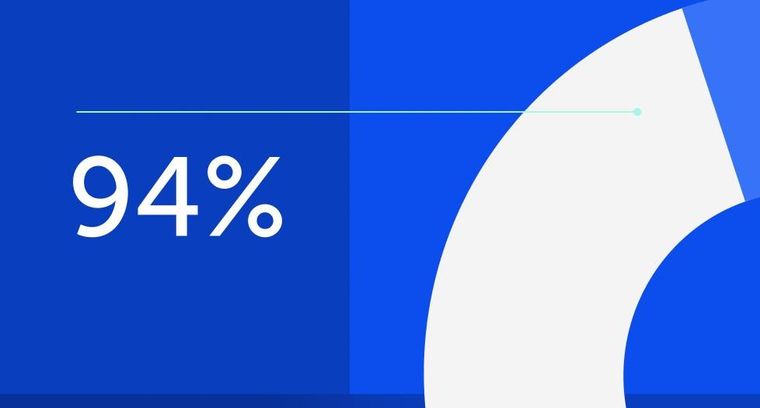
94% of researchers rate our articles as excellent or good
Learn more about the work of our research integrity team to safeguard the quality of each article we publish.
Find out more
HYPOTHESIS AND THEORY article
Front. Ecol. Evol., 06 December 2021
Sec. Population, Community, and Ecosystem Dynamics
Volume 9 - 2021 | https://doi.org/10.3389/fevo.2021.752110
This article is part of the Research TopicAnimal Seed Dispersal: An Ecosystem Service in CrisisView all 10 articles
Phenological shifts are a widely studied consequence of climate change. Little is known, however, about certain critical phenological events, nor about mechanistic links between shifts in different life-history stages of the same organism. Among angiosperms, flowering times have been observed to advance with climate change, but, whether fruiting times shift as a direct consequence of shifting flowering times, or respond differently or not at all to climate change, is poorly understood. Yet, shifts in fruiting could alter species interactions, including by disrupting seed dispersal mutualisms. In the absence of long-term data on fruiting phenology, but given extensive data on flowering, we argue that an understanding of whether flowering and fruiting are tightly linked or respond independently to environmental change can significantly advance our understanding of how fruiting phenologies will respond to warming climates. Through a case study of biotically and abiotically dispersed plants, we present evidence for a potential functional link between the timing of flowering and fruiting. We then propose general mechanisms for how flowering and fruiting life history stages could be functionally linked or independently driven by external factors, and we use our case study species and phenological responses to distinguish among proposed mechanisms in a real-world framework. Finally, we identify research directions that could elucidate which of these mechanisms drive the timing between subsequent life stages. Understanding how fruiting phenology is altered by climate change is essential for all plant species but is particularly critical to sustaining the large numbers of plant species that rely on animal-mediated dispersal, as well as the animals that rely on fruit for sustenance.
Phenological shifts are among the most visible ecological effects of global climate change. Research on individual species (e.g., CaraDonna et al., 2014), meta-analyses (Root et al., 2003; Munguía-Rosas et al., 2011), and community-wide taxonomic surveys (Ovaskainen et al., 2013) demonstrate that phenological, or the timing of life history, events in most species and stages are advancing in response to warmer temperatures. Well-studied life history stages include migration (Mayor et al., 2017) and breeding (Burger et al., 2012) in birds, hibernation in mammals (Sheriff et al., 2011), adult emergence in insects (Bartomeus et al., 2011; Renner and Zohner, 2018), and green-up and flowering in plants (Calinger et al., 2013; Rafferty and Nabity, 2017; Ettinger et al., 2018). In contrast, other life history stages, such as fruiting, have received relatively little attention (Chuine and Régnière, 2017; Mendoza et al., 2017; Ettinger et al., 2018). An important gap in our understanding concerns whether the latter of successive events in the life histories of an organism (e.g., fruiting which follows flowering) are shifting independently of, or are functionally constrained by, earlier stages.
It is reasonable to predict that climate-induced shifts in the timing of early life history stages result in parallel shifts in subsequent life-history stages of the same organism. The timing of life history stages is driven by a combination of external (climate) and internal (physiological or endogenous) factors. It is largely unknown, however, to what extent each factor drives the timing of one life history stage relative to earlier ones (McDermott and DeGroote, 2017; Ettinger et al., 2018; Gougherty and Gougherty, 2018; Augspurger and Zaya, 2020; Buonaiuto et al., 2021). With the exception of a few pioneering studies (e.g., Menzel et al., 2001; Post et al., 2008a,b; Haggerty and Galloway, 2011; Jiang et al., 2016; Segrestin et al., 2018), little research has yet explored whether successive life history stages predictably shift in concert with each other in response to climate change, nor, when they do, what drivers underlie this relationship (Chuine and Régnière, 2017; Ettinger et al., 2018). Parallel shifts might occur either if the interval between successive life-history events is constant or nearly so (likely a product of internal factors such as physiology or development), or if the external, proximate climatic cues for the two events are the same or tightly associated. Parallel shifts in successive life history stages could be related to reproductive strategy and traits (Forrest and Miller-Rushing, 2010; Ettinger et al., 2018; reviewed in Gougherty and Gougherty, 2018; Buonaiuto et al., 2021), genetics and selective processes (Crozier et al., 2008; Wilczek et al., 2010), and temporal boundaries on the growing or breeding season (Morales et al., 2005). Alternatively, successive life history stages could respond to different climatic conditions (Kingsolver et al., 2011), resulting in a changing interval between them as the climate changes (Lany et al., 2016). Determining which internal or external mechanisms are acting on the timing of life history events should offer critical predictive insights into whether and how climate change will affect the persistence not only of individual taxa, but also of the interactions among them.
Perhaps the most thoroughly documented phenological shift in response to climate change involves flowering. Advances in flowering time (conventionally noted by opening of flowers on an earlier date) have been found both across communities and within them, and contrasting responses across regions and taxa have been explored in some depth (e.g., Fitter and Fitter, 2002; Root et al., 2003; Miller-Rushing and Primack, 2008; CaraDonna et al., 2014; Rafferty and Nabity, 2017). Here, we use this wealth of knowledge on flowering phenology to explore whether subsequent life stages are linked, and if so, how they are linked. Although several investigators have speculated about whether advances in flowering time are accompanied by parallel shifts in fruiting time (Primack, 1987; Eriksson and Ehrlén, 1991; Forrest and Miller-Rushing, 2010), there are as yet few tests of these ideas (Chmielewski et al., 2004; Sherry et al., 2007; Segrestin et al., 2018). As a consequence, the response of fruiting phenologies to a changing climate remains poorly understood (Chuine and Régnière, 2017).
Experimental warming studies have shown that with higher temperatures, most species fruit earlier in the season (Sherry et al., 2007; Post et al., 2008a,b). However, observational studies of fruiting phenology, particularly those using datasets that span decades, vastly lag behind the number published for flowering. One possible reason for the focus on flowering phenology over fruiting is that assigning a date to fruiting is less straightforward because fruit morphology is more diverse across taxa than flower morphology, and stages of fruit development are less easily identified through observation. We follow convention of previous studies and, unless otherwise noted, use “fruiting” or “fruiting time” to denote the first date on which the presence of mature fruits or seeds is observed (e.g., Gordo and Sanz, 2009; Haggerty and Galloway, 2011; Ettinger et al., 2018), and, in our discussions of published studies and within the case study we present, we compare across taxa with different fruiting structures (e.g., fleshy, dry, indehiscent, dehiscent, animal-dispersed, and wind-dispersed; Menzel et al., 2006, 2020; Ge et al., 2015).
Here, we explore promising pathways for advancing understanding of which and how fruiting times are linked to flowering times. In animal-dispersed plants, shifts in fruiting phenology have the potential to affect or even disrupt seed-dispersal interactions (Forrest, 2014; Rafferty et al., 2015). Thus, an understanding of shifting fruiting phenologies is important to our ability to predict the effects of global change on plant-animal communities (Rogers et al., 2021). We incorporate plants that are animal-dispersed as well as those that employ abiotic seed dispersal, but we focus on the interaction and community repercussions for animal-dispersed plants. First, we synthesize current understanding of the linkages between flowering and fruiting stages across angiosperms. Second, to ground our discussion of life history stage linkages in a real-world framework, we report on a case study designed to determine how the flowering and fruiting times of individuals tracked over multiple decades at the same location are linked. Third, we leverage our extensive knowledge of climate-mediated shifts in flowering phenology to develop a conceptual model for how successive life history stages are linked and how climate change could affect these linkages. We then return to our case study results to demonstrate how to distinguish and eliminate proposed mechanisms for these linkages. Finally, we suggest directions for future research to test these mechanisms, and discuss the implications of climate change-driven shifts in fruiting phenology for ecological communities.
Few studies have determined the extent to which flowering and fruiting phenology are linked or physiologically constrained. We argue here that examining the flowering-to-fruiting interval can provide insight. We define the flowering-to-fruiting interval (hereafter, FTFI) as the period from flower opening to the first date on which the presence of mature fruits or seeds is observed. The FTFI is “constrained” if it remains fixed in length, including within warming experiments or in a changing climate. The FTFI is a trait defined at the level of the reproductive structure; i.e., it is the length of time from flowering to fruiting measured on a single flower developing into a fruit on an individual plant. In order to make use of historical datasets in which observations were not collected at this level, most previous studies have largely used population- or species-level measurements, with a few examples of individual-level measurements (Table 1). If flowering and fruiting are linked, internal drivers will dictate that fruiting times are related to flowering times as well as to the FTFI. If external factors are instead driving phenology, the FTFI could lengthen or shorten as flowering and fruiting respond to these external factors independently. If flowering and fruiting are responding independently to the same external factors, or if by chance these two life stages are responding in similar ways to different independent factors, the FTFI will stay the same.
Table 1. Evidence from previous observational and experimental studies on the length of the flowering to fruiting interval (FTFI), the time between the flowering and ripe fruit phenophases, and for external and internal drivers of FTFI duration.
The few previous studies of how flowering and fruiting are linked provide evidence for internal drivers, but also evidence for independent responses of flowering and fruiting to external factors (Post et al., 2008a,b; Ettinger et al., 2018; Segrestin et al., 2018; Table 1). Studies that present evidence for external factors independently driving fruiting and flowering times often show some evidence for internal drivers as well (Lechowicz, 1995; Menzel et al., 2006, 2020; Haggerty and Galloway, 2011; Jiang et al., 2016; Sethi et al., 2020; Table 1). Table 1 synthesizes the results of these previous studies and the mixed evidence for internal and external drivers of flowering and fruiting. An early study of the FTFI based on a nearly 30-year dataset found no evidence for a linear relationship between flowering and fruiting dates across many temperate species, even when grouped by time of season in which fruiting occurs (Lechowicz, 1995). In contrast, a more recent single-year study did find evidence for this linear relationship in 25 U.S. species (Ettinger et al., 2018), as did a 12-year study of 100 European species (Segrestin et al., 2018). Even concurrent studies on the same species have produced mixed evidence for internal and external drivers of the FTFI. A 1-year warming experiment revealed that higher temperatures shortened the entire reproductive cycle of Betula nana by 27 days on average, from flower bud set to fruit set (Post et al., 2008b), thereby also shortening the FTFI. However, a concurrent 2-year warming experiment showed that higher temperatures did not decrease the FTFI in B. nana (Post et al., 2008a), even though flowering and fruiting were advanced.
Other experimental studies have yielded evidence for both internal and external drivers of the FTFI. Alpine plants transplanted to warmer and cooler altitudes for 3 years flowered earlier and later, respectively, but fruiting times remained the same (Jiang et al., 2016). These results support external drivers of the FTFI, but a different elevation transplant study (Haggerty and Galloway, 2011) showed evidence for both external and internal drivers: in a single-year common garden experiment, Campanulastrum americanum populations planted at lower elevations had a shortened FTFI relative to those planted at higher elevations. However, populations from low elevations planted at either elevation had a longer reproductive cycle overall than those from high elevations (Haggerty and Galloway, 2011).
Observational studies have similarly yielded mixed evidence. A recent observational study on 28 species of U.S. alpine plants showed mostly shortened FTFI with climate change, comparing observations taken in 2015 to those taken in 2011–2014 (Sethi et al., 2020). Shorter FTFI were shorter by 3–15 days, but two species showed an FTFI change of a day or less, and the FTFI of three species increased by 3–5 days (Sethi et al., 2020). Menzel et al. (2006) used phenological records to determine (1) how timing of life history stages was changing in 14 European countries over 30 years and (2) how these phenological changes correlated with temperature across 9 European countries over 15 years. While flowering time was largely negatively correlated with temperature, fruiting time was more variable: the correlation with temperature was negative for most species, but positive for a few others. A recent update of this study incorporating data on the same European species from 1951 to 2018 showed the same pattern (Menzel et al., 2020). As temperature increases and flowering times advance, Menzel et al.’s (2006, 2020) findings imply that the FTFI will shorten for some species, remain the same for some, and lengthen for others, depending on how internal and external factors interact to determine fruiting times.
Nearly all of the few studies that directly analyze the FTFI do so on the scale of a year or a few years, and in the case of multi-year analyses, only for a single species (Table 1). Publicly available fruiting data at time scales longer than 20 years are particularly sparse (Chuine and Régnière, 2017; see Supplementary Appendix 1 for descriptions of publicly available phenology databases). Yet, time series > 30 years are needed to estimate robust trends (Dose and Menzel, 2004; Menzel et al., 2020). Here, we use a three-and-a-half-decade dataset (from the PEP725 database; Templ et al., 2018) to assess how the FTFI is changing in individual plants. Our case study both illustrates the challenges of analyzing the phenology of certain life history stages on a decadal scale using publicly available data. At the same time, it provides compelling evidence that such data, when available, can be used to analyze changes to the length of time between phenophases with climate change. We do not present here a comprehensive treatment of FTFI across species. Although more long-term data are needed to assess changes in the FTFI across diverse taxa and regions (Mendoza et al., 2017; Supplementary Appendix 1), we use this case study to illustrate the potential power of analyzing relationships between the timing of different life history stages.
We examined whether a close temporal link exists between the flowering and fruiting life stages, and assessed the strength of this linkage. To determine how the FTFI has changed over time, we used phenology data from the PEP725 database (Templ et al., 2018) over 35 years (1980–2015). The FTFI is defined at the level of a single reproductive structure on an individual plant. Our data did not allow for this level of precision so instead we used the next most precise level, that of the individual. We used all six tree and shrub species with > 100 individuals per year for which both first flowering and first ripe fruits were recorded in the database: Aesculus hippocastanum, Sorbus aucuparia, Vaccinium myrtillus, Sambucus nigra, Ribes grossularia, and Ribes rubrum. These species represent both animal-dispersed (Vaccinium myrtillus, Sambucus nigra, Ribes grossularia, and Ribes rubrum) and wind-dispersed (Aesculus hippocastanum and Sorbus aucuparia) species; given that we had access to data on only a few species, we were unable to compare FTFI responses in biotically vs. abiotically dispersed species. We calculated the FTFI by subtracting the first flowering day from the first day at which ripe fruits were noted, for each individual within each species for each year. Number of days between flowering and fruiting became our response variable for a single multi-species analysis with a Bayesian regression framework (for analysis details see Supplementary Appendix 2: Methods 2).
The FTFI remained constant over time in three of the six species: Sorbus aucuparia, Vaccinium myrtillus, and Sambucus nigra. The other three species experienced either a significant increase (Aesculus hippocastanum) or significant decrease (Ribes rubrum and Ribes grossularia) in FTFI over time (Figure 1). If a close linkage exists, we would expect no change in FTFI. If, however, a close linkage does not exist because flowering and fruiting are responding to different external cues and a changing climate independently, the result could be a shortening or lengthening or lack of change in the interval between flowering and fruiting. The divergent results between species suggests that a range of mechanisms might be determining the role of climate change on the FTFI.
Figure 1. Mean phenological changes in the flowering to fruiting interval (FTFI; top) and fruiting time (bottom) of 6 and 14 European trees and shrubs, respectively, from 1980 to 2005. Mean changes are represented with dots and 95% credible intervals are represented with bars. Species names for which the FTFI or fruiting time changed significantly are starred and in dark gray. Species names for which the FTFI or fruiting time did not significantly change are in green. Across-species mean of the shift in fruiting time (–4.2 days/decade) is denoted by the red dotted line (bottom) with the 95% credible interval in orange.
The magnitude of a shift in fruiting phenology for a species, and in which direction this shift occurs, can provide additional information about the mechanism driving the FTFI. To determine whether or not fruiting alone was advancing over the same time period as our FTFI analysis, we performed an additional analysis on fruiting for the same 6 plus an additional 8 European species. We analyzed fruiting at the level of the population, to be consistent with previous analyses of fruiting, which allowed us to include species that did not have enough individual observations spanning multiple years to be in the FTFI analysis. The incorporation of these additional 8 species allowed us to more broadly compare the results of our fruiting analysis to previous studies. We selected native, broad-leaved woody plant species that had records that occurred consistently from 1980 to 2015 in one location (for additional methods see Supplementary Appendix 2: Methods 1).
We found that fruiting had advanced by an average of 4.2 days per decade (days/decade; 95% CI: 2.4–6.2 days/decade, Figure 1), or 14.7 days from 1980 to 2015 (for additional results see Supplementary Appendix 2: Results 1). The 4.2 days/decade advancement in fruiting in our species is consistent with Ge et al.’s (2015) meta-analysis of 104 Chinese tree, shrub, and herb species, in which spring/summer phenophases, including fruiting, advanced by an average of approximately 2 days for trees and shrubs and 5.5 days/decade for herbs from 1960 to 2011. It is also consistent with Gordo and Sanz’s (2009) findings of 3.2 days/decade for 29 perennial Spanish species from 1943 to 2003. It is likewise consistent with Menzel et al.’s (2006) findings of 2.4 days/decade for 542 European species from 1971 to 2000 and 1–2.5 days/decade, depending on the season of fruiting, for the same species from 1951 to 2018 (Menzel et al., 2020). Collectively, these findings highlight the need to conduct similar, long-term, studies of how fruiting phenology is responding to climate change.
Fruiting advanced for all of the six species in our FTFI analysis (Figure 1). The species in which fruiting advanced the most were also the species for which the FTFI remained constant. Vaccinium myrtillus, Sambucus nigra, and Sorbus aucuparia advanced fruiting by an average of 9.0, 6.0, and 5.2 days/decade, respectively, while their FTFI did not significantly change. For Ribes rubrum and R. grossularia, fruiting advanced by an average of 4.3 and 3.8 days/decade while the FTFI shortened by a mean of 1.0 and 0.9 days/decade, respectively. Fruiting in Aesculus hippocastanum advanced by 2.1 days/decade, while the FTFI lengthened by a mean of 2.0 days/decade. The similar responses of fruiting in these six species but differing responses of the FTFI suggest that separate mechanisms may be operating for each species.
If the timing between flowering and fruiting is physiologically constrained, a shift in timing in flowering should have a direct effect on the timing of fruiting. This could result in flowering and fruiting times advancing in parallel, as in three of the six species in our case study. However, we cannot rule out the possibility that separate, possibly correlated external factors are acting on each of the life history stages. Furthermore, external factors could act on flowering and fruiting separately or jointly, such that the FTFI lengthens or shortens, as seen in the other three species in our case study. Below we propose four general mechanisms for how flowering and fruiting times may jointly or independently shift with climate change. While the specifics of these mechanisms can relate more strongly to one seed dispersal type than another, as noted below, all four mechanisms apply to plants with either biotic or abiotic seed dispersal. We additionally recognize that multiple mechanisms could be operating at once.
The FTFI will always be somewhat variable across individuals and reproductive structures for any given species, but internal constraints such as developmental processes or seed and fruit size should impose limits on the variability possible for this interval and result in parallel shifts in flowering and fruiting, as shown in Table 2 and Figure 2. Certain fruit or seed traits such as size could be correlated with, and could possibly dictate, the length of the fruit development and maturation periods (Primack, 1987; Segrestin et al., 2018). For example, animal-dispersed seeds, which are on average larger than wind-dispersed seeds, have a physiologically constrained lower limit on seed development time that is greater than that of smaller seeds (Primack, 1987), and a longer FTFI on average than wind-dispersed seeds (Segrestin et al., 2018). The minimum developmental time for fruits theoretically constrains the FTFI, regardless of external environmental effects like drought (Gordo and Sanz, 2010; Segrestin et al., 2018).
Table 2. Proposed mechanisms for how the flowering to fruiting interval (FTFI), the time between the flowering and ripe fruit phenophases, will change or remain stable with climate change.
Figure 2. Each proposed general mechanism (column 1) is expected to result in changes to the FTFI (column 3), based on the relationship between flowering and fruiting time (column 2) of single reproductive structures on single individual plants (dots). An internal mechanism results in a tight correlation between flowering and fruiting (Mechanism 1), whereas an external mechanism results in no clear relationship (Mechanisms 2–4). Although Mechanisms 3 and 4 are driven by external processes, these processes could by chance result in a relationship between flowering and fruiting that appears tightly correlated like an internal mechanism. An internal mechanism results in a shift of the FTFI from historical (top of column 3), but the length of the FTFI is preserved. External mechanisms result in several possible changes to the FTFI from historical wherein timing of flowering, fruiting, or both are altered. Bars represent the beginning of the flowering stage (yellow) to the fruit maturity stage (red) for a single reproductive structure on a single individual. Advanced flowering is represented by the dashed line labeled as earlier in year, and delayed fruiting is represented by the dashed line labeled as later in year. Historical flowering and fruiting times are represented by the two central dashed lines. All possible options for changes to fruiting, given advanced flowering currently and in the future, are displayed for each mechanism.
Similarly, seed mass and the amount of time that fruits need from flowering to ripening are phylogenetically constrained and positively correlated (Heydel and Tackenberg, 2016), suggesting that certain evolutionary histories dictate fruit development and maturation periods. Plants with life history stages such as leaf-out and flowering that occur earlier in the spring display traits that are a product of rapid growth, which may translate to a shorter FTFI, resulting in smaller seeds or fruits due to the shorter development time (Wolkovich and Cleland, 2014). If phenology is phylogenetically constrained, we would expect the FTFI to be phylogenetically constrained. Studies have found phylogenetic signals in leaf-out, flowering, and fruiting phenology, but their strengths are highly variable, with some clades exhibiting a stronger pattern than others (Marco and Páez, 2002; Wolkovich and Ettinger, 2014; Gougherty and Gougherty, 2018). While this signal seems to indicate phylogenetic constraints, it may instead be that phenology is correlated with other plant traits that are themselves evolutionarily conserved (Marco and Páez, 2002; Davis et al., 2010; Wolkovich and Ettinger, 2014; Gougherty and Gougherty, 2018).
Stabilizing selection could be indirectly acting on fruiting times by promoting genotypes linked to a particular disperser-fruiting time relationship (e.g., Palacio et al., 2021) or climate-fruiting time (e.g., Inouye et al., 2019). If physiological and developmental constraints were keeping the FTFI the same length and stabilizing selection via an external driver were maintaining fruiting times, we would expect to see both unchanging fruiting times and unchanging flowering times in the face of climate change. However, we know that flowering time is largely advancing; therefore, we would expect stable fruiting times and thus lengthened FTFIs if fruiting time were driven by stabilizing selection (Table 2 and Figure 2). When a fruit reaches maturity could be tightly associated with the historical activity of seed dispersers (e.g., bird-dispersed fruits, Noma and Yumoto, 1997) and could remain constant, even though the timing of disperser activity is itself shifting with climate change (e.g., in birds, Thomas and Lennon, 1999; Cotton, 2003; Marra et al., 2005; Tingley et al., 2009).
The second way in which stabilizing selection could be acting is if the fruiting time-climate relationship evolved in response to an abiotic driver that is unlikely to be affected in a direction predictable by climate change, such as solar irradiance and photoperiod (Hamann, 2004; Chapman et al., 2005; Zimmerman et al., 2007; Mendoza et al., 2017; Ettinger et al., 2021) or the onset of monsoon rains (Singh and Kushwaha, 2006). If fruit ripening times are synchronized by a biotic or abiotic event that does not significantly shift over time with climate change, whereas flowering phenology is responsive to shifting environmental cues, an increase or decrease in the length of the interval could result (Table 2 and Figure 2).
The genetic underpinnings of flowering phenology, and less so fruiting phenology, in response to temperature have been well-studied in certain species, most of which are commercially grown (e.g., Usenik and Štampar, 2011; Satake et al., 2013; Marrano et al., 2019; Bernard et al., 2020; Chen et al., 2020). These studies show diverse responses of life history stages to climate change between closely related species and even individuals of the same species, and suggest that the underlying genetic differences influencing these life history stages dictate whether or not the FTFI is altered by climate change. In contrast, there is poor understanding of the genetics of fruiting phenology in wild species, and experiments on intraspecific genetic variation interacting with climate change-induced environmental conditions are rare (e.g., Faticov et al., 2020). Most of our understanding is based on observational and experimental studies of plant responses to temperature and precipitation without knowledge of the underlying genetic architecture, which suggest either selection favoring genotypes that are able to fruit earlier in the growing season, or plastic phenological responses to temperature and precipitation. In conjunction with flowering responses to climate change, we can make inferences about how selection on or plasticity of fruiting times will affect the flowering to fruiting interval (Table 2 and Figure 2). In multiple studies, higher temperatures generally advanced flowering and advanced fruiting by a greater magnitude than flowering, which shortened the FTFI (Sherry et al., 2007; Misson et al., 2010; Haggerty and Galloway, 2011; Gallinat et al., 2015; Carbognani et al., 2018), but in some U.S. and European species, fruiting advanced less than flowering, resulting in a longer FTFI (Sherry et al., 2007; Gordo and Sanz, 2009). A temperature-based modeling framework for flowering phenology (e.g., Chuine et al., 1999) extended to the FTFI largely predicts a shorter FTFI (Darbyshire et al., 2014).
Both increased and decreased precipitation have led to variable effects on FTFI in observational and experimental studies (Peñuelas et al., 2004; Sherry et al., 2007; Mazer et al., 2015; Dunham et al., 2018; Table 2 and Figure 2). Doubling precipitation had no effect on fruiting times and FTFI in a controlled experiment (Sherry et al., 2007). In three studies that did not track the FTFI, increased precipitation resulted in delayed fruiting (Peñuelas et al., 2004; Mazer et al., 2015) as did decreased precipitation during the dry season (Dunham et al., 2018). If we assume that most species in these studies experienced advanced flowering, we can then assume that FTFI has lengthened as a consequence. However, more studies on the effects of precipitation generally are needed, especially because studies on the effects of decreased precipitation on fruiting specifically are lacking (but see Sethi et al., 2020).
As previously stated, many of the responses of the FTFI to climate listed under Mechanism 3 could have been due to phenotypic plasticity in response to environmental conditions, since genetic responses were not tracked. Segrestin et al. (2018) found that across plant species, the onset of fruiting was more variable than the onset of flowering, perhaps indicating that the timing of fruiting is more plastic. Warmer air temperatures and earlier snow melt were found to be correlated with reductions in the FTFI of alpine plants (Sethi et al., 2020), indicating a plastic response to these environmental changes. Phenotypic plasticity could allow the FTFI to lengthen, shorten, or remain the same in response to biotic interactions or a variety of environmental conditions, including flowering and fruiting phenologies responding independently to these drivers (Table 2 and Figure 2).
Plasticity in flower longevity, i.e., how long an individual flower is open, is another way in which plants could respond to environmental conditions and might result in a change to the FTFI. Some species can show variable flower longevity in response to pollen receipt, wherein the flower closes anywhere from a few hours to a few days after successful pollination (Primack, 1985; Proctor and Harder, 1995; van Doorn, 1997; Niu et al., 2011; Trunschke and Stöcklin, 2017). The time from pollination to fruiting could thus be fixed by physiological and developmental constraints on fruit development, as discussed above, but the FTFI may instead reflect the time from flower opening to pollination (Segrestin et al., 2018). If a species’ flowering time becomes partially mismatched from pollinator availability, time to pollination could lengthen, resulting in a longer FTFI (Table 2 and Figure 2).
Flower longevity, and thus the FTFI, could also be influenced by environmental factors such as warmer or cooler temperatures (Arroyo et al., 1981; Molau, 1997; Steinacher and Wagner, 2010; Nagahama et al., 2018) or increased precipitation (Moore and Lauenroth, 2017), resulting in a shortened or lengthened FTFI (Table 2 and Figure 2). For example, Nagahama et al. (2018) found that climate warming causes flowers to senesce earlier, which could result in a shorter FTFI. Flower longevity increases due to lower temperatures (Arroyo et al., 1981), meaning that advanced flowering could result in flowers periodically experiencing lower than normal temperatures, which could result in longer flower longevity and thus a lengthened FTFI. Increased precipitation resulted in increased flower longevity for late-season species (Moore and Lauenroth, 2017), indicating that changes to precipitation in either direction could also alter flower longevity, and in turn, the FTFI.
A third way in which environmental conditions could influence the FTFI is through resource acquisition pathways. When a fruit matures may depend on how quickly a plant is able to acquire resources from photosynthesis, resulting in a shorter or longer FTFI. Resource acquisition via photosynthesis, in turn, can be impacted by an array of factors (Table 2 and Figure 2). Warmer temperatures could result in fewer leaves damaged by frost, allowing plants to acquire resources more quickly, thus advancing fruiting times and shortening the FTFI (Chuine and Beaubien, 2001; Guillaume et al., 2018). Alternately, warmer temperatures could result in earlier leaf-out times, which could lead to greater frost damage of leaves, leading to a slower acquisition of resources, thus delaying fruiting times and lengthening the FTFI (Guillaume et al., 2018). Changing climate and the timing of insect emergence or population booms could either result in decreased or increased herbivore damage, leading to faster or slower acquisition of resources, respectively (Klapwijk et al., 2013). Lastly, with warmer temperatures, plants could produce more leaf mass or area, or thicker leaves, increasing photosynthetic capacity and leading to a faster acquisition of resources and advanced fruiting times (Tripathi et al., 2016).
Several of the mechanisms proposed above for how fruiting and flowering phenology respond jointly or independently to climate change could produce identical results in the FTFI over time (Table 2 and Figure 2). Here we return to our case study to demonstrate how we could use the FTFI in combination with additional research to tease apart the mechanisms behind changes, or lack thereof, to the FTFI (Figure 3). For example, an absence of change in the FTFI, such as we found in three of our six species (Figure 1), indicates that the mechanism driving the FTFI for these species could be internal. Stabilizing selection is expected to produce a change in the FTFI, so that mechanism can be discarded. Constancy in the FTFI is consistent with Mechanisms 3, directional selection, and 4, environmental controls. The mechanism of environmental controls is difficult to rule out because, for example, an incremental increase in temperature across many years could have an equally strong correlation with fruiting as flowering has with fruiting. If, over many years and across yearly temperature and/or precipitation fluctuations, fruiting time is more strongly correlated with flowering time than with environmental cues, we could rule out environmental controls. The other processes affecting environmental controls are either difficult to track or require vastly more data. If length of flowering time, specifically from flower opening to pollination, does not affect fruiting time and thus FTFI, environmental controls via flower longevity could be ruled out. If any of the processes related to the acquisition of resources, like frost or herbivore damage to leaves, are not correlated with FTFI, we can rule out environmental controls. However, collecting data to discriminate multiple processes related to environmental controls is a massive undertaking. This will make discerning the importance of environmental controls via resource acquisition difficult. If we find no genetic evidence for directional selection on fruiting times (e.g., see Giménez-Benavides et al., 2011; Munguía-Rosas et al., 2011; Anderson et al., 2012; Yeoh et al., 2017, for studies on flowering), or if we find no fitness benefits for those individuals for which the FTFI remains constant, we can rule out directional selection.
Figure 3. Framework for using the observed changes, or lack thereof, in the flower to fruiting interval (FTFI) over time along with additional data and analyses to distinguish the underlying mechanism driving the FTFI. This flowchart only illustrates how the four general mechanisms can be distinguished. Ways to tease apart and eliminate the pathways through which each general mechanism could operate is described within the main text. The order shown here in which mechanisms can be progressively eliminated is suggested; there are multiple approaches to eliminating the possible mechanisms driving the FTFI. For example, directional selection could be eliminated after environmental controls or before stabilizing selection for the absence of a change or a lengthening or shortening of the FTFI, respectively.
Ruling out stabilizing selection, directional selection, and environmental controls leaves only physiological and developmental constraints as mechanisms producing a constant FTFI over time and in the face of climate change. Comparing the FTFI among species within the same genus and to species of genera with similar fruit or seed sizes could point to whether phylogenetic or developmental constraints are operating. Accounting for phylogenetic signal in the analysis of changes in FTFI across multiple years, species, and continents (i.e., Davis et al., 2010 for flowering) could point to the relative influence of evolutionary history on phenological responses to changing climate. However, none of these approaches permits elimination of either pathway through which the internal mechanism could be operating.
One of the six species in our case study, Aesculus hippocastanum, experienced an increase in FTFI over time (Figure 1). We expect physiological and developmental constraints to result in a constant FTFI over time, suggesting that the other three mechanisms we have proposed—stabilizing selection, directional selection, or environmental controls—could be responsible. Stabilizing selection could be acting if the time of first ripe fruits remains constant. However, for A. hippocastanum the timing of fruiting shifted earlier (Figure 1). Another indication of stabilizing selection might be fruit ripening that remains constant in reference to an abiotic or biotic event. If we found no correlation between fruiting times and abiotic or biotic events, or no genetic evidence for stabilizing selection on fruiting times, we could rule out this mechanism. Furthermore, if we found no genetic evidence of directional selection on fruiting times, or if we found no fitness benefits for those individuals for which the FTFI increases, we could rule out directional selection. If flower longevity was driving the lengthening of the FTFI by an increased amount of time between flower opening (the beginning of the FTFI, as defined above) and flower closing, we would expect to observe a lengthening of the first flowering to flower senescence interval over multiple years. We would also potentially expect to see a strong correlation between date of pollination and first ripe fruit dates. If neither this correlation nor a correlation between pollination and first ripe fruits were found, environmental controls via flower longevity could be rejected. If both flowering and fruiting were correlated with the same or independent environmental conditions, with no evidence for selection on either, environmental controls might be driving the increased FTFI. However, correlations with environmental conditions may be direct (e.g., temperature and/or precipitation acting directly on flowering and fruiting) or indirect, via resource acquisition. A number of pathways that affect photosynthesis could be involved in this indirect relationship, and teasing them apart would require extensive data collection in, for example, leaf damage and cloudless days.
Two of the six species represented in this case study experienced a decreased FTFI (Figure 1), again suggesting that stabilizing selection, directional selection, or environmental controls could be responsible. As with A. hippocastanum, in Ribes rubrum, and R. grossularia fruiting occurred earlier (Figure 1), indicating that stabilizing selection could only be acting if fruit ripening time remained constant in reference to an unknown abiotic or biotic event. The same process of eliminating mechanisms just described for A. hippocastanum could be applied to these Ribes species.
Throughout this paper, we have stressed that additional research is needed to further distinguish which mechanisms are driving the FTFI, and through what pathways. Here we discuss research directions for internal drivers of the FTFI, how genetics and selection influence the FTFI and will determine future fruiting responses to climate change, and the interactions among different environmental controls and how these affect fruiting both now and with climate change. Although different species may be driven by different mechanisms, and all of the mechanisms discussed here may be operational in nature, simultaneous, standardized studies of multiple species could provide evidence for or eliminate possible mechanisms on a broad scale. We additionally emphasize that long-term studies of phenology are essential because short-term interannual variation could reflect phenological plasticity rather than evolutionary change.
Further research could elucidate how physiological mechanisms determine the FTFI, and to what extent different mechanisms co-occur and can interact. Taking a trait-based approach to determine differences in seed and fruit development time across the range of seed and fruit traits could advance our understanding of the extent to which the time of fruit development is constrained, or varies, along trait axes (e.g., Singh and Kushwaha, 2006). Determining which families or clades have long fruit or seed development times closely linked to the number of growing degree days within a season could shed light on the extent to which phylogenetic constraints work in concert with environmental controls to determine the length of the fruit development period. Similarly, studies that compare the relative influence of evolutionary history and environmental controls could tease apart the extent to which internal vs. external process are driving phenology (e.g., Staggemeier et al., 2015), particularly with the incorporation of phenological shifts due to climate change (Davis et al., 2010). We suggest incorporating standardized FTFI measures into existing phenological data collection protocols so as to more accurately compare across datasets, as Buonaiuto et al. (2021) have suggested for the interval between flowering and leaf-out.
If fruit or seed dispersal mode does not predict the strength of selection on fruit ripening times (e.g., Schluter, 1988; Kingsolver et al., 2001; Palacio et al., 2021), it is less likely that stabilizing selection is acting on fruit ripening times across many species. Additionally, if peak activity of seed dispersers and fruit ripening times are shifting and becoming out of phase with climate change, instead of shifting in parallel, biotic interactions driving stabilizing selection in animal-dispersed fruits can be discarded as a general pattern. To determine how peak activity of dispersers and fruit ripening times are responding to each other and climate change, more long-term studies that track disperser phenology along with fruiting phenology, particularly in the tropics where biotic interactions are assumed to play a greater role than climatic factors, are sorely needed (Mendoza et al., 2017).
Mechanistic research that addresses the molecular and regulatory basis of fruit ripening in wild species would further disentangle selection for particular genes associated with ripening from changing environmental cues and altered physiological processes (Chen et al., 2020). Additionally, determining how selection is acting on the reaction norm for fruit ripening times (Inouye et al., 2019) as climate changes, and how genetic variation in wild species of genes associated with fruiting responds to changes in temperature, precipitation, and other climatic factors, would help further our understanding of how a changing environment is interacting with selective pressures. Agricultural scientists are exploring the genetic underpinnings of plant phenology (e.g., Satake et al., 2013; Marrano et al., 2019; Chen et al., 2020) and phenological responses to climate change of cultivated species (e.g., Chmielewski et al., 2004; Darbyshire et al., 2014); future research that draws on this body of literature could inform our understanding of wild plants in natural systems. Additionally, studies that correlate climatic cues with gene expression in genes associated with fruiting in wild plants (e.g., see Kudoh, 2016; Yeoh et al., 2017 for this type of research with genes associated with flowering) would increase our ability to tease apart the pathways and extent to which different climatic variables impact the genetic component of fruiting. Lastly, in animal-dispersed species, disperser activity could directionally select for fruiting phenology, and further studies are needed to explore to what extent and in which species dispersers drive phenotypic selection, particularly with reference to the FTFI and population-level traits like crop size and fruiting duration (Palacio et al., 2021).
We have emphasized that different types of environmental controls can interact with each other. Further studies are needed to understand and differentiate among these effects. For example, in an analysis of the midpoint of listed fruiting dates of 11,605 Chinese species within a compiled flora, Du et al. (2020) found that mean annual precipitation, precipitation seasonality, temperature seasonality, and temperature of the coldest quarter were included in the best supported models of the variation in fruiting times across species. Reduced soil moisture was a climate-related variable that was correlated with shortened FTFI in some alpine plant species, as was increased temperature in most species, although the interaction between them was not specifically tested (Sethi et al., 2020). Further, interactions between temperature and precipitation (e.g., Misson et al., 2010; Butt et al., 2015; Mazer et al., 2015) with climate change could be additive and cause fruiting times to advance more than changes to temperature or precipitation alone. Further research is also needed on how precipitation affects fruiting phenology, including the effect of higher or lower precipitation on the FTFI and how timing of precipitation affects fruiting (e.g., Molau, 1997; Fitter and Fitter, 2002; Parmesan and Yohe, 2003; Du et al., 2020). Additionally, seasonality of precipitation and how it relates to fruiting phenology in tropical wet forests needs to be further investigated, as current studies show contradictory patterns (Mendoza et al., 2017). Finally, environmental controls could interact with other factors influencing a species’ phenology and our other proposed mechanisms. For example, higher temperatures due to climate change could interact with biogeography (e.g., Loarie et al., 2009; Butt et al., 2015; Ge et al., 2015, reviewed in Du et al., 2020), phylogeny (Davis et al., 2010), and life form (Du et al., 2020; Ganjurjav et al., 2021) to produce patterns in the FTFI across many species.
Lastly, we recommend that future research precisely define the beginning and end of the FTFI and that these definitions are incorporated into study design. Because FTFI is defined at the level of a single reproductive structure on an individual plant, the start and end of the FTFI can be defined in terms of flower opening, closing, or pollen deposition, and the end of the FTFI can be defined as when the individual fruit is ripe, with the recognition that “ripeness” will need to be precisely delineated for each species. While our case study was limited by available phenological data, future studies can tease apart flower longevity by defining the beginning of the FTFI at the time of pollen deposition or flower closing and can investigate intraspecific, and even intraindividual, variation in FTFI. Long-term studies using these definitions on the boundaries of the FTFI, which are currently lacking, can then be used to determine interannual variation.
The mechanisms we propose that could drive the FTFI in the context of a changing climate are relevant to all plants with biotic and abiotic seed dispersal. However, FTFI and fruiting phenology changes in animal-dispersed plants are particularly important to study since they have larger repercussions for the communities of which these plants are a part. For example, changes in fruiting phenology and animal abundance or activity could result in a temporal mismatch between partners, especially if plants and animals are responding to different environmental cues or responding in different ways (Forrest, 2014; Rafferty et al., 2015; Palacio et al., 2021). If the timing of peak disperser activity is shifting in response to environmental changes in a similar direction to fruiting times, with fruiting times responding via either genotypic variation or environmental controls in plants, no phenological mismatch will result. However, if the FTFI is internally driven, fruiting times will shift earlier in the season in conjunction with earlier flower times. If the FTFI is externally driven, changing environmental conditions could result in fruiting times that are out of step with peak disperser activity, resulting in a similar phenological mismatch outcome as if FTFI were internally driven. The same is true if the FTFI is a result of stabilizing selection, unless other selective pressures act to re-align fruiting times with peak disperser activity. Phenological mismatch could result in dispersal failure via fruiting occurring before dispersers are active (Warren and Bradford, 2013), lower plant fitness via reduced disperser activity (McConkey and Drake, 2006; Traveset et al., 2012; Rogers et al., 2017), animal population declines via reduced fecundity or increased mortality (van Schaik et al., 1993; Wright et al., 1999; Saino et al., 2011), or changes in community composition (Moegenburg and Levey, 2003; Peralta et al., 2020).
Phenological mismatch is not the only way in which plant and animal populations, communities, and ecosystems could be affected by climate change impacts on the FTFI. Plant and seed-disperser populations could be affected by reduced fruit production resulting from higher than average temperatures, increases or decreases in precipitation, or a combination (Young et al., 2004; Augspurger, 2009; CaraDonna and Bain, 2016; Babweteera et al., 2018; Benlloch-González et al., 2018; Chapman et al., 2018; Lee et al., 2018; Mendoza et al., 2018; Pardee et al., 2018; Nussbaumer et al., 2020; Rogers et al., 2021) if internal or external drivers of the FTFI pull fruiting times into less than ideal climatic conditions. Additionally, fruiting phenology could shift earlier at different magnitudes across elevation or space (e.g., Rafferty et al., 2020), leading to shifting spatial activity of seed dispersers in response to changed resource levels across the landscape (Curran and Leighton, 2000). Lastly, major shifts in frugivorous animal activity could lead to a cascade of effects that impact ecosystem function (Rogers et al., 2021), even if seed dispersal is not directly affected. For example, red elderberry fruiting early in Alaska caused bears to leave salmon runs to gorge on fruits, disrupting a strong predator-prey interaction (Deacy et al., 2017) and likely causing a reduction in nitrogen influx to the forest (Helfield and Naiman, 2006).
We have leveraged here the extensive data on flowering phenology to explore how flowering and fruiting phenologies are linked. We found strong evidence in some species but not in others for a link between flowering and fruiting times. These results suggest that we should rapidly expand our understanding of the FTFI and shifts in fruiting phenology to enable better predictions for future climate change-influenced conditions. For those plant species demonstrating climate-driven phenological shifts in either direction, we need to explore when and to what extent those shifts will affect the ecological functioning and conservation concerns of plant and/or animal populations and/or mutualisms (e.g., Saino et al., 2011; Rafferty et al., 2015; Renner and Zohner, 2018). A better understanding of the magnitude of the effects on plant and animal populations and communities, as well as the factors that produce those effects, could enable parameterization of fitness models, and increase our ability to predict population trajectories and community composition in a changing climate.
Publicly available datasets were analyzed in this study. Data were provided by the members of the PEP725 project: http://www.pep725.eu (PEP725).
MES performed case study analyses. MES, CEA, LP, and JLB jointly wrote the first draft of the manuscript. All authors contributed substantially to revisions.
The ideas for this paper emerged from an NSF-funded workshop (Grant DEB-1548194) at the National Socio-Environmental Synthesis Center. This work was supported by the NSF (Grant DEB-1701858).
The authors declare that the research was conducted in the absence of any commercial or financial relationships that could be construed as a potential conflict of interest.
All claims expressed in this article are solely those of the authors and do not necessarily represent those of their affiliated organizations, or those of the publisher, the editors and the reviewers. Any product that may be evaluated in this article, or claim that may be made by its manufacturer, is not guaranteed or endorsed by the publisher.
We would like to extend our gratitude to all participants of the NSF-funded workshop at the National Socio-Environmental Synthesis Center, and especially Noelle Beckman, Haldre Rogers, Janneke Hille Ris Lambers, Florian Hartig, Sebastian Schreiber, and Rebecca S. Snell. We are grateful to two reviewers for helpful comments on an earlier version of the manuscript.
The Supplementary Material for this article can be found online at: https://www.frontiersin.org/articles/10.3389/fevo.2021.752110/full#supplementary-material
Anderson, J. T., Inouye, D. W., McKinney, A. M., Colautti, R. I., and Mitchell-Olds, T. (2012). Phenotypic plasticity and adaptive evolution contribute to advancing flowering phenology in response to climate change. Proc. R. Soc. Lond. B Biol. Sci. 279, 3843–3852. doi: 10.1098/rspb.2012.1051
Arroyo, M. T. K., Armesto, J. J., and Villagran, C. (1981). Plant phenological patterns in the high Andean Cordillera of central Chile. J. Ecol. 69, 205–223. doi: 10.2307/2259826
Augspurger, C. K. (2009). Spring 2007 warmth and frost: phenology, damage and refoliation in a temperate deciduous forest. Funct. Ecol. 23, 1031–1039. doi: 10.1111/j.1365-2435.2009.01587.x
Augspurger, C. K., and Zaya, D. N. (2020). Concordance of long-term shifts with climate warming varies among phenological events and herbaceous species. Ecol. Monogr. 90:e01421. doi: 10.1002/ecm.1421
Babweteera, F., Plumptre, A. J., Adamescu, G. S., Shoo, L. P., Beale, C. M., Reynolds, V., et al. (2018). The ecology of tree reproduction in an African medium altitude rain forest. Biotropica 50, 405–417. doi: 10.1111/btp.12563
Bartomeus, I., Ascher, J. S., Wagner, D., Danforth, B. N., Colla, S., Kornbluth, S., et al. (2011). Climate-associated phenological advances in bee pollinators and bee-pollinated plants. Proc. Natl. Acad. Sci. U.S.A. 201115559. doi: 10.1073/pnas.1115559108
Benlloch-González, M., Sánchez-Lucas, R., Benlloch, M., and Ricardo, F.-E. (2018). An approach to global warming effects on flowering and fruit set of olive trees growing under field conditions. Sci. Hortic. 240, 405–410. doi: 10.1016/j.scienta.2018.06.054
Bernard, A., Marrano, A., Donkpegan, A., Brown, P. J., Leslie, C. A., Neale, D. B., et al. (2020). Association and linkage mapping to unravel genetic architecture of phenological traits and lateral bearing in Persian walnut (Juglans regia L.). BMC Genomics 21:203. doi: 10.1186/s12864-020-6616-y
Buonaiuto, D. M., Morales-Castilla, I., and Wolkovich, E. M. (2021). Reconciling competing hypotheses regarding flower–leaf sequences in temperate forests for fundamental and global change biology. New Phytol. 229, 1206–1214. doi: 10.1111/nph.16848
Burger, C., Belskii, E., Eeva, T., Laaksonen, T., Mägi, M., Mänd, R., et al. (2012). Climate change, breeding date and nestling diet: how temperature differentially affects seasonal changes in pied flycatcher diet depending on habitat variation. J. Anim. Ecol. 81, 926–936. doi: 10.1111/j.1365-2656.2012.01968.x
Butt, N., Seabrook, L., Maron, M., Law, B. S., Dawson, T. P., Syktus, J., et al. (2015). Cascading effects of climate extremes on vertebrate fauna through changes to low-latitude tree flowering and fruiting phenology. Glob. Change Biol. 21, 3267–3277. doi: 10.1111/gcb.12869
Calinger, K. M., Queenborough, S., and Curtis, P. S. (2013). Herbarium specimens reveal the footprint of climate change on flowering trends across north-central North America. Ecol. Lett. 16, 1037–1044. doi: 10.1111/ele.12135
CaraDonna, P. J., and Bain, J. A. (2016). Frost sensitivity of leaves and flowers of subalpine plants is related to tissue type and phenology. J. Ecol. 104, 55–64. doi: 10.1111/1365-2745.12482
CaraDonna, P. J., Iler, A. M., and Inouye, D. W. (2014). Shifts in flowering phenology reshape a subalpine plant community. Proc. Natl. Acad. Sci. U.S.A. 111, 4916–4921. doi: 10.1073/pnas.1323073111
Carbognani, M., Tomaselli, M., and Petraglia, A. (2018). Different temperature perception in high-elevation plants: new insight into phenological development and implications for climate change in the alpine tundra. Oikos 127, 1014–1023. doi: 10.1111/oik.04908
Chapman, C. A., Chapman, L. J., Struhsaker, T. T., Zanne, A. E., Clark, C. J., and Poulsen, J. R. (2005). A long-term evaluation of fruiting phenology: importance of climate change. J. Trop. Ecol. 21, 31–45. doi: 10.1017/S0266467404001993
Chapman, C. A., Valenta, K., Bonnell, T. R., Brown, K. A., and Chapman, L. J. (2018). Solar radiation and ENSO predict fruiting phenology patterns in a 15-year record from Kibale National Park, Uganda. Biotropica 50, 384–395. doi: 10.1111/btp.12559
Chen, T.-S., Aoike, T., Yamasaki, M., Kajiya-Kanegae, H., and Iwata, H. (2020). Predicting rice heading date using an integrated approach combining a machine learning method and a crop growth model. Front. Genet. 11:599510. doi: 10.3389/fgene.2020.599510
Chmielewski, F.-M., Müller, A., and Bruns, E. (2004). Climate changes and trends in phenology of fruit trees and field crops in Germany, 1961–2000. Agric. For. Meteorol. 121, 69–78. doi: 10.1016/S0168-1923(03)00161-8
Chuine, I., and Beaubien, E. G. (2001). Phenology is a major determinant of tree species range. Ecol. Lett. 4, 500–510. doi: 10.1046/j.1461-0248.2001.00261.x
Chuine, I., Cour, P., and Rousseau, D. D. (1999). Selecting models to predict the timing of flowering of temperate trees: implications for tree phenology modelling. Plant Cell Environ. 22, 1–13. doi: 10.1046/j.1365-3040.1999.00395.x
Chuine, I., and Régnière, J. (2017). Process-based models of phenology for plants and animals. Annu. Rev. Ecol. Evol. Syst. 48, 159–182. doi: 10.1146/annurev-ecolsys-110316-022706
Cotton, P. A. (2003). Avian migration phenology and global climate change. Proc. Natl. Acad. Sci. U.S.A. 100, 12219–12222. doi: 10.1073/pnas.1930548100
Crozier, L. G., Hendry, A. P., Lawson, P. W., Quinn, T. P., Mantua, N. J., Battin, J., et al. (2008). Potential responses to climate change in organisms with complex life histories: evolution and plasticity in Pacific salmon. Evol. Appl. 1, 252–270. doi: 10.1111/j.1752-4571.2008.00033.x
Curran, L. M., and Leighton, M. (2000). Vertebrate responses to spatiotemporal variation in seed production of mast-fruiting Dipterocarpaceae. Ecol. Monogr. 70, 101–128.
Darbyshire, R., Webb, L., Goodwin, I., and Barlow, E. W. R. (2014). Challenges in predicting climate change impacts on pome fruit phenology. Int. J. Biometeorol. 58, 1119–1133. doi: 10.1007/s00484-013-0705-4
Davis, C. C., Willis, C. G., Primack, R. B., and Miller-Rushing, A. J. (2010). The importance of phylogeny to the study of phenological response to global climate change. Philos. Trans. R. Soc. Lond. B Biol. Sci. 365, 3201–3213. doi: 10.1098/rstb.2010.0130
Deacy, W. W., Armstrong, J. B., Leacock, W. B., Robbins, C. T., Gustine, D. D., Ward, E. J., et al. (2017). Phenological synchronization disrupts trophic interactions between Kodiak brown bears and salmon. Proc. Natl. Acad. Sci. U.S.A. 114, 10432–10437. doi: 10.1073/pnas.1705248114
Dose, V., and Menzel, A. (2004). Bayesian analysis of climate change impacts in phenology. Glob. Change Biol. 10, 259–272. doi: 10.1111/j.1529-8817.2003.00731.x
Du, Y., Mao, L., Queenborough, S. A., Primack, R., Comita, L. S., Hampe, A., et al. (2020). Macro-scale variation and environmental predictors of flowering and fruiting phenology in the Chinese angiosperm flora. J. Biogeogr. 47, 2303–2314. doi: 10.1111/jbi.13938
Dunham, A. E., Razafindratsima, O. H., Rakotonirina, P., and Wright, P. C. (2018). Fruiting phenology is linked to rainfall variability in a tropical rain forest. Biotropica 50, 396–404. doi: 10.1111/btp.12564
Eriksson, O., and Ehrlén, J. (1991). Phenological variation in fruit characteristics in vertebrate-dispersed plants. Oecologia 86, 463–470. doi: 10.1007/BF00318311
Ettinger, A. K., Buonaiuto, D. M., Chamberlain, C. J., Morales-Castilla, I., and Wolkovich, E. M. (2021). Spatial and temporal shifts in photoperiod with climate change. New Phytol. 230, 462–474. doi: 10.1111/nph.17172
Ettinger, A. K., Gee, S., and Wolkovich, E. M. (2018). Phenological sequences: how early-season events define those that follow. Am. J. Bot. 105, 1771–1780. doi: 10.1002/ajb2.1174
Faticov, M., Ekholm, A., Roslin, T., and Tack, A. J. M. (2020). Climate and host genotype jointly shape tree phenology, disease levels and insect attacks. Oikos 129, 391–401. doi: 10.1111/oik.06707
Fitter, A. H., and Fitter, R. S. R. (2002). Rapid changes in flowering time in British plants. Science 296, 1689–1691. doi: 10.1126/science.1071617
Forrest, J., and Miller-Rushing, A. J. (2010). Toward a synthetic understanding of the role of phenology in ecology and evolution. Philos. Trans. R. Soc. Lond. B Biol. Sci. 365, 3101–3112. doi: 10.1098/rstb.2010.0145
Forrest, J. R. K. (2014). Plant–pollinator interactions and phenological change: What can we learn about climate impacts from experiments and observations? Oikos 124, 4–13. doi: 10.1111/oik.01386
Gallinat, A. S., Primack, R. B., and Wagner, D. L. (2015). Autumn, the neglected season in climate change research. Trends Ecol. Evol. 30, 169–176. doi: 10.1016/j.tree.2015.01.004
Ganjurjav, H., Gornish, E., Hu, G., Wu, J., Wan, Y., Li, Y., et al. (2021). Phenological changes offset the warming effects on biomass production in an alpine meadow on the Qinghai–Tibetan Plateau. J. Ecol. 109, 1014–1025. doi: 10.1111/1365-2745.13531
Ge, Q., Wang, H., Rutishauser, T., and Dai, J. (2015). Phenological response to climate change in China: a meta-analysis. Glob. Change Biol. 21, 265–274. doi: 10.1111/gcb.12648
Giménez-Benavides, L., García-Camacho, R., Iriondo, J. M., and Escudero, A. (2011). Selection on flowering time in Mediterranean high-mountain plants under global warming. Evol. Ecol. 25, 777–794. doi: 10.1007/s10682-010-9440-z
Gordo, O., and Sanz, J. J. (2009). Long-term temporal changes of plant phenology in the Western Mediterranean. Glob. Change Biol. 15, 1930–1948. doi: 10.1111/j.1365-2486.2009.01851.x
Gordo, O., and Sanz, J. J. (2010). Impact of climate change on plant phenology in Mediterranean ecosystems. Glob. Change Biol. 16, 1082–1106. doi: 10.1111/j.1365-2486.2009.02084.x
Gougherty, A. V., and Gougherty, S. W. (2018). Sequence of flower and leaf emergence in deciduous trees is linked to ecological traits, phylogenetics, and climate. New Phytol. 220, 121–131. doi: 10.1111/nph.15270
Guillaume, C., Isabelle, C., Marc, B., and Thierry, A. (2018). Assessing frost damages using dynamic models in walnut trees: exposure rather than vulnerability controls frost risks: frost risks in walnut trees. Plant Cell Environ. 41, 1008–1021. doi: 10.1111/pce.12935
Haggerty, B. P., and Galloway, L. F. (2011). Response of individual components of reproductive phenology to growing season length in a monocarpic herb. J. Ecol. 99, 242–253. doi: 10.1111/j.1365-2745.2010.01744.x
Hamann, A. (2004). Flowering and fruiting phenology of a Philippine submontane rain forest: climatic factors as proximate and ultimate causes. J. Ecol. 92, 24–31. doi: 10.1111/j.1365-2745.2004.00845.x
Helfield, J. M., and Naiman, R. J. (2006). Keystone interactions: Salmon and bear in riparian forests of Alaska. Ecosystems 9, 167–180. doi: 10.1007/s10021-004-0063-5
Heydel, F., and Tackenberg, O. (2016). How are the phenologies of ripening and seed release affected by species’ ecology and evolution? Oikos 126, 738–747. doi: 10.1111/oik.03442
Inouye, B. D., Ehrlén, J., and Underwood, N. (2019). Phenology as a process rather than an event: from individual reaction norms to community metrics. Ecol. Monogr. 89:e01352. doi: 10.1002/ecm.1352
Jiang, L. L., Wang, S. P., Meng, F. D., Duan, J. C., Niu, H. S., Xu, G. P., et al. (2016). Relatively stable response of fruiting stage to warming and cooling relative to other phenological events. Ecology 97, 1961–1969. doi: 10.1002/ecy.1450
Kingsolver, J. G., Arthur Woods, H., Buckley, L. B., Potter, K. A., MacLean, H. J., and Higgins, J. K. (2011). Complex life cycles and the responses of insects to climate change. Integr. Comp. Biol. 51, 719–732. doi: 10.1093/icb/icr015
Kingsolver, J. G., Hoekstra, H. E., Hoekstra, J. M., Berrigan, D., Vignieri, S. N., Hill, C. E., et al. (2001). The strength of phenotypic selection in natural populations. Am. Nat. 157, 245–261.
Klapwijk, M. J., Csóka, G., Hirka, A., and Björkman, C. (2013). Forest insects and climate change: long-term trends in herbivore damage. Ecol. Evol. 3, 4183–4196. doi: 10.1002/ece3.717
Kudoh, H. (2016). Molecular phenology in plants: in natura systems biology for the comprehensive understanding of seasonal responses under natural environments. New Phytol. 210, 399–412. doi: 10.1111/nph.13733
Lany, N. K., Ayres, M. P., Stange, E. E., Sillett, T. S., Rodenhouse, N. L., and Holmes, R. T. (2016). Breeding timed to maximize reproductive success for a migratory songbird: the importance of phenological asynchrony. Oikos 125, 656–666. doi: 10.1111/oik.02412
Lechowicz, M. J. (1995). Seasonality of flowering and fruiting in temperate forest trees. Can. J. Bot. 73, 175–182.
Lee, S. G., Kim, S. K., Lee, H. J., Lee, H. S., and Lee, J. H. (2018). Impact of moderate and extreme climate change scenarios on growth, morphological features, photosynthesis, and fruit production of hot pepper. Ecol. Evol. 8, 197–206. doi: 10.1002/ece3.3647
Loarie, S. R., Duffy, P. B., Hamilton, H., Asner, G. P., Field, C. B., and Ackerly, D. D. (2009). The velocity of climate change. Nature 462, 1052–1055. doi: 10.1038/nature08649
Marco, D., and Páez, S. A. (2002). Phenology and phylogeny of animal-dispersed plants in a Dry Chaco forest (Argentina). J. Arid Environ. 52, 1–16. doi: 10.1016/S0140-1963(02)90976-6
Marra, P. P., Francis, C. M., Mulvihill, R. S., and Moore, F. R. (2005). The influence of climate on the timing and rate of spring bird migration. Oecologia 142, 307–315. doi: 10.1007/s00442-004-1725-x
Marrano, A., Sideli, G. M., Leslie, C. A., Cheng, H., and Neale, D. B. (2019). Deciphering of the genetic control of phenology, yield, and pellicle color in Persian Walnut (Juglans regia L.). Front. Plant Sci. 10:1140. doi: 10.3389/fpls.2019.01140
Mayor, S. J., Guralnick, R. P., Tingley, M. W., Otegui, J., Withey, J. C., Elmendorf, S. C., et al. (2017). Increasing phenological asynchrony between spring green-up and arrival of migratory birds. Sci. Rep. 7:1902. doi: 10.1038/s41598-017-02045-z
Mazer, S. J., Gerst, K. L., Matthews, E. R., and Evenden, A. (2015). Species-specific phenological responses to winter temperature and precipitation in a water-limited ecosystem. Ecosphere 6, 1–27. doi: 10.1890/ES14-00433.1
McConkey, K. R., and Drake, D. R. (2006). Flying foxes cease to function as seed dispersers long before they become rare. Ecology 87, 271–276.
McDermott, M. E., and DeGroote, L. W. (2017). Linking phenological events in migratory passerines with a changing climate: 50 years in the Laurel Highlands of Pennsylvania. PLoS One 12:e0174247. doi: 10.1371/journal.pone.0174247
Mendoza, I., Condit, R. S., Wright, S. J., Caubère, A., Châtelet, P., Hardy, I., et al. (2018). Inter-annual variability of fruit timing and quantity at Nouragues (French Guiana): insights from hierarchical Bayesian analyses. Biotropica 50, 431–441. doi: 10.1111/btp.12560
Mendoza, I., Peres, C. A., and Morellato, L. P. C. (2017). Continental-scale patterns and climatic drivers of fruiting phenology: a quantitative Neotropical review. Glob. Planet. Change 148, 227–241. doi: 10.1016/j.gloplacha.2016.12.001
Menzel, A., Estrella, N., and Fabian, P. (2001). Spatial and temporal variability of the phenological seasons in Germany from 1951 to 1996. Glob. Change Biol. 7, 657–666. doi: 10.1111/j.1365-2486.2001.00430.x
Menzel, A., Sparks, T. H., Estrella, N., Koch, E., Aasa, A., Ahas, R., et al. (2006). European phenological response to climate change matches the warming pattern. Glob. Change Biol. 12, 1969–1976. doi: 10.1111/j.1365-2486.2006.01193.x
Menzel, A., Yuan, Y., Matiu, M., Sparks, T., Scheifinger, H., Gehrig, R., et al. (2020). Climate change fingerprints in recent European plant phenology. Glob. Change Biol. 26, 2599–2612. doi: 10.1111/gcb.15000
Miller-Rushing, A. J., and Primack, R. B. (2008). Global warming and flowering times in Thoreau’s Concord: a community perspective. Ecology 89, 332–341. doi: 10.1890/07-0068.1
Misson, L., Degueldre, D., Collin, C., Rodriguez, R., Rocheteau, A., Ourcival, J.-M., et al. (2010). Phenological responses to extreme droughts in a Mediterranean forest. Glob. Change Biol. 17, 1036–1048. doi: 10.1111/j.1365-2486.2010.02348.x
Moegenburg, S. M., and Levey, D. J. (2003). Do frugivores respond to fruit harvest? An experimental study of short-term responses. Ecology 84, 2600–2612.
Molau, U. (1997). Responses to natural climatic variation and experimental warming in two tundra plant species with contrasting life forms: Cassiope tetragona and Ranunculus nivalis. Glob. Change Biol. 3, 97–107. doi: 10.1111/j.1365-2486.1997.gcb138.x
Moore, L. M., and Lauenroth, W. K. (2017). Differential effects of temperature and precipitation on early- vs. late-flowering species. Ecosphere 8:e01819. doi: 10.1002/ecs2.1819
Morales, M. A., Dodge, G. J., and Inouye, D. W. (2005). A phenological mid-domain effect in flowering diversity. Oecologia 142, 83–89. doi: 10.1007/s00442-004-1694-0
Munguía-Rosas, M. A., Ollerton, J., Parra-Tabla, V., and De-Nova, J. A. (2011). Meta-analysis of phenotypic selection on flowering phenology suggests that early flowering plants are favoured. Ecol. Lett. 14, 511–521. doi: 10.1111/j.1461-0248.2011.01601.x
Nagahama, A., Kubota, Y., and Satake, A. (2018). Climate warming shortens flowering duration: a comprehensive assessment of plant phenological responses based on gene expression analyses and mathematical modeling. Ecol. Res. 33, 1059–1068. doi: 10.1007/s11284-018-1625-x
Niu, Y., Yang, Y., Zhang, Z.-Q., Li, Z.-M., and Sun, H. (2011). Floral closure induced by pollination in gynodioecious Cyananthus delavayi (Campanulaceae): effects of pollen load and type, floral morph and fitness consequences. Ann. Bot. 108, 1257–1268. doi: 10.1093/aob/mcr224
Noma, N., and Yumoto, T. (1997). Fruiting phenology of animal-dispersed plants in response to winter migration of frugivores in a warm temperate forest on Yakushima Island, Japan. Ecol. Res. 12, 119–129. doi: 10.1007/BF02523777
Nussbaumer, A., Meusburger, K., Schmitt, M., Waldner, P., Gehrig, R., Haeni, M., et al. (2020). Extreme summer heat and drought lead to early fruit abortion in European beech. Sci. Rep. 10:5334.
Ovaskainen, O., Skorokhodova, S., Yakovleva, M., Sukhov, A., Kutenkov, A., Kutenkova, N., et al. (2013). Community-level phenological response to climate change. Proc. Natl. Acad. Sci. U.S.A. 110, 13434–13439. doi: 10.1073/pnas.1305533110
Palacio, F. X., Cataudela, J. F., Montalti, D., and Ordano, M. (2021). Do frugivores exert selection on fruiting phenology? Potential scenarios across three plant populations of a Neotropical vine, Passiflora caerulea. Evol. Ecol. 35, 555–574.
Pardee, G. L., Inouye, D. W., and Irwin, R. E. (2018). Direct and indirect effects of episodic frost on plant growth and reproduction in subalpine wildflowers. Glob. Change Biol. 24, 848–857. doi: 10.1111/gcb.13865
Parmesan, C., and Yohe, G. (2003). A globally coherent fingerprint of climate change impacts across natural systems. Nature 421, 37–42. doi: 10.1038/nature01286
Peñuelas, J., Filella, I., Zhang, X., Llorens, L., Ogaya, R., Lloret, F., et al. (2004). Complex spatiotemporal phenological shifts as a response to rainfall changes. New Phytol. 161, 837–846. doi: 10.1111/j.1469-8137.2004.01003.x
Peralta, G., Vázquez, D. P., Chacoff, N. P., Lomáscolo, S. B., Perry, G. L. W., and Tylianakis, J. M. (2020). Trait matching and phenological overlap increase the spatio-temporal stability and functionality of plant–pollinator interactions. Ecol. Lett. 23, 1107–1116. doi: 10.1111/ele.13510
Post, E., Pedersen, C., Wilmers, C. C., and Forchhammer, M. C. (2008a). Warming, plant phenology and the spatial dimension of trophic mismatch for large herbivores. Proc. R. Soc. Lond. B Biol. Sci. 275, 2005–2013. doi: 10.1098/rspb.2008.0463
Post, E. S., Pedersen, C., Wilmers, C. C., and Forchhammer, M. C. (2008b). Phenological sequences reveal aggregate life history response to climatic warming. Ecology 89, 363–370. doi: 10.1890/06-2138.1
Primack, R. B. (1987). Relationships among flowers, fruits, and seeds. Annu. Rev. Ecol. Syst. 18, 409–430. doi: 10.1146/annurev.es.18.110187.002205
Proctor, H. C., and Harder, L. D. (1995). Effect of pollination success on floral longevity in the orchid Calypso bulbosa (Orchidaceae). Am. J. Bot. 82, 1131–1136. doi: 10.2307/2446066
Rafferty, N. E., CaraDonna, P. J., and Bronstein, J. L. (2015). Phenological shifts and the fate of mutualisms. Oikos 124, 14–21. doi: 10.1111/oik.01523
Rafferty, N. E., Diez, J. M., and Bertelsen, C. D. (2020). Changing climate drives divergent and nonlinear shifts in flowering phenology across elevations. Curr. Biol. 30, 432–441. doi: 10.1016/j.cub.2019.11.071
Rafferty, N. E., and Nabity, P. D. (2017). A global test for phylogenetic signal in shifts in flowering time under climate change. J. Ecol. 105, 627–633. doi: 10.1111/1365-2745.12701
Renner, S. S., and Zohner, C. M. (2018). Climate change and phenological mismatch in trophic interactions among plants, insects, and vertebrates. Annu. Rev. Ecol. Evol. Syst. 49, 165–182.
Rogers, H. S., Buhle, E. R., HilleRisLambers, J., Fricke, E. C., Miller, R. H., and Tewksbury, J. J. (2017). Effects of an invasive predator cascade to plants via mutualism disruption. Nat. Commun. 8:14557. doi: 10.1038/ncomms14557
Rogers, H. S., Donoso, I., Traveset, A., and Fricke, E. C. (2021). Cascading impacts of seed disperser loss on plant communities and ecosystems. Annu. Rev. Ecol. Evol. Syst. 52, 641–666.
Root, T. L., Price, J. T., Hall, K. R., Schneider, S. H., Rosenzweig, C., and Pounds, J. A. (2003). Fingerprints of global warming on wild animals and plants. Nature 421, 57–60. doi: 10.1038/nature01333
Saino, N., Ambrosini, R., Rubolini, D., von Hardenberg, J., Provenzale, A., Hüppop, K., et al. (2011). Climate warming, ecological mismatch at arrival and population decline in migratory birds. Proc. R. Soc. Lond. B Biol. Sci. 278, 835–842. doi: 10.1098/rspb.2010.1778
Satake, A., Kawagoe, T., Saburi, Y., Chiba, Y., Sakurai, G., and Kudoh, H. (2013). Forecasting flowering phenology under climate warming by modelling the regulatory dynamics of flowering-time genes. Nat. Commun. 4:2303. doi: 10.1038/ncomms3303
Schluter, D. (1988). Estimating the form of natural selection on a quantitative trait. Evolution 42, 849–861. doi: 10.1111/j.1558-5646.1988.tb02507.x
Segrestin, J., Bernard-Verdier, M., Violle, C., Richarte, J., Navas, M.-L., and Garnier, E. (2018). When is the best time to flower and disperse? A comparative analysis of plant reproductive phenology in the Mediterranean. Funct. Ecol. 32, 1770–1783. doi: 10.1111/1365-2435.13098
Sethi, M. L., Theobald, E. J., Breckheimer, I., and Lambers, J. H. R. (2020). Early snowmelt and warmer, drier summers shrink postflowering transition times in subalpine wildflowers. Ecology 101:e03171. doi: 10.1002/ecy.3171
Sheriff, M. J., Kenagy, G. J., Richter, M., Lee, T., Tøien, Ø., Kohl, F., et al. (2011). Phenological variation in annual timing of hibernation and breeding in nearby populations of Arctic ground squirrels. Proc. R. Soc. Lond. B Biol. Sci. 278, 2369–2375. doi: 10.1098/rspb.2010.2482
Sherry, R. A., Zhou, X., Gu, S., Arnone, J. A., Schimel, D. S., Verburg, P. S., et al. (2007). Divergence of reproductive phenology under climate warming. Proc. Natl. Acad. Sci. U.S.A. 104, 198–202. doi: 10.1073/pnas.0605642104
Singh, K. P., and Kushwaha, C. P. (2006). Diversity of flowering and fruiting phenology of trees in a tropical deciduous Forest in India. Ann. Bot. 97, 265–276. doi: 10.1093/aob/mcj028
Staggemeier, V. G., Diniz-Filho, J. A. F., Zipparro, V. B., Gressler, E., de Castro, E. R., Mazine, F., et al. (2015). Clade-specific responses regulate phenological patterns in Neotropical Myrtaceae. Perspect. Plant Ecol. Evol. Syst. 17, 476–490. doi: 10.1016/j.ppees.2015.07.004
Steinacher, G., and Wagner, J. (2010). Flower longevity and duration of pistil receptivity in high mountain plants. Flora Morphol. Distrib. Funct. Ecol. Plants 205, 376–387. doi: 10.1016/j.flora.2009.12.012
Templ, B., Koch, E., Bolmgren, K., Ungersböck, M., Paul, A., Scheifinger, H., et al. (2018). Pan European Phenological database (PEP725): a single point of access for European data. Int. J. Biometeorol. 62, 1109–1113.
Thomas, C. D., and Lennon, J. J. (1999). Birds extend their ranges northwards. Nature 399:213. doi: 10.1038/20335
Tingley, M. W., Monahan, W. B., Beissinger, S. R., and Moritz, C. (2009). Birds track their Grinnellian niche through a century of climate change. Proc. Natl. Acad. Sci. U.S.A. 106, 19637–19643. doi: 10.1073/pnas.0901562106
Traveset, A., González-Varo, J. P., and Valido, A. (2012). Long-term demographic consequences of a seed dispersal disruption. Proc. R. Soc. Lond. B Biol. Sci. 279, 3298–3303. doi: 10.1098/rspb.2012.0535
Tripathi, A., Tripathi, D. K., Chauhan, D. K., Kumar, N., and Singh, G. S. (2016). Paradigms of climate change impacts on some major food sources of the world: a review on current knowledge and future prospects. Agric. Ecosyst. Environ. 216, 356–373. doi: 10.1016/j.agee.2015.09.034
Trunschke, J., and Stöcklin, J. (2017). Plasticity of flower longevity in alpine plants is increased in populations from high elevation compared to low elevation populations. Alp Bot. 127, 41–51.
Usenik, V., and Štampar, F. (2011). The effect of environmental temperature on sweet cherry phenology. Eur. J. Hortic. Sci. 76, 1–5.
van Doorn, W. G. (1997). Effects of pollination on floral attraction and longevity. J. Exp. Bot. 48, 1615–1622.
van Schaik, C. P., Terborgh, J. W., and Wright, S. J. (1993). The phenology of tropical forests: adaptive significance and consequences for primary consumers. Annu. Rev. Ecol. Syst. 24, 353–377. doi: 10.1146/annurev.es.24.110193.002033
Warren, R. J., and Bradford, M. A. (2013). Mutualism fails when climate response differs between interacting species. Glob. Change Biol. 20, 466–474. doi: 10.1111/gcb.12407
Wilczek, A. M., Burghardt, L. T., Cobb, A. R., Cooper, M. D., Welch, S. M., and Schmitt, J. (2010). Genetic and physiological bases for phenological responses to current and predicted climates. Philos. Trans. R. Soc. Lond. B Biol. Sci. 365, 3129–3147. doi: 10.1098/rstb.2010.0128
Wolkovich, E. M., and Cleland, E. E. (2014). Phenological niches and the future of invaded ecosystems with climate change. AoB Plants 6:plu013. doi: 10.1093/aobpla/plu013
Wolkovich, E. M., and Ettinger, A. K. (2014). Back to the future for plant phenology research. New Phytol. 203, 1021–1024. doi: 10.1111/nph.12957
Wright, S. J., Carrasco, C., Calderón, O., and Paton, S. (1999). The El Niño Southern Oscillation, Variable Fruit Production, and Famine in a Tropical Forest. Ecology 80, 1632–1647.
Yeoh, S. H., Satake, A., Numata, S., Ichie, T., Lee, S. L., Basherudin, N., et al. (2017). Unravelling proximate cues of mass flowering in the tropical forests of South-East Asia from gene expression analyses. Mol. Ecol. 26, 5074–5085. doi: 10.1111/mec.14257
Young, L. W., Wilen, R. W., and Bonham-Smith, P. C. (2004). High temperature stress of Brassica napus during flowering reduces micro- and megagametophyte fertility, induces fruit abortion, and disrupts seed production. J. Exp. Bot. 55, 485–495. doi: 10.1093/jxb/erh038
Keywords: global change, flowering, fruiting, life history stages, phenological shifts, seed dispersal
Citation: Sandor ME, Aslan CE, Pejchar L and Bronstein JL (2021) A Mechanistic Framework for Understanding the Effects of Climate Change on the Link Between Flowering and Fruiting Phenology. Front. Ecol. Evol. 9:752110. doi: 10.3389/fevo.2021.752110
Received: 02 August 2021; Accepted: 08 November 2021;
Published: 06 December 2021.
Edited by:
Isabel Donoso, Consejo Superior de Investigaciones Científicas (CSIC), SpainReviewed by:
Irene Mendoza, Estación Biológica de Doñana (EBD), SpainCopyright © 2021 Sandor, Aslan, Pejchar and Bronstein. This is an open-access article distributed under the terms of the Creative Commons Attribution License (CC BY). The use, distribution or reproduction in other forums is permitted, provided the original author(s) and the copyright owner(s) are credited and that the original publication in this journal is cited, in accordance with accepted academic practice. No use, distribution or reproduction is permitted which does not comply with these terms.
*Correspondence: Manette E. Sandor, bWFuZXR0ZS5zYW5kb3JAY29sdW1iaWEuZWR1
Disclaimer: All claims expressed in this article are solely those of the authors and do not necessarily represent those of their affiliated organizations, or those of the publisher, the editors and the reviewers. Any product that may be evaluated in this article or claim that may be made by its manufacturer is not guaranteed or endorsed by the publisher.
Research integrity at Frontiers
Learn more about the work of our research integrity team to safeguard the quality of each article we publish.