- 1Department of Entomology, University of Nebraska-Lincoln, Lincoln, NE, United States
- 2Corn Host Plant Resistance Research Unit, USDA-ARS, Mississippi State, MS, United States
- 3Wheat, Sorghum, and Forage Research Unit, USDA-ARS, Lincoln, NE, United States
- 4Department of Biochemistry, University of Nebraska-Lincoln, Lincoln, NE, United States
The European corn borer (ECB; Ostrinia nubilalis) is an economically damaging insect pest of maize (Zea mays L.), an important cereal crop widely grown globally. Among inbred lines, the maize genotype Mp708 has shown resistance to diverse herbivorous insects, although several aspects of the defense mechanisms of Mp708 plants are yet to be explored. Here, the changes in root physiology arising from short-term feeding by ECB on the shoot tissues of Mp708 plants was evaluated directly using transcriptomics, and indirectly by monitoring changes in growth of western corn rootworm (WCR; Diabrotica virgifera virgifera) larvae. Mp708 defense responses negatively impacted both ECB and WCR larval weights, providing evidence for changes in root physiology in response to ECB feeding on shoot tissues. There was a significant downregulation of genes in the root tissues following short-term ECB feeding, including genes needed for direct defense (e.g., proteinase inhibitors and chitinases). Our transcriptomic analysis also revealed specific regulation of the genes involved in hormonal and metabolite pathways in the roots of Mp708 plants subjected to ECB herbivory. These data provide support for the long-distance signaling-mediated defense in Mp708 plants and suggest that altered metabolite profiles of roots in response to ECB feeding of shoots likely negatively impacted WCR growth.
Introduction
Plants have evolved various strategies to defend themselves against insect attack, mostly through direct and indirect defenses (War et al., 2018; Nalam et al., 2019). While feeding on host plants, insects secrete herbivore-associated molecular patterns (HAMPs), which are analogous to pathogen-associated molecular patterns (PAMPs), or microbe-associated molecular patterns in pathogens or microbes. Among HAMPs, oral secretions (OS), saliva, frass, and herbivore-associated endosymbionts from chewing insects have been identified in influencing plant defenses (Basu et al., 2018). Plants presumably use surface immune receptors to recognize these insect HAMPs (Steinbrenner et al., 2020), and further induce a multitude of downstream defenses to curb the insect growth and/or colonization on host plants (Basu et al., 2018; Acevedo et al., 2019; Zogli et al., 2020).
The European corn borer (ECB; Ostrinia nubilalis), known as the “billion-dollar bug,” is a damaging pest of maize (Zea mays L.) and estimated to cost a billion dollars annually for plant treatment and lost crop yield (Hutchison et al., 2010). ECB management using transgenic maize plants was highly successful for several years, however, recently there are concerns regarding field-evolved ECB resistance to transgenic maize plants (Smith et al., 2019). ECB feeds on the aboveground parts of the maize plants and uses OS or saliva to modulate the plant defense responses. It was suggested that the indole-3-acetic acid (IAA) present in the OS of ECB may function as a HAMP in modulating maize defense responses (Dafoe et al., 2013). Another study showed that ECB saliva strongly induced direct defenses in maize (Louis et al., 2013). ECB saliva induced defense-related transcripts including lipoxygenase (LOX) and 12-phytodienoic acid (OPR) genes, which are involved in the biosynthesis of jasmonic acid (JA; Louis et al., 2013). It was also shown that ECB caterpillars utilized host-specific salivary factors in modulating plant defenses (Louis et al., 2013). In addition to ECB OS/saliva, ECB frass was also reported to modulate maize defense responses (Ray et al., 2016b).
Maize inbred line Mp708, which exhibits broad-based resistance to insect pests, was developed by crossing the insect resistant Mp704 and susceptible Tx601 plants (Williams et al., 1990). Several studies demonstrated that Mp708 provides resistance to both above and belowground feeding caterpillars (Pechan et al., 2000; Gill et al., 2011; Castano-Duque et al., 2017) as well as to piercing-sucking insects (Louis et al., 2015; Varsani et al., 2016, 2019; Pingault et al., 2021). Mp708’s resistance to insect pests is mediated by the defense protein Maize Insect Resistance1-Cysteine Protease (Mir1-CP), which damages the peritrophic matrix of caterpillars and significantly reduces caterpillar growth (Pechan et al., 2000, 2002). Other defense-related proteins such as proteases, protease inhibitors, and peroxidases were also induced by caterpillar feeding on maize plants, which could also negatively impact caterpillar growth on Mp708 plants (Castano-Duque and Luthe, 2018). In addition, several other factors are also involved in providing maize resistance to chewing insects. For example, resistance to western corn rootworm (WCR; Diabrotica virgifera virgifera) can be influenced by root architecture (Branson et al., 1982), biomechanical strength (Meihls et al., 2012), or biochemical composition (Van Dam, 2009) of host plants. Among direct defense, plants can adapt their morphology by increasing trichome densities that create a physical barrier for insects (Tian et al., 2012). At the molecular level, chitinases, protease inhibitors, and related compounds are synthesized to counter insect invasion (Jamal et al., 2013; War et al., 2018).
Phytohormones also play a key role in modulating maize defense against chewing insects. Among them, phytohormones such as JA, salicylic acid (SA), and ethylene (ET) are the critical players in providing resistance to chewing pests (Erb et al., 2012). ECB feeding on maize foliage resulted in an increased accumulation of phytoalexins such as benzoxianoids and kauralexins (Dafoe et al., 2011). Interestingly, JA acts as a regulator of benzoxianoid and kauralexin biosynthesis (Oikawa et al., 2002), when acting in concert with ET. Whereas, JA can act synergistically with ET, SA and indole acetic acid can negatively regulate JA levels and actions (Koornneef and Pieterse, 2008). SA, JA, and abscisic acid have also been found in the fall armyworm (FAW; Spodoptera frugiperda) saliva (Acevedo et al., 2019). Application of these phytohormones on plants with the same concentrations detected in the caterpillar saliva induced the expression of mir1 in maize and lowered herbivore-induced defenses in tomato (Acevedo et al., 2019).
Aboveground to belowground communication is vital for plants to activate appropriate defenses against various stresses. When attacked by insect pests, plants activate defense responses both locally and systemically (Balmer et al., 2013). For example, several studies indicate that aboveground feeding by insect pests trigger belowground root-based defenses and vice-versa (Erb et al., 2009, 2015; Louis et al., 2015; Varsani et al., 2016). Similarly, caterpillar feeding on maize foliage can modulate long distance defense-related gene expression in roots (Ankala et al., 2013). Based on previous findings, we hypothesize that the aboveground feeding by ECB will trigger belowground defenses and modulate transcriptomic signatures in the belowground root tissues of the insect resistant Mp708 genotype. In this study, we used RNA-seq to investigate the dynamics of maize root transcriptome after short-term (24 h) aboveground feeding by ECB on maize foliage. We also evaluated how changes in root physiology induced by ECB herbivory affect larval growth of WCR, a major root-feeding insect pest of maize.
Materials and Methods
Plant and Insect Materials
Maize inbred lines, Mp708 and Tx601, used in this study were grown in soil mixed with vermiculite and perlite (PRO-MIX BX BIOFUNGICIDE + MYCORRHIZAE, Premier Tech Horticulture Ltd., Canada) in growth chambers with 14:10 (L:D) h photoperiod, 160 μE light m–2 s–1, 25°C, and 50–60% relative humidity. All plants for the experiments were used at the V2-V3 developmental stage (∼2 weeks; Ritchie et al., 1998). These plants were grown in 3.8 cm × 21.0 cm plastic Cone-tainers (Hummert International, Earth City, MO, United States). ECB and WCR were obtained from Benzon Research Inc., PA, and Crop Characteristics Inc., MN, respectively. ECB eggs obtained from Benzon Research Inc. were reared on artificial diet in growth chamber with 14:10 (L:D) h photoperiod at 23°C as described previously (Louis et al., 2013). Non-diapausing WCR eggs were supplied by Crop Characteristics Inc. in 2015. WCRs were originally collected from susceptible non-Bt maize fields in Minnesota, United States, and this colony was maintained on non-Bt maize plants at Crop Characteristics Inc. for more than 80 generations (Scott Sargent, Crop Characteristics Inc. personal communication). Thus, these WCR populations were never exposed to selection for Bt resistance, and therefore, remain susceptible to Bt plants. WCR eggs obtained were kept in growth chamber with 14:10 (L:D) h photoperiod at 23°C. Larvae were hatched at 23°C in growth chambers and were used for experiments.
Insect Bioassays
For insect bioassays, two newly molted 2nd instar ECB each weighing approximately 8–12 mg were introduced into the whorl region of maize plants. Individual plants were caged and after 5 days ECB larvae were collected. Only one ECB larva was able to be recovered from each maize plant due to ECB cannibalism, and the final weight individual larva was recorded. Similarly, to understand the interaction between ECB and WCR, two newly molted 5th instar ECB were introduced into the whorl region of Mp708 plants. After 24 h, ECB larvae were removed from the whorl region, and two newly hatched neonates of WCR were used to infest each plant. A fine-tipped paint brush (size O) was used to transfer neonate WCR larvae to maize plants as described previously (Jakka et al., 2016). Briefly, newly hatched WCR larvae were placed on the exposed nodal roots of maize plants and a thin layer of soil was used to cover the exposed roots. Bioassay plants were kept in a growth chamber with 14:10 (L:D) h photoperiod, 25°C, and 50–60% relative humidity. These plants were watered as needed. After 12 days, the soil content of Cone-tainers were combined and the WCR were collected as described previously (Gill et al., 2011). Briefly, the soil content of all pants within a treatment was combined and sifted by hand until the collection of WCR was occasional. Subsequently, the soil was sifted through two mesh screens of different sizes (2.000 and 0.833 mm) to find the remaining WCR larvae in the soil. Finally, we ceased to monitor the presence of WCR in the soil content until none were obtained after 15–20 min of incessant searching. Mp708 plants that were uninfested with ECB on the whorl region were used as the control. Both bioassays were replicated twice with similar results and data from both independent experiments were combined and presented in Figure 1. The insect bioassay data were analyzed using mixed-model analysis of variance with experiment replication as the random effect. Analysis of variance (ANOVA) was performed using PROC GLIMMIX (SAS 9.3, SAS Institute, Cary, NC, United States), and means, when significant, were separated using the least significant difference procedure (P < 0.05).
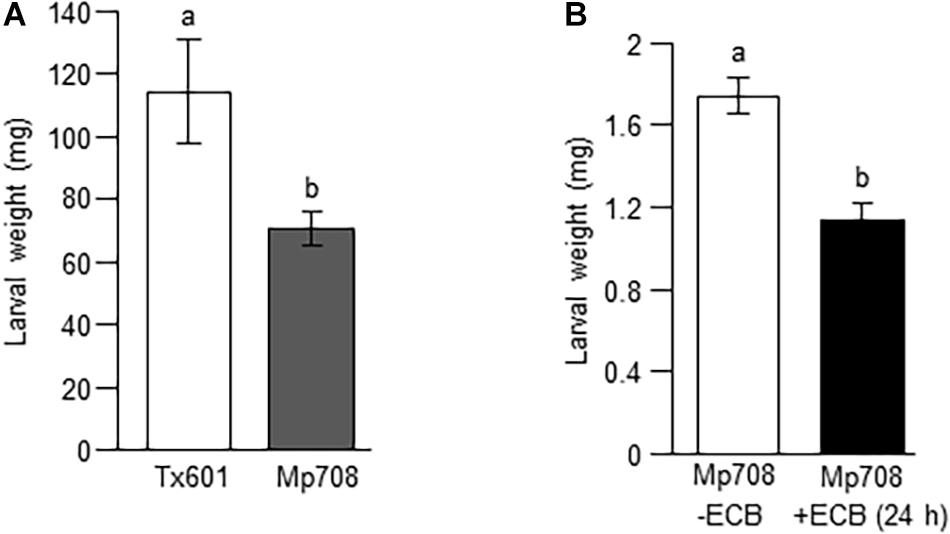
Figure 1. Mp708 provides enhanced resistance to ECB and WCR. (A) Larval weight of ECB feeding on Tx601 and Mp708 plants. Two newly molted 2nd instar ECB larvae each were introduced into the whorl region of maize plants. Individual plants were caged and after 5 days ECB larvae were collected, and final weight were recorded. (B) Aboveground feeding by ECB negatively impacts the growth of belowground feeding WCR. Two newly molted 5th instar ECB (+ECB) were introduced into the whorl region of Mp708 plants. After 24 h, ECB larvae were removed from the whorl region, and two newly hatched neonates of WCR were introduced. 12 days after the introduction of WCR, the fresh weight of WCR was measured. Mp708 plants that were uninfested with ECB (–ECB) on the whorl region were used as the control. For both (A,B), different letters above the bars indicate values that are significantly different from each other (P < 0.05). Error bars represent ± SE.
Western-Blot Analysis
The hallmark of Mp708’s defense is the accumulation of Mir1-CP protein both at the site of insect feeding or in systemic leaves or tissues away from the site of insect feeding (i.e., distal roots; Pechan et al., 2000; Louis et al., 2015; Varsani et al., 2016). To determine the presence of the Mir1-CP protein in Mp708 roots after aboveground feeding by ECB, we performed a western-blot analysis. Protein extraction and western-blot analysis were performed as described previously (Ankala et al., 2009; Varsani et al., 2016). Protein concentration was determined with Qubit reagent (Q33211) and Qubit 4 Fluorometer. A total of 60 μg of protein was used for electrophoresis. Upon gel electrophoresis, proteins were transferred to PVDF membrane using the iBlot 2 gel transfer system. Images were finally captured with iBright FL1000 Imaging System.
RNA Extraction, RNA-seq Libraries Construction and Sequencing
At the V2-V3 stage of Mp708 plants, two newly molted 5th instar ECB were introduced into the whorl region. Individual plants were caged, and root samples were collected 24 h after infestation. ECB uninfested Mp708 plants were used as the control. In total, three biological replicates were produced for each treatment. Each biological replicate consisted of three individual maize tissues (root) pooled together. For each replicate, three ECB-infested and uninfested root tissues were collected and flash-frozen in liquid nitrogen. Maize Mp708 root tissues (80–100 mg) were ground using 2010 Geno/Grinder (SPEX SamplePrep, NJ, United States) for 40 s at 1,400 strokes min–1. Total RNA was extracted from the homogenized tissue using Qiagen RNeasy Plant Mini Kit. Extracted total RNA was quantified through Nanodrop 2000c Spectrophotometer (Thermo Scientific TM). Then, RNA-seq libraries were constructed by using the mRNA-seq standard TruSeq protocol from Illumina. RNA-seq libraries were sequenced in 50 bp paired-end with 20 million reads on average per library.
Analysis of RNA-Seq Libraries
The quality check of the RNA-seq libraries was performed with FASTQC (Andrews, 2010) and reads with a Phred score lower than 20 and length below 45 base pairs were removed with Trimmomatic v0.39 (Bolger et al., 2014). Then, trimmed reads were mapped on the maize reference genome v41 with Tophat2 (Kim et al., 2013) using the following parameters: 1 mismatch (-N 1), 0 splicing mismatch (-m 0), unique mapped reads (-g 1 -M). The transcripts reconstruction was performed with Cufflinks v2.2.1 with the following parameters: quantification against the reference annotation only (-G), multi-read-correct (-u) and frag-biais-correct (-b). The differentially expressed gene analysis was performed with Cuffdiff 2.2.1. Differentially expressed genes (DEGs) were identified with the following parameters: multiple-testing corrected P-values ≤ 5% and expression ratios of | log2(Infested/Control)| ≥ log2(2). Gene ontology (GO) were analyzed with MaizeMine2 by using the reference annotation as a template.
Functional Annotation
The GOBU package was used for enrichment calculations (Lin et al., 2006). The full set of maize gene annotation was used as the reference comparison set against down or upregulated DEGs. The P-values were calculated using Fisher’s exact test and corrected for multiple testing with FDR method using the R module called “P-adjust.”
Results
Mp708 Provided Enhanced Resistance to European Corn Borer and Aboveground Feeding by European Corn Borer Negatively Impacted the Growth of Belowground Feeding Western Corn Rootworm
Previously, it was shown that Mp708 provides enhanced resistance to different feeding guilds of insect pests (Pechan et al., 2000; Lopez et al., 2007; Gill et al., 2011; Louis et al., 2015; Varsani et al., 2016; Pingault et al., 2021). Here, we examined whether Mp708 provides enhanced resistance to stem boring insect, ECB. Two independent experiments with similar experimental set up were performed. In the first and second bioassays,10 and 14 plants each of Tx601 and Mp708, respectively, were used and the combined data is presented in Figure 1A. Our results show that ECB growth was lower on the Mp708 plants compared to the maize inbred line Tx601 (Figure 1A). As mentioned before, the hallmark of Mp708’s defense is the accumulation of Mir1-CP at the site (i.e., leaf) and distal parts (i.e., roots) of the plant after insect infestation (Louis et al., 2015; Varsani et al., 2016). To determine if Mir1-CP accumulates in the roots after foliar feeding by ECB, we monitored the Mir1-CP levels in the roots of Mp708 plants using immunoblot analysis. Our results suggest an increased accumulation of Mir1-CP levels in the roots after ECB feeding in the aboveground parts of the plant for 24 h (Supplementary Figure 1). Next, we monitored whether aboveground feeding by ECB on Mp708 plants can induce resistance to subsequent herbivory by belowground feeding of WCR. In the first bioassay, out of 16 WCR larvae introduced on eight Mp708 plants, we recovered 12 and 13 WCR larvae on Mp708 plants that were preinfested with ECB (+ECB) and Mp708 plants that were uninfested with ECB (-ECB), respectively. Similarly, in the second bioassay, out of 24 WCR larvae introduced on 12 Mp708 plants, we recovered 17 and 19 WCR larvae on Mp708 plants that were preinfested with ECB (+ECB) and Mp708 plants that were uninfested with ECB (-ECB), respectively, and the fresh weight of each WCR was recorded. Here, the datasets from two independent experiments with similar experimental set up were combined pooled and the combined data is presented in Figure 1B. Our insect bioassay results indicate that aboveground feeding by ECB for 24 h provided enhanced resistance to subsequent herbivory by belowground feeding of WCR (Figure 1B). Collectively, these data suggest that aboveground feeding by ECB can trigger belowground defenses in maize.
European Corn Borer Feeding Activated Hormone Pathways and Suppressed Biosynthesis of Secondary Metabolites in the Roots of Mp708 Plants
After 24 h of ECB feeding on the whorl region of the Mp708 plants, we identified 4,793 genes that were differentially expressed in Mp708 roots. Among these, 1,629 genes were upregulated, while 3,164 genes were downregulated (Figure 2 and Supplementary Table 1). The gene ontology enrichment was performed for each up and downregulated gene sets. The top ten gene ontology molecular functions terms enriched in upregulated DEGs were related to “protein kinase activity” (141 genes), “carbohydrate binding” (48 genes), “protein serine/threonine kinase activity” (97 genes), “Oxidoreductase activity, acting on paired donors, with incorporation, or reduction of molecular oxygen” (51 genes; Figure 2 and Supplementary Table 2). Molecular function terms enriched in downregulated genes included “peroxidase activity” (69 genes), “oxidoreductase activity” (294 genes), “antioxidant activity” (72 genes; Figure 2 and Supplementary Table 2).
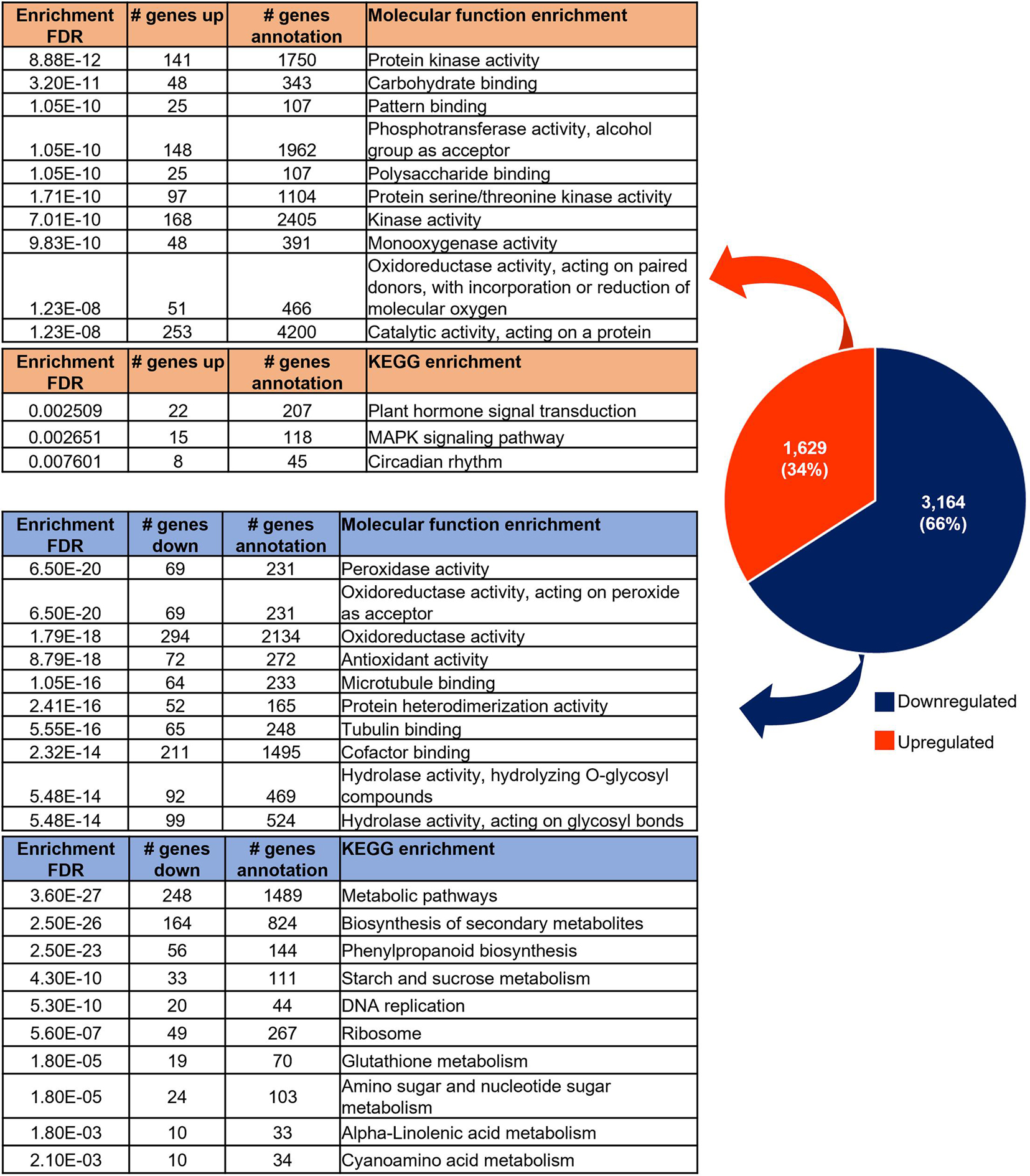
Figure 2. Number of up or downregulated differentially expressed genes (DEGs) and their associated functional enrichment.
The gene ontology biological process enrichment analysis revealed that downregulated genes are related with functions enriched in “response to oxidative stress,” “oxidation-reduction process,” or “carbohydrate metabolic process” while upregulated genes are related to functions as: “protein phosphorylation,” “regulation of nucleic acid-templated transcription,” “regulation of transcription, DNA-templated” (Supplementary Table 2).
The KEGG pathway analysis revealed three pathways enriched in the upregulated genes: “plant hormones signal transduction” (22 genes), “MAPK signaling pathway” (15 genes) and “Circadian rhythm” (8 genes; Figure 2 and Supplementary Table 2). Downregulated genes were enriched in “biosynthesis of secondary metabolites” (164 genes), “phenylpropanoid biosynthesis” (56 genes), “starch and sucrose metabolism” (33 genes; Figure 2 and Supplementary Table 2). In addition, 10 downregulated genes had function associated with “early nodulin-related” or “early nodulin-like protein.”
Transcription Factors Involved in Phytohormone Pathways Were Activated in Roots in Response to European Corn Borer Feeding on Whorl Region of Mp708 Plants
Among the DEGs, 670 were transcription factors (TFs) and 18 families were represented by >1% of the TFs (Figure 3). Among the 18 families, 13 were composed of at least 50% of downregulated genes (Figure 3 and Supplementary Table 3), including bHLH (152 DEGs and 95 DEGs downregulated), HB (78 DEGs and 44 DEGs downregulated), NAC (62 DEGs and 32 DEGs downregulated), and AP2 (39 DEGs and 28 DEGs downregulated; Figure 3 and Supplementary Table 3). Conversely, TFs families represented by at least 50% of DEGs upregulated are G2-like (56 DEGs and 36 upregulated DEGs), WRKY (21 DEGs and 12 upregulated DEGs) and ERF (ETHYLENE RESPONSIVE FACTORS, 17 DEGs, and 13 upregulated DEGs; Figure 3 and Supplementary Table 3). The large number of upregulated ERF transcription factors in Mp708 plants further confirm our previous observation that ET acts as a central node in mediating maize defense against various feeding guilds of insect pests (Louis et al., 2015).
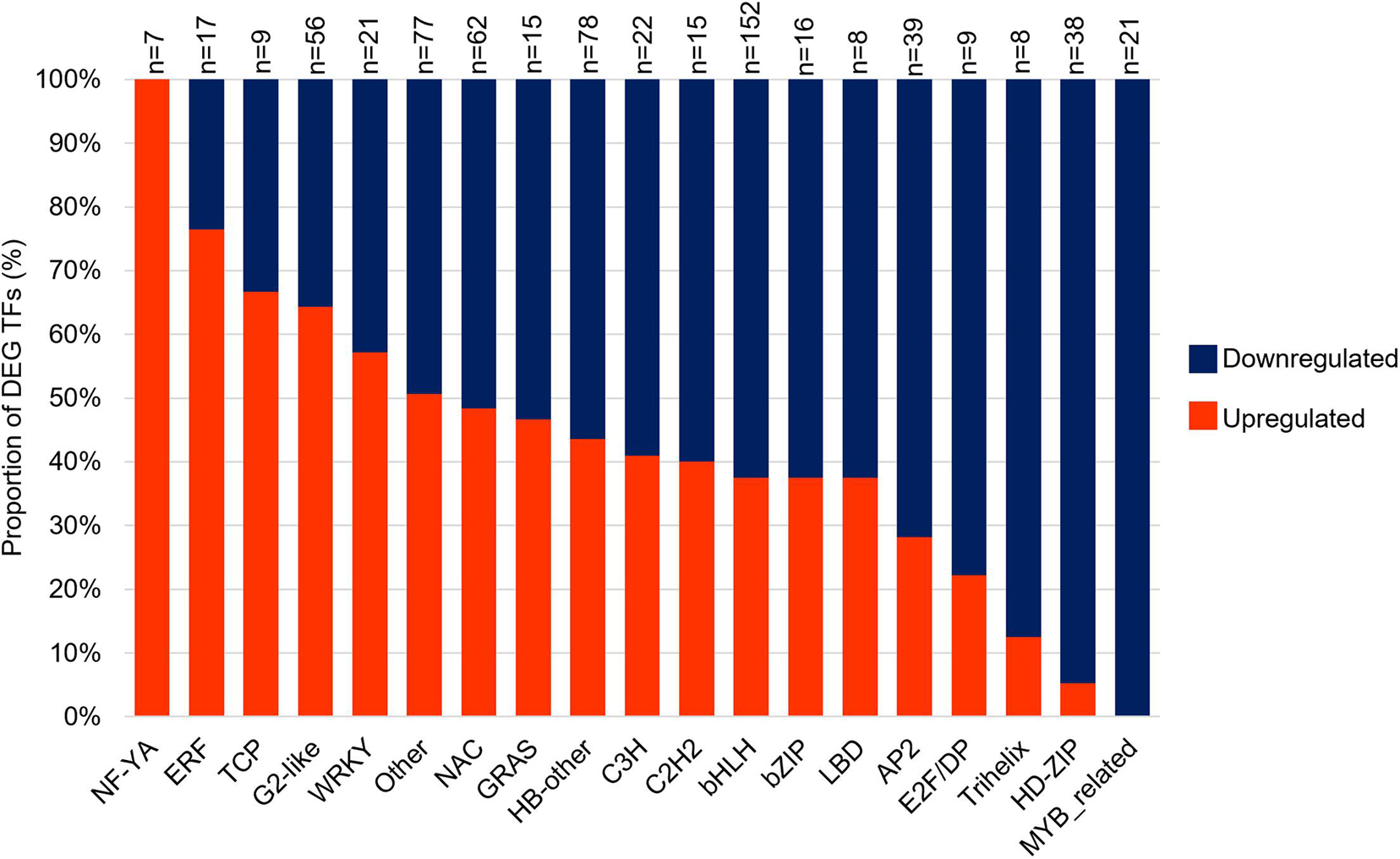
Figure 3. Differentially expressed genes (DEGs) analysis of transcription factors (TF). TFs were classified into families of related genes, and the proportion of up and downregulated within each class of TF are shown. “n” indicates the total number of TF for each family.
Genes Related to Phytohormone Pathways Were Activated in Roots Upon European Corn Borer Feeding on Mp708 Shoots
To gain a better understanding for the role of phytohormones in defense against root feeders in response to aboveground herbivory, we investigated the DEGs involved in phytohormone-related pathways. Thirty-one DEGs were found and divided between ET (11 DEGs), JA (12 DEGs), and SA (8 DEGs) pathways (Figure 4 and Supplementary Table 4). Among the 11 DEGs related to the ET pathway, six genes were upregulated: Ethylene-insensitive protein 2 (EIN2; Zm00001d039341), Ethylene-Insensitive-Like 8 (EIL8; Zm00001d016924), and four members of the 1-Aminocyclopropane-1-Carboxylic Acid Oxidase (ACO) family (ZmACO6: Zm00001d000163, ZmACO4: Zm00001d024852, ZmACO4: Zm00001d024853, and ZmACO20: Zm00001d052136). Interestingly, two gene members of the Acetyl-CoA synthetase (ACS) family were both downregulated (ACS1: Zm00001d039487 and ACS6: Zm00001d033862; Figure 4 and Supplementary Table 4). In contrast to ET, a majority of the DEGs involved in JA pathway were downregulated (11 genes) while only two genes were upregulated: JASMONATE RESISTANT 1, (JAR1; Zm00001d039345) and OPR5 (Zm00001d003584; Figure 4 and Supplementary Table 4). The three remaining genes of the OPR family, along with the six genes of the OPDA family and two genes of the ACS family, were downregulated (Figure 4 and Supplementary Table 4). All the DEGs involved in the SA pathway were downregulated in the roots of Mp708 plants after ECB feeding on the shoot region for 24 h (Figure 4 and Supplementary Table 4).
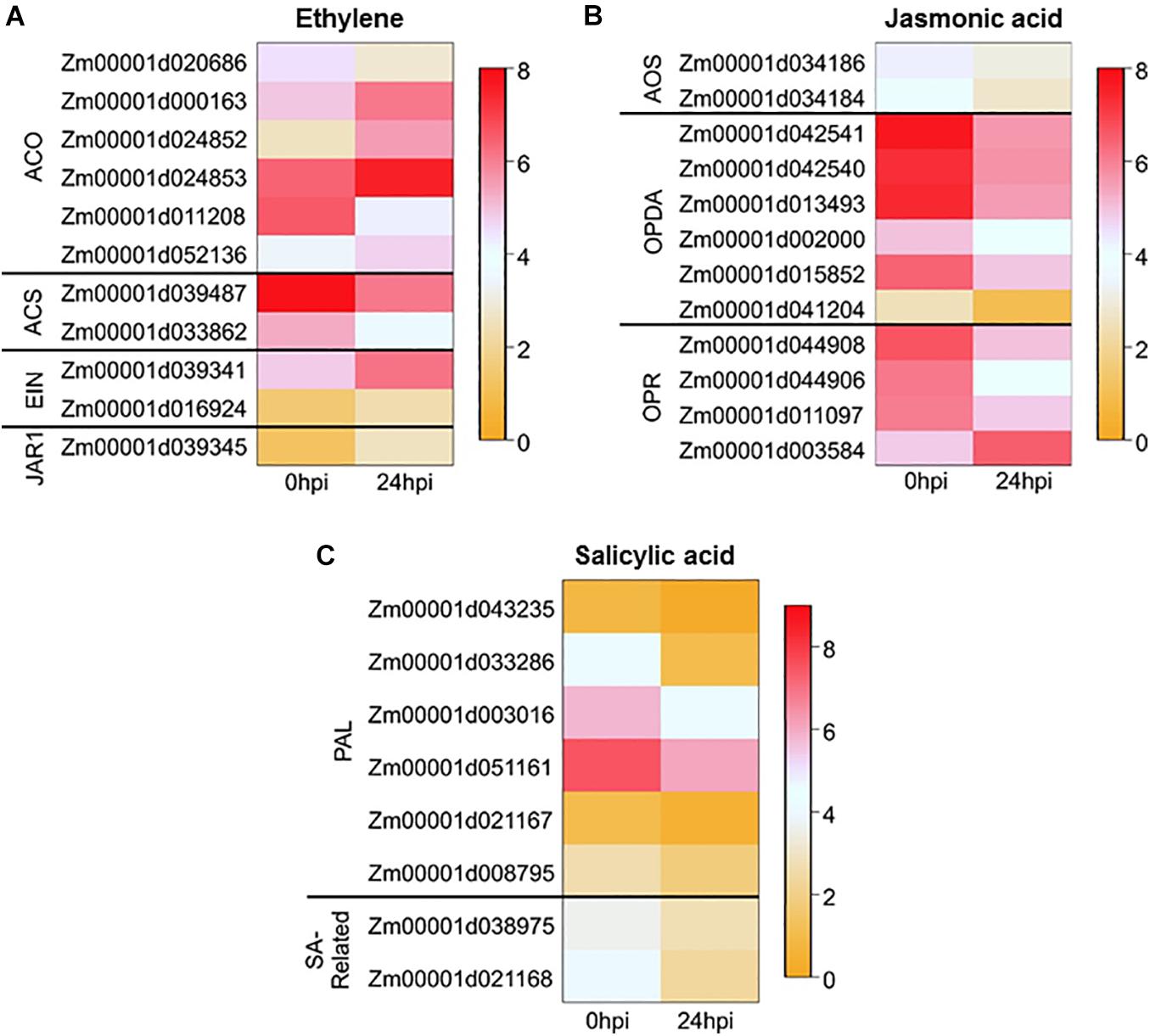
Figure 4. Heatmap of the expression ECB feeding-induced genes involved in different hormone pathways: (A) ethylene, (B) jasmonic acid, and (C) salicylic acid. For the columns Mp708_0 hpi and Mp708_24 hpi, each cell contains the relative expression level [log2(FPKM + 1)].
Altered Expression of Genes Involved in Plant Direct Defenses in Mp708 Roots After European Corn Borer Feeding on the Whorl Region
Mir1-CP, encoded by mir1, is characterized as a key component for the resistance to insect pests in maize (Pechan et al., 2000; Louis et al., 2015). Our results indicate that mir1 (Zm00001d036542) was upregulated in Mp708 roots at 24 hpi of ECB feeding on the whorl region with a high fold-change (FC; log2FC = 2.075). Protease inhibitors have also been identified in playing a role in direct plant defense mechanisms in response to insect attack (Koiwa et al., 1997). Here, one gene encoding a serpin, Zm00001d049148, was downregulated. Zm00001d002960, a member of Kunitz type inhibitor (KTI) family, was also downregulated. However, another KTI (smart serine protease inhibitor), WIN2 (Zm00001d015279), was upregulated. Three genes encoding Bowman-Birk type inhibitors were differentially expressed, among them two (Zm00001d003304 and Zm00001d040027) were downregulated and one, Zm00001d012939, was upregulated (Supplementary Table 1). Chitinases have also been identified as playing a role in plant defense and suppressing herbivore defenses (Ray et al., 2016a). Among the DEGs, 11 genes were functionally annotated as “chitinase family related,” “Chinase A,” “basic chitinase,” or “homolog of carrot EP3-3 chitinase.” Among the 11 genes encoding chitinases, five were upregulated (Zm00001d036370, Zm00001d014840, Zm00001d043988, Zm00001d003190, and Zm00001d027525) in the roots of Mp708 plants after ECB feeding for 24 h on the shoot region, with a log2FC from 1.05 (Zm00001d027525) to 2.38 (Zm00001d036370), and six genes were downregulated (Zm00001d010911, Zm00001d037656, Zm00001d036366, Zm00001d048903, Zm00001d017152, and Zm00001d042769) with a log2FC from -1.39 to -3.06 (Supplementary Table 1).
Expression Pattern of Genes Involved in Metabolite Pathways That Modulate Defense in Maize Roots Against Root Feeding Herbivores
Root feeding insects are often attracted to metabolites released from the host plant, such as flavonoids, sugars, amino acids, or terpenes (Erb et al., 2013). Genes involved in these pathways were mined from the maize annotation using KEGG ID (Supplementary Table 5) and the expression profile is provided in Figure 5. One and ten genes were upregulated in the phenylpropanoid and flavonoid pathways, respectively, (Figure 5). Among the upregulated genes, one gene encoded a flavanone 3-hydroxylase (F3H, Zm00001d001960), two genes encoded chalcone and stilbene synthase family proteins (CHS, Zm00001d052673 and Zm00001d007403), and three genes encoded 2-oxoglutarate (2OG) and Fe(II)-dependent oxygenase superfamily proteins (Zm00001d037487, Zm00001d042192, and Zm00001d051896), including DMR6 (DOWNY MILDEW RESISTANT 6, Zm00001d051896). DMR6 was proven to be involved in plant immunity for Arabidopsis (Van Damme et al., 2008). Zm00001d002287 (FLS2, Leucine-rich receptor-like protein kinase family protein) was downregulated with a log2FC (-6.04). FLS2 was described as a pattern recognition receptor that perceives the PAMP (Chinchilla et al., 2006). Three genes encoding 2-oxoglutarate (2OG) and Fe(II)-dependent oxygenase superfamily proteins were also downregulated (Zm00001d002564, Zm00001d012456, and Zm00001d047744). Three genes encoding UDP-glucosyl transferases were downregulated (Figure 5). Among the DEGs involved in amino acid metabolism, two genes were upregulated (Zm00001d028303, Zm00001d037455), and the remaining 13 genes were downregulated, as well as the seven and five DEGs involved in sugar and terpene pathways, respectively, (Figure 5). These data indicated a complex change in root metabolism within 24 h of aboveground feeding by ECB, with potential changes in the profiles and abundances of metabolites secreted by roots.
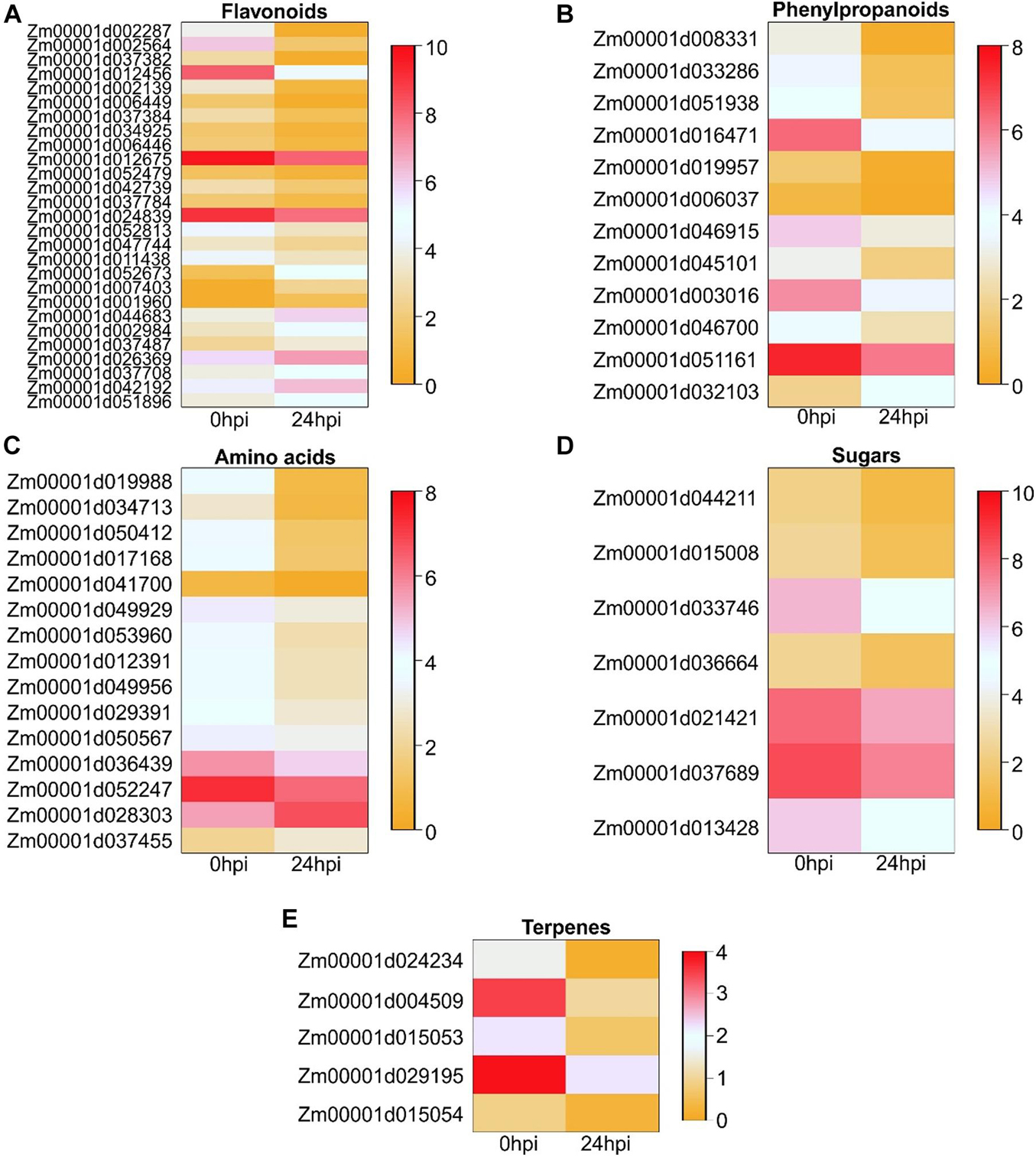
Figure 5. Heatmap of the expression ECB feeding-induced genes involved in different pathways: (A) Flavonoids, (B) Phenylpropanoids, (C) Amino acids, (D) Sugars, and (E) Terpenes. For the columns Mp708_0 hpi and Mp708_24 hpi, each cell contains the relative expression level [log2(FPKM + 1)].
Discussion
Plant response to insect herbivory varies from one genotype to another and from one pest to another. The maize genotype Mp708 has been investigated in the past for its response to FAW or WCR (Pechan et al., 2000; Castano-Duque et al., 2017). Here, we used RNA-seq to investigate the dynamics of Mp708 root transcriptome before and after ECB feeding (24 h) on the aboveground part of the plant. Previously, it was reported that short-term feeding by ECB did not impact ECB larval weight, suggesting a physiological adaptation of ECB to maize defense mechanisms (Dafoe et al., 2011). Here, the ECB larval weight was reduced after feeding on Mp708 compared to Tx601, which is susceptible to ECB, suggesting that Mp708 had resistance mechanisms not present in Tx601. Consistent with our earlier studies with corn leaf aphids (Varsani et al., 2016), our current work also documented an increased accumulation of the Mir1-CP protein in Mp708 roots following ECB herbivory on the whorl region of the plants.
At the transcriptional level, aboveground feeding by ECB for 24 h resulted in the downregulation of a majority of the DEGs in the roots of Mp708 plants. This suggests that ECB feeding resulted in the shutdown of plant functional mechanisms after ECB feeding for 24 h, including the genes involved in reactive oxygen species (ROS) metabolism. ROS and ROS metabolism play an important role in cell growth and development, as well as in the responses to biotic and abiotic stresses, and are well known modulators of plant defense (Koch et al., 2016). Both ROS and antioxidants respond to insect salivary compounds (Shinya et al., 2016). In this study, several genes encoding peroxidases were downregulated, suggesting that modulation of ROS was part of the early response of Mp708 plants to ECB herbivory. There was a significant positive enrichment of genes involved in protein phosphorylation, which might be important for plant defense signaling in Mp708 plants (Park et al., 2012; Jagodzik et al., 2018).
Compounds released by plant tissues can act as signals for insects to locate or avoid a host plant for herbivory (Erb et al., 2013). CO2 emitted by plant root as well as sugars, terpenes, and amino acids have been characterized as attractants for herbivory but other compounds such as flavonoids can act as repellents for herbivory (Erb et al., 2013). Several genes encoding proteins associated with flavonoid biosynthesis were upregulated in roots of Mp708 plants challenged with ECB, and genes associated with sugar, amino acid, and terpene metabolism were downregulated. These data indicated that even short-term herbivory of Mp708 plants by ECB changed root physiology, possibly strengthening defense mechanisms against root attacking pests, such as the WCR. The negative effects of aboveground ECB challenge on WCR growth would support this contention.
Ethylene was also described as an attractant to WCR (Erb et al., 2013). In our transcriptomic data set, several genes encoding proteins of the ET pathway (Wang et al., 2002; Lacey and Binder, 2014) were upregulated. These included ET biosynthetic enzymes ACO and an ET receptor (EIN). Similarly, JA is another important hormone required for plant defense, and several proteins are required for its biosynthesis and perception of action. Additionally, JA regulates the transcription of many genes (Staswick and Tiryaki, 2004; Ankala et al., 2009; Afrin et al., 2015; Van Moerkercke et al., 2019; Yuan et al., 2019). ECB feeding on aboveground tissues downregulated a large majority of genes involved in JA pathway in the roots. However, JAR1 (Zm00001d039345; Staswick and Tiryaki, 2004) and COI (Zm00001d012338) were upregulated after 24 h of ECB feeding, suggesting that the JA-Ile formation was induced by ECB-feeding. It is known that JA-Ile binding to COI1-JAZ induces JA responsive genes (Pauwels et al., 2010; Zhang et al., 2017), such as mir1. Here, Mp708 showed an accumulation of mir1 transcripts in response to ECB feeding. Furthermore, it was previously shown in Mp708 that Mir1-CP accumulation was positively regulated by JA (Ankala et al., 2009).
Insect herbivores use diverse strategies to manipulate the JA-SA antagonism in plants to improve fitness outcomes. In many cases, herbivores use their salivary secretion to activate SA pathway and suppress JA-regulated defenses (Weech et al., 2008; Diezel et al., 2009). In Mp708 plants, ECB herbivory downregulated the genes in the SA pathway after 24 h of feeding, indicating that the defense response of Mp708 plants was not shifted by ECB feeding to increase SA responses. Potentially, this could be part of the resistance mechanism of Mp708 plants relative to other genetically related but susceptible maize lines. It has also been shown that ET not only had a synergistic interaction with JA (Penninckx et al., 1998) but was also a modulator of JA (Bostock, 2005; Von Dahl and Baldwin, 2007; Pieterse et al., 2009). ET can influence SA levels, but SA can antagonistically interact with JA. Thus, the crosstalk between ET, JA, and SA is a finely regulated process (Yang et al., 2019). In response to ECB feeding, early defense mechanisms were mostly associated with the increase of ET pathway genes, but the apparent antagonism of JA and SA was not induced by ECB feeding for 24 h. Future research will address how chewing insects influence ET, JA, and SA regulated resistance mechanisms in Mp708 plants compared to related susceptible lines.
Conclusion
European corn borer herbivory of aerial tissues in maize genotype Mp708 triggered large scale transcriptomic modulation in root tissues after 24 h of ECB introduction. Many genes associated with ROS mitigation were downregulated. However, several genes encoding protein kinases were upregulated, suggesting signaling cascades responsive to long-distance signals from the shoots. Transcriptomic analysis showed that genes encoding proteins associated with phytohormones ET and JA were activated after short-term ECB feeding. A similar upregulation of genes related to SA were not observed, indicating that ECB were not manipulating the JA-SA relationship in the root system. These transcriptomic data suggests that resistance mechanisms in Mp708 plants to chewing insects might be based on finely tuned regulation of plant hormonal pathways, and subsequent changes in plant metabolism.
Data Availability Statement
The datasets presented in this study can be found in online repositories. The names of the repository/repositories and accession number(s) can be found below: NCBI (accession: PRJNA684328).
Author Contributions
JL conceived and designed the research. LP performed the computational analysis. SB and PZ performed the experiments. GS and NP contributed to methods development, reagents, and data analysis. WPW developed the maize genotype used in the study. LP, GS, and JL wrote the article with contributions from all authors. All authors have read and agreed to the final version of the manuscript.
Funding
This work was partially supported by the Nebraska Agricultural Experiment Station with funding from the Hatch Act (Accession #1007272) through the USDA National Institute of Food and Agriculture and funds from the University of Nebraska-Lincoln to JL. GS and NP were supported by the USDA-ARS CRIS project 3042-21000-034-00D.
Conflict of Interest
The authors declare that the research was conducted in the absence of any commercial or financial relationships that could be construed as a potential conflict of interest.
Publisher’s Note
All claims expressed in this article are solely those of the authors and do not necessarily represent those of their affiliated organizations, or those of the publisher, the editors and the reviewers. Any product that may be evaluated in this article, or claim that may be made by its manufacturer, is not guaranteed or endorsed by the publisher.
Acknowledgments
We thank University of Minnesota Genomics Center for RNA-sequencing. We also thank Sajjan Grover for the help with statistical analysis of insect bioassays.
Supplementary Material
The Supplementary Material for this article can be found online at: https://www.frontiersin.org/articles/10.3389/fevo.2021.765940/full#supplementary-material
Supplementary Figure 1 | Mir1-CP accumulation in the roots after ECB feeding on Mp708 leaves.
Supplementary Table 1 | Differentially expressed genes expression level, contrast analysis.
Supplementary Table 2 | Differentially expressed genes function enrichment. (C: cellular component, F: molecular function and P: biological process).
Supplementary Table 3 | Transcription factor (TF) families frequency.
Supplementary Table 4 | Differentially expressed genes expression level of genes involved in phytohormone pathways.
Supplementary Table 5 | Differentially expressed genes expression level of genes involved in metabolite pathways.
Footnotes
- ^ https://genome.jgi.doe.gov/portal/pages/dynamicOrganismDownload.jsf?organism=Zmays
- ^ http://maizemine.rnet.missouri.edu:8080/maizemine/begin.do
References
Acevedo, F. E., Smith, P., Peiffer, M., Helms, A., Tooker, J., and Felton, G. W. (2019). Phytohormones in fall armyworm saliva modulate defense responses in plants. J. Chem. Ecol. 45, 598–609. doi: 10.1007/s10886-019-01079-z
Afrin, S., Huang, J.-J., and Luo, Z.-Y. (2015). JA-mediated transcriptional regulation of secondary metabolism in medicinal plants. Sci. Bull. 60, 1062–1072. doi: 10.1007/s11434-015-0813-0
Andrews, S. (2010). FastQC: A Quality Control Tool for High Throughput Sequence Data. Cambridge: The Babraham Institute.
Ankala, A., Kelley, R. Y., Rowe, D. E., Williams, W. P., and Luthe, D. S. (2013). Foliar herbivory triggers local and long distance defense responses in maize. Plant Sci. 199, 103–112. doi: 10.1016/j.plantsci.2012.09.017
Ankala, A., Luthe, D. S., Williams, W. P., and Wilkinson, J. R. (2009). Integration of ethylene and jasmonic acid signaling pathways in the expression of maize defense protein Mir1-CP. Mol. Plant Microbe. Interact. 22, 1555–1564. doi: 10.1094/MPMI-22-12-1555
Balmer, D., de Papajewski, D. V., Planchamp, C., Glauser, G., and Mauch-Mani, B. (2013). Induced resistance in maize is based on organ-specific defence responses. Plant J. 74, 213–225. doi: 10.1111/tpj.12114
Basu, S., Varsani, S., and Louis, J. (2018). Altering plant defenses: herbivore-associated molecular patterns and effector arsenal of chewing herbivores. Mol. Plant Microbe. Interact. 31, 13–21. doi: 10.1094/MPMI-07-17-0183-FI
Bolger, A. M., Lohse, M., and Usadel, B. (2014). Trimmomatic: a flexible trimmer for Illumina sequence data. Bioinformatics 30, 2114–2120. doi: 10.1093/bioinformatics/btu170
Bostock, R. M. (2005). Signal crosstalk and induced resistance: straddling the line between cost and benefit. Ann. Rev. Phytopathol. 43, 545–580. doi: 10.1146/annurev.phyto.41.052002.095505
Branson, T. F., Sutter, G. R., and Fisher, J. R. (1982). Comparison of a tolerant and a susceptible maize inbred under artificial infestations of Diabrotica virgifera virgifera?: yield and adult emergence. Environ. Entomol. 11, 371–372. doi: 10.1093/ee/11.2.371
Castano-Duque, L., Loades, K. W., Tooker, J. F., Brown, K. M., Paul Williams, W., and Luthe, D. S. (2017). A maize inbred exhibits resistance against western corn rootwoorm, Diabrotica virgifera virgifera. J. Chem. Ecol. 43, 1109–1123. doi: 10.1007/s10886-017-0904-2
Castano-Duque, L., and Luthe, D. S. (2018). Protein networks reveal organ-specific defense strategies in maize in response to an aboveground herbivore. Arthropod Plant Interac. 12, 147–175. doi: 10.1007/s11829-017-9562-0
Chinchilla, D., Bauer, Z., Regenass, M., Boller, T., and Felix, G. (2006). The Arabidopsis receptor kinase FLS2 binds flg22 and determines the specificity of flagellin perception. Plant Cell 18, 465–476. doi: 10.1105/tpc.105.036574
Dafoe, N. J., Huffaker, A., Vaughan, M. M., Duehl, A. J., Teal, P. E., and Schmelz, E. A. (2011). Rapidly induced chemical defenses in maize stems and their effects on short-term growth of Ostrinia nubilalis. J. Chem. Ecol. 37, 984–991. doi: 10.1007/s10886-011-0002-9
Dafoe, N. J., Thomas, J. D., Shirk, P. D., Legaspi, M. E., Vaughan, M. M., Huffaker, A., et al. (2013). European Corn Borer (Ostrinia nubilalis) induced responses enhance susceptibility in maize. PLoS One 8:e73394. doi: 10.1371/journal.pone.0073394
Diezel, C., von Dahl, C. C., Gaquerel, E., and Baldwin, I. T. (2009). Different lepidopteran elicitors account for cross-talk in herbivory-induced phytohormone signaling. Plant Physiol. 150, 1576–1586. doi: 10.1104/pp.109.139550
Erb, M., Flors, V., Karlen, D., de Lange, E., Planchamp, C., D’Alessandro, M., et al. (2009). Signal signature of aboveground-induced resistance upon belowground herbivory in maize. Plant J. 59, 292–302. doi: 10.1111/j.1365-313X.2009.03868.x
Erb, M., Huber, M., Robert, C. A. M., Ferrieri, A. P., Machado, R. A. R., and Arce, C. C. M. (2013). “Chapter Two - The Role of Plant Primary and Secondary Metabolites in Root-Herbivore Behaviour, Nutrition and Physiology,” in Advances in Insect Physiology Behaviour and Physiology of Root Herbivores, eds S. N. Johnson, I. Hiltpold, and T. C. J. Turlings (Cambridge: Academic Press), 53–95. doi: 10.1016/B978-0-12-417165-7.00002-7
Erb, M., Meldau, S., and Howe, G. A. (2012). Role of phytohormones in insect-specific plant reactions. Trends Plant Sci. 17, 250–259. doi: 10.1016/j.tplants.2012.01.003
Erb, M., Robert, C. A. M., Marti, G., Lu, J., Doyen, G. R., Villard, N., et al. (2015). A physiological and behavioral mechanism for leaf herbivore-induced systemic root resistance. Plant Physiol. 169, 2884–2894. doi: 10.1104/pp.15.00759
Gill, T. A., Sandoya, G., Williams, P., and Luthe, D. S. (2011). Belowground resistance to western corn rootworm in lepidopteran-resistant maize genotypes. J. Eco. Entomol. 104, 299–307. doi: 10.1603/EC10117
Hutchison, W. D., Burkness, E. C., Mitchell, P. D., Moon, R. D., Leslie, T. W., Fleischer, S. J., et al. (2010). Areawide suppression of European corn borer with Bt maize reaps savings to non-Bt maize growers. Science 330, 222–225. doi: 10.1126/science.1190242
Jagodzik, P., Tajdel-Zielinska, M., Ciesla, A., Marczak, M., and Ludwikow, A. (2018). Mitogen-Activated protein kinase cascades in plant hormone signaling. Front. Plant Sci. 9:1387. doi: 10.3389/fpls.2018.01387
Jakka, S. R., Shrestha, R. B., and Gassmann, A. J. (2016). Broad-spectrum resistance to Bacillus thuringiensis toxins by western corn rootworm (Diabrotica virgifera virgifera). Sci. Rep. 6, 1–9. doi: 10.1038/srep27860
Jamal, F., Pandey, P. K., Singh, D., and Khan, M. Y. (2013). Serine protease inhibitors in plants: nature’s arsenal crafted for insect predators. Phytochem. Rev. 12, 1–34. doi: 10.1007/s11101-012-9231-y
Kim, D., Pertea, G., Trapnell, C., Pimentel, H., Kelley, R., and Salzberg, S. L. (2013). TopHat2: accurate alignment of transcriptomes in the presence of insertions, deletions and gene fusions. Genome Biol. 14:R36. doi: 10.1186/gb-2013-14-4-r36
Koch, K. G., Chapman, K., Louis, J., Heng-Moss, T., and Sarath, G. (2016). Plant tolerance: a unique approach to control hemipteran pests. Front. Plant Sci. 7:1363. doi: 10.3389/fpls.2016.01363
Koiwa, H., Bressan, R. A., and Hasegawa, P. M. (1997). Regulation of protease inhibitors and plant defense. Trends Plant Sci. 2, 379–384. doi: 10.1016/S1360-1385(97)90052-2
Koornneef, A., and Pieterse, C. M. J. (2008). Cross talk in defense signaling. Plant Physiol. 146, 839–844. doi: 10.1104/pp.107.112029
Lacey, R. F., and Binder, B. M. (2014). How plants sense ethylene gas — The ethylene receptors. J. Inorganic. Biochem. 133, 58–62. doi: 10.1016/j.jinorgbio.2014.01.006
Lin, W.-D., Chen, Y.-C., Ho, J., and Hsiao, C. (2006). GOBU: toward an integration interface for biological objects. J. Inf. Sci. Eng. 22, 19–29.
Lopez, L., Camas, A., Shivaji, R., Ankala, A., Williams, P., and Luthe, D. (2007). Mir1-CP, a novel defense cysteine protease accumulates in maize vascular tissues in response to herbivory. Planta 226, 517–527. doi: 10.1007/s00425-007-0501-7
Louis, J., Basu, S., Varsani, S., Castano-Duque, L., Jiang, V., Williams, W. P., et al. (2015). Ethylene Contributes to maize insect resistance1-mediated maize defense against the phloem sap-sucking corn leaf aphid. Plant Physiol. 169, 313–324. doi: 10.1104/pp.15.00958
Louis, J., Peiffer, M., Ray, S., Luthe, D. S., and Felton, G. W. (2013). Host-specific salivary elicitor(s) of European corn borer induce defenses in tomato and maize. New Phytol. 199, 66–73. doi: 10.1111/nph.12308
Meihls, L. N., Kaur, H., and Jander, G. (2012). Natural variation in maize defense against insect herbivores. Cold Spring Harb. Symp. Quant. Biol. 77, 269–283. doi: 10.1101/sqb.2012.77.014662
Nalam, V., Louis, J., and Shah, J. (2019). Plant defense against aphids, the pest extraordinaire. Plant Sci. 279, 96–107. doi: 10.1016/j.plantsci.2018.04.027
Oikawa, A., Ishihara, A., and Iwamura, H. (2002). Induction of HDMBOA-Glc accumulation and DIMBOA-Glc 4-O-methyltransferase by jasmonic acid in poaceous plants. Phytochemistry 61, 331–337. doi: 10.1016/S0031-9422(02)00225-X
Park, C.-J., Caddell, D. F., and Ronald, P. C. (2012). Protein phosphorylation in plant immunity: insights into the regulation of pattern recognition receptor-mediated signaling. Front. Plant Sci. 3:177. doi: 10.3389/fpls.2012.00177
Pauwels, L., Barbero, G. F., Geerinck, J., Tilleman, S., Grunewald, W., Pérez, A. C., et al. (2010). NINJA connects the co-repressor TOPLESS to jasmonate signalling. Nature 464, 788–791. doi: 10.1038/nature08854
Pechan, T., Cohen, A., Williams, W. P., and Luthe, D. S. (2002). Insect feeding mobilizes a unique plant defense protease that disrupts the peritrophic matrix of caterpillars. Proc. Natl. Acad. Sci. USA 99, 13319–13323. doi: 10.1073/pnas.202224899
Pechan, T., Ye, L., Chang, Y., Mitra, A., Lin, L., Davis, F. M., et al. (2000). A Unique 33-kD cysteine proteinase accumulates in response to larval feeding in maize genotypes resistant to fall armyworm and other lepidoptera. Plant Cell 12, 1031–1041.
Penninckx, I. A. M. A., Thomma, B. P. H. J., Buchala, A., Métraux, J.-P., and Broekaert, W. F. (1998). Concomitant activation of jasmonate and ethylene response pathways is required for induction of a plant defensin gene in arabidopsis. Plant Cell 10, 2103–2113. doi: 10.1105/tpc.10.12.2103
Pieterse, C. M. J., Leon-Reyes, A., Van der Ent, S., and Van Wees, S. C. M. (2009). Networking by small-molecule hormones in plant immunity. Nat. Chem. Biol. 5, 308–316. doi: 10.1038/nchembio.164
Pingault, L., Varsani, S., Palmer, N., Ray, S., Williams, W. P., Luthe, D. S., et al. (2021). Transcriptomic and volatile signatures associated with maize defense against corn leaf aphid. BMC Plant Biol. 21:138. doi: 10.1186/s12870-021-02910-0
Ray, S., Alves, P. C. M. S., Ahmad, I., Gaffoor, I., Acevedo, F. E., Peiffer, M., et al. (2016a). Turnabout is fair play: herbivory-induced plant chitinases excreted in fall armyworm frass suppress herbivore defenses in maize. Plant Physiol. 171, 694–706. doi: 10.1104/pp.15.01854
Ray, S., Basu, S., Rivera-Vega, L. J., Acevedo, F. E., Louis, J., Felton, G. W., et al. (2016b). Lessons from the far end: Caterpillar FRASS-induced defenses in maize, rice, cabbage, and tomato. J. Chem. Ecol. 42, 1130–1141. doi: 10.1007/s10886-016-0776-x
Ritchie, J. T., Singh, U., Godwin, D. C., and Bowen, W. T. (1998). “Cereal growth, development and yield,” in Understanding Options for Agricultural Production Systems Approaches for Sustainable Agricultural Development, eds G. Y. Tsuji, G. Hoogenboom, and P. K. Thornton (Dordrecht: Springer), 79–98. doi: 10.1007/978-94-017-3624-4_5
Shinya, T., Hojo, Y., Desaki, Y., Christeller, J. T., Okada, K., Shibuya, N., et al. (2016). Modulation of plant defense responses to herbivores by simultaneous recognition of different herbivore-associated elicitors in rice. Sci. Rep. 6:32537. doi: 10.1038/srep32537
Smith, J. L., Farhan, Y., and Schaafsma, A. W. (2019). Practical Resistance of Ostrinia nubilalis (Lepidoptera: Crambidae) to Cry1F Bacillus thuringiensis maize discovered in Nova Scotia. Can. Sci. Rep. 9:18247. doi: 10.1038/s41598-019-54263-2
Staswick, P. E., and Tiryaki, I. (2004). The oxylipin signal jasmonic acid is activated by an enzyme that conjugates it to isoleucine in Arabidopsis. Plant Cell 16, 2117–2127. doi: 10.1105/tpc.104.023549
Steinbrenner, A. D., Muñoz-Amatriaín, M., Chaparro, A. F., Aguilar-Venegas, J. M., Lo, S., Okuda, S., et al. (2020). A receptor-like protein mediates plant immune responses to herbivore-associated molecular patterns. Proc. Natl. Acad. Sci. USA 117, 31510–31518. doi: 10.1073/pnas.2018415117
Tian, D., Tooker, J., Peiffer, M., Chung, S. H., and Felton, G. W. (2012). Role of trichomes in defense against herbivores: comparison of herbivore response to woolly and hairless trichome mutants in tomato (Solanum lycopersicum). Planta 236, 1053–1066. doi: 10.1007/s00425-012-1651-9
Van Dam, N. M. (2009). Belowground herbivory and plant defenses. Annu. Rev. Ecol. Evol. Syst. 40, 373–391. doi: 10.1146/annurev.ecolsys.110308.120314
Van Damme, M., Huibers, R. P., Elberse, J., and Van den Ackerveken, G. (2008). Arabidopsis DMR6 encodes a putative 2OG-Fe(II) oxygenase that is defense-associated but required for susceptibility to downy mildew. Plant J. 54, 785–793. doi: 10.1111/j.1365-313X.2008.03427.x
Van Moerkercke, A., Duncan, O., Zander, M., Šimura, J., Broda, M., Vanden Bossche, R., et al. (2019). A MYC2/MYC3/MYC4-dependent transcription factor network regulates water spray-responsive gene expression and jasmonate levels. Proc. Natl. Acad. Sci. USA 116, 23345–23356.
Varsani, S., Basu, S., Williams, W. P., Felton, G. W., Luthe, D. S., and Louis, J. (2016). Intraplant communication in maize contributes to defense against insects. Plant Signal. Behav. 11:e1212800. doi: 10.1080/15592324.2016.1212800
Varsani, S., Grover, S., Zhou, S., Koch, K. G., Huang, P. C., Kolomiets, M. V., et al. (2019). 12-Oxo-phytodienoic acid acts as a regulator of maize defense against corn leaf aphid. Plant Physiol. 179, 1402–1415. doi: 10.1104/pp.18.01472
Von Dahl, C. C., and Baldwin, I. T. (2007). Deciphering the role of ethylene in plant–herbivore interactions. J. Plant Growth Regul. 26, 201–209. doi: 10.1007/s00344-007-0014-4
Wang, K. L.-C., Li, H., and Ecker, J. R. (2002). Ethylene biosynthesis and signaling networks. Plant Cell 14, s131–s151. doi: 10.1105/tpc.001768
War, A. R., Taggar, G. K., Hussain, B., Taggar, M. S., Nair, R. M., and Sharma, H. C. (2018). Plant defence against herbivory and insect adaptations. AoB Plants 10:37. doi: 10.1093/aobpla/ply037
Weech, M.-H., Chapleau, M., Pan, L., Ide, C., and Bede, J. C. (2008). Caterpillar saliva interferes with induced Arabidopsis thaliana defence responses via the systemic acquired resistance pathway. J. Exp. Bot. 59, 2437–2448. doi: 10.1093/jxb/ern108
Williams, W. P., Davis, F. M., and Windham, G. L. (1990). Registration of Mp708 Germplasm Line of Maize. Crop. Sci. 30:82. doi: 10.2135/cropsci1990.0011183X003000030082x
Yang, J., Duan, G., Li, C., Liu, L., Han, G., Zhang, Y., et al. (2019). The crosstalks between jasmonic acid and other plant hormone signaling highlight the involvement of jasmonic acid as a core component in plant response to biotic and abiotic stresses. Front. Plant Sci. 10:1349. doi: 10.3389/fpls.2019.01349
Yuan, X., Wang, H., Cai, J., Li, D., and Song, F. (2019). NAC transcription factors in plant immunity. Phytopathol. Res. 1:3. doi: 10.1186/s42483-018-0008-0
Zhang, L., Zhang, F., Melotto, M., Yao, J., and He, S. Y. (2017). Jasmonate signaling and manipulation by pathogens and insects. J. Exp. Bot. 68, 1371–1385. doi: 10.1093/jxb/erw478
Keywords: maize, Mp708, European corn borer, RNA-seq, phytohormones, roots, long-distance communication
Citation: Pingault L, Basu S, Zogli P, Williams WP, Palmer N, Sarath G and Louis J (2021) Aboveground Herbivory Influences Belowground Defense Responses in Maize. Front. Ecol. Evol. 9:765940. doi: 10.3389/fevo.2021.765940
Received: 27 August 2021; Accepted: 21 October 2021;
Published: 10 November 2021.
Edited by:
Flor Acevedo, The Pennsylvania State University (PSU), United StatesReviewed by:
Maya L. Evenden, University of Alberta, CanadaXin-Cheng Zhao, Henan Agricultural University, China
Copyright © 2021 Pingault, Basu, Zogli, Williams, Palmer, Sarath and Louis. This is an open-access article distributed under the terms of the Creative Commons Attribution License (CC BY). The use, distribution or reproduction in other forums is permitted, provided the original author(s) and the copyright owner(s) are credited and that the original publication in this journal is cited, in accordance with accepted academic practice. No use, distribution or reproduction is permitted which does not comply with these terms.
*Correspondence: Joe Louis, am9lbG91aXNAdW5sLmVkdQ==
†Present address: Saumik Basu, Department of Entomology, Washington State University, Pullman, WA, United States