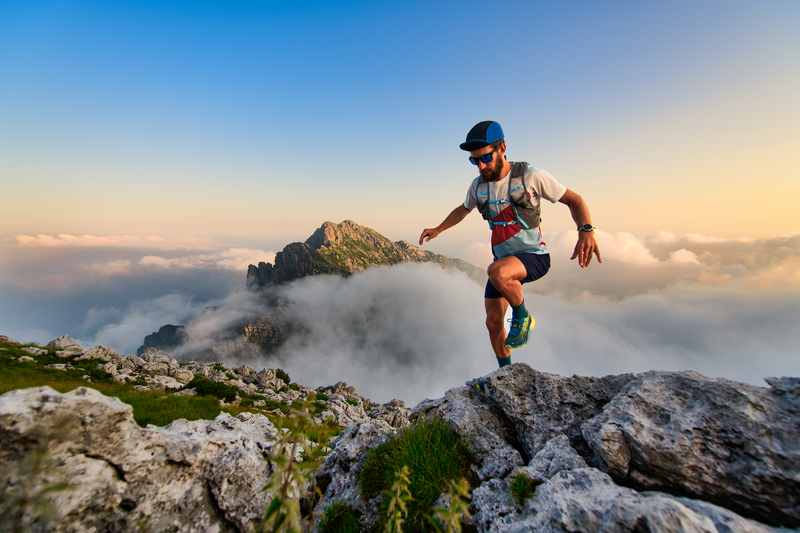
95% of researchers rate our articles as excellent or good
Learn more about the work of our research integrity team to safeguard the quality of each article we publish.
Find out more
ORIGINAL RESEARCH article
Front. Ecol. Evol. , 01 November 2021
Sec. Environmental Informatics and Remote Sensing
Volume 9 - 2021 | https://doi.org/10.3389/fevo.2021.759732
This article is part of the Research Topic Big Earth Data Intelligence for Environmental Modeling View all 15 articles
Sargassum golden tides have bloomed frequently in many sea areas throughout the world, and negatively impacted on the local marine ecology. Sargassum muticum commonly inhabits rocky shores. It is now distributed worldwide due to its invasiveness, and recently drifting individuals have been observed on the coasts of Canary Islands. However, as a potential golden tide alga, physiological, and ecological studies of this species have not been frequently explored. To investigate the responses of S. muticum to light and nitrogen, two key environmental factors in golden tide formation, we established three light levels (LL, low light, 10 μmol photons m–2 s–1; ML, medium light, 60 μmol photons m–2 s–1, and HL, high light, 300 μmol photons m–2 s–1) and two nitrogen levels (LN, low nitrogen, 25.0 μM of natural seawater; HN, high nitrogen, 125.0 μM), and cultivated the thalli under different conditions for 12 days before measuring the physiological properties of alga. The results showed that higher light and/or nitrogen levels enhanced the relative algal growth rate. The maximum net photosynthesis rate of alga increased with the light, while it remained unaffected by the nitrogen. The HN treatment had no effect on the apparent photosynthetic efficiency of algae in the LL culture, while increased it in the ML and HL cultures. The irradiance saturation point of photosynthesis was approximately 300 μmol photons m–2 s–1 with no significant difference among the six treatments, except for a slight increase under HLHN in contrast to the LLHN and MLLN treatments. HL treatment decreased the maximum quantum yield of photosynthesis (Fv/Fm) in both nitrogen levels. In the HN culture, ML and HL led to lower values of photoinhibition, indicating higher survivability in the alga. The HN culture led to higher nitrogen uptake but had no effects on Fv/Fm and the contents of pigments and soluble protein, regardless of culture light level. Based on these results, we speculate that drifting individuals of S. muticum would be possible to form a golden tide owing to its rapid growth rate at light level of 300 μmol photons m–2 s–1, when they encountered the sustained lower light level on the sea surface (≤300 μmol photons m–2 s–1). A high nitrogen supply caused by eutrophication of seawater might facilitate this process. Our results provide an important reference for the prediction of golden tides formed by S. muticum.
In recent years, there have been massive inundations of the genus Sargassum aggregating on the surface of sea, known as “golden tides,” in the central Atlantic Ocean, Gulf of Mexico (Smetacek and Zingone, 2013; Schell et al., 2015; Wang et al., 2019; Qi et al., 2020), East China Sea and Yellow Sea (Qi et al., 2017; Xing et al., 2017; Liu et al., 2018). When the golden tide blooms as an invasive genus, mats of floating Sargassum can change the ecosystem structure of the original sea area (van Tussenbroek et al., 2017; Baker et al., 2018; Caselle et al., 2018), negatively impact offshore tourism and maritime transport (Williams and Feagin, 2010; Milledge and Harvey, 2016), and lead to the deterioration of seawater quality after they sink and decay (Cruz-Rivera et al., 2015). If an episode was to occur in aquaculture areas, golden tides could cause enormous losses to the local mariculture industry, for example, blooming of Sargassum horneri golden tide in the Yellow Sea in December 2016, caused economic loss of 0.5 billion CNY due to the damaged local seaweed aquaculture (Xing et al., 2017).
The occurrence of golden tides has been linked to changes in marine environmental factors, such as nutrients, temperature, and light (Sfriso and Facca, 2013; Smetacek and Zingone, 2013). Satellite remote sensing monitoring data have confirmed that the formation of golden tides often occurs in eutrophic oceanic areas with the availability of upwelled (Brooks et al., 2018; Wang et al., 2019) and river (Sfriso and Facca, 2013; Louime et al., 2017; van Tussenbroek et al., 2017) plume nutrients, which provide sufficient nitrogen and phosphorus for the growth of golden tide algae. The fact that high nitrogen resulted in high growth rate has been found in in several algal species known to cause golden tides, namely, S. horneri (Yu et al., 2019), Sargassum natans and Sargassum fluitans (Wang et al., 2019). Therefore, many scientists hypothesize that eutrophication plays an important role in inducing the formation of Sargassum golden tides (Sfriso and Facca, 2013; Smetacek and Zingone, 2013; Louime et al., 2017; van Tussenbroek et al., 2017; Wang et al., 2019).
Alternatively, golden tide algae drifting on the sea surface receive more light, compared with those individuals attached to the seabed, owing to the light attenuated by seawater (Kouassi and Zika, 1992; Tedetti and Sempéré, 2006; Wu et al., 2010). Typically, the majority of macroalgae manifest higher photosynthesis and growth rates at higher light levels (Xu et al., 2014; Erin Cox and Smith, 2015). However, levels of light that are too high could depress macroalgal photosynthesis, which is designated photoinhibition (Yakovleva and Titlyanov, 2001; Murata et al., 2007). However, that floating Sargassum receiving more light energy, may grow explosively to form a golden tide when exposed to nutrient enrichment, for example S. natans and S. fluitans (Wang et al., 2019). Moreover, the upper and lower sides of the floating Sargassum layer will also suffer from differing light conditions owing to the thickness of the floating layer. Unfortunately, little is known about the effects of light levels on the photosynthesis related to growth of floating Sargassum, particularly when enriched by nutrients.
Currently, three species have been observed to form golden tides. S. natans and S. fluitans are the predominant species in golden tides that bloom in the central Atlantic Ocean and the Gulf of Mexico (Smetacek and Zingone, 2013; Schell et al., 2015; van Tussenbroek et al., 2017; Wang et al., 2019), while S. horneri is the only species that causes golden tides in the East China Sea and Yellow Sea (Qi et al., 2017; Xing et al., 2017; Liu et al., 2018). Although no other Sargassum species have been currently observed to form golden tides, whether they will do so in the case of appropriate environmental conditions in the future remains unclear. Sargassum muticum is widely distributed on the coast of the Western Pacific North and has recently invaded the Northern European and North American coasts (Engelen et al., 2015), resulting in significant negative impacts on local marine ecosystems (Cacabelos et al., 2013; Salvaterra et al., 2013; Sánchez and Fernández, 2018; Belattmania et al., 2020). Most seriously, many drifting alga individuals were observed on the coasts of Canary Islands (Eastern Atlantic Ocean) in March 2020 (Álvarez-Canali et al., 2021). Therefore, we suggest that more intensive study on this potential golden tide species is merited. The aim of this study was to investigate the physiological responses of S. muticum to light and nitrogen levels, two key factors during the formation of golden tides, which expectedly provides references for the future prediction of golden tides caused by this species.
Samples of S. muticum were collected from an attached population at a depth of 2 m in Lidao Bay, Rongcheng City, Shandong Province, China (37°15′ N, 122°35′ E) on April 5, 2019, where temperature is 20°C, salinity is 30 PSU, NO3–, 25.0 μM and dissolved inorganic phosphorus (DIP) is 1.6 μM in the natural seawater. Sporophytes of approximately 60 cm long were selected and brought to the laboratory in an insulated cooler of 4oC in 2 h. After cleaned with autoclaved seawater, segments that were approximately 5 cm long were randomly cut from several branches and pre-cultured in autoclaved natural seawater (salinity, 30 PSU; NO3–, 25.0 μM; NH4+, 0.8 μM and DIP, 1.6 μM) for 24 h. The temperature was set at 20°C, and the light level was 60 μmol photons m–2 s–1 with light and dark periods of 12 h:12 h. The media were continuously aerated.
After preincubation for 24 h, 5−6 segments [∼4.0 g of fresh weight (FW)] of the algae were cultured in a conical flask with 2 L of modified Provasoli’s enriched seawater (PESI, Provasoli, 1968). The DIP concentration of 5 μM in PESI medium was obtained by the addition of NaH2PO4 to ensure adequate supply of P. In addition, NaNO3 was added separately for the high nitrogen levels. According to the observation that inorganic nitrogen concentration in golden tide bloom area increased by 3–4 times (Wang et al., 2019), two nitrogen levels were established in the experiment: low nitrogen (LN, the concentration in natural seawater, 25.0 μM) and high nitrogen (HN, 125.0 μM). The effect of NH4+ was ignored due to its low content of 0.8 μM in both LN and HN treatments. The media were continuously aerated and renewed every other day. The culture density was maintained at 2.0 g FW L–1 during the experiment by removing the excess growth part of the thalli when the media was renewed.
The containers were positioned in growth chambers (MGC-250P, Yiheng Technical Co., Ltd., Shanghai, China) with constant temperature of 20°C and 12 h:12 h light and dark periods. By regulating the light source of growth chambers, light that reached the algae was set at three levels (LL, low light, 10 μmol photons m–2 s–1; ML, middle light, 60 μmol photons m–2 s–1; HL, high light, 300 μmol photons m–2 s–1),based on the daily mean irradiance in spring received by the attached thalli [the lowest and highest values in the distribution depths of 2−6 m, referred to Liu et al. (2021)] and the floating individuals (Rumyantseva et al., 2019), respectively. Light source was provided by LED lamps emitting white light in chambers, and the light levels were measured using a handheld optical quantum meter (QRT1, Hansatech, Norfolk, United Kingdom). The samples were cultured under different nitrogen and light conditions for 12 days, with three replicates of each treatment. At the end of 12-day culture, the growth rate, photosynthesis, contents of pigments and soluble protein, and nitrogen uptake of the algae were determined.
The growth of algae under different nitrogen and light conditions was measured during the final 2 days of culture in order to reflect acclimated physiological performance of thalli, and the relative growth rate (RGR) was calculated using the following formula:
Where Nt represents the FW on day t (day 12); No represents the initial FW (day 10), and t represents the time interval (2 days). The FW was determined through electronic analytical balance (ME104, Mettler-Toledo, Switzerland) after removing surface water drops of thalli by lightly blotting with tissue paper.
Dark respiration (Rd) and the rates of evolution of photosynthetic O2 were determined using a Clarktype oxygen electrode (Chlorolab-3, Hansatech, Norfolk, United Kingdom) at 20°C. Approximately 0.15 g FW of algae harvested from the culture flask on day 12 was transferred to the oxygen electrode cuvette that contained 8 ml of autoclaved natural seawater, and the media were stirred during measurement. The dark respiration rate was measured after covering the cuvette with an opaque cloth. The photosynthetic O2 evolution rates (Ek) were measured during 5 min under six levels of light (20, 60, 120, 200, 400, and 600 μmol photons m–2 s–1). White light was provided by a metal halide lamp, and different levels of irradiance were provided by altering the distance between light source and the oxygen electrode cuvette. The light levels were measured through a handheld optical quantum meter (QRT1, Hansatech, Norfolk, United Kingdom). Therefore, the P−E curve (photosynthetic rates at different irradiance levels) was obtained using the model of Eilers and Peeters (1988) and fitting the data by iteration:
Where P are the net photosynthesis rates (μmol O2 h–1 g–1 FW) at different irradiance levels; E is the irradiance (μmol photons m–2 s–1), and a, b, and c are the adjustment parameters. The parameter “a” is the photoinhibition term. The apparent photosynthetic efficiency (α), maximum net photosynthetic rate (Pmax) and irradiance saturation point (Ek) are expressed as a function of the parameters a, b, and c as follows:
The chlorophyll fluorescence yield data were measured using a plant efficiency analyzer (Handy PEA, Hansatech, Norfolk, United Kingdom) during the 6th hour of the photoperiod at end of 12 days of culture. The thalli were dark adapted for 15 min to allow all the reaction centers to open before the measurements were taken. The minimal fluorescence (F0) was induced by low irradiation of the measuring light (approximately 0.15 μmol photons m–2 s–1), and the maximum fluorescence (Fm) was obtained after exposed to a single saturating pulse irradiance of 5,000 μmol photons m–2 s–1 for 800 ms. The maximum quantum yield of photosystem II (PSII) was then estimated as:
Approximately 0.1 g FW thalli from each treatment were ground with an aqueous solution of 90% acetone. The solution was brought to 8 ml and extracted for 12 h at 4°C in the dark. After centrifugation (4,000 r min–1, 15 min), the absorption spectrum of 400–700 nm of supernatant was determined using a spectrophotometer (DU 530, Beckman Coulter, Fullerton, CA, United States). The contents (mg g–1 FW) of chlorophyll (Chl a, Chl c) and carotenoids (Car) were calculated as described by Jeffrey and Humphrey (1975) and Parsons et al. (1984), respectively.
Where A was the absorbance value of supernatant at differing wavelengths; V was the volume of solution at a constant volume (8 mL), and M was the FW of algae (g).
To extract the soluble protein, approximately 0.2 g of FW thalli from each treatment were ground in a mortar, and the volume was brought to 5 mL with phosphate buffer (0.1 mol L–1, pH 6.8). After centrifugation at 5,000 g for 10 min, the soluble protein was estimated from the supernatant using the Bradford (1976) assay with bovine serum albumin as the standard.
The rate of nitrogen uptake of the thalli was estimated from the decrease in rate of nitrate in the culture seawater over a 2-day interval when the medium was being renewed. The concentration of nitrate was determined as described by Strickland and Parsons (1972), and the rate of nitrogen uptake was calculated using the following equation:
Where N0 and Nt were the concentrations of nitrate in the medium at the initial and end of culture, respectively. V was the volume of the culture medium; M was the initial FW of the thalli (g), and t was the culture time (2 days).
The results were expressed as the means of replicates ± standard deviation. The data were analyzed using SPSS v. 21 (IBM, Inc., Armonk, NY, United States). The data under every treatment conformed to a normal distribution (Shapiro–Wilk, P > 0.05), and the variances can be considered equal (Levene’s test, P > 0.05). Two-way analysis of variance (ANOVA) was conducted to assess the effects of light and N on RGR, photosynthetic parameters from the P−E curves, dark respiration rate, Fv/Fm, pigments, soluble protein, and nitrate uptake rate. Tukey’s honest significance difference (HSD) was conducted for a post hoc investigation. A confidence interval of 95% was set for all tests.
The RGRs of S. muticum cultured under different light and nitrogen conditions are shown in Figure 1. Light and nitrogen had no interactive effect on RGR of S. muticum (ANOVA, P = 0.088), but each factor had a main effect (ANOVA, P < 0.001 for light; ANOVA, P < 0.001 for nitrogen). An increase in the light intensity enhanced the RGR at both LN andHN treatments (Tukey’s HSD, P < 0.05). Compared with LL, treatment with ML and HL increased the RGR of algae by 55.9 and 190.6% at LN levels, respectively, while under HN condition, the RGR were enhanced by 60.3% under ML and 168.9% under HL treatments. The enrichment of nitrogen also significantly promoted the growth of thalli under all three light conditions (Tukey’s HSD, P < 0.05). HN increased the RGR by 11.7, 14.8, and 3.4% in LL, ML, and HL treatments, respectively, compared with LN conditions.
Figure 1. Relative growth rates (RGRs) in Sargassum muticum grown at different light and nitrogen levels, which were determined during 2 days at the end of 12 days of incubation. Significant (P < 0.05) differences among the treatments are indicated by different lowercase letters. Vertical bars represent ± SD for the means of three samples.
The irradiance saturation point (Ek), photoinhibition term (a), apparent photosynthetic efficiency (α), and maximum net photosynthesis rate (Pmax) are presented in Table 1. The irradiance saturation point (Ek) was approximately 300 photons m–2 s–1, with no significant difference between the six treatments (Tukey’s HSD, P > 0.05), with the exception of a slight increase under HLHN in contrast to the LLHN and MLLN treatments (Tukey’s HSD, P < 0.05). Light and nitrogen had an interactive effect on Pmax (ANOVA, P < 0.001), α (ANOVA, P < 0.001) and the photoinhibition term “a” (ANOVA, P < 0.05). In all six treatments of different light and nitrogen levels, the thalli cultured in MLHN and HLHN expressed lower “a” values (Tukey’s HSD, P < 0.05), suggesting that algae that had adapted to HN and HL conditions were more tolerant of strong light inhibition. Regardless of the nitrogen concentration, Pmax increased with the light level of culture, with the exception of an insignificant difference between MLHN and HLHN (Tukey’s HSD, P > 0.05). At the ML and HL levels, the nitrogen level insignificantly affected Pmax (Tukey’s HSD, P > 0.05), while in LL treatments, HN enhanced the Pmax of thalli by 28.3%, compared with LN (Tukey’s HSD, P < 0.05). In the LN culture, the light level had no effect on the α of algae (Tukey’s HSD, P > 0.05). However, in HN culture, the value of α differed significantly between the three light levels (Tukey’s HSD, P < 0.05). The highest (0.169 ± 0.005) and lowest (0.105 ± 0.012) values of α occurred at ML and LL, respectively.
Table 1. Photosynthetic parameters from the P−E curves of Sargassum muticum cultured under different light and nitrogen conditions.
In the LL and ML culture, the dark respiration rate (Rd) of thalli remained unaffected interactively by different levels of culture light and nitrogen (ANOVA, P > 0.05, Figure 2). However, HL culture (300 μmol photons m–2 s–1) remarkably increased Rd in both nitrogen concentrations, compared with the LL and ML treatments (Tukey’s HSD, P < 0.05). In addition, in HL culture, HN enhanced the Rd by 24.6%, in contrast to that in LN culture (Tukey’s HSD, P < 0.05).
Figure 2. Dark respiration rates of S. muticum grown at different light and nitrogen levels for 12 days. Significant (P < 0.05) differences among the treatments are indicated by different lowercase letters. Vertical bars represent ± SD for the means of three samples.
The maximum photochemical yields (Fv/Fm) of the algae cultured at different light and nitrogen levels are shown in Figure 3. Light and nitrogen had no interactive effect on Fv/Fm of algae (ANOVA, P > 0.05). The nitrogen levels had an insignificant effect on Fv/Fm regardless of the culture light levels (ANOVA, P > 0.05). In both LN and HN cultures, the values of Fv/Fm expressed insignificant differences between the LL and ML conditions (Tukey’s HSD, P > 0.05), while it decreased remarkably in the HL treatment compared with the LL and ML treatments (Tukey’s HSD, P < 0.05).
Figure 3. The maximum quantum yield of photosystem II (Fv/Fm) in S. muticum grown at different light and nitrogen levels for 12 days. Significant (P < 0.05) differences among the treatments are indicated by different lowercase letters. Vertical bars represent ± SD for the means of three samples.
The contents of photosynthetic pigments (Chl a, Chl c, and Car) of the thalli that had adapted to different light and nitrogen conditions are shown in Figure 4. Light and nitrogen had no interactive effect on the contents of all three pigments (ANOVA, P = 0.671 for Chl a, P = 0.419 for Chl c, and P = 0.512 for Car). Regardless of the culture light levels, the contents of Chl a, Chl c, and Car were not affected by the nitrogen level in cultures (ANOVA, P = 0.057 for Chl a, P = 0.417 for Chl c, and P = 0.148 for Car). However, light had main effects on three pigments (ANOVA, P < 0.001 for Chl a, P < 0.001 for Chl c, and P < 0.01 for Car). In the LN or HN treatment, HL treatment reduced the contents of all three photosynthetic pigments by approximately 30−45% compared with the LL and ML conditions (Tukey’s HSD, P < 0.05), while no significant difference was observed between the LL and ML culture (Tukey’s HSD, P > 0.05).
Figure 4. Contents of Chl a, Chl c and Car in S. muticum grown at different light and nitrogen levels for 12 days. Significant (P < 0.05) differences among the treatments are indicated by different lowercase letters. Vertical bars represent ± SD for the means of three samples.
Light and nitrogen had no interactive effect on the content of soluble proteins (ANOVA, P > 0.05, Figure 5). The culture nitrogen levels had no impact on the content of soluble proteins at LL, ML, or HL treatment (ANOVA, P > 0.05). However, light had a main effect on it (ANOVA, P < 0.001). The LL culture markedly enhanced the content of soluble protein in thalli grown under LN or HN conditions (Tukey’s HSD, P < 0.05), despite no significant difference was observed between the ML and HL cultures (Tukey’s HSD, P > 0.05).
Figure 5. Content of soluble proteins in S. muticum grown at different light and nitrogen levels for 12 days. Significant (P < 0.05) differences among the treatments are indicated by different lowercase letters. Vertical bars represent ± SD for the means of three samples.
Light and nitrogen had an interactive effect on the nitrogen uptake rates of algae (ANOVA, P < 0.001, Figure 6), and each factor had a main effect (ANOVA, P < 0.001 for light; ANOVA, P < 0.001 for nitrogen). Compared with the LN treatment, HN culture increased the rate of uptake of nitrogen by 7.6 times at low light, 1.3 times in ML treatment, and 0.8 times in HL treatment. In LN cultures, ML and HL improved the rate of nitrogen uptake of algae by 3.0 and 8.5 times, respectively, compared with the LL culture, while ML and HL improved it by 9.0 and 96.0%, respectively, when cultured in HN treatment.
Figure 6. Nitrogen uptake rates of S. muticum grown at different light and nitrogen levels, which were determined during 2 days at the end of 12 days of incubation. Significant (P < 0.05) differences among the treatments are indicated by different lowercase letters. Vertical bars represent ± SD for the means of three samples.
In this study, higher light culture led to a higher RGR in S. muticum. The growth of macroalgae is closely related to algal photosynthesis (Lobban et al., 1985; Xu and Gao, 2009). Light provides the initial energy for photosynthesis, and the increase in culture light level can usually promote the photosynthesis of macroalgae by affecting electron transfer, pigment synthesis, and/or the activity of enzymes associated with carbon utilization (Xu et al., 2014; Wu et al., 2018; Dudgeon and Kübler, 2020; Eismann et al., 2020). In this study, a higher culture light level induced a greater maximum net photosynthesis rate, which indicated a higher photosynthetic capacity, and ultimately contributed to the higher growth rate of S. muticum.
The apparent photosynthetic efficiency (α) is regarded as the indication of efficiency of the alga to utilize light energy under limited light intensity (Eilers and Peeters, 1988). In this study, α showed the highest value in middle light culture of 60 μmol photons m–2 s–1 and comparatively decreased in high light culture of 300 μmol photons m–2 s–1. Moreover, the value of Fv/Fm also decreased in high light culture, suggesting that S. muticum thalli suffered from light stress in the high light culture of 300 μmol photons m–2 s–1. Such findings may be explained by the reduction of photosynthetic pigments in the high light culture compared with low and middle light culture. Algae can alter the contents of reaction center and light-harvesting pigments to adapt to different culture light intensities (Makarov, 2012). Previous studies have shown that high light intensity may reduce the photosynthetic pigment yields of algae in minutes [reviewed by Eismann et al. (2020)]. Photopigments, particularly light-harvesting pigments, usually play an important role in photoprotection and are damaged first under the high light stress, resulting in the reduction of contents (Xu and Gao, 2012; Quintano et al., 2019). However, in this study, the decrease induced by high light was not found in the growth rate and Pmax of S. muticum. The possible explanation could be that the light stress at 300 μmol photons m–2 s–1 did not damage the reaction center protein owing to the protection by its pigments, or the improved repair rate was enough to alleviate such photodamage to the reaction center (Murata et al., 2007; Zhang et al., 2020). As indirect evidence, the elevated dark respiration rate in the high light culture at 300 μmol photons m–2 s–1 is likely to provide sufficient energy to repair the damage of photosynthetic reaction center in S. muticum (Xu and Gao, 2009, 2012).
Excessive light energy generally leads to the damage of photosynthesis reaction center of algae, and such a deleterious effect is often counteracted by the protective strategies of algae, such as the accumulation of photoprotective compounds, repair mechanisms, and the removal of reactive oxygen species (Bonomi-Barufi et al., 2020; Zhang et al., 2020). In the case that damage exceeds protection, the algae will reduce their evolution of photosynthetic oxygen, which is designated “photoinhibition” (Murata et al., 2007). In this study, the irradiance saturation point of oxygen Ek was ∼300 μmol photons m–2 s–1 with no significant difference in all six treatments of different light and nitrogen levels. Beyond this irradiance point, the rate of evolution of photosynthetic oxygen of algae decreased drastically with the increase in light intensity, i.e., photoinhibition was induced in S. muticum. In contrast, many species of macroalgae have light saturation points at ∼600 μmol photons m–2 s–1 (Xu and Gao, 2012; Xu et al., 2014). Especially, S. horneri, a golden tide species, did not exhibit photoinhibition even at a light level of 600 μmol photons m–2 s–1, and it maintained enhanced photosynthetic and growth rates with the increase in light intensity (Liu et al., 2019). In the north temperate zone, which is inhabited by S. muticum and S. horneri, the daily mean irradiance of sea surface is approximately 300–800 μmol photons m–2 s–1 during the spring, summer, and autumn (Rumyantseva et al., 2019), the seasons in which golden tides have been observed. At such light levels, compared with S. horneri, drifting S. muticum is not prone to form a golden tide owing to the induction of photoinhibition.
Nitrogen is an essential nutrient for the survival and growth of algae, and an increased supply of nitrogen generally results in the promotion of growth rate in algae, owing to enhanced photosynthesis (Gao et al., 2018; Liu et al., 2019; Jiang et al., 2020). Such enhanced growth rates by N were also found in three golden tide species: S. horneri (Yu et al., 2019), S. natans and S. fluitans (Wang et al., 2019). The fact that enhanced photosynthesis induced by high nitrogen is usually attributed to an enhanced electron transfer rate, pigment content, or enzyme activity associated with carbon fixation (e.g., RUBISCO and carbonic anhydrase among others) in the photosynthetic system of algae (Giordano et al., 2005; Xu et al., 2014; Gao et al., 2018; Liu et al., 2019; Rugiu et al., 2020). In this study, high nitrogen enhanced the growth rate of S. muticum cultured at three light levels. Correspondingly, the photosynthesis of thalli was increased by culture in high nitrogen, reflected by the increased “Pmax” at low light and increased “α” at middle and high light levels. Such findings were consistent with previous reports in other species of macroalgae, including Ulva prolifera, S. horneri, Gracilariopsis lemaneiformis, Saccharina latissima, etc., (Xu et al., 2014; Liu et al., 2019; Yu et al., 2019; Jiang et al., 2020; Rugiu et al., 2020). Moreover, in the case of an adequate supply of nitrogen, the macroalgae can rapidly absorb it to supply the demand for nitrogen for growth and other physiological processes (Xu and Gao, 2012; Jiang et al., 2020), and excessive nitrogen may be stored in cells as nitrogen pools in the forms of pigments, soluble proteins, and/or amino acids (Teichberg et al., 2010; Yu et al., 2019; Jiang et al., 2020). In this study, a high supply of nitrogen induced a high rate of nitrogen uptake in S. muticum, while neither the contents of pigments nor soluble proteins were affected by the high nitrogen treatment. Therefore, we hypothesized that the greater amounts of nitrogen absorbed in the high nitrogen culture contributed to the higher rates of photosynthesis and growth in S. muticum. In addition, a higher acquisition of nitrogen may be used to improve the capability of the algae to endure high light stress (Murata et al., 2007; Xu and Gao, 2012), which was reflected by the lower “a” value of the photoinhibition term in S. muticum cultured under high nitrogen conditions.
Sargassum muticum is naturally distributed in the rocky shores of the Western Pacific North and has been colonized to the Northern European and North American coasts as an invasive species (Sánchez and Fernández, 2018; Belattmania et al., 2020). Owing to the concern that this species could form a golden tide (Álvarez-Canali et al., 2021), we investigated its physiological responses to different light and nitrogen levels in this study. Our results showed that higher light and/or nitrogen levels generally promoted the photosynthesis and growth of the algae. However, when the intensity of light exceeded 300 μmol photons m–2 s–1, its photosynthesis was inhibited, regardless of nitrogen conditions set in this study. Based on these findings, we hypothesized that the lower value of irradiance saturation point resulted in drifting individuals of S. muticum that were unable to tolerate the light intensity of sea surface on sunny days (Rumyantseva et al., 2019). Therefore, for this Sargassum species, it would not easily form a golden tide, compared with S. horneri. However, when encountered a sustained suitable light level (≤300 μmol photons m–2 s–1), it would also be possible for the drifting individuals of S. muticum to form a golden tide due to its rapid growth rate (∼8% d–1 at light level of 300 μmol photons m–2 s–1). And a high supply of nitrogen might facilitate this process.
The original contributions presented in the study are included in the article/supplementary material, further inquiries can be directed to the corresponding authors.
FY and LL conceived and designed the experiment with help from ZX and HW. FY carried out the experiment, with help from LL and DY. FY and LL performed the data analyses, with help from DY, CC, and SZ. FY wrote the manuscript with contributions from LL, DY, CC, SZ, ZX, and HW. All authors reviewed and gave their approval for the final manuscript.
This work was supported by the Shandong Provincial Natural Science Foundation of China to ZX (ZR2020MD092), HW (ZR2019MC015), and SZ (ZR2020QC025), and grants from the open project of Rongcheng Marine Industrial Technology Research Institute, the Ludong University awarded to ZX (KF20180001).
The authors declare that the research was conducted in the absence of any commercial or financial relationships that could be construed as a potential conflict of interest.
All claims expressed in this article are solely those of the authors and do not necessarily represent those of their affiliated organizations, or those of the publisher, the editors and the reviewers. Any product that may be evaluated in this article, or claim that may be made by its manufacturer, is not guaranteed or endorsed by the publisher.
Álvarez-Canali, D., Sangil, C., and Sansón, M. (2021). Fertile drifting individuals of the invasive alien Sargassum muticum (Fucales, Phaeophyceae) reach the coasts of the Canary Islands (eastern Atlantic Ocean). Aquat. Bot. 168:103322. doi: 10.1016/j.aquabot.2020.103322
Baker, P., Minzlaff, U., Schoenle, A., Schwabe, E., Hohlfeld, M., Jeuck, A., et al. (2018). Potential contribution of surface-dwelling Sargassum algae to deep-sea ecosystems in the southern North Atlantic. Deep-Sea Res. 148, 21–34. doi: 10.1016/j.dsr2.2017.10.002
Belattmania, Z., Chaouti, A., Engelen, A. H., Serrao, E. A., and Sabour, B. (2020). Spatiotemporal variation of the epifaunal assemblages associated to Sargassum muticum on the NW Atlantic coast of Morocco. Environ. Sci. Pollut. R. 27, 35501–35514. doi: 10.1007/s11356-020-09851-5
Bonomi-Barufi, J., Figueroa, F. L., Korbee, N., Momoli, M. M., and Oliveira, M. C. (2020). How macroalgae can deal with radiation variability and photoacclimation capacity: the example of Gracilaria tenuistipitata (Rhodophyta) in laboratory. Algal Res. 50:102007. doi: 10.1016/j.algal.2020.102007
Bradford, M. M. (1976). A rapid and sensitive method for the quantitation of microgram quantities of protein utilizing the principle of protein-dye binding. Anal. Biochem. 72, 248–254. doi: 10.1016/0003-2697(76)90527-3
Brooks, M. T., Coles, V. J., Hood, R. R., and Gower, J. F. R. (2018). Factors controlling the seasonal distribution of pelagic Sargassum. Mar. Ecol. Prog. Ser. 599, 1–18. doi: 10.3354/meps12646
Cacabelos, E., Olabarria, C., Viejo, R. M., Rubal, M., Veiga, P., Incera, M., et al. (2013). Invasion of Sargassum muticum in intertidal rockpools: patterns along the Atlantic Iberian Peninsula. Mar. Environ. Res. 90, 18–26. doi: 10.1016/j.marenvres.2013.05.008
Caselle, J. E., Davis, K., and Marks, L. M. (2018). Marine management affects the invasion success of a nonnative species in a temperate reef system in California, USA. Ecol. Lett. 21, 43–53. doi: 10.1111/ele.12869
Cruz-Rivera, E., Flores-Díaz, M. C., and Hawkins, A. (2015). A fish kill coincident with dense Sargassum accumulation in a tropical bay. B. Mar. Sci. 91, 455–456. doi: 10.5343/bms.2015.1048
Dudgeon, S., and Kübler, J. E. (2020). A multistressor model of carbon acquisition regulation for macroalgae in a changing climate. Limnol. Oceanogr. 65, 2541–2555. doi: 10.1002/lno.11470
Eilers, P. H. C., and Peeters, J. C. H. (1988). A model for the relationship between light intensity and the rate of photosynthesis in phytoplankton. Ecol. Model. 42, 199–215. doi: 10.1016/0304-3800(88)90057-9
Eismann, A. I., Reis, R. P., da Silva, A. F., and Cavalcanti, D. N. (2020). Ulva spp. carotenoids: responses to environmental conditions. Algal Res. 48:101916. doi: 10.1016/j.algal.2020.101916
Engelen, A. H., Serebryakova, A., Ang, P., Britton-Simmons, K., Mineur, F., Pedersen, M. F., et al. (2015). Circumglobal invasion by the brown seaweed Sargassum muticum. Oceanogr. Mar. Biol. 53, 81–126. doi: 10.1201/b18733-4
Erin Cox, T., and Smith, C. M. (2015). Photosynthetic rapid light curves for Padina sanctaecrucis vary with irradiance, aerial exposure, and tides in Hawaii’s micro-intertidal zones. Mar. Biol. 162, 1061–1076. doi: 10.1007/s00227-015-2649-1
Gao, G., Xia, J. R., Yu, J. L., and Zeng, X. P. (2018). Physiological response of a red tide alga (Skeletonema costatum) to nitrate enrichment, with special reference to inorganic carbon acquisition. Mar. Environ. Res. 133, 15–23. doi: 10.1016/j.marenvres.2017.11.003
Giordano, M., Beardall, J., and Raven, J. A. (2005). CO2 concentrating mechanisms in algae: mechanisms, environmental modulation, and evolution. Annu. Rev. Plant Biol. 56, 99–131. doi: 10.1146/annurev.arplant.56.032604.144052
Jeffrey, S. W., and Humphrey, G. F. (1975). New spectrophotometric equations for determining chlorophylls a, b, c1 and c2 in higher plants, algae and natural phytoplankton. Biochem. Physiol. Pflanzen 167, 191–194. doi: 10.1016/S0015-3796(17)30778-3
Jiang, H., Liao, X. H., Zou, D. H., Huang, B., and Liu, Z. W. (2020). The regulations of varied carbon-nitrogen supplies to physiology and amino acid contents in Gracilariopsis lemaneiformis (Gracilariales, Rhodophyta). Algal Res. 47:101818. doi: 10.1016/j.algal.2020.101818
Kouassi, A. M., and Zika, R. G. (1992). Light-induced destruction of the absorbance property of dissolved organic matter in seawater. Toxicol. Environ. Chem. 35, 195–211. doi: 10.1080/02772249209357816
Liu, F., Liu, X. F., Wang, Y., Jin, Z., Moejes, F. W., and Sun, S. (2018). Insights on the Sargassum horneri golden tides in the Yellow Sea inferred from morphological and molecular data. Limnol. Oceanogr. 63, 1762–1773. doi: 10.1002/lno.10806
Liu, T., Ma, Z. L., Li, H., and Xu, Z. G. (2019). Effects of light intensity and nitrate level on growth and photosynthetic characteristics of Sargassum horneri. Chin. J. Ecol. 38, 762–769. doi: 10.13292/j.1000-4890.201903.026 (in Chinese with English abstract).
Liu, W., Xin, M. L., Zhou, J., Zhan, D. M., Ding, G., and Wu, H. Y. (2021). Habitat hierarchies distribution of Sargassum muticum in Lidao bay, Shandong, China based on habitat suitability index model. Chin. J. Appl. Ecol. 2, 1061–1068. doi: 10.13287/j.1001-9332.202103.034 (in Chinese with English abstract).
Lobban, C. S., Harrison, P. J., and Duncan, M. J. (1985). The Physiological Ecology of Seaweeds. New York, NY: Cambridge University Press, 29–34.
Louime, C., Fortune, J., and Gervais, G. (2017). Sargassum invasion of coastal environments: A growing concern. Amer. J. Environ. Sci. 13, 58–64. doi: 10.3844/ajessp.2017.58.64
Makarov, M. V. (2012). Adaptation of the light-harvesting complex of the Barents Sea brown seaweed Fucus vesiculosus L. to light conditions. Dokl. Biol. Sci. 442, 58–61. doi: 10.1134/S0012496612010176
Milledge, J. J., and Harvey, P. J. (2016). Golden tides: problem or golden opportunity? The valorization of Sargassum from beach inundations. J. Mar. Sci. Eng. 4, 1–19. doi: 10.3390/jmse4030060
Murata, N., Takahashi, S., Nishiyama, Y., and Allakhverdiev, S. I. (2007). Photoinhibition of photosystem II under environmental stress. BBA-Bioenergetics 1767, 414–421. doi: 10.1016/j.bbabio.2006.11.019
Parsons, T. R., Maita, Y., and Lalli, C. M. (1984). A Manual of Chemical & Biological Methods for Seawater Analysis. New York, NY: Pergamon Press, 173. doi: 10.1016/B978-0-08-030287-4.50034-7
Provasoli, L. (1968). “Media and prospects for cultivation of marine algae,” in Cultures and Collections of Algae, eds A. Watanabe and A. Hattori (Kyoto: Japanese Society for Plant Physiology), 63–75.
Qi, L., Hu, C. M., Mikelsons, K., Wang, M. H., Lance, V., Sun, S. J., et al. (2020). In search of floating algae and other organisms in global oceans and lakes. Remote Sen. Environ. 239:111659. doi: 10.1016/j.rse.2020.111659
Qi, L., Hu, C. M., Wang, M. Q., Shang, S. L., and Wilson, C. (2017). Floating algae blooms in the east China sea. Geophys. Res. Lett. 44, 11501–11509. doi: 10.1002/2017GL075525
Quintano, E., Celis-Plá, P. S. M., Martínez, B., Díez, I., Muguerza, N., Figueroa, F. L., et al. (2019). Ecophysiological responses of a threatened red alga to increased irradiance in an in situ transplant experiment. Mar. Environ. Res. 144, 166–177. doi: 10.1016/j.marenvres.2019.01.008
Rugiu, L., Hargrave, M. S., Enge, S., Sterner, M., Nylund, G. M., and Pavia, H. (2020). Kelp in IMTAs: small variations in inorganic nitrogen concentrations drive different physiological responses of Saccharina latissimi. J. Appl. Phycol. 33, 1021–1034. doi: 10.1007/s10811-020-02333-8
Rumyantseva, A., Henson, S., Martin, A., Thompson, A. F., Damerell, G. M., Kaiser, J., et al. (2019). Phytoplankton spring bloom initiation: the impact of atmospheric forcing and light in the temperate North Atlantic ocean. Prog. Oceanogr. 178:102202. doi: 10.1016/j.pocean.2019.102202
Salvaterra, T., Green, D. S., Crowe, T. P., and O’Gorman, E. J. (2013). Impacts of the invasive alga Sargassum muticum on ecosystem functioning and food web structure. Biol. Invasions 15, 2563–2576. doi: 10.1007/s10530-013-0473-4
Sánchez, I., and Fernández, C. (2018). Impact of the invasive species Sargassum muticum (Phaeophyta) on tide pool macroalgal assemblages of northern Spain. Estuar. Coast. Shelf S. 210, 45–54. doi: 10.1016/j.ecss.2018.06.003
Schell, J. M., Goodwin, D. S., and Siuda, A. N. S. (2015). Recent Sargassum inundation events in the Caribbean: shipboard observations reveal dominance of a previously rare form. Oceanography 28, 8–10. doi: 10.5670/oceanog.2015.70
Sfriso, A., and Facca, C. (2013). Annual growth and environmental relationships of the invasive species Sargassum muticum and Undaria pinnatifida in the lagoon of Venice. Estuar. Coast. Shelf S. 129, 162–172. doi: 10.1016/j.ecss.2013.05.031
Smetacek, V., and Zingone, A. (2013). Green and golden seaweed tides on the rise. Nature 504, 84–88. doi: 10.1038/nature12860
Strickland, J. D. H., and Parsons, T. R. (1972). A Practical Handbook of Seawater Analysis. Ottawa: The Alger Press Ltd, 293.
Tedetti, M., and Sempéré, R. (2006). Penetration of ultraviolet radiation in the marine environment. A review. Photochem. Photobiol. 82, 389–397. doi: 10.1562/2005-11-09-IR-733
Teichberg, M., Fox, S. E., Olsen, Y. S., Valiela, I., Martinetto, P., Iribarne, O., et al. (2010). Eutrophication and macroalgal blooms in temperate and tropical coastal waters: nutrient enrichment experiments with Ulva spp. Glob. Change Biol. 16, 2624–2637. doi: 10.1111/j.1365-2486.2009.02108.x
van Tussenbroek, B. I., Arana, H. A. H., Rodríguez-Martínez, R. E., Espinoza-Avalos, J., Canizales-Flores, H. M., González-Godoy, C. E., et al. (2017). Severe impacts of brown tides caused by Sargassum spp. on near-shore Caribbean seagrass communities. Mar. Pollut. Bull. 122, 272–281. doi: 10.1016/j.marpolbul.2017.06.057
Wang, M. Q., Hu, C. M., Barnes, B. B., Mitchum, G., Lapointe, B., and Montoya, J. P. (2019). The great Atlantic Sargassum belt. Science 365, 83–87. doi: 10.1126/science.aaw7912
Williams, A., and Feagin, R. (2010). Sargassum as a natural solution to enhance dune plant growth. Environ. Manage. 46, 738–747. doi: 10.1007/s00267-010-9558-3
Wu, H. L., Gao, G., Zhong, Z. H., Li, X. S., and Xu, J. T. (2018). Physiological acclimation of the green tidal alga Ulva prolifera to a fast-changing environment. Mar. Environ. Res. 137, 1–7. doi: 10.1016/j.marenvres.2018.02.018
Wu, Y. P., Gao, K. S., Li, G., and Helbling, E. W. (2010). Seasonal impacts of solar UV radiation on the photosynthesis of phytoplankton assemblages in the coastal water of the South China Sea. Photochem. Photobiol. 86, 586–592. doi: 10.1111/j.1751-1097.2009.00694.x
Xing, Q. G., Guo, R. H., Wu, L. L., An, D. Y., Cong, M., Qin, S., et al. (2017). High-resolution satellite observations of a new hazard of golden tides caused by floating Sargassum in winter in the yellow sea. IEEE Geosci. Remote. Sens. Lett. 14, 1815–1819. doi: 10.1109/LGRS.2017.2737079
Xu, Z. G., and Gao, K. S. (2009). Impacts of UV radiation on growth and photosynthetic carbon acquisition in Gracilaria lemaneiformis (Rhodophyta) under phosphorus-limited and replete conditions. Funct. Plant Biol. 36, 1057–1064. doi: 10.1071/FP09092
Xu, Z. G., and Gao, K. S. (2012). NH4+ enrichment and UV radiation interact to affect the photosynthesis and nitrogen uptake of Gracilaria lemaneiformis (Rhodophyta). Mar. Pollut. Bull. 64, 99–105. doi: 10.1016/j.marpolbul.2011.10.016
Xu, Z. G., Wu, H. Y., Zhan, D. M., Sun, F. X., Sun, J. Z., and Wang, G. C. (2014). Combined effects of light intensity and NH4+-enrichment on growth, pigmentation, and photosynthetic performance of Ulva prolifera (Chlorophyta). Chin. J. Oceanol. Limn. 32, 1016–1023. doi: 10.1007/s00343-014-3332-y
Yakovleva, I. M., and Titlyanov, E. A. (2001). Effect of high visible and UV irradiance on subtidal Chondrus crispus: stress, photoinhibition and protective mechanisms. Aquat. Bot. 71, 47–61. doi: 10.1016/S0304-3770(01)00167-X
Yu, J., Li, J. Y., Wang, Q. H., Liu, Y., and Gong, Q. L. (2019). Growth and resource accumulation of drifting Sargassum horneri (Fucales, Phaeophyta) in response to temperature and nitrogen supply. J. Ocean U. China 18, 1216–1226. doi: 10.1007/s11802-019-3835-4
Keywords: floating macroalgae, eutrophication, photosynthesis, photoinhibition, growth
Citation: Yan F, Li L, Yu D, Cui C, Zang S, Xu Z and Wu H (2021) Physiological Responses of Sargassum muticum, a Potential Golden Tide Species, to Different Levels of Light and Nitrogen. Front. Ecol. Evol. 9:759732. doi: 10.3389/fevo.2021.759732
Received: 17 August 2021; Accepted: 08 October 2021;
Published: 01 November 2021.
Edited by:
Peng Liu, Institute of Remote Sensing and Digital Earth, Chinese Academy of Sciences (CAS), ChinaReviewed by:
Yaping Wu, Jiangsu Ocean University, ChinaCopyright © 2021 Yan, Li, Yu, Cui, Zang, Xu and Wu. This is an open-access article distributed under the terms of the Creative Commons Attribution License (CC BY). The use, distribution or reproduction in other forums is permitted, provided the original author(s) and the copyright owner(s) are credited and that the original publication in this journal is cited, in accordance with accepted academic practice. No use, distribution or reproduction is permitted which does not comply with these terms.
*Correspondence: Zhiguang Xu, Ymlnd2lkZUAxNjMuY29t; Hongyan Wu, c2R3dWhvbmd5YW5AMTI2LmNvbQ==
†These authors have contributed equally to this work and share first authorship
Disclaimer: All claims expressed in this article are solely those of the authors and do not necessarily represent those of their affiliated organizations, or those of the publisher, the editors and the reviewers. Any product that may be evaluated in this article or claim that may be made by its manufacturer is not guaranteed or endorsed by the publisher.
Research integrity at Frontiers
Learn more about the work of our research integrity team to safeguard the quality of each article we publish.