act as a pollutant far away from its origin and therefore has the potential to affect biodiversity over large spatial scales. Skyglow is dynamic as it depends on atmospheric constituents, seasonal effects such as leaf cover and ground albedo (Jechow and Hölker, 2019b) and can be dramatically amplified by clouds (Kyba et al., 2011) causing ground illuminance brighter than moonlight in extreme situations (Jechow et al., 2020; Figure 3). Skyglow is often insufficiently quantified with single channel spectral and spatial measurements at zenith. Again, multispectral (RGB) fisheye-lens digital camera systems are promising for skyglow measurements with more ecologically relevant information but such systems need wider application in ecology (Thums et al., 2016; Levin et al., 2020). Permanent installations can track skyglow dynamics across the full sky dome (Jechow et al., 2018) and if a similar measurement system is used for quantification of direct light pollution, results become comparable.
Linkage between skyglow and remotely sensed night-time light is possible via modeling. While a static world-wide model for skyglow exists and is being widely used in ecological studies (Falchi et al., 2016), a dynamic model that includes atmospheric changes, cloud cover, snow, vegetation cover and similar factors is still lacking.
Increasing evidence shows that the interference of ALAN with natural cycles of light and darkness, i.e., changes of photoperiod, intensity and spectra, influences a wide range of biological processes, from gene expression to ecosystem functioning, yet many questions remain about species and trait specific sensitivities to ALAN, and how these affect biodiversity at different scales (Gaston et al., 2015; Hopkins et al., 2018; Dominoni et al., 2020a; Falcon et al., 2020; Sanders et al., 2021; Figure 2). In this context, the following eight questions (Q3–Q10) need to be addressed.
Q3 What are the relevant photoreceptor systems and their key sensitivities?
Photosensory systems are near ubiquitous in nature and are found across the animal and plant kingdom down to single-celled organisms. Accordingly, photosensory systems and their response to light are hugely diverse, ranging from single photoreceptor cells to complex image forming camera-type eyes, which can capture (spatial) information and facilitate color guided behaviors as well as polarization patterns invisible to humans (Horváth et al., 2009; Land and Nilsson, 2012).
Photic stimuli, however, strongly vary between biological realms, across seasons and over the course of a day (Figures 2A, 3). For example, the optical properties of freshwater and seawater strongly attenuate the light aquatic organisms are exposed to, shaping light environments that greatly differ in spectral composition, intensity and spatial information from those on land. Seawater in the open ocean attenuates blue light the least and hence many marine organisms are sensitive in this spectral region. In contrast, coastal waters and freshwater systems are transparent at different (typically longer) wavelengths and variable in their inherent optical properties, causing a less specific adaptation of organisms for specific wavelengths (Grubisic et al., 2019; Kühne et al., 2021).
Many nocturnal and crepuscular organisms are adapted to dim photic stimuli; some can use celestial bodies as a source of information (Dacke et al., 2003; Ugolini et al., 2003; Foster et al., 2018). Moonlight serves as a major environmental cue, for example entraining diel vertical migration on zooplankton down to 100 m (Last et al., 2016). On the other hand, the high sensitivity to low intensities of natural light makes those organisms (both terrestrial and aquatic) prone to disruptions even by low intensity ALAN, such as the globally widespread artificial skyglow (Moore et al., 2000; Kupprat et al., 2020; Torres et al., 2020; Figure 3).
ALAN research is inevitably inhibited by the lack of species for which photoreceptor systems and key spectral and light sensitivities of photobiological responses have been adequately described (e.g., overview for marine organisms in Tidau et al., 2021). For one of the best photobiologically studied classes, insects, a recent literature search revealed information on the spectral sensitivity of photoreceptors of only 221 insect species from 82 genera and 13 orders (van der Kooi et al., 2021). With almost 1 million species, half of which are nocturnal (Hölker et al., 2010b), this represents less than 0.03% of all insect species. Nonetheless, phylogenetically conserved patterns of sensitivity have been identified in some animal classes. Both visual and non-visual photoreceptor systems and their corresponding spectral sensitivities can be conserved within taxonomic groups (e.g., in mammals melanopsin and sensitivity to blue wavelengths). Most terrestrial insects are particularly attracted to ultraviolet and blue light (Donners et al., 2018; but see also Owens and Lewis, 2021). Similarly, most arthropods show a greater responsivity to blue light and most vertebrates show a lower responsivity to longer wavelengths (Davies et al., 2013; Longcore et al., 2018; Grubisic et al., 2019). Furthermore, within habitats, specific spectral sensitivities might be common to the organisms living there. For example, many species of aquatic turtles and fish are more sensitive to longer wavelengths in freshwaters and to shorter wavelengths in clear marine systems, i.e., their sensitivities relate to the optical water properties where they typically evolved (Grubisic et al., 2019; Wyneken and Salmon, 2020).
More fundamental research in visual biology is needed to describe both the inherent sensitivity of animal visual and non-visual systems to base ALAN research on. In addition, the spectral dependence of behavioral and physiological responses to light, and their relationship to intensity, ecological context, previous light exposure, and other factors demand attention.
Q4 Which species traits are most sensitive to ALAN?
Generalizations about which traits of species may be most sensitive to certain factors of global change are useful for predicting their ecological consequences. As with many manmade impacts on the natural environment, nighttime lighting can filter out species with functional response traits that cause species to be more sensitive to ALAN (Franzén et al., 2020; Cox et al., 2021; Voigt et al., 2021). To give an example, high sensitivity to light in the eyes of North American bird species has been associated with a greater advancement of reproductive timing in response to light exposure, possibly leading to phenological mismatches (Senzaki et al., 2020). Elucidating biological traits that predicate sensitivity to ALAN is therefore critical for identifying ALAN-vulnerable species around the world (Secondi et al., 2020).
Light-dark cycles vary along latitudinal gradients (Hut et al., 2013), hence species traits that predicate sensitivity to ALAN are also expected to vary with latitude (Secondi et al., 2020). While birds in lower latitudes started to sing earlier when exposed to ALAN, in high latitudes the seasonal increase of natural light can mask ALAN effects on the onset of bird song (Da Silva and Kempenaers, 2017). Furthermore, 47 out of 140 bird species studied in North America are becoming more abundant with increasing light exposure during longer nights, probably because light extends the perceived photoperiod and birds take advantage of higher visibility (Wilson et al., 2021).
Meta-analyses of species demonstrably impacted by ALAN proved to be useful for identifying ALAN sensitive traits (e.g., Sanders et al., 2021), which may include eye/body size ratios indicating light sensitivity, aspects of eye morphology, mobility (e.g., sessile organisms vs. mobile), geographical range size (e.g., probability that migrating species have of encountering ALAN), life history traits, temporal niche (e.g., nocturnality or crepuscularity), habitat affiliation, and seasonal and lunar phenological events (e.g., timing of reproduction). We are just beginning to understand which biological traits predict sensitivity to the disruption of natural light intensity, cycles, and spectra due to ALAN (Grubisic et al., 2019; Sanders et al., 2021). Future studies should also examine in more animal and plant species how functional traits and contexts (e.g., latitude, habitat affiliation) relate to sensitivity to ALAN.
Q5 Above which thresholds does ALAN exposure become critical?
The majority of documented ALAN effects on species are in response to single exposure levels (Davies et al., 2017; Manfrin et al., 2017; van Grunsven et al., 2020). These studies have proved valuable for drawing attention to the sheer scale of ALAN impacts on individual species. In reality, irradiance of ALAN perceived by organisms varies spatially. An important prerequisite for upscaling to different ecosystems and landscapes (see Q9) is an understanding of changes in the measured biological responses as a function of multiple exposure levels (Brüning et al., 2015; Sanders et al., 2015; de Jong et al., 2016). Although challenging to deliver, and sometimes giving idiosyncratic results, dose-response experiments have proved powerful at identifying critical exposure thresholds in toxicology (Vandenberg et al., 2012), and should be a focal point for current and future ecological light pollution experiments (Brüning et al., 2015; de Jong et al., 2016; Kupprat et al., 2020). In particular, we need more studies testing for the effects of ALAN of lower intensities (e.g., from skyglow), which many organisms may experience throughout large areas worldwide (Kyba and Hölker, 2013; Grubisic et al., 2019) (see Q2).
Similarly, we lack an understanding of the sensitivity of species to the spectra of ALAN. Quantifying wavelength-dependent responses to ALAN is critical to predicting the impact of different lighting technologies and identifying spectra that can minimize deleterious impacts (Spoelstra et al., 2015; Brüning et al., 2016; Donners et al., 2018; Longcore et al., 2018). The utility of this approach is empirically well demonstrated; however, its application across a broader range of species is confined to those whose spectral response curves have been quantified. Consistencies in the number of photoreceptors and maximal wavelengths of sensitivity can and are used to form generalizations across broad taxonomic groupings (e.g., class, order; Davies et al., 2013; van Grunsven et al., 2014; Kühne et al., 2021). High precision insights into the responses of individual taxa however remain constrained by published spectral sensitivity information. Advances in our understanding of ALAN impacts are, in this way (and many others), partly limited by advances in fundamental photobiology and visual ecology (see Q3).
Q6 How and at what rates can populations adapt to ALAN?
The spatial light distribution, spectral composition, and the intensity of ALAN are unprecedented on evolutionary time scales (Hopkins et al., 2018). As when studying many anthropogenic impacts on the natural world, initial experiments have quantified behavioral and/or physiological responses to ALAN (Tuomainen and Candolin, 2011; Gaston et al., 2015). These effects on the individual will, however, compromise organism fitness (Sih et al., 2011) such as survival and reproductive success, ultimately manifesting impacts on population demography, and/or lead to microevolution (Figure 2C). To give one example, insects that are drawn to light will either die (e.g., by predation or exhaustion) or have reduced fitness compared to individuals of the same population that are less attracted by light. If this variation in light attraction has a heritable basis, this may lead to a response to the selection of genotypes in the population that are less attracted to light (Altermatt and Ebert, 2016). In those cases, ALAN will lead to micro-evolution. To date, much of the available knowledge on ALAN impacts is based on short-term experiments that are not able to observe evolutionary compensation mechanisms over long periods of time (Gaston et al., 2015; Kalinkat et al., 2021).
The challenge of analyzing ALAN-related trait changes (e.g., body size, relative eye size, and wing length, see Q4) over many generations could be addressed by examining museum vouchers collected for long periods of time (e.g., Keinath et al., 2021). Furthermore, ALAN research could adopt approaches and tools from quantitative genetics to understand and predict how species evolutionarily adapt to changes in their light environment. For this, both selection on ALAN-related traits needs to be measured and the heritability of these traits estimated. The rate of genetic change may, however, be small, as has been shown for wild species (Charmantier and Gienapp, 2014), and is likely to be too slow to adapt. This is because anthropogenic disturbances often introduce more rapid rates of environmental change compared to what organisms have experienced in their evolutionary past (Palumbi, 2001). The rate of genetic change is likely to be higher for species with a short generation time and standing genetic variation, such as microorganisms or insects. Indeed, some examples have been documented of the micro-evolution of insect species in response to climate change (Bradshaw and Holzapfel, 2001; Van Asch et al., 2007). In this context, evolutionary trap theory may offer a framework for understanding and mitigating the effects of ALAN (Haynes and Robertson, 2021).
Q7 How does ALAN alter biodiversity by redistributing species?
ALAN is known to affect the behavior of species, including altered orientation, navigation, foraging, and predator avoidance behaviors of a wide range of organisms (McLaren et al., 2018; Manríquez et al., 2019). One frequently observed phenomenon is the aggregation of individuals in artificially lit patches, reducing their presence in the darker surroundings. Conversely, the density of species repelled by light is likely to increase in dark locations neighboring illuminated areas (Manfrin et al., 2017; Giavi et al., 2020). Such heterogeneous responses to ALAN among and within taxonomic groups change species distribution patterns and create novel communities (Hölker et al., 2015; Sanders and Gaston, 2018; Voigt et al., 2021; Figure 2C) with potential cascading effects on ecosystem functions such as mineralization, pollination, or seed dispersal (Lewanzik and Voigt, 2014; Knop et al., 2017; van Grunsven et al., 2018). To date, our knowledge on how the effects of ALAN on community composition might be scaling up to affect ecosystem processes remains limited (Knop et al., 2017; Grubisic et al., 2018; Giavi et al., 2020).
Long term monitoring studies replicated at large spatial scales represent one option for quantifying changes in species distributions in response to ALAN, but they are still very rare (van Grunsven et al., 2020; Kalinkat et al., 2021). One reason is that such experiments present significant logistical and financial challenges, principally due to the large levels of replication required to control for multiple confounding environmental factors that are likely collinear with ALAN. A further challenge is to have data on how long and with which light properties ALAN has been applied (see Q2). Since there can be marked between-year variation in the influences of ALAN, it is critical to run such experiments linked to environmental context and seasonal timing over several generations of key species (ideally more than 10 years, van Grunsven et al., 2020; Kalinkat et al., 2021).
Q8 How does ALAN affect biodiversity through indirectly altering species interactions?
Global environmental pressures threaten biodiversity directly through changes in species’ physiology and behavior, and indirectly through interactions between impacted species and other species within ecological communities (Tylianakis et al., 2008). To date only a few studies have quantified indirect effects of ALAN caused by altered species interactions within (Knop et al., 2017; Giavi et al., 2020, 2021), or across trophic levels (Bennie et al., 2018; Manfrin et al., 2018; Sanders et al., 2018; Maggi et al., 2020), and we are far from being able to predict where indirect effects occur and their likely importance for the wider ecosystem.
Further mesocosm experiments that manipulate a more diverse array of interacting communities are needed (Sanders et al., 2018). Also, further field studies are necessary such as studies on changes in the structure of entire species interaction networks and linking these to ecosystem functions (e.g., Knop et al., 2017). Furthermore, indirect approaches that document altered species interactions due to ALAN, such as stable isotope analyses (Manfrin et al., 2018), molecular analyses of gut contents or fecal samples (Cravens et al., 2018), GPS data analyses of predator–prey dynamics (Ditmer et al., 2021), or the analyses of pollen transport networks (Macgregor et al., 2017) are other promising approaches. Finally, more emphasis should also be placed on spatial (Giavi et al., 2020) and on temporal indirect effects of ALAN on species interactions.
Q9 What are the effects of ALAN on biodiversity at the ecosystem and at the landscape level?
Effects of global change drivers on biodiversity and ecosystem functioning might vary between ecosystems (Sage, 2020). In this vein, we can expect that the effect of ALAN on biodiversity will not be consistent across ecosystems and landscapes with some systems and areas being more susceptible to light pollution than others. On the one hand, the spread of light within ecosystems might vary depending on ecosystem type, lighting technology and medium (e.g., air, water, see Q3). The structure of a forest, for example, leads to a stronger attenuation of light (vertically and horizontally) compared to grasslands, which may make species adapted to closed habitats more vulnerable to ALAN compared to species adapted to open habitats (Voigt et al., 2021; Wilson et al., 2021). On the other hand, different latitudes harbor different ecosystems and hence species inhabiting them. Yet, the extent to which photoperiod and climate modulate exposure to ALAN at a given latitude is unknown (Secondi et al., 2020). Also, ecosystems interact and thus changes in one ecosystem due to ALAN will likely also impact linked ecosystems (e.g., Manfrin et al., 2017; Figure 2C). Finally, there is mounting evidence of ALAN impacts on ecosystem engineers such as corals and intertidal crabs, which can again modify the environmental context they are embedded in and hence affect biodiversity at the ecosystem level (Ayalon et al., 2021; Nuñez et al., 2021).
Light corridors and networks (e.g., illuminated roads), as well as light patches (e.g., an illuminated gas station in a dark environment) can impact landscapes by acting as barriers to movement and dispersal, and as population sinks (Degen et al., 2016; Laforge et al., 2019; van Grunsven et al., 2020). Changing the ability of species to move through landscapes may alter foraging and reproductive opportunities for individuals, modifying habitat connectivity and gene flow between populations, disrupting recolonization of habitat patches, and altering metapopulation dynamics (Caplat et al., 2016; Grubisic et al., 2018; Camacho et al., 2021; Gaston et al., 2021).
Even though the effects of ALAN on biodiversity might vary across ecosystems and landscapes, most research has focused on a very limited range of ecosystems, namely on natural terrestrial systems of temperate and developed regions. Also, nearly all documented effects are on individuals and ecological communities, while our understanding of effects on ecosystems and at the landscape level remains limited (Secondi et al., 2020). Empirical upscaling of individual level responses (see Q5) to changes in species biogeography would provide compelling evidence of ALAN’s potential to reshape nature at the landscape scale. Thus, despite challenges in accessing certain ecosystems (marine offshore, high altitudes, lakes) remain, more work in a wider array of ecosystems and landscapes is warranted, both within and between biogeographic realms.
Q10 How does ALAN interact with multiple global change stressors?
ALAN is considered to be a major driver of global change with negative consequences for biodiversity (Hölker et al., 2010b; Davies and Smyth, 2018). It typically co-occurs with other global change drivers, such as climate change, anthropogenic noise, or land-use change, especially in urban areas (Perkin et al., 2011; Halfwerk and Slabbekoorn, 2015; Swaddle et al., 2015; Dominoni et al., 2020a; Figure 2D). Interactive effects of ALAN with other global change drivers are therefore likely (Rillig et al., 2019), which could be additive, antagonistic or synergistic (Jackson et al., 2016; Birk et al., 2020). To give one example, Miller et al. (2017) demonstrate that night-time warming combined with light pollution had non-additive impacts on predator–prey interactions. These stressors, however, often vary in parallel making it challenging to disentangle their effects on biodiversity.
A number of approaches exist to quantify the impact of ALAN on biodiversity in combination with other global change factors. While none of these in isolation will be enough to resolve the complexity of multiple interacting stressors, they can provide discrete novel insights that collectively provide a weight of evidence to direct future research. Firstly, under controlled conditions fully crossed factorial experiments can empirically quantify the existence of interactions between global change stressors (McMahon et al., 2017; Dominoni et al., 2020b). Secondly, when ALAN is experimentally controlled in natural situations for years, and a second factor varies over time, like temperature or precipitation, the interaction between ALAN and these stressors can be assessed. For instance, there is only an effect of ALAN on seasonal timing in great tits (Parus major) in cold springs, when the birds lay on average late (Dominoni et al., 2020c). A similar observation has been made for the impact of ALAN on the timing of bud-burst, with a pronounced effect of light on late-budding tree species (Ffrench-Constant et al., 2016). Thirdly, when the intensity or spectral characteristics of ALAN are changed and another stressor remains constant, a BACI (before-after, control-impact) approach could be used to quantify any emergent responses over time. Fourthly, in a recent study, Wilson et al. (2021) introduced another promising approach to disentangle the combined effects of different anthropogenic stressors. They used a large data set generated by community and citizen scientists1 that allowed them to analyze effects of ALAN and noise pollution on bird occurrences. Although this approach is prone to various spatial and temporal biases (e.g., Geldmann et al., 2016), the large scale and high number of observations in such projects may enable insightful analyses given carefully tailored statistical models (e.g., Bird et al., 2014). In a similar vein, so-called distributed experiments by multiple research teams across countries and continents (e.g., the NutNet experiment; Borer et al., 2014) are another option to capture a much larger range of co-exposure to ALAN and other relevant stressors. To date we are not aware of any distributed experiments that explicitly address ALAN in combination with other stressors. Finally, the additive or interactive effects of stressors can be estimated from meta-analysis, provided that there are a sufficient number of studies (Birk et al., 2020).
Light pollution is only recently coming to the attention of those beyond the interested scientific communities such as lighting professionals (Schulte-Römer et al., 2019; Pérez Vega et al., 2021). The effect of light pollution in environmental and social considerations remains largely under-acknowledged. The consequence is that “sustainable lighting” currently aims mainly toward energy efficient technology to reduce the carbon footprint of lighting while ignoring the adverse effects of light pollution on biodiversity. To bend the curve of biodiversity loss (i.e., to reverse the decline) solid transdisciplinary solutions that have emerged from a collaboration of practice, research, production, decision-making and planning are crucial.
Q11 What are opportunities and challenges for an effective management of ALAN?
Although there is already evidence of readily available, and inexpensive, mitigation strategies that work (e.g., light orientation, proper shielding, intensity scaled to intended use, and spectral tuning (Hölker et al., 2010a; Gaston et al., 2012; Schroer and Hölker, 2017), uncertainty remains regarding which approaches are best for reducing the ecological effects of ALAN. The pros and cons of different approaches continue to be debated at length as a result of conflicting cultural, political, economic, and institutional demands (Figure 2E). At the same time, novel lighting technologies and concepts are constantly emerging. Thus, biodiversity-friendly ALAN solutions need to be solicited with a broad range of actors originating from different backgrounds, which makes successful negotiations for sustainable lighting challenging (see also Q1).
Many attempts to reduce light pollution run up against positive connotations of lighting (e.g., aesthetics, modernity, and security), which are deeply ingrained in modern societies (Jakle and Thompson, 2001; Hölker et al., 2010a). While there is a general perception that urban lighting improves safety and security regarding traffic accidents and crime, the empirical evidence is not very solid (Marchant et al., 2020). Awareness raising campaigns are needed to garner public support for implementing biodiversity friendly ALAN management strategies (Zielińska-Dabkowska et al., 2020), however such campaigns should draw on the benefits of darkness as a source of quietness and recovery, as much as its importance for biodiversity conservation.
Despite numerous attempts to control light pollution and to reduce its impact through policies regulating the use of outdoor ALAN, minimal success has been achieved in a limited number of geographic areas (Barentine, 2020). One reason for this is that the regulatory management of ALAN depends heavily on the political and administrative actors involved, who must take into account various aspects such as safety and security, energy efficiency, design, and health and environmental concerns. Future transdisciplinary policy initiatives to address light pollution must therefore consider the many benefits of ALAN while addressing its negative impacts (Hölker et al., 2010a; Challéat et al., 2021). A legislative shortcoming in environmental protection is that often only species with special protection status are protected if they show, for example, avoidance behavior toward ALAN. Adverse effects on species and landscapes without special protection status are rarely considered by existing regulations (Schroer et al., 2020). In addition, outdoor lighting policies should consider the entire makeup of urban lighting (for example advertising, architectural lighting, and sports lighting), rather than focus solely on road lights (Kyba et al., 2021). A promising example is the German “insect protection” law recently implemented in the Federal Nature Conservation Act (Thomas, 2021), which aims to achieve a balance between emission regulation and immission control. The respective ordinance is still pending, where several issues on the measurement and assessment have to be specified (especially Q1–Q5). The conservation concept of implementing dark ecological networks consisting of core areas, corridors, and buffer zones to limit the impacts of light pollution on biodiversity at the landscape level is another interesting example (e.g., Challéat et al., 2021).
Although lighting professionals (e.g., design and industry) increasingly acknowledge ALAN as a threat to biodiversity, there are diverging views regarding potential obstacles to light pollution mitigation (Schulte-Römer et al., 2019; Pérez Vega et al., 2021). Current technological advancements in outdoor lighting, particularly LEDs, in principle allow developing lighting mitigation strategies that balance conflicting interests between humans and biodiversity, but this potential remains largely untapped (Longcore, 2018; Bolliger et al., 2020; Deichmann et al., 2021; Jägerbrand and Bouroussis, 2021). Furthermore, LEDs are an energy efficient technology that promises net savings in energy consumption. Past experience has shown that lighting is often subject to a strong rebound effect, where an increase in luminous efficacy resulted in higher light consumption rather than the targeted energy savings. Unfortunately, such a rebound effect is most likely also currently observed for LED technology, which can ultimately lead to further loss of natural nightscapes (Hölker et al., 2010a; Kyba et al., 2014).
A systematic consideration of ALAN issues that facilitates successful translation to a future sustainable lighting policy that harmonizes the needs of diverse stakeholder groups is still lacking (Pérez Vega et al., 2021). Achieving this demands inter- and transdisciplinary research involving collaboration between lighting engineers, ecologists, and other relevant stakeholder groups. Interdisciplinary institutions that work on the topic of light pollution and biodiversity conservation are currently lacking, in part because consideration of nighttime ecology is significantly underrepresented in ecological research. Gaston (2019) argues for a synthetic research program in this area of science. Interdisciplinary institutions such as research institutes or university departments for nighttime and light pollution research could be of great help to develop multi-level and cross-scale concepts, assessments, and evaluations of developments toward sustainable lighting (Kyba et al., 2020).
Due to anthropogenic activities, biodiversity has declined around the globe (IPBES, 2019) and global biodiversity is facing a sixth mass extinction (Barnosky et al., 2011). ALAN is one of the global change drivers (Davies and Smyth, 2018) contributing to the worldwide decline of biodiversity. Our summary of 11 key pressing questions shows that future research needs to address a mix of complex and interrelated questions to better assess the consequences of ALAN for biodiversity and to have a basis for designing efficient measures to minimize its ecological impacts. The goal for future interdisciplinary research should be to guide the diverse field of research, so information on biodiversity-relevant nocturnal light will be accessible, rigorous, and comparable across studies and disciplines. This requires a broader thinking about how to best characterize and measure ALAN from the perspective of the species or habitat of concern. Furthermore, we have to move from focusing on the physiological and behavioral effects on single species to how ALAN affects all levels of biodiversity including genotypes, communities, ecosystems, and landscapes, including direct and indirect interactions within and among those levels. The different natural light cycles, their role in shaping biodiversity, and their interaction with ALAN impacts needs more attention. Furthermore, ALAN should no longer be considered in isolation from other global change drivers but rather be addressed in a multiple stressor framework where sufficient knowledge of singular impacts allows. The same is true for considering multiple realms (e.g., aerial, aquatic, and terrestrial) and their interactions. This requires that we advance our techniques for quantifying spectral, spatial, and temporal ALAN patterns at multiple scales. Finally, it is not enough to only report that ALAN negatively impacts airborne, aquatic, and terrestrial organisms and ecosystems. More socio-ecological research needs to be directed toward understanding the cultural, political, economic, and institutional barriers that prevent implementation of mitigation measures and toward testing whether and how ALAN can be regulated and light pollution abated effectively.
We conclude that to further develop effective conservation measures aimed at reducing ALAN-induced biodiversity loss, a variety of challenges need to be addressed, ranging from broadening disciplinary perspectives (e.g., from individual species to communities) to strengthening transdisciplinary approaches and ultimately protecting species, ecosystems, and landscapes through effective conservation measures. For now, the information available to inform mitigation strategies remains modest, and as such the precautionary principle should be adopted as the basis for management recommendations while we answer the open questions identified in this study.
FH and EK contributed to the conception, design of the article, and wrote the first draft of the manuscript. FH, AJ, and EK produced the figures. All authors contributed manuscript text, manuscript revisions, and approved the final version.
The contributions of GK, AJ, and FH were supported by the projects “species protection through environmental friendly lighting” by the German Federal Agency for Nature Conservation (BfN) within the framework of the Federal Programme for Biological Diversity with funds from the Federal Ministry for the Environment, Nature Conservation and Nuclear Safety (BMU; FKZ: 3518685A08) and “Beleuchtungsplanung: Verfahren und Methoden für eine naturschutzfreundliche Beleuchtungsgestaltung” by the BfN with funds from the BMU (FKZ: 3521 84 1000). The contributions of ST and TD were supported by the Natural Environment Research Council (grant no. NE/S003533/2 awarded to TD. The publication of this article was funded by the Open Access Publishing funds of the Leibniz Association and Agroscope.
The authors declare that the research was conducted in the absence of any commercial or financial relationships that could be construed as a potential conflict of interest.
All claims expressed in this article are solely those of the authors and do not necessarily represent those of their affiliated organizations, or those of the publisher, the editors and the reviewers. Any product that may be evaluated in this article, or claim that may be made by its manufacturer, is not guaranteed or endorsed by the publisher.
We would like to thank Catherine Pérez Vega who edited Figures 1, 2.
Aceituno, J. Sánchez, S. F., Aceituno, F. J. Galadí-Enr quez, D., Negro, J. J., Soriguer, R. C., et al. (2011). An all-sky transmission monitor: ASTMON. Publ. Astron. Soc. Pac. 123, 1076–1086.
Alamús, R., Bará, S., Corbera, J., Escofet, J., Palà, V., Pipia, L., et al. (2017). Ground-based hyperspectral analysis of the urban nightscape. ISPRS J. Photogr. Rem. Sens. 124, 16–26. doi: 10.1016/j.isprsjprs.2016.12.004
Altermatt, F., and Ebert, D. (2016). Reduced flight-to-light behaviour of moth populations exposed to long-term urban light pollution. Biol. Lett. 12:20160111. doi: 10.1098/rsbl.2016.0111
Aubé, M. (2015). Physical behaviour of anthropogenic light propagation into the nocturnal environment. Philos. Trans. R. Soc. B 370:20140117. doi: 10.1098/rstb.2014.0117
Ayalon, I., Rosenberg, Y., Benichou, J. I., Campos, C. L. D., Sayco, S. L. G., Nada, M. A. L., et al. (2021). Coral gametogenesis collapse under artificial light pollution. Curr. Biol. 31, 413–419. doi: 10.1016/j.cub.2020.10.039
Barentine, J. C. (2020). Who speaks for the night? The regulation of light pollution in the ‘Rights of Nature’ legal framework. Int. J. Sust. Light. 22, 28–36. doi: 10.26607/ijsl.v22i2.104
Barentine, J. C., Walczak, K., Gyuk, G., Tarr, C., and Longcore, T. (2021). A case for a new satellite mission for remote sensing of night lights. Rem. Sens. 13:2294. doi: 10.3390/rs13122294
Barnosky, A. D., Matzke, N., Tomiya, S., Wogan, G. O., Swartz, B., Quental, T. B., et al. (2011). Has the Earth’s sixth mass extinction already arrived? Nature 471, 51–57. doi: 10.1038/nature09678
Bennie, J., Davies, T. W., Cruse, D., Bell, F., and Gaston, K. J. (2018). Artificial light at night alters grassland vegetation species composition and phenology. J. Appl. Ecol. 55, 442–450. doi: 10.1111/1365-2664.12927
Bird, T. J., Bates, A. E., Lefcheck, J. S., Hill, N. A., Thomson, R. J., Edgar, G. J., et al. (2014). Statistical solutions for error and bias in global citizen science datasets. Biol. Cons. 173, 144–154. doi: 10.1016/j.biocon.2013.07.037
Birk, S., Chapman, D., Carvalho, L., Spears, B. M., Andersen, H. E., Argillier, C., et al. (2020). Impacts of multiple stressors on freshwater biota across spatial scales and ecosystems. Nat. Ecol. Evol. 4, 1060–1068. doi: 10.1038/s41559-020-1216-4
Bolliger, J., Hennet, T., Wermelinger, B., Bösch, R., Pazur, R., Blum, S., et al. (2020). Effects of traffic-regulated street lighting on nocturnal insect abundance and bat activity. Basic Appl. Ecol. 47, 44–56. doi: 10.1016/j.envpol.2021.117765
Borer, E. T., Harpole, W. S., Adler, P. B., Lind, E. M., Orrock, J. L., Seabloom, E. W., et al. (2014). Finding generality in ecology: a model for globally distributed experiments. Methods Ecol. Evol. 5, 65–73.
Bouroussis, C. A., and Topalis, F. V. (2020). Assessment of outdoor lighting installations and their impact on light pollution using unmanned aircraft systems–the concept of the drone-gonio-photometer. J. Quant. Spectrosc. Radiat. Transf. 253:107155.
Bradshaw, W. E., and Holzapfel, C. M. (2001). Genetic shift in photoperiodic response correlated with global warming. Proc. Natl. Acad. Sci. U.S.A. 98, 14509–14511.
Brüning, A., Hölker, F., Franke, S., Kleiner, W., and Kloas, W. (2016). Impact of different colours of artificial light at night on melatonin rhythm and gene expression of gonadotropins in European perch. Sci. Total Environ. 543, 214–222. doi: 10.1016/j.scitotenv.2015.11.023
Brüning, A., Hölker, F., Franke, S., Preuer, T., and Kloas, W. (2015). Spotlight on fish: light pollution affects circadian rhythms of European perch but does not cause stress. Sci. Total Environ. 511, 516–522. doi: 10.1016/j.scitotenv.2014.12.094
Camacho, L. F., Barragán, G., and Espinosa, S. (2021). Local ecological knowledge reveals combined landscape effects of light pollution, habitat loss, and fragmentation on insect populations. Biol. Conserv. 262:109311.
Caplat, P., Edelaar, P., Dudaniec, R. Y., Green, A. J., Okamura, B., Cote, J., et al. (2016). Looking beyond the mountain: dispersal barriers in a changing world. Front. Ecol. Environ. 14:261–268. doi: 10.1002/fee.1280
Cardiel, N., Zamorano, J. Bará, S., Sánchez de Miguel, A., Cabello, C., Gallego, J., et al. (2021). Synthetic RGB photometry of bright stars: definition of the standard photometric system and UCM library of spectrophotometric spectra. Mon. Not. R. Astron. Soc. 504, 3730–3748.
Challéat, S., Barré, K., Laforge, A., Lapostolle, D., Franchomme, M., Sirami, C., et al. (2021). Grasping darkness: the dark ecological network as a social-ecological framework to limit the impacts of light pollution on biodiversity. Ecol. Soc. 26:15. doi: 10.5751/ES-12156-260115
Charmantier, A., and Gienapp, P. (2014). Climate change and timing of avian breeding and migration: evolutionary versus plastic changes. Evol. Appl. 7, 15–28. doi: 10.1111/eva.12126
Colantonio, C., Pelosi, C. D’Alessandro, L., and Sottile, S. Calabrò, G., and Melis, M. (2018). Hypercolorimetric multispectral imaging system for cultural heritage diagnostics: an innovative study for copper painting examination. Eur. Phys. J. Plus 133:526.
Cox, D. T. C., Gardner, A. S., and Gaston, K. J. (2021). Diel niche variation in mammals associated with expanded trait space. Nat. Commun. 12:1753. doi: 10.1038/s41467-021-22023-4
Cravens, Z. M., Brown, V. A., Divoll, T. J., and Boyles, J. G. (2018). Illuminating prey selection in an insectivorous bat community exposed to artificial light at night. J. Appl. Ecol. 55, 705–713.
Da Silva, A., and Kempenaers, B. (2017). Singing from North to South: latitudinal variation in timing of dawn singing under natural and artificial light conditions. J. Anim. Ecol. 86, 1286–1297.
Dacke, M., Nilsson, D. E., Scholtz, C. H., Byrne, M., and Warrant, E. J. (2003). Animal behaviour: insect orientation to polarized moonlight. Nature 424:33.
Davies, T. W., Bennie, J., Cruse, D., Blumgart, D., Inger, R., and Gaston, K. J. (2017). Multiple night-time light-emitting diode lighting strategies impact grassland invertebrate assemblages. Glob. Change Biol. 23, 2641–2648. doi: 10.1111/gcb.13615
Davies, T. W., Bennie, J., Inger, R., de Ibarra, N. H., and Gaston, K. J. (2013). Artificial light pollution: are shifting spectral signatures changing the balance of species interactions? Glob. Change Biol. 19, 1417–1423. doi: 10.1111/gcb.12166
Davies, T. W., McKee, D., Fishwick, J., Tidau, S., and Smyth, T. (2020). Biologically important artificial light at night on the seafloor. Sci. Rep. 10:12545. doi: 10.1038/s41598-020-69461-6
Davies, T. W., and Smyth, T. (2018). Why artificial light at night should be a focus for global change research in the 21st century. Glob. Change Biol. 24, 872–882. doi: 10.1111/gcb.13927
Degen, T., Mitesser, O., Perkin, E. K. Weiß, N. S., Oehlert, M., Mattig, E., et al. (2016). Street lighting: sex-independent impacts on moth movement. J. Anim. Ecol. 85, 1352–1360.
de Jong, M., Jeninga, L., Ouyang, J. Q., van Oers, K., Spoelstra, K., and Visser, M. E. (2016). Dose-dependent responses of avian daily rhythms to artificial light at night. Physiol. Behav. 155, 172–179. doi: 10.1016/j.physbeh.2015.12.012
Deichmann, J. L., Ampudia Gatty, C., Andía Navarro, J. M., Alonso, A., Linares-Palomino, R., and Longcore, T. (2021). Reducing the blue spectrum of artificial light at night minimises insect attraction in a tropical lowland forest. Insect Conserv. Divers 14, 247–259. doi: 10.1111/icad.12479
Díaz, S., Zafra-Calvo, N., Purvis, A., Verburg, P. H., Obura, D., Leadley, P., et al. (2020). Set ambitious goals for biodiversity and sustainability. Science 370, 411–413.
Ditmer, M. A., Stoner, D. C., Francis, C. D., Barber, J. R., Forester, J. D., Choate, D. M., et al. (2021). Artificial nightlight alters the predator–prey dynamics of an apex carnivore. Ecography 44, 149–161.
Dominoni, D. M., Halfwerk, W., Baird, E., Buxton, R. T., Fernández-Juricic, E., Fristrup, K. M., et al. (2020a). Why conservation biology can benefit from sensory ecology. Nat. Ecol. Evol. 4, 502–511. doi: 10.1038/s41559-020-1135-4
Dominoni, D. M., Jensen, J. K., de Jong, M., Visser, M. E., and Spoelstra, K. (2020c). Artificial light at night, in interaction with spring temperature, modulates timing of reproduction in a passerine bird. Ecol. Appl. 30, e02062. doi: 10.1002/eap.2062
Dominoni, D. M., Smit, J. A. H., Visser, M. E., and Halfwerk, W. (2020b). Multisensory pollution: artificial light at night and noise have interactive effects on activity patterns of a songbird. Environ. Pollut. 256:113314. doi: 10.1016/j.envpol.2019.113314
Donners, M., van Grunsven, R. H., Groenendijk, D., van Langevelde, F., Bikker, J. W., Longcore, T., et al. (2018). Colors of attraction: modeling insect flight to light behavior. J. Exp. Zool. A 329, 434–440.
Falchi, F., Cinzano, P., Duriscoe, D., Kyba, C. C., Elvidge, C. D., Baugh, K., et al. (2016). The new world atlas of artificial night sky brightness. Sci. Adv. 2:e1600377.
Falcon, J., Torriglia, A., Attia, D., Viénot, F., Gronfier, C., Behar-Cohen, F., et al. (2020). Exposure to artificial light at night and the consequences for flora, fauna, and ecosystems. Front. Neurosci. 14:1183. doi: 10.3389/fnins.2020.602796
Ffrench-Constant, R. H., Somers-Yeates, R., Bennie, J., Economou, T., Hodgson, D., Spalding, A., et al. (2016). Light pollution is associated with earlier tree budburst across the United Kingdom. Proc. R. Soc. B 283:20160813. doi: 10.1098/rspb.2016.0813
Fiorentin, P., Bertolo, A., Cavazzani, S., and Ortolani, S. (2020). Calibration of digital compact cameras for sky quality measures. J. Quant. Spectrosc. Radiat. Transf. 255:107235. doi: 10.1016/j.jqsrt.2020.107235
Foster, J. J., Smolka, J., Nilsson, D. E., and Dacke, M. (2018). How animals follow the stars. Proc. R. Soc. B 285:20172322.
Foster, J. J., Tocco, C., Smolka, J., Khaldy, L., Baird, E., Byrne, M. J., et al. (2021). Light pollution forces a change in dung beetle orientation behavior. Curr. Biol. 31, 3935–3942.
Franzén, M., Betzholtz, P. E., Pettersson, L. B., and Forsman, A. (2020). Urban moth communities suggest that life in the city favours thermophilic multi-dimensional generalists. Proc. R. Soc. B 287:20193014. doi: 10.1098/rspb.2019.3014
Gaston, K. J. (2019). Nighttime ecology: the “nocturnal problem” revisited. Am. Nat. 193, 481–502. doi: 10.1086/702250
Gaston, K. J., Davies, T. W., Bennie, J., and Hopkins, J. (2012). Reducing the ecological consequences of night-time light pollution: options and developments. J. Appl. Ecol. 49, 1256–1266.
Gaston, K. J., Ackermann, S., Bennie, J., Cox, D. T., Phillips, B. B., de Miguel, S., et al. (2021). Pervasiveness of biological impacts of artificial light at night. Int. Comp. Biol. 61, 1098–1110. doi: 10.1093/icb/icab145
Gaston, K. J., Bennie, J., Davies, T. W., and Hopkins, J. (2013). The ecological impacts of nighttime light pollution: a mechanistic appraisal. Biol. Rev. Camb. Philos. Soc. 88, 912–927. doi: 10.1111/brv.12036
Gaston, K. J., Visser, M. E., and Hölker, F. (2015). The biological impacts of artificial light at night: the research challenge. Philos. Trans. R. Soc. B 370:20140133.
Geldmann, J., Heilmann-Clausen, J., Holm, T. E., Levinsky, I., Markussen, B., Olsen, K., et al. (2016). What determines spatial bias in citizen science? Exploring four recording schemes with different proficiency requirements. Divers. Distrib. 22, 1139–1149.
Giavi, S., Blösch, S., Schuster, G., and Knop, E. (2020). Artificial light at night can modify ecosystem functioning beyond the lit area. Sci. Rep. 10:11870. doi: 10.1038/s41598-020-68667-y
Giavi, S., Fontaine, C., and Knop, E. (2021). Impact of artificial light at night on diurnal plant-pollinator interactions. Nat. Commun. 12:1690. doi: 10.1038/s41467-021-22011-8
Grubisic, M., Haim, A., Bhusal, P., Dominoni, D. M., Gabriel, K., Jechow, A., et al. (2019). Light pollution, circadian photoreception, and melatonin in vertebrates. Sustainability 11:6400. doi: 10.3390/su11226400
Grubisic, M., van Grunsven, R. H., Kyba, C. C. M., Manfrin, A., and Hölker, F. (2018). Insect declines and agroecosystems: does light pollution matter? Ann. Appl. Biol. 173, 180–189.
Halfwerk, W., and Slabbekoorn, H. (2015). Pollution going multimodal: the complex impact of the human-altered sensory environment on animal perception and performance. Biol. Lett. 11:20141051. doi: 10.1098/rsbl.2014.1051
Hänel, A., Posch, T., Ribas, S. J., Aubé, M., Duriscoe, D., Jechow, A., et al. (2018). Measuring night sky brightness: methods and challenges. J. Quant. Spectrosc. Radiat. Transf. 205, 278–290.
Haynes, K. J., and Robertson, B. A. (2021). A transdisciplinary research agenda for understanding insect responses to ecological light pollution informed by evolutionary trap theory. Curr. Opin. Insect Sci. 45, 91–96. doi: 10.1016/j.cois.2021.02.004
Hölker, F., Moss, T., Griefahn, B., Kloas, W., Voigt, C. C., Henckel, D., et al. (2010a). The dark side of light: a transdisciplinary research agenda for light pollution policy. Ecol. Soc. 15:13.
Hölker, F., Wolter, C., Perkin, E. K., and Tockner, K. (2010b). Light pollution as a biodiversity threat. Trends Ecol. Evol. 25, 681–682. doi: 10.1016/j.tree.2010.09.007
Hölker, F., Wurzbacher, C., Weißenborn, C., Monaghan, M. T., Holzhauer, S. I., and Premke, K. (2015). Microbial diversity and community respiration in freshwater sediments influenced by artificial light at night. Philos. Trans. R. Soc. B 370:20140130. doi: 10.1098/rstb.2014.0130
Hopkins, G. R., Gaston, K. J., Visser, M. E., Elgar, M. A., and Jones, T. M. (2018). Artificial light at night as a driver of evolution across urban rural landscapes. Front. Ecol. Environ. 16:472–479. doi: 10.1002/fee.1828
Horváth, G., Kriska, G., Malik, P., and Robertson, B. (2009). Polarized light pollution: a new kind of ecological photopollution. Front. Ecol. Environ. 7:317–325. doi: 10.1890/080129
Hut, R. A., Paolucci, S., Dor, R., Kyriacou, C. P., and Daan, S. (2013). Latitudinal clines: an evolutionary view on biological rhythms. Proc. R. Soc. B 280:20130433. doi: 10.1098/rspb.2013.0433
IPBES (2019). “Summary for policymakers of the global assessment report on biodiversity and ecosystem services of the intergovernmental science-policy platform on biodiversity and ecosystem services,” in Intergovernmental Science-Policy Platform on Biodiversity and Ecosystem Services, ed. J. Díaz (Bonn: IPBES Secretariat).
Jackson, M. C., Loewen, C. J. G., Vinebrooke, R. D., and Chimimba, C. T. (2016). Net effects of multiple stressors in freshwater ecosystems: a meta-analysis. Glob. Change Biol. 22, 180–189.
Jägerbrand, A. K., and Bouroussis, C. A. (2021). Ecological impact of artificial light at night: effective strategies and measures to deal with protected species and habitats. Sustainability 13:5991. doi: 10.3390/su13115991
Jakle, J. A., and Thompson, G. F. (2001). City Lights: Illuminating the American Night. Baltimore, MD: Johns Hopkins University Press.
Jechow, A., and Hölker, F. (2019a). How dark is a river? Artificial light at night in aquatic systems and the need for comprehensive night-time light measurements. WIREs Water 6:e1388. doi: 10.1002/wat2.1388
Jechow, A., and Hölker, F. (2019b). Snowglow–the amplification of skyglow by snow and clouds can exceed full moon illuminance in suburban areas. J. Imaging 5:69. doi: 10.3390/jimaging5080069
Jechow, A., Kyba, C. C. M., and Hölker, F. (2019). Beyond all-sky: assessing ecological light pollution using multi-spectral full-sphere fisheye lens imaging. J. Imaging 5:46. doi: 10.3390/jimaging5040046
Jechow, A., Kyba, C. C. M., and Hölker, F. (2020). Mapping the brightness and color of urban to rural skyglow with all-sky photometry. J. Quant. Spectrosc. Radiat. Transf. 250:106988. doi: 10.1016/j.jqsrt.2020.106988
Jechow, A., Ribas, S. J., Domingo, R. C., Hölker, F., Kolláth, Z., and Kyba, C. C. M. (2018). Tracking the dynamics of skyglow with differential photometry using a digital camera with fisheye lens. J. Quant. Spectrosc. Radiat. Transf. 209, 212–223.
Kalinkat, G., Grubisic, M., Jechow, A., van Grunsven, R. H., Schroer, S., and Hölker, F. (2021). Assessing long-term effects of artificial light at night on insects: what is missing and how to get there. Insect Conserv. Divers. 14, 260–270. doi: 10.1111/icad.12482
Keinath, S., Hölker, F., Müller, J., and Rödel, M. O. (2021). Impact of light pollution on moth morphology–a 137-year study in Germany. Basic Appl. Ecol. 56, 1–10. doi: 10.1016/j.baae.2021.05.004
Knop, E., Zoller, L., Ryser, R., Gerpe, C., Hörler, M., and Fontaine, C. (2017). Artificial light at night as a new threat to pollination. Nature 548, 206–209. doi: 10.1038/nature23288
Kocifaj, M. Kómar, L., and Kundracik, F. (2018). PePSS-A portable sky scanner for measuring extremely low night-sky brightness.J. Quant. Spectr. Rad. Transf. 210, 74–81.
Kolláth, Z., Cool, A., Jechow, A., Kolláth, K., Száz, D., and Tong, K. P. (2020). Introducing the Dark Sky Unit for multi-spectral measurement of the night sky quality with commercial digital cameras. J. Quant. Spectrosc. Radiat. Transf. 253:107162. doi: 10.1016/j.jqsrt.2020.107162
Kühne, J. L., van Grunsven, R. H. A., Jechow, A., and Hölker, F. (2021). Impact of different wavelengths of artificial light at night on phototaxis in aquatic insects. Int. Comp. Biol. 61, 1182–1190. doi: 10.1093/icb/icab149
Kupprat, F., Hölker, F., and Kloas, W. (2020). Can skyglow reduce nocturnal melatonin concentrations in Eurasian perch? Environ. Pollut. 262:114324. doi: 10.1016/j.envpol.2020.114324
Kyba, C., Pritchard, S. B., Ekirch, A. R., Eldridge, A., Jechow, A., Preiser, C., et al. (2020). Night matters—why the interdisciplinary field of “Night Studies” is needed. J. 3, 1–6. doi: 10.3390/j3010001
Kyba, C. C., Kuester, T., De Miguel, A. S., Baugh, K., Jechow, A., Hölker, F., et al. (2017). Artificially lit surface of Earth at night increasing in radiance and extent. Sci. Adv. 3:e1701528. doi: 10.1126/sciadv.1701528
Kyba, C. C. M., Hänel, A., and Hölker, F. (2014). Redefining efficiency for outdoor lighting. Energ. Environm. Sci. 7, 1806–1809.
Kyba, C. C. M., and Hölker, F. (2013). Do artificially illuminated skies affect biodiversity in nocturnal landscapes? Landsc. Ecol. 28, 1637–1640.
Kyba, C. C. M., Ruby, A., Kuechly, H. U., Kinzey, B., Miller, N., Sanders, J., et al. (2021). Direct measurement of the contribution of street lighting to satellite observations of nighttime light emissions from urban areas. Light Res. Technol. 53, 189–211.
Kyba, C. C. M., Ruhtz, T., Fischer, J., and Hölker, F. (2011). Cloud coverage acts as an amplifier for ecological light pollution in urban ecosystems. PLoS One 6:e17307. doi: 10.1371/journal.pone.0017307
Kyba, C. C. M., Ruhtz, T., Fischer, J., and Hölker, F. (2012). Red is the new black: how the colour of urban skyglow varies with cloud cover. Mon. Not. R. Astron. Soc. 425, 701–708.
Laforge, A., Pauwels, J., Faure, B., Bas, Y., Kerbiriou, C., Fonderflick, J., et al. (2019). Reducing light pollution improves connectivity for bats in urban landscapes. Landsc. Ecol. 34, 793–809. doi: 10.1007/s10980-019-00803-0
Last, K. S., Hobbs, L., Berge, J., Brierley, A. S., and Cottier, F. (2016). Moonlight drives ocean-scale mass vertical migration of zooplankton during the Arctic winter. Curr. Biol. 26, 244–251. doi: 10.1016/j.cub.2015.11.038
Levin, N., Kyba, C. C. M., Zhang, Q., Román, M. O., Li, X., Portnov, B. A., et al. (2020). Remote sensing of night lights: a review and an outlook for the future. Rem. Sens. Environ. 237:111443. doi: 10.1016/j.rse.2019.111443
Lewanzik, D., and Voigt, C. C. (2014). Artificial light puts ecosystem services of frugivorous bats at risk. J. Appl. Ecol. 51, 388–394. doi: 10.1111/1365-2664.12206
Longcore, T., Duriscoe, D., Aubé, M., Jechow, A., Kyba, C., and Pendoley, K. L. (2020). Commentary: brightness of the night sky affects loggerhead (Caretta caretta) sea turtle hatchling misorientation but not nest site selection. Front. Mar. Sci. 7:706. doi: 10.3389/fmars.2020.00706
Longcore, T., Rodríguez, A., Witherington, B., Penniman, J. F., Herf, L., and Herf, M. (2018). Rapid assessment of lamp spectrum to quantify ecological effects of light at night. J. Exp. Zool. A 329, 511–521. doi: 10.1002/jez.2184
Macgregor, C. J., Evans, D. M., Fox, R., and Pocock, M. J. (2017). The dark side of street lighting: impacts on moths and evidence for the disruption of nocturnal pollen transport. Glob. Change Biol. 23, 697–707. doi: 10.1111/gcb.13371
Maggi, E., Bongiorni, L., Fontanini, D., Capocchi, A., Dal Bello, M., and Giacomelli, A. (2020). Artificial light at night erases positive interactions across trophic levels. Funct. Ecol. 34, 694–706. doi: 10.1111/1365-2435.13485
Manfrin, A., Lehmann, D., van Grunsven, R. H., Larsen, S., Syväranta, J., Wharton, G., et al. (2018). Dietary changes in predators and scavengers in a nocturnally illuminated riparian ecosystem. Oikos 127, 960–969.
Manfrin, A., Singer, G., Larsen, S., Weiß, N., van Grunsven, R. H., Weiß, N. S., et al. (2017). Artificial light at night affects organism flux across ecosystem boundaries and drives community structure in the recipient ecosystem. Front. Env. Sci. 5:61. doi: 10.3389/fenvs.2017.00061
Manríquez, P. H., Jara, M. E., Diaz, M. I., Quijón, P. A., Widdicombe, S., Pulgar, J., et al. (2019). Artificial light pollution influences behavioral and physiological traits in a keystone predator species, Concholepas concholepas. Sci. Total Env. 661, 543–552. doi: 10.1016/j.scitotenv.2019.01.157
Marchant, P., Hale, J. D., and Sadler, J. P. (2020). Does changing to brighter road lighting improve road safety? Multilevel longitudinal analysis of road traffic collision frequency during the relighting of a UK city. J. Epidemiol. Community Health 74, 467–472. doi: 10.1136/jech-2019-212208
McLaren, J. D., Buler, J. J., Schreckengost, T., Smolinsky, J. A., Boone, M., van Loon, E., et al. (2018). Artificial light at night confounds broad-scale habitat use by migrating birds. Ecol. Lett. 21, 356–364. doi: 10.1111/ele.12902
McMahon, T. A., Rohr, J. R., and Bernal, X. E. (2017). Light and noise pollution interact to disrupt interspecific interactions. Ecology 98, 1290–1299. doi: 10.1002/ecy.1770
Miller, C. R., Barton, B. T., Zhu, L., Radeloff, V. C., Oliver, K. M., Harmon, J. P., et al. (2017). Combined effects of night warming and light pollution on predator–prey interactions. Proc. R. Soc. B 284:20171195. doi: 10.1098/rspb.2017.1195
Moore, M. V., Pierce, S. M., Walsh, H. M., Kvalvik, S. K., and Lim, J. D. (2000). Urban light pollution alters the diel vertical migration of Daphnia. Verh. lnternat. Verein. Limnol. 27, 779–782.
Nilsson, D. E., and Smolka, J. (2021). Quantifying biologically essential aspects of environmental light. J. R. Soc. Interface 18:20210184.
Nuñez, J. D., Sbragaglia, V., Spivak, E. D., Chiaradia, N. M., and Luppi, T. A. (2021). The magnitude of behavioural responses to artificial light at night depends on the ecological context in a coastal marine ecosystem engineer. Mar. Env. Res. 165:105238. doi: 10.1016/j.marenvres.2020.105238
Owens, A. C. S., and Lewis, S. M. (2021). Narrow-spectrum artificial light silences female fireflies (Coleoptera: Lampyridae). Insect. Conserv. Divers. 199, 199–210. doi: 10.1111/icad.12487
Patat, F. (2008). The dancing sky: 6 years of night-sky observations at Cerro Paranal. Astron. Astrophys. 481, 575–591.
Pérez Vega, C., Zielinska-Dabkowska, K. M., and Hölker, F. (2021). Urban lighting research transdisciplinary framework—a collaborative process with lighting professionals. Int. J. Environ. Res. Public Health 18:624. doi: 10.3390/ijerph18020624
Perkin, E. K., Hölker, F., Richardson, J. S., Sadler, J. P., Wolter, C., and Tockner, K. (2011). The influence of artificial light on stream and riparian ecosystems: questions, challenges, and perspectives. Ecosphere 2, 1–16. doi: 10.1890/ES11-00241.1
Rillig, M. C., Ryo, M., Lehmann, A., Aguilar-Trigueros, C. A., Buchert, S., Wulf, A., et al. (2019). The role of multiple global change factors in driving soil functions and microbial biodiversity. Science 366, 886–890. doi: 10.1126/science.aay2832
Sage, R. F. (2020). Global change biology: a primer. Glob. Change Biol. 26, 3–30. doi: 10.1111/gcb.14893
Sánchez de Miguel, A., Aubé, M., Zamorano, J., Kocifaj, M., Roby, J., and Tapia, C. (2017). Sky Quality Meter measurements in a colour-changing world. Mon. Not. R. Astron. Soc. 467, 2966–2979.
Sánchez de Miguel, A., Zamorano, J., Aubé, M., Bennie, J., Gallego, J., Ocaña, F., et al. (2021). Colour remote sensing of the impact of artificial light at night (II): calibration of DSLR-based images from the International Space Station. Rem. Sens. Environ. 264:112611. doi: 10.1016/j.rse.2021.112611
Sanders, D., Frago, E., Kehoe, R., Patterson, C., and Gaston, K. J. (2021). A meta-analysis of biological impacts of artificial light at night. Nat. Ecol. Evol. 5, 74–81. doi: 10.1038/s41559-020-01322-x
Sanders, D., and Gaston, K. J. (2018). How ecological communities respond to artificial light at night. J. Exp. Zool. A 329, 394–400. doi: 10.1002/jez.2157
Sanders, D., Kehoe, R., Cruse, D., van Veen, F. F., and Gaston, K. J. (2018). Low levels of artificial light at night strengthen top-down control in insect food web. Curr. Biol. 28, 2474–2478. doi: 10.1016/j.cub.2018.05.078
Sanders, D., Kehoe, R., Tiley, K., Bennie, J., Cruse, D., Davies, T. W., et al. (2015). Artificial nighttime light changes aphid-parasitoid population dynamics. Sci. Rep. 5, 15232–15232. doi: 10.1038/srep15232
Schroer, S.Hölker, F. (2017). “Light pollution reduction,” in Handbook of Advanced Lighting Technology, eds R. Karlicek, C. C. Sun, G. Zissis, and R. Ma (Cham: Springer International Publishing), 991–1010.
Schroer, S., Huggins, B. J., Azam, C., and Hölker, F. (2020). Working with inadequate tools: legislative shortcomings in protection against ecological effects of artificial light at night. Sustainability 12:2551. doi: 10.3390/su12062551
Schulte-Römer, N., Meier, J., Dannemann, E., and Söding, M. (2019). Lighting professionals versus light pollution experts? Investigating views on an emerging environmental concern. Sustainability 11:1696. doi: 10.3390/su11061696
Secondi, J., Davranche, A., Théry, M., Mondy, N., and Lengagne, T. (2020). Assessing the effects of artificial light at night on biodiversity across latitude–current knowledge gaps. Glob. Ecol. Biogeogr. 29, 404–419. doi: 10.1111/geb.13037
Senzaki, M., Barber, J. R., Phillips, J. N., Carter, N. H., Cooper, C. B., Ditmer, M. A., et al. (2020). Sensory pollutants alter bird phenology and fitness across a continent. Nature 587, 605–609. doi: 10.1038/s41586-020-2903-7
Seymoure, B. M., Linares, C., and White, J. (2019). Connecting spectral radiometry of anthropogenic light sources to the visual ecology of organisms. J. Zool. 308, 93–110.
Shiwen, L., Steel, L., Dahlsjö, C. A., Peirson, S. N., Shenkin, A., Morimoto, T., et al. (2021). Hyperspectral characterisation of natural illumination in woodland and forest environments. SPIE J. 11815, 1181506–1181511. doi: 10.1111/gcb.14903
Sih, A., Ferrari, M. C., and Harris, D. J. (2011). Evolution and behavioural responses to human-induced rapid environmental change. Evol. Appl. 4, 367–387. doi: 10.1111/j.1752-4571.2010.00166.x
Simons, A. L., Yin, X., and Longcore, T. (2020). High correlation but high scale-dependent variance between satellite measured night lights and terrestrial exposure. Environ. Res. Commun. 2:021006.
Spitschan, M., Aguirre, G. K., Brainard, D. H., and Sweeney, A. M. (2016). Variation of outdoor illumination as a function of solar elevation and light pollution. Sci. Rep. 6:26756. doi: 10.1038/srep26756
Spoelstra, K., van Grunsven, R. H., Donners, M., Gienapp, P., Huigens, M. E., Slaterus, R., et al. (2015). Experimental illumination of natural habitat—an experimental set-up to assess the direct and indirect ecological consequences of artificial light of different spectral composition. Philos. Trans. R. Soc A 370:20140129. doi: 10.1098/rstb.2014.0129
Swaddle, J. P., Francis, C. D., Barber, J. R., Cooper, C. B., Kyba, C. C., Dominoni, D. M., et al. (2015). A framework to assess evolutionary responses to anthropogenic light and sound. Trends Ecol. Evol. 30, 550–560. doi: 10.1016/j.tree.2015.06.009
Thums, M., Whiting, S. D., Reisser, J., Pendoley, K. L., Pattiaratchi, C. B., Proietti, M., et al. (2016). Artificial light on water attracts turtle hatchlings during their near shore transit. R. Soc. Open Sci. 35:160142. doi: 10.1098/rsos.160142
Tidau, S., Smyth, T., McKee, D., Wiedenmann, J., D’Angelo, C., Wilcockson, D., et al. (2021). Marine artificial light at night: an empirical and technical guide. Methods Ecol. Evol. 12, 1588–1601. doi: 10.1111/2041-210X.13653
Torres, D., Tidau, S., Jenkins, S., and Davies, T. (2020). Artificial skyglow disrupts celestial migration at night. Curr. Biol. 30, R696–R697.
Tuomainen, U., and Candolin, U. (2011). Behavioural responses to human-induced environmental change. Biol Rev. 86, 640–657. doi: 10.1111/j.1469-185x.2010.00164.x
Tylianakis, J. M., Didham, R. K., Bascompte, J., and Wardle, D. A. (2008). Global change and species interactions in terrestrial ecosystems. Ecol. Lett. 11, 1351–1363. doi: 10.1111/j.1461-0248.2008.01250.x
Ugolini, A., Fantini, T., and Innocenti, R. (2003). Orientation at night: an innate moon compass in sandhoppers (Amphipoda: Talitridae). Proc. Biol. Sci. 270, 279–281. doi: 10.1098/rspb.2002.2221
Van Asch, M., Van Tienderen, P. H., Holleman, L. J. M., and Visser, M. E. (2007). Predicting adaptation of phenology in response to climate change, an insect herbivore example. Glob. Change Biol. 13, 1596–1604. doi: 10.1111/j.1365-2486.2007.01400.x
van der Kooi, C. J., Stavenga, D. G., Arikawa, K., Belušič, G., and Kelber, A. (2021). Evolution of insect color vision: from spectral sensitivity to visual ecology. Annu. Rev. Entomol. 66, 435–461. doi: 10.1146/annurev-ento-061720-071644
van Grunsven, R. H., Donners, M., Boekee, K., Tichelaar, I., Van Geffen, K. G., Groenendijk, D., et al. (2014). Spectral composition of light sources and insect phototaxis, with an evaluation of existing spectral response models. J. Insect Conserv. 18, 225–231. doi: 10.1007/s10841-014-9633-9
van Grunsven, R. H., Jähnichen, D., Grubisic, M., and Hölker, F. (2018). Slugs (Arionidae) benefit from nocturnal artificial illumination. J. Exp. Zool. A 329, 429–433. doi: 10.1002/jez.2170
van Grunsven, R. H., van Deijk, J. R., Donners, M., Berendse, F., Visser, M. E., Veenendaal, E., et al. (2020). Experimental light at night has a negative long-term impact on macro-moth populations. Curr. Biol. 30, 694–695. doi: 10.1016/j.cub.2020.04.083
Vandenberg, L. N., Colborn, T., Hayes, T. B., Heindel, J. J., Jacobs, D. R. Jr., Lee, D. H., et al. (2012). Hormones and endocrine-disrupting chemicals: low-dose effects and nonmonotonic dose responses. Endocr. Rev. 33, 378–455. doi: 10.1210/er.2011-1050
Voigt, C. C., Dekker, J., Fritze, M., Gazaryan, S., Hölker, F., Jones, G., et al. (2021). The impact of light pollution on bats varies according to foraging guild and habitat context. BioScience 71, 1103–1109.
Wilson, A. A., Ditmer, M. A., Barber, J. R., Carter, N. H., Miller, E. T., Tyrrell, L. P., et al. (2021). Artificial night light and anthropogenic noise interact to influence bird abundance over a continental scale. Glob. Change Biol. 27, 3987–4004. doi: 10.1111/gcb.15663
Wyneken, J., and Salmon, M. (2020). Linking ecology, morphology, and behavior to conservation: lessons learned from studies of sea turtles. Int. Comp. Biol. 60, 440–455. doi: 10.1093/icb/icaa044
Keywords: ecological light pollution, biodiversity loss, thresholds, traits, populations, ecosystems, interdisciplinary, mitigation
Citation: Hölker F, Bolliger J, Davies TW, Giavi S, Jechow A, Kalinkat G, Longcore T, Spoelstra K, Tidau S, Visser ME and Knop E (2021) 11 Pressing Research Questions on How Light Pollution Affects Biodiversity. Front. Ecol. Evol. 9:767177. doi: 10.3389/fevo.2021.767177
Received: 30 August 2021; Accepted: 17 November 2021;
Published: 08 December 2021.
Edited by:
Davide M. Dominoni, University of Glasgow, United KingdomReviewed by:
Clinton D. Francis, California Polytechnic State University, United StatesCopyright © 2021 Hölker, Bolliger, Davies, Giavi, Jechow, Kalinkat, Longcore, Spoelstra, Tidau, Visser and Knop. This is an open-access article distributed under the terms of the Creative Commons Attribution License (CC BY). The use, distribution or reproduction in other forums is permitted, provided the original author(s) and the copyright owner(s) are credited and that the original publication in this journal is cited, in accordance with accepted academic practice. No use, distribution or reproduction is permitted which does not comply with these terms.
*Correspondence: Franz Hölker, aG9lbGtlckBpZ2ItYmVybGluLmRl; Eva Knop, ZXZhLmtub3BAaWV1LnV6aC5jaA==
Disclaimer: All claims expressed in this article are solely those of the authors and do not necessarily represent those of their affiliated organizations, or those of the publisher, the editors and the reviewers. Any product that may be evaluated in this article or claim that may be made by its manufacturer is not guaranteed or endorsed by the publisher.
Research integrity at Frontiers
Learn more about the work of our research integrity team to safeguard the quality of each article we publish.