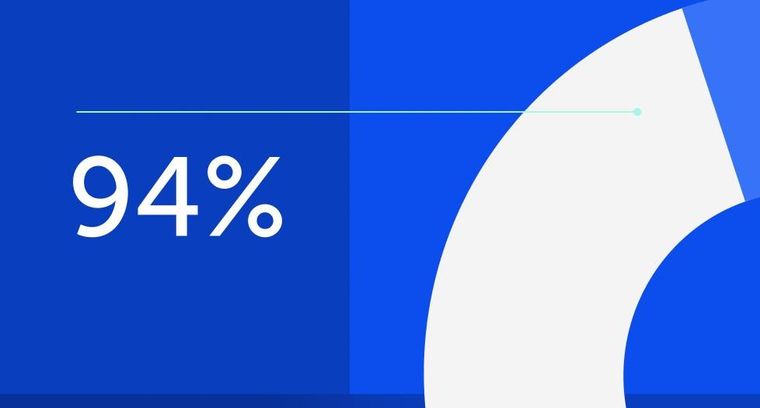
94% of researchers rate our articles as excellent or good
Learn more about the work of our research integrity team to safeguard the quality of each article we publish.
Find out more
PERSPECTIVE article
Front. Ecol. Evol., 25 January 2022
Sec. Behavioral and Evolutionary Ecology
Volume 9 - 2021 | https://doi.org/10.3389/fevo.2021.766725
This article is part of the Research TopicVision in Limited Visibility: Optical Properties, Image Formation, and Evolutionary Adaptations in Attenuating MediaView all 4 articles
Amphibian larvae typically inhabit relatively shallow freshwater environments, and within these boundaries there is considerable diversity in the structure of the habitats exploited by different species. This diversity in habitat structure is usually taken into account in relation to aspects such as locomotion and feeding, and plays a fundamental role in the classification of tadpoles into ecomorphological guilds. However, its impact in shaping the sensory worlds of different species is rarely addressed, including the optical qualities of each of these types of water bodies and the challenges and limitations that they impose on the repertoire of visual abilities available for a typical vertebrate eye. In this Perspective article, we identify gaps in knowledge on (1) the role of turbidity and light-limited environments in shaping the larval visual system; and (2) the possible behavioral and phenotypic responses of larvae to such environments. We also identify relevant unaddressed study systems paying special attention to phytotelmata, whose small size allows for extensive quantification and manipulation providing a rich and relatively unexplored research model. Furthermore, we generate hypotheses ranging from proximate shifts (i.e., red-shifted spectral sensitivity peaks driven by deviations in chromophore ratios) to ultimate changes in tadpole behavior and phenotype, such as reduced foraging efficiency and the loss of antipredator signaling. Overall, amphibians provide an exciting opportunity to understand adaptations to visually limited environments, and this framework will provide novel experimental considerations and interpretations to kickstart future research based on understanding the evolution and diversity of strategies used to cope with limited visibility.
An incredible diversity of animals inhabits aquatic environments for either part or the entirety of their lives. As a medium, water poses unique challenges and opportunities compared to air, which has resulted in distinct physiological adaptations of even temporarily aquatic animals. For example, short-range communication using chemical signals is facilitated in water (Wisenden, 2000), whereas communication using sound in shallow water environments is compromised (Ladich and Winkler, 2017). The visual world underwater is shaped by the way in which light gets refracted and attenuated, and by the inherent optical properties of water (e.g., high absorbance of blue light). In this default scenario, physical variables such as pool type, volume, and geometry, and biological variables, such as the amount and nature of organic matter, interact in shaping the luminic environment (Jerlov, 1976; Lythgoe, 1979). Here, we will use “limited visibility” and “turbidity” in the sense implied throughout Lythgoe’s (1979) “The Ecology of Vision,” as follows. Limited visibility refers to a number of situations in which increased scattering and/or selective absorption alter the quality and quantity of light available for a visual system, including, but not limited to, depth-dependent filtering of spectral bands at the boundaries of the visible spectrum, darkening/coloring due to absorption by dissolved pigments, etc. Turbidity, in this context, is a particular case of limited visibility; its most defining feature is the presence of a “veil” of scattered light originating from suspended particles in the visual scene, and its direct consequence is the decrease of brightness contrast of objects against the background. Thus, even though suspended matter can also absorb light, the main challenge it poses to visual performance is decreased visibility via an increase in “noise” in the visual scene -rather than a decrease in light availability as is the case of other visibility-reduced environments, such as the “deep” or “colored” waters mentioned just above.
Despite the intricate visual systems that aquatic animals have evolved throughout time, individuals can be challenged by the rapid and unexpected change in the structure of their visual environments. Sudden increases in dissolved organic matter through algal blooms, agricultural/anthropogenic run-off, or from resuspended particulate matter can drastically change the visibility of aquatic habitats (Granqvist and Mattila, 2004; Engström-Öst and Candolin, 2006; Chivers et al., 2012). In these situations, the question is no longer about the refinement of an animal’s visual system for a specific habitat, but about the flexibility of that system to maintain image quality and visual performance in the face of change. Research on the plasticity of visual systems so far has mostly dealt with its biochemical and genetic bases (see Corbo, 2021 and Carleton and Yourick, 2020, respectively, for reviews on examples of these topics), whereas focused research on its consequences for ecologically relevant, visually guided behaviors is still missing in the scientific literature. In an era where the stressors of agricultural practices, land use, and climate change are more present than ever before, understanding the responses to turbidity from both proximate and ultimate perspectives will help to better understand the responses of aquatic animals in these increasingly stressed environments (Rowe and Dean, 1998; Sundin et al., 2010).
Tadpoles can rely on vision, at least to some degree, for social behavior (Katz et al., 1981; Caldwell, 1989; Sontag et al., 2006) and complex space use (Beiswenger, 1977). Despite this, they are nearsighted (Mathis et al., 1988), and have typically been assessed as having poor vision overall (Hoff et al., 1999). This mindset has shaped both the experimental design and interpretation of results of studies testing their reliance on different sensory modalities (Rot-Nikcevic et al., 2006; Saidapur et al., 2009). However, other recent work has begun to question this, providing support for a more prominent role of visual cues in predator detection (Hettyey et al., 2012), identification of conspecifics (Gouchie et al., 2008; Kumpulainen, 2021) and habitat assessment (Rot-Nikcevic et al., 2005; Hettyey et al., 2012). For many amphibian species, tadpoles are aquatic and adults are (semi-)terrestrial. While “immature” in the grand scheme of ontogeny, larvae are adapted to their aquatic habitats with the same degree of refinement with which adults are adapted to their environments post-metamorphosis (McDiarmid and Altig, 1999). Such a clearly defined biphasic life cycle, unparalleled among vertebrates, presents a unique opportunity to study the plasticity of visual systems: even within species the visual system can be differentially tuned to match the surrounding environment before and after metamorphosis, as has been shown for relative eye size (Shrimpton et al., 2021) and expression levels of genes that determine spectral sensitivity (Schott et al., 2021).
Overall, amphibian vision is relatively well documented in adults from the point of view of phototransduction mechanisms, spectral tuning, retinal topography and signal processing, and optical performance, but less work has been done on larval stages (see Donner and Yovanovich, 2020 for a review). To the extent to which tadpoles’ visual systems have been studied, they expectedly share the organization and functionality of vertebrates in general, and amphibians in particular, and showcase some differences with their adult selves in aspects that impact performance in aquatic vs. aerial media. For example, tadpoles’ lenses tend to be spherical, while those of terrestrial adults become flattened (Mathis et al., 1988), and their visual pigments tend to be shifted to long wavelengths compared to adults (Crescitelli, 1958), with implications discussed in the upcoming sections. Amphibian larvae constitute a unique model to understand the evolution of vertebrates’ visual systems in the transition from aquatic to aerial environments, and the effect of ontogeny on the maintenance or change of ocular adaptation. Thus, their visual ecology and the behavioral adaptations resulting from environmental changes provide an exciting and relatively unexplored framework of questions to address.
To date, researchers have focused primarily on fishes to understand how predator-prey interactions, mate choice, and foraging adaptations are affected in environments with limited visibility (e.g., turbid). The bulk of these studies show that when challenged with turbidity animals demonstrate a relaxed selection in mate choice (Järvenpää and Lindström, 2004; Sundin et al., 2010), poorer foraging efficiency (Rowe and Dean, 1998; Horppila et al., 2004), and reduced perception of predation risk (Kimbell and Morrell, 2015). Overall, these trends are not without exception, as turbidity can both provide cover to prey while also impairing reaction time/evasive maneuvering, thus the (dis)advantage of turbidity is more generally determined by the modalities used by the community. The majority of turbidity studies have been conducted in North America and Northern Europe, leaving most of the equatorial regions and its diverse (semi-) aquatic fauna understudied. Thus, much of the diversity with respect to species, geography, and life histories, is available for novel comparisons and new insights into animal adaptations in visually challenging environments.
One such environment that has yet to be addressed in studies on limited visibility are phytotelmata. These ephemeral pools of water are formed in plant structures, and distributed throughout the vertical gradient. Phytotelmata, which are notably different from ponds and lakes, commonly occur in tropical regions and are frequently invaded by insects and amphibian tadpoles (Kam et al., 2001; Lehtinen, 2004; Summers and Tumulty, 2013; Biju et al., 2016; Cossio et al., 2021; Fouilloux et al., 2021) and constitute a model system that is relatively easy to access and extensively quantify, and lends itself very well to experimental manipulations (e.g., Serrano-Rojas and Pašukonis, 2021). Occupied pools range widely in size and shape (Fouilloux et al., 2021), and in turbidity and/or water color as well, according to qualitative field observations (unpublished data recorded by CF, Figure 1). Additionally, phytotelmata themselves contribute to defining the light environment in pools. For example, light reflected from fresh green bromeliad leaves will be different from that reflected from decomposing bark or decaying palm bracts. To our knowledge, thus far there have been no quantitative measurements of light or turbidity levels in phytotelmata, which only emphasizes the knowledge gap within this system.
Figure 1. Water samples (collected by CF) from phytotelmata occupied by Dendrobates tinctorius tadpoles in French Guiana. Based on water samples, it appears that tadpoles are deposited in pools with a wide range of visibility. Colored labels refer the water sample (top of figure) to the pool from which it was collected. We hypothesize that in darker waters tadpoles’ spectral sensitivity is red-shifted (lower Vitamin A1:A2 ratio). Photos: Chloe A. Fouilloux.
One could expect that a consistent decrease in light availability (based on denser canopy cover) and visibility would have a measurable impact on larval development. For instance, tadpoles of the Amazonian phytotelm-breeder Dendrobates tinctorius that develop in pools with dark-tinted water appear to be collectively smaller than those deposited in clear waters despite an abundance of prey availability in both cases (e.g., mosquito larvae, BR unpublished obs.). We hypothesize that the decreased body condition of these D. tinctorius larvae may indicate that, in environments with limited visibility, phytotelm-reared tadpoles may experience decreased hunting efficiency, and shift their feeding strategies from omnivorous/predacious to mostly herbivorous. The constraints of turbid environments may not only affect predatory behavior by carnivorous tadpoles, but could also impact other species with specialized feeding strategies like oophagy, which relies on the ritualized parent-offspring feeding behavior initiated after tadpoles visually recognize an adult and increase activity to indicate hunger (Stynoski and Noble, 2012). Ultimately, we hypothesize that turbid or otherwise visually limiting microhabitats could have profound effects on both body condition and survival in a variety of phytotelm-based species (Figure 2).
Figure 2. Summary of the potential effects of turbidity on tadpoles that we hypothesize and elaborate on in the text, at the biochemical/phototransduction level (top left), and on foraging/predation (A) and anti-predation (B) strategies and efficiency.
Turbidity could also have notable effects on phytotelmata communities. In estuary systems, for example, it appears that biodiversity decreases in turbid conditions, where the few species that use chemoreception (crabs) outcompete those that rely more heavily on visual cues (fish; Reustle and Smee, 2020). Diverse tadpoles (Kitching, 2001; Summers and McKeon, 2004), insects (Fincke, 1992; Caldwell, 1993), and crustaceans (Pettitt et al., 2018) (co-)exist in phytotelmata, yet the effect of limited visibility on community-wide changes has not been tested in these systems. We propose that phytotelmata provide natural mesocosms to investigate and compare the responses of different community structures (and the diverse visual systems of the animals that these communities contain) to habitat disturbance. Out of the handful of studies that have considered amphibian responses to visually limited conditions, most have followed trends found in fishes (i.e., reduced anti-predator behavior in salamanders, Zabierek and Gabor, 2016; decreased growth rates in frogs, Wood and Richardson, 2009). In amphibians, these experiments could be taken one step further by considering potential carry-over effects of how rearing environments shape behavior through metamorphosis. Overall, it has been shown that environmental stress has significant carry-over effects from larval to adult stages in amphibians (size: Scott, 1994; reproductive organs: Harper and Semlitsch, 2007). Thus, tadpoles raised in the stress of turbid conditions may not only be physically smaller as a result of reduced foraging performance but also have reduced fitness in adulthood compared to their counterparts raised in clear water conditions.
In turbid habitats, the best option to improve visual function is to filter out the added background light arising from scattering that does not contribute to image formation (Lythgoe, 1979). While scattering from large particles tends to be wavelength-independent, scattering coming from small particles (Rayleigh scattering) that also contributes to image quality degradation is enriched in short wavelengths (i.e., ultraviolet, violet, blue) (Douglas and Marshall, 1999). Thus, a decreased sensitivity in the “blue” part of the spectrum, be it at the level of the eye as a whole or the individual retinal photoreceptor cells, would help to some degree to deal with the detrimental effects of turbidity. Conversely, an increased sensitivity in the long-wavelength (i.e., “red”) part of the spectrum would arguably improve vision in freshwater aquatic environments which tend to be redder than marine ones (Lythgoe, 1979). Thus, we would expect considerable overlap between the strategies that visual systems can use to cope with turbid environments and with red-colored environments (such as the ones we show in Figure 1), even if they differ substantially in overall light availability.
Several species of frogs have some kind of pigment in their crystalline lenses, many of them resembling those of fishes in their absorbance profile, which selectively remove short wavelength light before it reaches the retina (Yovanovich et al., 2020). However, because these data come from adult individuals, it remains to be investigated if these filtering properties are already present in the tadpoles of relevant species, and whether larval stage could provide a compelling biological explanation for those cases in which the adult filtering pattern does not follow any immediately obvious logic. Interestingly, other recent work has found differences in expression levels of genes related to lens composition in tadpoles versus juveniles of the frog Lithobates sphenocephalus (Schott et al., 2021). If these gene products were ones to affect light transmittance in a wavelength-dependent manner (e.g., Röll, 2001), the finding would argue against the speculation outlined just above, and highlight the need to explore the issue in more species to find -or rule- out potential patterns.
At the level of the retina, the availability of photoreceptors with peak sensitivities at short wavelengths is another potential point to control scattering-related visual noise. As it turns out, the highly blue-sensitive rods unique to amphibians, which allow color discrimination in extremely dim light (Yovanovich et al., 2017), only appear toward the end of the larval phase in Xenopus laevis (Chang and Harris, 1998; Parker et al., 2010). It is tempting to speculate that such a delay in the acquisition of blue sensitivity “protects” the tadpoles’ visual system from the degradation of image quality caused by short wavelength scattering, but here again we must take this finding as an encouragement to study photoreceptors’ ontogeny synchronization in a variety of tadpoles from different ecomorphological backgrounds, rather than settling on it as the default pattern.
The components discussed so far cannot be expected to adjust to short-term changes in the visual environment, and so the spectral absorbance profile of individual photoreceptor cells remains the best candidate to allow tadpoles’ visual systems to adapt to changes in turbidity in physiological time-scales. This profile is a property of the visual pigment (opsin apoprotein + Vitamin A-derived chromophore) housed in the photoreceptors membranes, and depends solely on the amino acid sequence of the opsin and the type of Vitamin A (1 or 2) used (Govardovskii et al., 2000). The opsin sequence is, naturally, genetically encoded, and thus cannot be changed during the lifetime of the animal in response to changes in the environment. Overall photoreceptor spectral sensitivity can, in fact, be worked around by regulating the gene expression levels in cases when several copies with slightly different sensitivity peaks are available such as the fast-speciating cichlid fishes radiations from the African great lakes (Carleton and Yourick, 2020). Furthermore, opsin expression levels within individuals can fluctuate as a consequence of manipulations in the light environment in killifish (Fuller et al., 2010; Fuller and Claricoates, 2011). However, such a diversified opsin gene pool is not typically available in amphibians, but all the opposite: the sensitivity peak for rhodopsin sits on a particularly narrow range in the spectrum compared to vertebrate standards (Crescitelli, 1958; Donner and Yovanovich, 2020). In contrast, switching from Vitamin A1 to Vitamin A2 (which confer higher sensitivity toward the blue and red parts of the spectrum, respectively) is quite straightforward and achieved by a single enzyme conserved across vertebrates, the expression levels of which are quite reactive to changes in the photic environment, even though the underlying mechanism remains unknown (Corbo, 2021). Documented cases among fishes include change of chromophore ratios in response to water temperature (Ueno et al., 2005), seasonality (Temple et al., 2006), and turbidity (Bridges, 1972; Beatty, 1984).
In the case of amphibians, the general trend is to go from a Vitamin A2-rich tadpole to Vitamin A1-rich adults, except in species who remain aquatic throughout life such as Xenopus laevis (see Donner and Yovanovich, 2020 for a review), supporting the view that Vitamin A2-rich retinas are beneficial in typically “red-shifted” freshwater environments (Corbo, 2021). The evidence that the A2/A1 transition occurs both at different ontogenetic stages and at different rates depending on the species (Hödl, 1975), and that the vitamin ratio can reversibly change during the tadpole phase in response to manipulations of light levels (Bridges, 1970), suggests that the Vitamin A system is the most readily available modulator of visual function in response to unpredictable changes in visually challenging environments.
The ultimate effect of shifting amphibian chromophore ratios on behavior remains unknown, but is a fascinating consideration for evolutionary ecologists to approach. For example, we have found an impressive range of tint and turbidity levels in the pools of water in which Dendrobates tinctorius tadpoles are deposited (Figure 1). Based on previous work on visual pigments, we would hypothesize that tadpoles deposited in more turbid pools of water would have a red-shifted chromophore system. Dendrobates tinctorius tadpoles are aggressive predators and appear to partially rely on vision to attack each other (Fouilloux et al., 2020), and thus presumably rely on vision to hunt other prey as well. Ultimately, with a noisier visual scape, red-shifted tadpoles may not be as effective at hunting, cannibalizing, or even foraging in general, but may perform better in visually challenging environments over having not shifted at all; overall, this flexibility could have remarkable impacts on tadpole fitness and survival (Figure 2).
One of the most covered topics in turbidity literature is predator-prey dynamics. The majority of work considers prey activity (reduced antipredator behavior: Meager et al., 2006; Kimbell and Morrell, 2015), and predator accuracy (Bonner and Wilde, 2002; Gadomski and Parsley, 2005) under various turbidity conditions. Although interactions between predator and prey most often hinge on whether the animal is more dependent on chemical or visual cues, or a combination of the two (Swanbrow Becker and Gabor, 2012), visually guided behaviors often break-down in turbid environments (Chivers et al., 2012; Swanbrow Becker and Gabor, 2012). This results in different advantages for predators or prey as a function of the modalities used for detection/evasion. For example, some tadpoles have adapted anti-predator phenotypes such that, when under high predation risk, individuals will express redder-colored fins, which have been hypothesized to serve either as camouflage or to prevent lethal attacks, compared to those that are not (Hyla chrysoscelis, McCollum and Van Buskirk, 1996; Dendropsophus ebraccatus, Touchon and Warkentin, 2008). The underlying rationale is that the redder fins may direct predators to strike non-lethal parts of the tadpole’s body, or that the spotty coloration could act as some kind of camouflage by “breaking up” the contour of the tadpole’s body. The effects of turbidity on the expression of anti-predator signaling in tadpoles remain unknown.
As turbidity interferes with color vision (Wilkins et al., 2016), its fluctuations can impact the interpretation of color as (including, but not limited to) a proxy for distance (Bartel et al., 2021). Until now, the change of color-based signals in response to visually challenging environments has been demonstrated only in a handful of studies, and limited to the context of adult signaling. In gudgeon fish, a two-week transplant experiment showed the overall expression of melanin decreased in turbid conditions, leading to lighter colored fishes (i.e., economy of pigments hypothesis; Côte et al., 2019). We hypothesize that increased turbidity levels will induce a “relaxed” phenotype where tadpoles do not express camouflage/disruptive coloration despite the potential presence of predators. The direction of which actor would benefit from this phenotype is dependent on the detection mechanisms used by predators and prey.
The change of color expression as a function of visually challenging environments could also be interesting in the context of aposematic signaling. In fire salamanders, it has been shown that the background albedo of larval environments influences juvenile phenotype (Sanchez et al., 2019), indicating that the light quality of larval environments can have impacts that persist through metamorphosis. The carry-over effects of larval rearing environment on adult coloration have yet to be explored in frogs; based on salamander responses, it is plausible that anuran tadpoles who have lived in turbid/red-shifted environments could carry over to the color expression in their (semi-) terrestrial adult forms, perhaps in having less saturated coloration as a result of developmental stress and a low-quality diet during their larval stages. This could have remarkable implications for aposematic amphibians who rely on color as an anti-predator (or sexual) signal (Brown, 2013; Segami Marzal et al., 2017).
In response to the impressive variation in color displayed among poison frogs and the interest in aposematic signaling throughout the clade, establishing a novel driver of color variation through turbidity-induced carry-over effects has the potential to be valuable across research disciplines. Exploring how colors change in the face of increased turbidity would be a great opportunity to understand the plasticity of color-based signals in antipredator contexts, and how these are shaped by optically challenging scenarios. Overall, amphibian larvae provide an exciting opportunity for exploring adaptations to visually limited environments from both proximate and ultimate perspectives, and framing these responses comparatively across clades, habitats, and geography will surely challenge evolutionary ecologists for years to come.
The original contributions presented in the study are included in the article, further inquiries can be directed to the corresponding author.
CF gave shape to the initial draft and designed both figures. All authors were directly involved in all stages from conceptualization to final editions.
BR and CF were funded by the Academy of Finland (Academy Research Fellowship to BR, Project No. 21000042021). CY was funded by the European Union’s Horizon 2020 program under grant agreement N° 101026409.
The authors declare that the research was conducted in the absence of any commercial or financial relationships that could be construed as a potential conflict of interest.
All claims expressed in this article are solely those of the authors and do not necessarily represent those of their affiliated organizations, or those of the publisher, the editors and the reviewers. Any product that may be evaluated in this article, or claim that may be made by its manufacturer, is not guaranteed or endorsed by the publisher.
BR was thankful to Walter Hödl for a stimulating discussion on tadpole vision under different environments. CF was thankful to her wonderful mother for all the grammar advice, thanks mom! We highly value equity, diversity, and inclusion in science. We are proud to have three female co-authors who represent different countries, backgrounds, and career stages – this diversity contributed to the mentorship and support that gave rise to this perspective work.
Bartel, P., Janiak, F. K., Osorio, D., and Baden, T. (2021). Colourfulness as a possible measure of object proximity in the larval zebrafish brain. Curr. Biol. 31, R235–R236. doi: 10.1016/j.cub.2021.01.030
Beatty, D. D. (1984). Visual pigments and the labile scotopic visual system of fish. Vision Res. 24, 1563–1573. doi: 10.1016/0042-6989(84)90314-6
Beiswenger, R. E. (1977). Diel patterns of aggregative behavior in tadpoles of bufo americanus, in relation to light and temperature. Ecology 58, 98–108.
Biju, S. D., Senevirathne, G., Garg, S., Mahony, S., Kamei, R. G., Thomas, A., et al. (2016). Frankixalus, a new rhacophorid genus of tree hole breeding frogs with Oophagous Tadpoles. PLoS One 11:e0145727. doi: 10.1371/journal.pone.0145727
Bonner, T. H., and Wilde, G. R. (2002). Effects of turbidity on prey consumption by prairie stream fishes. Trans. Am. Fish. Soc. 131, 1203–1208. doi: 10.1577/1548-8659(2002)131<1203:eotopc>2.0.co;2
Bridges, C. D. B. (1970). Reversible Visual Pigment Changes in Tadpoles exposed to Light and Darkness. Nature 227, 956–957. doi: 10.1038/227956a0
Bridges, C. D. B. (1972). “The rhodopsin-porphyropsin visual system,” in Photochemistry of Vision, ed. H. J. A. Dartnall (Berlin: Springer-Verlag).
Brown, J. L. (2013). The evolution of parental care, aposematism and color diversity in Neotropical poison frogs. Evol. Ecol. 27, 825–829. doi: 10.1007/s10682-013-9642-2
Caldwell, J. P. (1989). Structure and behavior of Hyla geographica Tadpole Schools, with Comments on Classification of Group Behavior in Tadpoles. Copeia 1989, 938–950. doi: 10.2307/1445980
Caldwell, J. P. (1993). Brazil nut fruit capsules as phytotelmata: interactions among anuran and insect larvae. Can. J. Zool. 71, 1193–1201. doi: 10.1139/z93-163
Carleton, K. L., and Yourick, M. R. (2020). Axes of visual adaptation in the ecologically diverse family Cichlidae. Semin. Cell Dev. Biol. 106, 43–52. doi: 10.1016/j.semcdb.2020.04.015
Chang, W. S., and Harris, W. A. (1998). Sequential genesis and determination of cone and rod photoreceptors in Xenopus. J. Neurobiol. 35, 227–244. doi: 10.1002/(sici)1097-4695(19980605)35:3<227::aid-neu1>3.0.co;2-0
Chivers, D. P., Al-Batati, F., Brown, G. E., and Ferrari, M. C. O. (2012). The effect of turbidity on recognition and generalization of predators and non-predators in aquatic ecosystems. Ecol. Evol. 3, 268–277. doi: 10.1002/ece3.454
Corbo, J. C. (2021). Vitamin A1/A2 chromophore exchange: its role in spectral tuning and visual plasticity. Dev. Biol. 475, 145–155. doi: 10.1016/j.ydbio.2021.03.002
Cossio, R., Wright, J., Acosta, R., and Rodríguez, A. (2021). Space use and parental care behaviour in Andinobates claudiae (Dendrobatidae). Behaviour 2021, 1–24. doi: 10.1163/1568539X-bja10139
Côte, J., Pilisi, C., Morisseau, O., Veyssière, C., Perrault, A., Jean, S., et al. (2019). Water turbidity affects melanin-based coloration in the gudgeon: a reciprocal transplant experiment. Biol. J. Linnean. Soc. 128, 451–459.
Crescitelli, F. (1958). The natural history of visual pigments. Ann. N. Y. Acad. Sci. 74, 230–255. doi: 10.1111/j.1749-6632.1958.tb39548.x
Donner, K., and Yovanovich, C. A. M. (2020). A frog’s eye view: foundational revelations and future promises. Semin. Cell Dev. Biol. 106, 72–85. doi: 10.1016/j.semcdb.2020.05.011
Douglas, R. H., and Marshall, N. J. (1999). “A review of vertebrate and invertebrate ocular filters,” in Adaptive Mechanisms in the Ecology of Vision, eds S. N. Archer, M. B. A. Djamgoz, E. R. Loew, J. C. Partridge, and S. Vallerga (Dordrecht: Springer), 95–162. doi: 10.1007/978-94-017-0619-3_5
Engström-Öst, J., and Candolin, U. (2006). Human-induced water turbidity alters selection on sexual displays in sticklebacks. Behav. Ecol. 18, 393–398. doi: 10.1093/beheco/arl097
Fincke, O. M. (1992). Interspecific competition for tree holes: consequences for mating systems and coexistence in neotropical damselflies. Am. Natural. 139, 80–101. doi: 10.1086/285314
Fouilloux, C. A., Serrano Rojas, S. J., Carvajal-Castro, J. D., Valkonen, J. K., Gaucher, P., Fischer, M., et al. (2021). Pool choice in a vertical landscape: tadpole-rearing site flexibility in phytotelm-breeding frogs. Ecol. Evol. 11, 9021–9038. doi: 10.1002/ece3.7741
Fouilloux, C., Fromhage, L., Valkonen, J. K., and Rojas, B. (2020). Size-dependent tradeoffs in aggressive behavior towards kin. bioRxiv [Preprint] bioRxiv 2020.10.26.350132, doi: 10.1101/2020.10.26.350132
Fuller, R. C., and Claricoates, K. M. (2011). Rapid light-induced shifts in opsin expression: finding new opsins, discerning mechanisms of change, and implications for visual sensitivity. Mol. Ecol. 20, 3321–3335. doi: 10.1111/j.1365-294X.2011.05180.x
Fuller, R. C., Noa, L. A., and Strellner, R. S. (2010). Teasing apart the many effects of lighting environment on opsin expression and foraging preference in bluefin killifish. Am. Natural. 176, 1–13. doi: 10.1086/652994
Gadomski, D. M., and Parsley, M. J. (2005). Effects of turbidity, light level, and cover on predation of white sturgeon larvae by prickly sculpins. Trans. Am. Fish. Soc. 134, 369–374. doi: 10.1577/t03-213.1
Gouchie, G. M., Roberts, L. F., and Wassersug, R. J. (2008). The effect of mirrors on African clawed frog (Xenopus laevis) larval growth, development, and behavior. Behav. Ecol. Sociobiol. 62, 1821–1829. doi: 10.1007/s00265-008-0611-7
Govardovskii, V. I., Fyhrquist, N., Reuter, T., Kuzmin, D. G., and Donner, K. (2000). In search of the visual pigment template. Vis. Neurosci. 17, 509–528. doi: 10.1017/S0952523800174036
Granqvist, M., and Mattila, J. (2004). The effects of turbidity and light intensity on the consumption of mysids by juvenile perch (Perca fluviatilis L.). Hydrobiologia 514, 93–101. doi: 10.1007/978-94-017-0920-0_9
Harper, E. B., and Semlitsch, R. D. (2007). Density dependence in the terrestrial life history stage of two anurans. Oecologia 153, 879–889. doi: 10.1007/s00442-007-0796-x
Hettyey, A., Rölli, F., Thürlimann, N., Zürcher, A.-C., and Van Buskirk, J. (2012). Visual cues contribute to predator detection in anuran larvae. Biol. J. Linnean. Soc. 106, 820–827.
Hödl, W. (1975). Die Entwicklung der spektralen Empfindlichkeit der Netzhaut von Bombina, Hyla, Pelobates und Rana. Zool. Jahrbücher Abteilung Allgemeine Zool. Physiol. Tiere 79, 173–203.
Hoff, K., Blaustein, A., Mcdiarmid, R. W., and Altig, R. (1999). “Behavior: interactions and their consequences,” in Tadpoles: Biology of Anuran Larvae, eds R. W. McDiarmid and R. Altig (Chicago, IL: University of Chicago Press), 215–239.
Horppila, J., Liljendahl-Nurminen, A., and Malinen, T. (2004). Effects of clay turbidity and light on the predator-prey interaction between smelts and chaoborids. Can. J. Fish. Aquat. Sci. 61, 1862–1870. doi: 10.1139/f04-123
Järvenpää, M., and Lindström, K. (2004). Water turbidity by algal blooms causes mating system breakdown in a shallow-water fish, the sand goby Pomatoschistus minutus. Proc. R. Soc. B 271, 2361–2365. doi: 10.1098/rspb.2004.2870
Kam, Y., Su, Y., Liu, J., and Lin, Y. (2001). Intraspecific interactions among oophagous tadpoles (Chirixalus eiffingeri: Rhacophoridae) living in bamboo stumps in Taiwan. J. Zool. 255, 519–524. doi: 10.1017/S0952836901001601
Katz, L. C., Potel, M. J., and Wassersug, R. J. (1981). Structure and mechanisms of schooling in tadpoles of the clawed frog, Xenopus laevis. Anim. Behav. 29, 20–33. doi: 10.1016/S0003-3472(81)80148-0
Kimbell, H. S., and Morrell, L. J. (2015). Turbidity influences individual and group level responses to predation in guppies, Poecilia reticulata. Anim. Behav. 103, 179–185. doi: 10.1016/j.anbehav.2015.02.027
Kitching, R. L. (2001). Food webs in phytotelmata: “Bottom-Up” and “Top-Down” explanations for Community Structure. Annu. Rev. Entomol. 46, 729–760. doi: 10.1146/annurev.ento.46.1.729
Kumpulainen, N. (2021). Communication Between the Tadpoles of the Dyeing Poison Frog Dendrobates tinctorius (Anura, Dendrobatidae). MSc thesis, Department of Biological and Environmental Science, University of Jyväskylä, Finland.
Ladich, F., and Winkler, H. (2017). Acoustic communication in terrestrial and aquatic vertebrates. J. Exp. Biol. 220, 2306–2317. doi: 10.1242/jeb.132944
Lehtinen, R. M. (2004). Tests for competition, cannibalism, and priority effects in two phytotelm-dwelling tadpoles from Madagascar. Herpetologica 60, 1–13. doi: 10.1655/02-88
Mathis, U., Schaeffel, F., and Howland, H. C. (1988). Visual optics in toads (Bufo americanus). J. Comp. Physiol. A 163, 201–213. doi: 10.1007/bf00612429
McCollum, S. A., and Van Buskirk, J. (1996). Costs and benefits of a predator-induced polyphenism in the gray treefrog Hyla chrysoscelis. Evolution 50, 583–593. doi: 10.1111/j.1558-5646.1996.tb03870.x
Meager, J. J., Domenici, P., Shingles, A., and Utne-Palm, A. C. (2006). Escape responses in juvenile Atlantic cod Gadus morhua L.: the effects of turbidity and predator speed. J. Exp. Biol. 209, 4174–4184. doi: 10.1242/jeb.02489
Parker, R. O., Mccarragher, B., Crouch, R., and Darden, A. G. (2010). Photoreceptor development in premetamorphic and metamorphic Xenopus laevis. Anatom. Rec. 293, 383–387. doi: 10.1002/ar.21079
Pettitt, B. A., Bourne, G. R., and Bee, M. A. (2018). Predictors and benefits of microhabitat selection for offspring deposition in golden rocket frogs. Biotropica 50, 919–928. doi: 10.1111/btp.12609
Reustle, J. W., and Smee, D. L. (2020). Cloudy with a chance of mesopredator release: turbidity alleviates top-down control on intermediate predators through sensory disruption. Limnol. Oceanogr. 65, 2278–2290. doi: 10.1002/lno.11452
Röll, B. (2001). Multiple origin of diurnality in geckos: evidence from eye lens crystallins. Naturwissenschaften 88, 293–296. doi: 10.1007/s001140100227
Rot-Nikcevic, I., Denver, R. J., and Wassersug, R. J. (2005). The influence of visual and tactile stimulation on growth and metamorphosis in anuran larvae. Funct. Ecol. 19, 1008–1016. doi: 10.1111/j.1365-2435.2005.01051.x
Rot-Nikcevic, I., Taylor, C. N., and Wassersug, R. J. (2006). The role of images of conspecifics as visual cues in the development and behavior of larval anurans. Behav. Ecol. Sociobiol. 60, 19–25. doi: 10.1007/s00265-005-0133-5
Rowe, D. K., and Dean, T. L. (1998). Effects of turbidity on the feeding ability of the juvenile migrant stage of six New Zealand freshwater fish species. New Zealand J. Mar. Freshw. Res. 32, 21–29. doi: 10.1080/00288330.1998.9516803
Saidapur, S. K., Veeranagoudar, D. K., Hiragond, N. C., and Shanbhag, B. A. (2009). Mechanism of predator–prey detection and behavioral responses in some anuran tadpoles. Chemoecology 19, 21–28. doi: 10.1016/j.chemosphere.2012.02.026
Sanchez, E., Pröhl, H., Lüddecke, T., Schulz, S., Steinfartz, S., and Vences, M. (2019). The conspicuous postmetamorphic coloration of fire salamanders, but not their toxicity, is affected by larval background albedo. J. Exp. Zool. Part B 332, 26–35. doi: 10.1002/jez.b.22845
Schott, R. K., Bell, R. C., Loew, E. R., Thomas, K. N., Gower, D. J., Streicher, J. W., et al. (2021). Genomic and spectral visual adaptation in southern leopard frogs during the ontogenetic transition from aquatic to terrestrial light environments. bioRxiv [Preprint] doi: 10.1101/2021.02.19.432049
Scott, D. E. (1994). The effect of larval density on adult demographic traits in Ambystoma opacum. Ecology 75, 1383–1396. doi: 10.2307/1937462
Segami Marzal, J. C., Rudh, A., Rogell, B., Ödeen, A., Løvlie, H., Rosher, C., et al. (2017). Cryptic female Strawberry poison frogs experience elevated predation risk when associating with an aposematic partner. Evol. Ecol. 7, 744–750. doi: 10.1002/ece3.2662
Serrano-Rojas, S. J., and Pašukonis, A. (2021). Tadpole-transporting frogs use stagnant water odor to find pools in the rainforest. J. Exp. Biol. 224, jeb243122. doi: 10.1242/jeb.243122
Shrimpton, S. J., Streicher, J. W., Gower, D. J., Bell, R. C., Fujita, M. K., Schott, R. K., et al. (2021). Eye-body allometry across biphasic ontogeny in anuran amphibians. Evol. Ecol. 35, 337–359. doi: 10.1007/s10682-021-10102-3
Sontag, C., Wilson, D. S., and Wilcox, R. S. (2006). Social foraging in Bufo americanus tadpoles. Anim. Behav. 72, 1451–1456. doi: 10.1016/j.anbehav.2006.05.006
Stynoski, J. L., and Noble, V. R. (2012). To beg or to freeze: multimodal sensory integration directs behavior in a tadpole. Behav. Ecol. Sociobiol. 66, 191–199. doi: 10.1007/s00265-011-1266-3
Summers, K., and McKeon, C. S. (2004). “The evolutionary ecology of phytotelmata use in Neotropical poison frogs,” in Ecology and Evolution of Phytotelm-Breeding Anurans, Vol. 193, ed. R. M. Lehtinen (Ann Arbor, MI: University of Michigan), 55–73.
Summers, K., and Tumulty, J. (2013). “Parental care, sexual selection, and mating systems in neotropical poison frogs,” in Sexual Selection: Perspectives and Models from the Neotropics, eds R. H. Macedo and G. Machado (New York, NY: Academic Press), 289–320. doi: 10.1111/j.1420-9101.2008.01609.x
Sundin, J., Berglund, A., and Rosenqvist, G. (2010). Turbidity hampers mate choice in a pipefish. Ethology 116, 713–721.
Swanbrow Becker, L. J., and Gabor, C. R. (2012). Effects of turbidity and visual vs. chemical cues on anti-predator response in the endangered fountain darter (Etheostoma fonticola). Ethology 118, 994–1000. doi: 10.1111/eth.12002
Temple, S. E., Plate, E. M., Ramsden, S., Haimberger, T. J., Roth, W. M., and Hawryshyn, C. W. (2006). Seasonal cycle in vitamin A1/A2-based visual pigment composition during the life history of coho salmon (Oncorhynchus kisutch). J. Comp. Physiol. A 192, 301–313. doi: 10.1007/s00359-005-0068-3
Touchon, J. C., and Warkentin, K. M. (2008). Fish and dragonfly nymph predators induce opposite shifts in color and morphology of tadpoles. Oikos 117, 634–640. doi: 10.1111/j.0030-1299.2008.16354.x
Ueno, Y., Ohba, H., Yamazaki, Y., Tokunaga, F., Narita, K., and Hariyama, T. (2005). Seasonal variation of chromophore composition in the eye of the Japanese dace, Tribolodon hakonensis. J. Comp. Physiol. A 191, 1137–1142. doi: 10.1007/s00359-005-0037-x
Wilkins, L., Marshall, N. J., Johnsen, S., and Osorio, D. (2016). Modelling fish colour constancy, and the implications for vision and signalling in water. J. Exp. Biol. 219, 1884–1892. doi: 10.1242/jeb.139147
Wisenden, B. D. (2000). Olfactory assessment of predation risk in the aquatic environment. Philos. Trans. R. Soc. Lond. B 355, 1205–1208. doi: 10.1098/rstb.2000.0668
Wood, S. L. R., and Richardson, J. S. (2009). Impact of sediment and nutrient inputs on growth and survival of tadpoles of the Western Toad. Freshw. Biol. 54, 1120–1134. doi: 10.1111/j.1365-2427.2008.02139.x
Yovanovich, C. A. M., Koskela, S. M., Nevala, N., Kondrashev, S. L., Kelber, A., and Donner, K. (2017). The dual rod system of amphibians supports colour discrimination at the absolute visual threshold. Philos. Trans. R. Soc. Lond. B 372, 20160066. doi: 10.1098/rstb.2016.0066
Yovanovich, C. A. M., Pierotti, M. E. R., Kelber, A., Jorgewich-Cohen, G., Ibáñez, R., and Grant, T. (2020). Lens transmittance shapes ultraviolet sensitivity in the eyes of frogs from diverse ecological and phylogenetic backgrounds. Proc. R. Soc. B 287:20192253. doi: 10.1098/rspb.2019.2253
Keywords: larval vision, turbidity, chromophore shift, phytotelmata, phenotypic plasticity
Citation: Fouilloux CA, Yovanovich CAM and Rojas B (2022) Tadpole Responses to Environments With Limited Visibility: What We (Don’t) Know and Perspectives for a Sharper Future. Front. Ecol. Evol. 9:766725. doi: 10.3389/fevo.2021.766725
Received: 30 August 2021; Accepted: 15 December 2021;
Published: 25 January 2022.
Edited by:
Derya Akkaynak, Florida Atlantic University, United StatesReviewed by:
Jennifer Kelley, University of Western Australia, AustraliaCopyright © 2022 Fouilloux, Yovanovich and Rojas. This is an open-access article distributed under the terms of the Creative Commons Attribution License (CC BY). The use, distribution or reproduction in other forums is permitted, provided the original author(s) and the copyright owner(s) are credited and that the original publication in this journal is cited, in accordance with accepted academic practice. No use, distribution or reproduction is permitted which does not comply with these terms.
*Correspondence: Carola A. M. Yovanovich, Yy5hLm0ueW92YW5vdmljaEBzdXNzZXguYWMudWs=
Disclaimer: All claims expressed in this article are solely those of the authors and do not necessarily represent those of their affiliated organizations, or those of the publisher, the editors and the reviewers. Any product that may be evaluated in this article or claim that may be made by its manufacturer is not guaranteed or endorsed by the publisher.
Research integrity at Frontiers
Learn more about the work of our research integrity team to safeguard the quality of each article we publish.