Erratum: Building on 150 years of knowledge: The freshwater isopod Asellus aquaticus as an integrative eco-evolutionary model system
- 1Department of Aquatic Ecology, Eawag: Swiss Federal Institute of Aquatic Science and Technology, Dübendorf, Switzerland
- 2Department of Biology, Lund University, Lund, Sweden
- 3Department of Fish Ecology and Evolution, Center of Ecology, Evolution and Biochemistry, Eawag: Swiss Federal Institute of Aquatic Science and Technology, Kastanienbaum, Switzerland
- 4Institute of Integrative Biology, ETH Zürich, Zurich, Switzerland
- 5Department of Biological and Environmental Science, University of Jyväskylä, Jyväskylä, Finland
Interactions between organisms and their environments are central to how biological diversity arises and how natural populations and ecosystems respond to environmental change. These interactions involve processes by which phenotypes are affected by or respond to external conditions (e.g., via phenotypic plasticity or natural selection) as well as processes by which organisms reciprocally interact with the environment (e.g., via eco-evolutionary feedbacks). Organism-environment interactions can be highly dynamic and operate on different hierarchical levels, from genes and phenotypes to populations, communities, and ecosystems. Therefore, the study of organism-environment interactions requires integrative approaches and model systems that are suitable for studies across different hierarchical levels. Here, we introduce the freshwater isopod Asellus aquaticus, a keystone species and an emerging invertebrate model system, as a prime candidate to address fundamental questions in ecology and evolution, and the interfaces therein. We review relevant fields of research that have used A. aquaticus and draft a set of specific scientific questions that can be answered using this species. Specifically, we propose that studies on A. aquaticus can help understanding (i) the influence of host-microbiome interactions on organismal and ecosystem function, (ii) the relevance of biotic interactions in ecosystem processes, and (iii) how ecological conditions and evolutionary forces facilitate phenotypic diversification.
Introduction
Interactions between organisms and their biotic and abiotic environment are central to our understanding of how biological diversity originates and how it is maintained (Pimentel, 1961; Kokko and López-Sepulcre, 2007; Hendry, 2017; Lion, 2018; Schwab et al., 2018; Govaert et al., 2019; Skúlason et al., 2019). Such interactions can be unidirectional or reciprocal, and involve dynamic processes operating across different levels of biological organization, from individuals and populations to communities and ecosystems. Examples of unidirectional processes include phenotypic responses to environmental conditions within an organism’s lifetime (e.g., phenotypic plasticity; Via and Lande, 1985; West-Eberhard, 2003; Sultan, 2021) and changes in population fitness across generations (e.g., adaptive evolution; Charlesworth et al., 2017). Examples of reciprocal interactions include coevolutionary dynamics between species (e.g., Anderson and May, 1982; Thompson, 1989), associations between hosts and microbial communities (e.g., microbiomes; Foster et al., 2017; Koskella et al., 2017), and evolution via organism-mediated modification of abiotic conditions (e.g., niche construction; Odling-Smee et al., 1996; Laland et al., 2016). Organism-environment interactions are inextricably linked with evolutionary processes, but their complexity and contingency represent a significant challenge to understanding biodiversity dynamics in natural ecosystems (Levins and Lewontin, 1985; Sultan, 2015; Hendry, 2017; Lion, 2018; Svensson, 2018; De Meester et al., 2019). Therefore, understanding how organisms and ecosystems respond to environmental heterogeneity in space and time remains an important challengue in evolutionary ecology, and necessitates studies that investigate organism-environment interactions across different hierarchical levels.
Much of our current mechanistic understanding of the emergence and evolution of phenotypic variation comes from studies using traditional microbe, plant, and animal model systems, which have provided great insight into how genetic, developmental, and environmental factors shape phenotypes (Lenski et al., 1991; Kohler, 1994; Silver, 1995; Dooley and Zon, 2000; Duvick, 2001; Barnett, 2007; Cresko et al., 2007; Fielenbach and Antebi, 2008; Bedford and Hoekstra, 2015; Hake and Ross-Ibarra, 2015). A mounting body of work has provided vast amounts of genomic resources and phenotypic data, as well as molecular toolkits and analytical methods (e.g., Ramos et al., 2014; O’Leary et al., 2016; Hunt et al., 2018; Thurmond et al., 2019). While some of the existing models have considerable ecological breadth and functional tractability both in laboratory and field settings (e.g., Skúlason et al., 2019; Duffy et al., 2021), many of these systems have only limited potential for integration across multiple scales of inquiry (i.e., from individual variation to dynamics within populations, communities, and ecosystems). Such integration is however critical in ecology and evolution, not only because natural ecosystems consist of a myriad of species interactions (De Meester et al., 2019; Travis, 2020) which are subject to adaptive change (Thompson, 1998; Hairston et al., 2005), but also because the hierarchical structure of natural ecosystems, ranging from the activities of individuals to ecosystem-level processes, is the relevant object of study (Wimsatt and Wimsatt, 2007). Thus, the introduction of model systems that are suitable for studies across hierarchical levels can enrich our understanding of the contextual nature of organism-environment interactions (Levins and Lewontin, 1985). Moreover, a wider set of empirical model systems is needed in order to make headway in integrative studies of microevolutionary processes in ecologically relevant settings, and to improve our ability to generalize the underlying processes (Bolker, 2014; Alfred and Baldwin, 2015).
In line with this, we introduce a model system to address fundamental questions in ecology and evolution from multiple perspectives and across scales: the freshwater isopod Asellus aquaticus, commonly known as aquatic sowbug, water slate, or waterlouse. This species is widely distributed, phenotypically variable, and has a very long history in research, ranging from environmental and ecotoxicological to developmental and evolutionary studies (Figure 1; e.g., Unwin and Stebbing, 1920; Kosswig and Kosswig, 1940; Konec et al., 2015; Fuller et al., 2018; O’Callaghan et al., 2019; Verovnik and Konec, 2019). This sets a solid, but to this day underexploited, foundation for integrative studies on organismal-environment interactions within an eco-evolutionary framework.
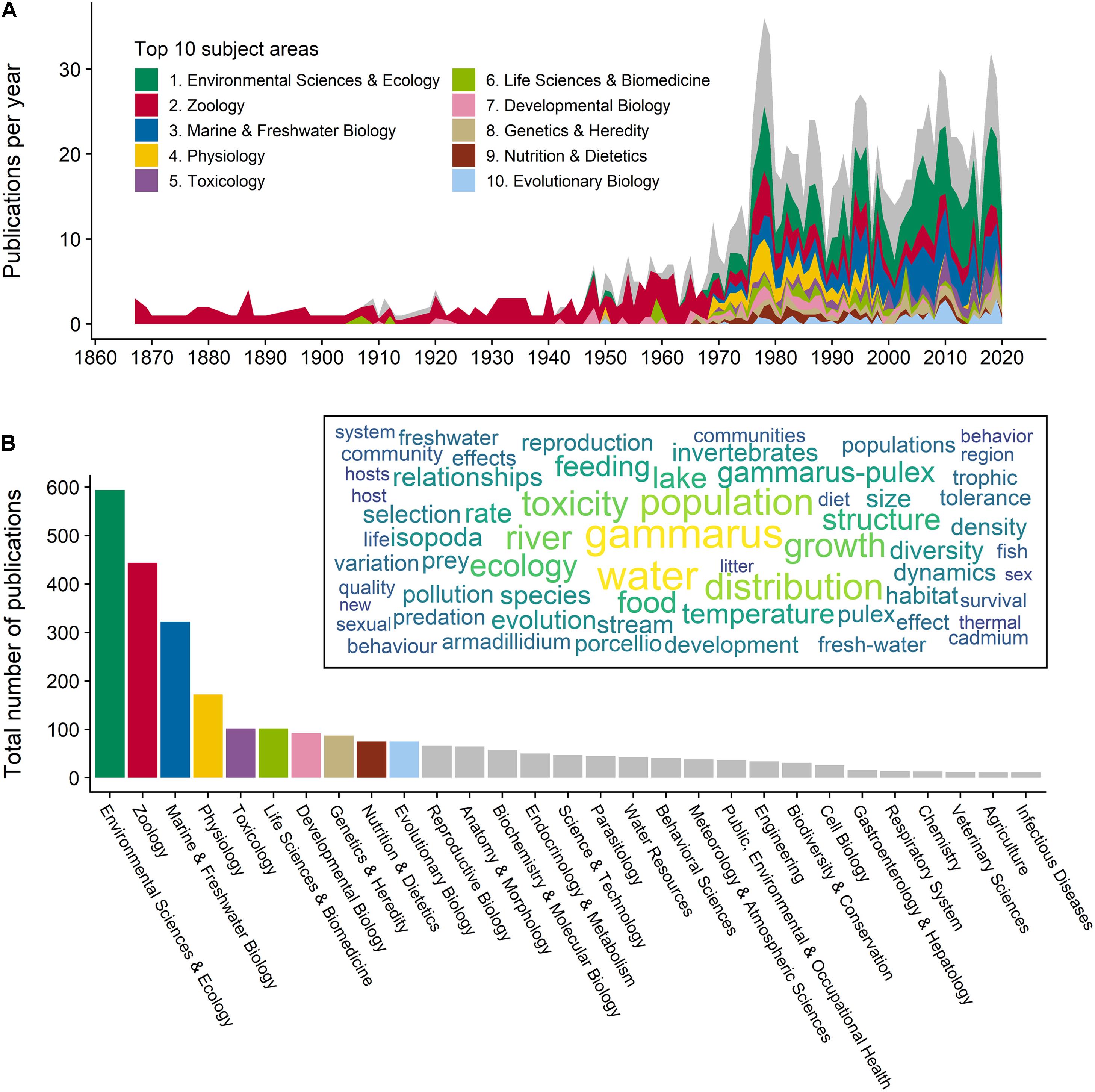
Figure 1. Over 150 years of research on and with Asellus aquaticus. The figure summarizes published scientific literature on A. aquaticus. We conducted a quantitative literature survey with the search tools of Web of Science (WOS; Clarivate analytics) by searching for the term “asellus aquaticus” in six databases (i.e., BIOSIS, CABI, FSTA, Medline, WOS Core Collection, and Zoological Records). We found 1235 records, published between 1867 and 2020. (A) The graph shows the number of publications per year within a given subject area, as designated by WOS. (B) The graph shows the total number of publications assigned to a specific subject area. The top 10 fields account for 72.58% of all publications, and are indicated by color coding in (A,B) (multiple assignments are possible, summing up to 2845 assignments). The inset in (B) shows a wordcloud with the 100 most used keywords from all A. aquaticus publications. Furthermore, we compiled all records with relevant information (e.g., title, keywords, research areas, and abstract) to a single file which is available online (Zenodo doi: https://zenodo.org/record/5070310#.YV1LQx1S_zU). More details can be found in the Supplementary Material.
We begin our review with an overview of key aspects of A. aquaticus life history, ecology, and evolution that facilitate such integrative studies (see section “Asellus aquaticus as a Model System for Integrative Studies”). Next, we highlight three major areas of integrative research in ecology and evolution for which A. aquaticus is particularly well-suited to address questions across different hierarchical scales (see section “Areas of Integrative Research Using Asellus aquaticus”). Starting at the organismal scale, we show how diverse host-microbiome interactions in A. aquaticus can help us understand host-microbiome evolution (see Koskella et al., 2017). Specifically, how host microbiomes, including endosymbionts and epibionts, can influence organismal performance and fitness, which in turn may affect ecosystem function. Broadening the scale to then include how individuals interact with their external biotic and abiotic environments, we propose that studies on A. aquaticus can aid in understanding species interactions and ecosystem processes. Finally, given substantial phenotypic variation and repeated cases of morphological diversification of this species (e.g., Verovnik and Konec, 2019), we suggest that integrative studies on A. aquaticus can shed light on how ecological conditions and evolutionary forces jointly shape phenotypes. Throughout the review, we emphasize how studies using this species can aid in bridging different disciplines (e.g., ecology, developmental, and evolutionary biology) and levels of biological organization (e.g., from individuals to ecosystems). We note that the species has been extensively used in the field of ecotoxicology (e.g., Bloor, 2011; O’Callaghan et al., 2019), but our goal here is to highlight, in particular, its use as an eco-evolutionary model.
Asellus Aquaticus as a Model System for Integrative Studies
Asellus aquaticus (Crustacea, Isopoda, Asellidae; Linnaeus, 1758) is a sexually reproducing arthropod, with several key features of ecological relevance and methodological benefits that facilitate establishing it as a model system for integrative research. Below, we provide a short historical overview and summarize the key characteristics that make A. aquaticus an excellent model system.
Asellus aquaticus has a long history in biological research (Figure 1). The earliest available scientific publications on A. aquaticus were written in the late 1800s and focused on anatomical and physiological features of organismal development (Dohrn, 1867; Zuelzer, 1907; Wege, 1909). These early studies included detailed descriptions of its life cycle and its ability to regenerate appendages (Unwin and Stebbing, 1920; Needham, 1950; Fano et al., 1976; Murphy and Learner, 1982; Marchetti and Montalenti, 1990). In Box 1, we summarize those and some other characteristics of A. aquaticus, including core aspects of its body plan, reproduction, development, and growth. Research on A. aquaticus shifted, in more recent years, from individual-level variation to population and ecosystem processes, prompting a wider use of this species in ecological and evolutionary studies (Graça et al., 1994; Hargeby et al., 2004; Eroukhmanoff et al., 2009a; Lürig et al., 2019; Lürig and Matthews, 2021).
BOX 1. Life cycle and reproduction in Asellus aquaticus.
Body plan: Adults are characterized anatomically by the presence of the derived traits distinctive of isopods’ body plan (Wilson, 1991; Balian et al., 2008; Verovnik et al., 2009; Vick and Blum, 2010). This body plan consists of a dorsoventrally flattened body divided in three tagma. First, the cephalon (ce), which results from the fusion of the mandibulate head and the first thoracic segment with maxillipeds. Second, the pereon (pe) with seven thoracic segments and walking legs (called pareopods), which increase in length posteriorly. Third, the pleon (or abdomen), with abdominal limbs (or pleopods) used for respiration, the uropods, and the gonads. Two of the pleonic segments are free while the others are fused to the telson, forming the pleotelson (pl) (Balian et al., 2008; Verovnik et al., 2009; Vick and Blum, 2010).
Reproduction: Asellus aquaticus is a gonochoric species (Bertin et al., 2002), with a karyotype consisting of eight homomorphic chromosome pairs (2n = 16) in both sexes (Montalenti and Rocchi, 1964; Salemaa, 1979; Valentino et al., 1983). It is presumed to have exclusively sexual reproduction (Unwin and Stebbing, 1920; Ridley and Thompson, 1979). Fertilization is only possible during a short time window (during approximately 24 hours), while the female oviducal openings are free (i.e., right after she molts the exoskeleton posterior to the fifth thoracic segment). Because of this time-limitation in fertility, copulation is preceded by mate guarding; a behavioral strategy common in isopods, during which the male guards the female by carrying her until insemination becomes possible (Jormalainen et al., 1994; Jormalainen, 1998). The duration of this pre-copula (or amplexus) phase is variable (Jormalainen and Merilaita, 1995). After copulation, insemination happens internally with the eggs in the mother’s brood pouch (bp) (within a marsupium) being fertilized during their transit in the oviduct (Wilson, 1991). Females of A. aquaticus are females are assumed not to have a true spermatheca, rather a temporary pouch in the oviduct (i.e., seminal receptacle) that can collect sperm and that regresses after egg-laying without sperm storage (Wilson, 1986). Females can produce a variable number of eggs (between a few dozen to over a hundred; e.g., Lürig and Matthews, 2021) and large females tend to produce more eggs (Arakelova, 2001).
Development and growth: Embryonic development happens within the mother’s brood pouch and different stages of embryogenesis can be easily detected in the brood pouch. Initially, eggs are round and surrounded by the chorion (ch) and the vitelline membrane (vi) (Brusca and Iverson, 1985; Wilson, 1991; Martínez and Defeo, 2006; Wolff, 2009; Vick and Blum, 2010). Early embryogenesis is characterized by the appearance of a dorsal curvature and start of the incorporation of the yolk (y) into the digestive glands (Vick and Blum, 2010). By late embryogenesis, the yolk is fully incorporated, the appendages are well developed, the embryo (e) has lengthened along the ventral curvature, and the thoracic segments are evident (Brusca and Iverson, 1985; Wilson, 1991; Martínez and Defeo, 2006; Wolff, 2009; Vick and Blum, 2010).
In most aspects, A. aquaticus follows the standard development described in isopods (Brusca and Iverson, 1985; Martínez and Defeo, 2006; Wolff, 2009; Vick and Blum, 2010), though analyses of expression patterns and regulation of several Hox genes (i.e., homeobox genes that specify regions of the animal body plan along the head-tail axis) have revealed novel patterns of gene expression (e.g., hindgut expression of Abdominal-B gene; Vick and Blum, 2010). This highlights the potential of this system to study evolutionary changes of segment identity and patterning (Vick and Blum, 2010; Mojaddidi et al., 2018). Embryos develop into small juveniles inside the marsupium and are released from the brood pouch at approximately 1 mm length. Individuals undergo indeterminate growth with biphasic molting; a type of ecdysis, characteristic of isopods (George, 1972; Steel, 1982), whereby the posterior and anterior body halves molt sequentially. First the hind-half (from fifth thoracic segment to posterior-most part of the body) molts, which is followed by hardening of the exoskeleton and subsequent molting of the fore-half (from fourth thoracic segment to anterior-most part of the body), with a time interval of about 24 hours (Unwin and Stebbing, 1920; George, 1972; Marcus, 1990). Sexual maturity, is reached at 1.5 to 3 months (depending on environmental conditions) (e.g., Lürig and Matthews, 2021), and at approximately 3–4 mm length. Growth is continuous after sexual maturation (Steel, 2009). As in other crustaceans, growth is dependent on ecdysis, which involves tissue growth and the synthesis of a new exoskeleton that eventually replaces the old one during the molt (Carlisle, 1956; Chang and Mykles, 2011).
Sexual dimorphism: In males, genitals consist of pairs of testes that are located in the region that binds together the pereon (pe) and the pleon (pl). In females, the ovaries are paired and lie parallel to the hindgut with oviducal openings on the fifth thoracic segment. When a female is about to produce eggs, the ovaries enlarge and extend along the length of the thorax (Unwin and Stebbing, 1920). Besides the gonads, sexual dimorphism in A. aquaticus is apparent in males being larger than females (e.g., Adams et al., 1985) (see section “Sexual Selection and Assortative Mating”) and a few morphological modifications, mostly involved in pre-copula formation (Bertin et al., 2002). Such morphological modifications involve the pleotelson (pl) and several paraeopods, which differ in shape between males and females: in males the fourth pair of paraeopods is reduced and curved, which allows them to position and carry the female during mate guarding, and the first pair of paraeopods bears apophyses that are absent in females (Unwin and Stebbing, 1920; Needham, 1942; Chang, 1986; Brusca and Wilson, 1991; Bertin et al., 2002). The oostegites of females, which are specialized limbs, enlarge to form the brood pouch in the anterior ventral side of the body, covering the oviducal openings.
Isopoda are known for showing extensive intraspecific morphological variation and for being successful colonizers of a wide range of environments (Wetzer, 2002; Wilson, 2008, 2009; Joca et al., 2015; Lins et al., 2017; Shen et al., 2017; Rudy et al., 2018; Alves et al., 2019). Though empirical tests are scarce thus far, some characteristic traits of A. aquaticus, such as biphasic molting (Unwin and Stebbing, 1920; George, 1972; Marcus, 1990; Balian et al., 2008), pre-copula formation (Unwin and Stebbing, 1920; Montalentl, 1990), and the development of brood pouches (Ridley and Thompson, 1979), have been associated with the evolutionary success of A. aquaticus, and of Isopoda in general (Hornung, 2011; Horváthová et al., 2017). In general, A. aquaticus inhabits a variety of natural (and man-made) aquatic habitats, and is common in lakes, ponds, rivers, and creeks across Europe and parts of Asia. In Box 2, we summarize what is known about A. aquaticus phylogeography, including its phylogenetic relationships, as well as patterns of global distribution and ecogeographic variation in life histories. Numerous subspecies of A. aquaticus have been described along the species’ total geographic range (Sket, 1994; Prevorcnik et al., 2004; Verovnik et al., 2009). Its broad distribution and diversity of colonized habitats exposes the species to ecological variation on local and continental scale, and provides opportunities for eco-evolutionary and phylogenetic studies across broad geographic scales.
BOX 2. Phylogeny and ecogeographical patterns.
Map showing the occurrence of A. aquaticus based on publicly available biodiversity databases (gray dots) and previously published studies (yellow dots and polygons). The distribution range of the species estimated by Birštejn (1951); Sket (1994), and Verovnik and Konec (2019), is shown with the orange, the green, and the pink polygons, respectively. This indicates a wide distribution of A. aquaticus throughout Europe and a poorly defined eastern edge of the geographical range (see also Sket, 1994). Note that the latter is likely due to lack of rigorous surveys rather than true differences in abundance (see Supplementray Table 1). Very few publications report explicitly the absence of A. aquaticus from specific locations (Williams, 1962b), most occurrence data comes from contributors from western European countries, and there are discrepancies between studies. It is therefore possible that the species is present in more locations but not yet explored or reported. More details can be found in the Supplementary Material.
Phylogeny: Asellus aquaticus belongs to the Isopoda (von Reumont et al., 2012; Rota-Stabelli et al., 2013; Misof et al., 2014; Thomas et al., 2020), which contains more than 10,000 species with extensive intraspecific morphological variation, sexual dimorphism, sequential hermaphroditism, and with a global distribution (Wetzer, 2002; Wilson, 2008, 2009; Joca et al., 2015; Lins et al., 2017; Shen et al., 2017; Rudy et al., 2018; Alves et al., 2019). Isopoda have successfully colonized a variety of environments, including deep sea, land, and freshwater (Hessler et al., 1979; Poulin, 1995; Wilson, 2008). The colonization of terrestrial and freshwater environments is thought to have occurred independently from marine environments (Carefoot and Taylor, 1995; Broly et al., 2013; Lins et al., 2017), but the timing of this transition to land is uncertain (Lins et al., 2017), due to the scarcity of fossil records for this group (Schmidt, 2008; Broly et al., 2013; Lins et al., 2017). Reconstructing the phylogenetic relationships of Isopoda has been a major challenge (Wetzer, 2002; Wilson, 2008, 2009; Joca et al., 2015; Lins et al., 2017; Shen et al., 2017; Rudy et al., 2018; Alves et al., 2019), and vastly different conclusions have been reached depending on whether phylogenetic reconstructions were based on morphological (e.g., biphasic molting, heart musculature) or molecular data (Zhang et al., 2019).
The combination of different types of data (e.g., mitochondrial and nuclear DNA) and methodological approaches (e.g., maximum likelihood or Bayesian inference; Lartillot et al., 2007; Crotty et al., 2019) has revealed the Asellota, the clade containing A. aquaticus, as the oldest branch of the Isopoda (Watling, 1981; Wilson, 2009; Yu et al., 2018; Zhang et al., 2019). Interestingly, two inversions of the origin of replication in the mitochondrial genome took place within the Isopoda; one occurred after the Asellota branched off (see Figure 5 in Zhang et al., 2019) and the other one in the highly derived taxon group composed of Cymothoidae and Corallanidae. Alternatively, and less parsimoniously, the basal isopod taxon could also be the Phreatoicidea (Wetzer, 2002; Kilpert et al., 2012; Zhang et al., 2019). Given the recency in the progress of elucidating the phylogenetic isopod relationships, the growing insights from fossil records (see e.g., Selden et al., 2016; Schädel et al., 2020), and the very speciose nature and extreme diversity of this clade, many interesting new insights might still await us, with genomic data increasing rapidly and becoming available for analyses.
Geographic distribution: Asellus aquaticus occurs throughout large parts of Europe (Sket, 1994; Verovnik et al., 2005; Sworobowicz et al., 2015), although its local distribution can be distinctively patchy (Williams, 1962b; Hynes and Williams, 1965; Wolff, 1973). It is hypothesized that A. aquaticus arrived in Europe around 8 to 12 million years ago through the brackish Paratethys basin (Sket, 1994; Verovnik et al., 2005). The species’ current distribution is assumed to reflect the last ice age, where the Appennine and the Balkan Peninsula served as major glacial refugia for many species in Europe (Hewitt, 1999, 2000). Asellus aquaticus probably diversified in such southern refugia before subsequently recolonizing the northern parts of Europe when the ice retreated (Verovnik et al., 2005; Sworobowicz et al., 2015). Recently, it was shown that A. aquaticus might have also survived in the extensive lacustrine systems present along the glacial margins in a vast network of glacial microrefugia across Europe (Sworobowicz et al., 2020). It has also been suggested that dispersal of A. aquaticus may have been influenced by human activity (Williams, 1962b; Verovnik et al., 2005; Sworobowicz et al., 2020), though empirical data supporting this view is still scarce. Genetic diversity is maintained by secondary contact of different phylogroups or lineages (Verovnik et al., 2005), and is highest around the Adriatic Sea, the Alps, and the south-eastern Balkan Peninsula, whereas central and north-eastern Europe seem to be dominated by populations with low genetic diversity (Sworobowicz et al., 2015). Further studies exploring the distribution patterns of this species in more detail could shed light onto its current geographical range and dispersal patterns. This may be particularly relevant given that the wide geographic distribution of A. aquaticus likely reflects a capacity to reach (via dispersal) and to tolerate (or adapt to) a broad range of environments (e.g., high resistance to desiccation, pollution, and changing temperatures; e.g., Aston and Milner, 1980; Weltje, 2006; Konec et al., 2016; O’Callaghan et al., 2019).
Ecogeographic variation in life histories: Studies in natural populations from different geographical locations show that A. aquaticus commonly has two complete generations per year (e.g., in England; Unwin and Stebbing, 1920; Adcock, 1979; Ridley and Thompson, 1979; in Norway; Økland, 1978). These give rise to spring and autumn cohorts (Unwin and Stebbing, 1920; Adcock, 1979; Ridley and Thompson, 1979), which may encounter different selective environments, as shown in other species (e.g., Leys et al., 2017). The first cohort develops from embryo to maturity entirely during the favorable growing season (i.e., summer months), whereas second cohort (overwintering generation) develop rapidly during the first weeks of life, reach sexual maturity, and then enter a state of reproductive stasis (or reproductive diapause) (Brasiello and Tadini, 1969; Adcock, 1979; Steel, 2009). Despite the aforementioned commonalities across geographically distinct locations, A. aquaticus also shows substantial geographic and seasonal variation in breeding patterns and life history characteristics, potentially reflecting adaptation (or plasticity) to differing environmental factors (Anderson, 1969; Økland, 1978; Murphy and Learner, 1982). The length of the breeding season can be highly variable (e.g., 5–11 months; Aston and Milner, 1980), with northern populations having shorter breeding seasons (Anderson, 1969) and periods of sexual inactivity, or reproductive diapause (Fano et al., 1976; Migliore et al., 1982). Such geographic variation in life cycles, including the reproductive diapause, has been proposed to be adaptive: in several northern locations faster development (than in southern locations) allows individuals to exploit resources following reproductive stasis (in spring and summer) (Fano et al., 1976; Vitagliano et al., 1994).
Along with its wide environmental distribution, several studies have documented phenotypic variation across different environments and habitats in A. aquaticus (e.g., Williams, 1962a; Aston and Milner, 1980; Hargeby et al., 2004; Sworobowicz et al., 2015; Lürig et al., 2019), indicating its high capacity for phenotypic change via both phenotypic plasticity and genetic adaptation (see section “Integration and Future Perspectives”). Of particular relevance from an eco-evolutionary perspective, are the repeated cases of ecotype differentiation within Swedish lakes (Eroukhmanoff et al., 2009a,b) and the evolution of cave-adapted populations in karstic areas (Kosswig and Kosswig, 1940; Konec et al., 2015; Mojaddidi et al., 2018). These examples of phenotypic divergence underscore the potential of this species to contribute to our understanding of adaptive diversification across different temporal and spatial scales.
Moreover, A. aquaticus is a key mediator of ecosystem level processes, being involved in a range of important trophic and non-trophic interactions in freshwater ecosystems. On the one hand, A. aquaticus is an efficient detritivore with a broad spectrum of diets from microbes to leaf litter, and thus, a major contributor to the recycling of nutrients and biomass (Carpenter and Lodge, 1986; Graça et al., 1994; Bjelke and Herrmann, 2005). On the other hand, A. aquaticus serves as a prey for mesopredators (e.g., fish, insects, and waterfowl; Hart and Gill, 1992; Hargeby et al., 2004), and acts as a host to parasites, endosymbionts, and epibionts (Cook et al., 1998; Dezfuli, 2000; Zimmer and Bartholmé, 2003).
The broad environmental distribution of A. aquaticus is considered to stem from its ability to cope with stressful environmental conditions (e.g., Aston and Milner, 1980; Maltby, 1995). For instance, the species is resilient to high levels of both organic and chemical pollution (Aston and Milner, 1980; Van Ginneken et al., 2017, 2019), and is able to bioaccumulate metals (Elangovan et al., 1999; Rauch and Morrison, 1999) – making it a well-suited study system also in ecotoxicology. There is a vast number of insightful studies exemplifying the relevance of this species for water quality assessment and for ecotoxicology (e.g., Rauch and Morrison, 1999; MacNeil et al., 2002; Christensen et al., 2013; Van Ginneken et al., 2017), as well as a recent review highlighting A. aquaticus as a model for biomonitoring (O’Callaghan et al., 2019; Figure 1), but we do not cover these aspects further here.
Finally, from a methodological point of view, individuals of A. aquaticus can be collected readily from the wild, and populations easily established and maintained over multiple generations in the laboratory at low-cost (e.g., Bloor, 2010, 2011). Asellus aquaticus is also well-suited for large scale phenotypic and genetic studies, with several phenotyping and genotyping tools already available, including a high throughput phenotyping pipeline (Lürig, 2021), a genome-wide linkage map and hundreds of genetic markers (Protas et al., 2011; Bakovic et al., 2021). This combination makes A. aquaticus highly amenable to controlled laboratory and mesocosm experiments (Rossi and Fano, 1979; Maltby, 1995; Lürig et al., 2019), as well as to in-depth genetic and phenotypic studies (e.g., Protas et al., 2011;, Lürig et al. 2019, Bakovic et al., 2021).
Areas of Integrative Research Using Asellus Aquaticus
Microbial Associations
The interactions between a host and its microbiome can have a substantial impact on organismal function and fitness and can mediate ecosystem processes (e.g., Douglas, 2014; Fisher et al., 2017; Hurst, 2017; Koskella et al., 2017; López-García et al., 2017). Isopods, such as A. aquaticus, are well-suited to studies on host-microbiome interactions as they bear a diverse community of bacteria and eukaryotic microorganisms inside and outside their bodies (Douglas, 1998; Wang et al., 2007; Fraune and Bosch, 2010; Gilbert et al., 2012; Dhanasekaran et al., 2021). A multitude of complex associations with microorganisms, ranging from intracellular associations to epibiotic symbiosis, have already been found or hypothesized to exist in A. aquaticus (Zimmer and Bartholmé, 2003; Wang et al., 2007; Bredon et al., 2020).
One of the most important functions provided by the microbiota is the ability to digest nutritionally challenging compounds, such as lignocellulose, that A. aquaticus and many terrestrial isopods, use as a major food source (Zimmer et al., 2001; Bredon et al., 2019, 2020). Such dietary endosymbiosis is thought to have been a key element during the radiation of isopods in terrestrial and freshwater habitats from a common marine ancestor (Zimmer and Bartholmé, 2003; Wang et al., 2007; Bouchon et al., 2016; Bredon et al., 2019, 2020).
Besides dietary endosymbiosis, the prevalence of the reproductive manipulator Wolbachia (Cordaux et al., 2012), and a diverse community of epibionts with understudied functions (Fontaneto and Ambrosini, 2010), make isopods promising study systems for host-microbiome interactions. In general, microbes may broaden host environmental tolerance and thereby allow hosts to have wide dietary niches, inhabit diverse abiotic environments, and cope with a variety of environmental stressors (Henry et al., 2021). Although little studied thus far in A. aquaticus, it seems possible that its broad environmental tolerance is, in part, achieved via host-microbiome interactions. Furthermore, dietary symbionts in A. aquaticus have the potential to directly mediate the role of their detritivore hosts in the ecosystems, which makes A. aquaticus, together with terrestrial isopods (Bouchon et al., 2016) also well-suited to explore the effects of symbiotic associations on ecosystem functioning (see section “Integration and Future Perspectives”).
Microbial Communities and Diet
The ability of isopods, including A. aquaticus, to break down cellulose and lignins (i.e., lignocellulose) from leaf litter is considered a crucial adaptation during its past colonization of freshwater habitats from marine ancestors (Zimmer et al., 2001, 2002a; Zimmer and Bartholmé, 2003). While marine isopods often feed on nutritious and easily digestible food sources, such as algae (Kennish and Williams, 1997; Wahlström et al., 2020), detritivores in freshwater ecosystems are often confronted with leaf litter and detritus of allochthonous origin (Grieve and Lau, 2018). This detritus typically has low nutrient levels and high concentrations of lignocellulose, phenolics, and other recalcitrant components of terrestrial plants (Webster and Benfield, 1986; Kritzberg et al., 2004; Cross et al., 2005; Solomon et al., 2008). Since most marine ancestors do not have the ability to degrade such compounds (Ray and Julian, 1952; Zimmer et al., 2001; Wang et al., 2007), it is hypothesized that the successful colonization of freshwater environments was facilitated by the adoption of gut microbial communities that produce enzymes for the digestion of recalcitrant plant detritus (Zimmer et al., 2001, 2002a; Bredon et al., 2019, 2020).
Asellus aquaticus is known to harbor bacterial endosymbionts in two digestive structures: the hepatopancreas (also called midgut gland; Wang et al., 2007; Bouchon et al., 2016; Bredon et al., 2019), a multilobed gland that is functionally analogous to the liver and pancreas of higher organisms (Brunet et al., 1994), and the hindgut (Zimmer and Bartholmé, 2003). The community of bacterial endosymbionts in the midgut gland produces digestive enzymes such as phenoloxidase and cellulase, allowing A. aquaticus to feed directly on recalcitrant allochthonous substrates (Robson, 1979; Zimmer and Bartholmé, 2003; Hasu et al., 2008; Bredon et al., 2020). Other crustacean detritivores (e.g., the amphipod Gammarus pulex; Zimmer and Bartholmé, 2003), and various terrestrial isopods (Wang et al., 2007; Kostanjšek et al., 2010; Bredon et al., 2020) also resort to enzymatic breakdown of leaf litter, but enzymes in these other species are produced by both host (Kostanjšek et al., 2010) and gut microbiota (Bredon et al., 2020). In contrast, A. aquaticus seems to mostly rely on its gut microbiome when digesting plant material of terrestrial origin (Bredon et al., 2020), providing evidence for a strong symbiotic relationship.
Similarly to other detritivores, A. aquaticus also relies on environmental bacteria for nutritional purposes via microbial establishment, i.e., microbial colonization of the substrate, which is thought to provide several benefits (Gessner et al., 1999). On the one hand, this microbial colonization results in a change of the physical and chemical status of terrestrial substrates (Gessner et al., 1999), preparing them for consumption by detritivores through enzymatic catalysis and leaf fragmentation (Bärlocher and Porter, 1986; Bärlocher, 1992). On the other hand, the algae, bacteria, and fungi colonizing the substrate and forming an extracellular polymeric substance matrix (e.g., biofilm; Flemming et al., 2007), acts directly as an important food source. Although A. aquaticus can feed on fresh plant material (e.g., macrophytes or filamentous algae; Marcus et al., 1978), it seems to prefer (and also grow faster on) substrates colonized by microbiota (Marcus et al., 1978; Rossi, 1985; Graça et al., 1993a; Bohmann, 2005). The optimal diet of A. aquaticus is likely to be a complex mix of components. For instance, algal compounds seem to be needed for optimal development, likely due to a specific fatty acid composition (Grieve and Lau, 2018). In addition, there is compelling evidence that A. aquaticus prefers fungal food sources, and even specific species of fungi, over other parts of the biofilm (Rossi and Fano, 1979; Graça et al., 1993a), possibly because higher growth rates can be sustained when feeding on phosphorus and nitrogen-rich fungi (Rossi and Fano, 1979; Graça et al., 1993b; Lürig and Matthews, 2021).
Microbial Reproductive Manipulators
A variety of microorganisms are known to affect reproduction and sex determination in arthropods (Stouthamer et al., 1990; Breeuwer and Jacobs, 1996; Groenenboom and Hogeweg, 2002), including isopods (e.g., Chebbi et al., 2019). Of these, the best studied is the intracellular bacterium Wolbachia, which may occur in as many as half of all arthropod species (Cowdry, 1923; Hilgenboecker et al., 2008; Weinert et al., 2015). Wolbachia can have a major impact on host fitness (Werren, 1997; Stouthamer et al., 1999; Werren et al., 2008; Becking et al., 2019) by for instance, protecting against viruses (Teixeira et al., 2008), providing nutrients (Brownlie et al., 2009) and, notably, by influencing reproduction and life-histories (Dobson et al., 2002; Cao et al., 2019). Being a maternally transmitted symbiont, Wolbachia has evolved mechanisms to promote its spread, either by inducing cytoplasmic incompatibility or by skewing offspring sex ratios toward females via feminization, male killing, or the induction of parthenogenesis (O’Neill et al., 1997). In the terrestrial isopods Armadillidium nasatum (Becking et al., 2019) and A. vulgare (Chebbi et al., 2019), Wolbachia is thought to have influenced sex chromosome evolution and cytoplasmic sex determination.
Asellus aquaticus is known to carry Wolbachia (Bouchon et al., 1998), and different lines of evidence suggest that sex determination in this species may be influenced by both sex determining genes and maternally transmitted factors (Vitagliano et al., 1994), such as Wolbachia. On the one hand, the karyotype of A. aquaticus consists of eight homomorphic chromosome pairs (2n = 16) in both sexes (Montalenti and Rocchi, 1964; Salemaa, 1979; Valentino et al., 1983). However, the presence of a difference in heterochromatin areas for one chromosome pair in males from an Italian population has been taken as an indication for an incipient sex chromosome differentiation in A. aquaticus (Rocchi et al., 1984; Volpi et al., 1992). On the other hand, analyses of hybrid offspring between populations differing in male to female ratios indicate that sex ratio in A. aquaticus can be highly dependant on the origin of the mother (Vitagliano et al., 1994). This potentially indicates a role for a maternally transmitted factor, such as Wolbachia, in sex determination and population sex ratios (e.g., Charlat et al., 2003). Empirical evidence suggests that switches from Wolbachia-driven to genetic sex determination may take place in different taxa (Charlat et al., 2003), including terrestrial isopods (O’Neill et al., 1997). Given these observations, and known Wolbachia effects in other arthropods, including isopods, A. aquaticus is an excellent candidate to evaluate how interactions between host genetics and reproductive symbionts influence sex determination, and to provide insight into the eco-evolutionary implications of these interacting processes.
Epibionts
In addition to the various internal microbes referred to above, aquatic crustaceans, including A. aquaticus, also host specialized epibiotic communities (e.g., bacteria, diatoms, protozoa, and rotifers) on their exoskeleton (Cook et al., 1998; Fontaneto and Ambrosini, 2010). While it is generally assumed that the only benefit is phoretic (i.e., mode of transportation for the epibiont; May, 1989), it has also been argued that certain rotifer species may feed on host-derived material (e.g., feces or bacteria; Beauchamp, 1932). Studies in pelagic species, such as copepods and cladocerans, suggest that surface colonization by protozoans may also be associated with predator avoidance (Steffan, 1967; Thiery and Cazaubon, 1992), increased oxygen uptake (Steffan, 1967; Thiery and Cazaubon, 1992), or greater intake of suspended matter (Green, 1974). The extensive colonization of certain body regions or organs, such as the gills in A. aquaticus, could also be harmful and potentially indicate a parasitic association (Cook et al., 1998). However, despite epibionts being common in aquatic invertebrates, we still know little about the nature of these colonizations and the impact they may have on host performance (Cook et al., 1998).
Interestingly, in A. aquaticus the composition of epibiotic communities can vary within (e.g., between dorsal and ventral body parts) and across individuals, as well as between populations (Cook et al., 1998). Asellus aquaticus may be a particularly suitable system to investigate the spatial and temporal dynamics of epibionts communities on their host. The bi-phasic sequential molting of A. aquaticus (Box 1) will induce time-restricted dispersal and recolonization events for epibionts (Cook et al., 1998) that could be easily traced. Moreover, organisms with bi-phasic molting never shed the entire exoskeleton at once, which may allow for more stable associations between hosts and epibionts compared to other arthropods. Further work in this direction could help elucidate the ecological and evolutionary significance of epibiont–host organism interactions.
Biotic Interactions
Interactions between organisms and their environment can influence organismal performance and evolutionary trajectories and, when involving keystone species such as A. aquaticus, have overreaching consequences for the entire food web or ecosystem (e.g., Visser et al., 2012). Classic examples in different taxa illustrate the extent to which competitive, predator-prey, and host-parasite interactions can influence ecosystem function (Levine, 1976; Berryman, 1992; Gandon and Van Zandt, 1998; Abrams, 2000; MacColl, 2011), such as, for instance, through trophic cascades (Best and Stachowicz, 2012; Walsh et al., 2016).
Asellus aquaticus is relevant for ecosystem functioning due to its role in organic matter processing and nutrient cycling within aquatic ecosystems, as well as in the aquatic-terrestrial interphase arising from its detritivory (i.e., it typically feeds on leaf litter fallen from trees) (Carpenter and Lodge, 1986; Graça et al., 1994; Bjelke and Herrmann, 2005). Moreover, A. aquaticus is an important component of aquatic food webs, as it serves as a prey for mesopredators (e.g., Hart and Gill, 1992; Hargeby et al., 2004) and as a host for trophically transmitted parasites (e.g., Dezfuli, 2000). However, and despite a long history of research on A. aquaticus, surprisingly little is known about the extent to which interactions between these isopods and their environment link ecosystem and evolutionary processes (i.e., eco-evolutionary dynamics) (Hairston et al., 2005, Matthews et al., 2011; Schoener, 2011; Miner et al., 2012; Reznick et al., 2019). Given the high degree of phenotypic variation (e.g., Hargeby et al., 2005; Lürig et al., 2019), and its role as a keystone species, A. aquaticus is a well-suited model for evolutionary ecosystem studies, such as those related to resource use and biotic interactions.
Detritivory and Competition
Among and within species variation in the ability to tolerate suboptimal environmental conditions and to utilize diverse diets can be major determinants of species distribution and competitive exclusion (Hoffmann and Hercus, 2000; Liancourt et al., 2005; de Araújo et al., 2014). Competitive interactions may be particularly relevant when considering keystone species, such as A. aquaticus, as they have the potential to influence key ecosystem processes (Lindeman, 1991; Bianchi, 2020). Benthic detritivores, in general, play a central role for the functioning of ecosystems aquatic environments with high productivity (Jeppesen et al., 1998; Bjelke and Herrmann, 2005; Adey and Loveland, 2007; Kenna et al., 2017). Therefore, differential environmental tolerances and competitive exclusion between co-existing benthic detritivore species can have important ecosystem level effects (Wallace and Webster, 1996; Little et al., 2019).
Asellus aquaticus seems to be a strong competitor to more environmentally sensitive taxa: it is resilient to both low oxygen levels (Edwards and Learner, 1960; Maltby, 1995; Bjelke, 2005) and high levels of unionized ammonia (Maltby, 1995), which presumably allows A. aquaticus to occupy a broader range of oligo- and eutrophic benthic microhabitats than some other detritivore taxa (e.g., Hargeby, 1990a; Graça et al., 1994; Bjelke, 2005). It has even been suggested that interspecific differences in physical and chemical tolerances may explain the non-overlapping distribution patterns between A. aquaticus and G. pulex (Costantini et al., 2005), a common gammarid amphipod that competes with A. aquaticus for space and for detrital resources (Åbjörnsson et al., 2000; Van den Brink et al., 2017). Specifically, A. aquaticus tolerates pollution better than does G. pulex, which may explain why it often dominates in polluted areas (Whitehurst and Lindsey, 1990; Whitehurst, 1991).
Another aspect that presumably makes A. aquaticus a strong competitor in benthic detrital food chains is its ability to use a broad range of dietary resources (Marcus et al., 1978; Willoughby and Marcus, 1979; Graça et al., 1993b; Lürig and Matthews, 2021). Asellus aquaticus is, in fact, able to use diets that important competitors cannot utilize. It is possible that a specific gut microbial community facilitates the digestion of epiphytic fungi and bacteria (see also section “Microbial Communities and Diet”), which then allows A. aquaticus to harness dietary sources that are not available to other benthic detritivores. The ability to digest fungi may be especially beneficial because of their high nutritional value and near optimal elemental ratios (i.e., low C:P and C:N ratios; Elser et al., 2000), which in turn, can positively affect growth and pose a competitive advantage for A. aquaticus.
Given the aforementioned specific trophic features, and the possibility for diet manipulations, A. aquaticus is amenable to tackling a broad range of food web related topics. Interesting and ecologically relevant questions include aspects related to differential resource base (e.g., biofilm versus leaf litter; Grieve and Lau, 2018; Santschi et al., 2018; Gossiaux et al., 2020) and to how species-specific responses to diverse pollutants (e.g., pesticides versus fungicides) influence food webs (Burdon et al., 2016; Feckler et al., 2016; Stamm et al., 2016).
Predator-Prey Interactions
Benthic species, such as A. aquaticus, are a key food source for mesopredators in aquatic ecosystems and disturbances in their populations can have far-reaching consequences (Rask and Hiisivuori, 1985; Brooks et al., 2009), and strongly influence nutrient cycling across food webs (Schmitz et al., 2018). Hence, understanding predator-prey interactions of keystone species can provide core insight into the role of biotic interactions in nature. Asellus aquaticus is an important component of aquatic food webs (Murphy and Learner, 1982; Hart and Ison, 1991; Graça et al., 1994; Persson and Eklöv, 1995) because it is a prey species to different invertebrate (e.g., insects; Thompson, 1978; Cockrell, 1984; Brooks et al., 2009) and vertebrate predators (e.g., fish, Rask and Hiisivuori, 1985; Hart and Gill, 1992; Salvanes and Hart, 1998; Harris et al., 2011). Locally, isopods can make up a very large proportion of a predator’s diet (up to 70%) (Rask and Hiisivuori, 1985) and can therefore be a main factor in determining the occurrence and abundance of predator species (Herrmann, 1984).
Predation can also be a major evolutionary driver behind trait divergence, from behavior to morphology and life histories (Johnson and Belk, 2020). Predator avoidance mechanisms range from behavioral avoidance to cryptic coloration and are sometimes highly dependent on the type of microhabitat (e.g., with or without shelter) and on how individual phenotypes match the microhabitat (e.g., via crypsis) (e.g., Edelaar et al., 2008, 2017; Ruxton et al., 2019; van Bergen and Beldade, 2019). For organisms such as A. aquaticus that typically hide in vegetation, macrophyte patches or leaf litter deposits can offer both nutritional resources and shelter from predation either via direct structural effect or via cryptic background matching (Hargeby et al., 2005; Warfe et al., 2008; Kovalenko et al., 2012). Intriguingly, A. aquaticus has evolved cryptic pigmentation presumably as a consequence of visual predation, whereby close matching of isopod body color and microhabitat background color has been found (Hargeby et al., 2004, 2005; Lürig et al., 2019) (see section “Ecotype Divergence Within Lakes” and Figure 2).
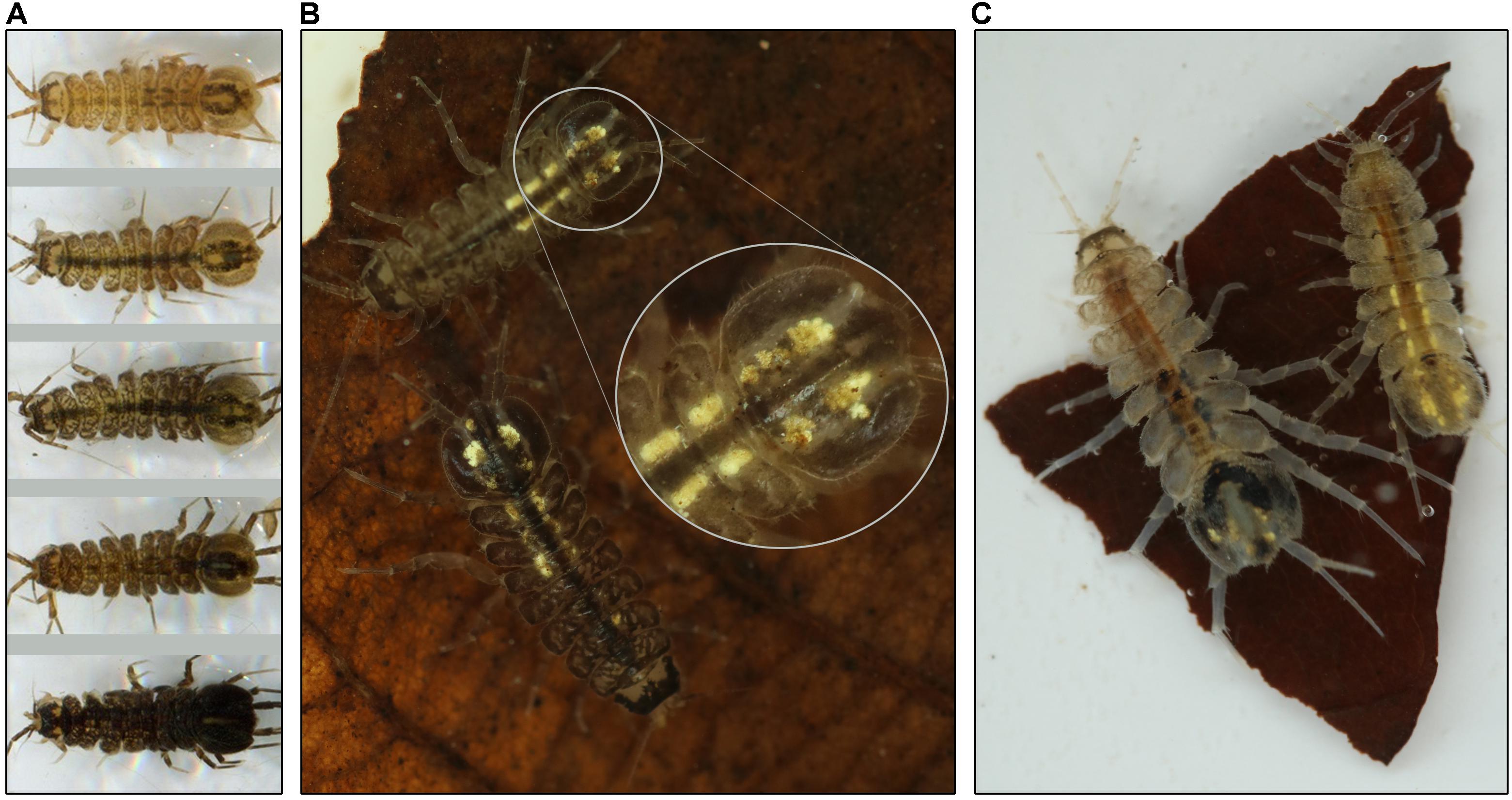
Figure 2. Determinants of body pigmentation in Asellus aquaticus. (A) Images show differences in pigmentation between individuals of A. aquaticus. Pigmentation in this species is a highly variable trait, ranging from light to dark brown in surface water forms (e.g., Hargeby et al., 2004, 2005; Lürig et al., 2019) to nearly colorless cave forms (Protas et al., 2011). Similar to other isopods, integumental pigmentation is mainly composed of the ommochromes xanthommatin and ommatin, which are deposited inside specialized skin cells (Needham, 1970). (B) Image showing two individuals of A. aquaticus with visible Zenker cells, which are a specialized cell type homologous to the tegumental glands of terrestrial isopods (Ter-Poghossian, 1909; Gorvett, 2009). In A. aquaticus, the Zenker cells form conspicuous fluorescent yellow stripes along thoracic and abdominal segments (Zimmer et al., 2002b). Zenker cells help in the storage of metabolic waste products, such as uric acid, which appears in large amounts in A. aquaticus (Lockwood, 1959). The storage of uric acid, along with increased pigmentation, has been proposed as a mechanism to avoid cell toxicity (Linzen, 1974). Furthermore, the ecological relevance of the conspicuousness produced by Zenker cells has been associated with predation (Zimmer et al., 2002b), in that the fluorescence emitted by Zenker cells causes intraspecific variation in external patterns, affecting individual visibility, and influencing predation risk (Zimmer et al., 2002b). (C) Image of individuals of A. aquaticus infected with Acanthocephalan parasites, which have disruptive effects on the pigmentation patterns (Oetinger and Nickol, 1981). Infected individuals generally show darker pigmentation in the posterior-most region of the body (Needham, 1974; Dezfuli et al., 1994), making them more conspicuous (Dezfuli et al., 1994), and potentially influencing trophic transmission to the next host (i.e., different species of freshwater fish) (e.g., Hasu et al., 2007; Benesh et al., 2009).
There is increasing evidence for a tight relationship between behavior, morphology, and performance (Garland and Losos, 1994), as well as for variation in that relationship across environments (Broom and Ruxton, 2005; Lürig et al., 2016). However, the extent to which variation in that relationship, in turn, influences ecoysytem function, is still poorly understood. Furthermore, anti-predator responses can come at a cost to other performance traits, which may also influence ecosystem function. For instance, in A. aquaticus, predator presence decreases feeding efficiency (Åbjörnsson et al., 2000; Calizza et al., 2013) and thus, it can indirectly affect nutrient cycling and decomposition of organic material. Given the potential for predator-prey interactions and phenotypic habitat matching in A. aquaticus, this species is suitable for investigating the impact of differential predation pressures on ecosystem function.
Host-Parasite Interactions
Another type of biotic interaction in A. aquaticus that has the potential to strongly influence ecosystem function is that of host-parasite interactions involving multiple trophic levels. Host-parasite interactions can influence population performance and dynamics at different scales (e.g., Penczykowski et al., 2016) from within-host (e.g., direct effect of parasites on host survival or reproduction) up to community-level effects (e.g., impact on the structure of the communities of their hosts) (Dobson and Hudson, 1986; Minchella and Scott, 1991). This is especially true in the case of transmission of parasites across two trophic levels via keystone species, such as A. aquaticus and fish.
Host-parasite interactions that have been best studied in A. aquaticus are those between the isopod and acanthocephalan parasites, including Acanthocephalus lucii, A. anguillae, and A. balkanicus (Brattey, 1983; Dezfuli, 2000; Amin et al., 2019). Species of acanthocephalans that frequently and specifically infect A. aquaticus have different species of fish – for example, perch, stickleback and/or eels – as their subsequent and final host (Dezfuli et al., 1994; Dezfuli, 2000; Lyndon and Kennedy, 2001; Hasu et al., 2007). The adult acantocephalan worms reside and reproduce in the fish intestine and transmission to A. aquaticus occurs when the isopod ingests the parasite’s eggs that have been released to the environment via fish feces. Once inside the isopod host, the eggs hatch, penetrate the gut wall, and the parasite subsequently develops inside the host body cavity (Dezfuli, 2000). Intriguingly, these trophically transmitted parasites are known for host manipulation, such as the induction of behavioral changes (e.g., infected isopods hide less than uninfected isopods; Benesh et al., 2008; Seppälä et al., 2008), increased body size, and/or changes in pigmentation (Brattey, 1983; Hasu et al., 2007; Benesh et al., 2009; see Figure 2). These parasites can also castrate female isopods by inhibiting secondary sexual development (Brattey, 1983; Dezfuli et al., 1994). While little is known about the consequences of parasitism on individuals and populations of A. aquaticus, the transmission of parasites across trophic levels, appeals to using this species in studies exploring ecosystem consequences and eco-evolutionary feedbacks of host-parasite interactions.
Phenotypic Divergence
Asellus aquaticus provides some fascinating examples of phenotypic variation across different temporal (i.e., within and between generations) and spatial (e.g., continental versus local) scales, making it a well-suited system to study the evolution of biological diversity. On a continental scale, multiple subspecies have formed throughout the species range (Box 2) and cave forms have evolved in multiple karstic areas in Europe (e.g., Konec et al., 2015; Mojaddidi et al., 2018). The latter has led to subterranean phenotypic adaptation and to the formation of troglomorphic subspecies (e.g., Kosswig and Kosswig, 1940; Turk-Prevorčnik and Blejec, 1998). On a regional scale, A. aquaticus has undergone repeated ecotype differentiation within lakes (Eroukhmanoff et al., 2009a,b), with divergence having been associated to both genetic (e.g., Bakovic et al., 2021) and plastic effects (Karlsson et al., 2010). Locally, A. aquaticus shows evidence of assortative mating (e.g., Ridley and Thompson, 1979; Adams et al., 1985), which has the potential to contribute to sexual selection and reproductive isolation (Jiang et al., 2013).
Available evidence indicates that the extensive phenotypic variation in A. aquaticus is driven, at least in part, by natural selection, offering an opportunity to explore the relationship between environmental variation and adaptive diversification (Nosil, 2012). In general, when environmental variation is spatially structured and gene flow is restricted, natural selection can promote local adaptation (Kawecki and Ebert, 2004; Hereford, 2009), whilst high spatio-temporal environmental variation can favor phenotypic plasticity (Via and Lande, 1985; Schlichting and Pigliucci, 1998; DeWitt and Scheiner, 2004; Lafuente and Beldade, 2019). Ultimately, the relationship between local adaptation and plasticity depends on the magnitude of the environmental differences and on the potential for dispersal and gene flow (Sultan and Spencer, 2002; Garant et al., 2007; Ghalambor et al., 2007; Räsänen and Hendry, 2008). The broad range of natural and man-made environments that the species inhabits, and the different temporal and spatial scales on which phenotypic variation occurs, make A. aquaticus well-suited for increasing our understanding of adaptive divergence across different scales.
Surface-Cave Divergence
The ability A. aquaticus to adapt to a broad range of conditions is particularly striking in surface versus cave environments in different parts of Europe (Kosswig and Kosswig, 1940; Sket, 1994; Turk et al., 1996; Turk-Prevorčnik and Blejec, 1998; Verovnik et al., 2004, reviewed in Verovnik and Konec, 2019). Generally, cave living organisms often show a pattern of multiple independently evolved populations (e.g., Bradic et al., 2013), and thus represent great systems to study mechanisms of adaptation and the prevalence (or lack thereof) of convergent and parallel evolution (e.g., Jeffery, 2009; Liu et al., 2017). Studies on surface-cave divergence of A. aquaticus have thus far provided the most extensive and detailed genetic and developmental analyses (and resources) for this species, which has resulted in extensive efforts to establish this species as an invertebrate model for studies of cave evolution (Protas et al., 2011; Konec et al., 2015; Mojaddidi et al., 2018; Verovnik and Konec, 2019). Based on molecular genetic analyses, the colonization of cave habitats happened independently in several locations, suggesting parallel or convergent evolution in subterranean populations (Konec et al., 2015). Closely related (and potentially ancestral) surface populations are still existent in many locations, and cave and surface forms can interbreed in captivity, which suggests incomplete reproductive isolation, and facilitates genetic and phenotypic analyses (Mojaddidi et al., 2018; Re et al., 2018).
As in many other cave-adapted taxa (e.g., Yoshizawa et al., 2012; Soares and Niemiller, 2013), cave populations of A. aquaticus show reduced or absent ommatidia (i.e., optical units that make up the compound eye of arthropods), depigmentation, elaborated sensory structures, and elongation of some appendages (Turk et al., 1996; Prevorcnik et al., 2004; Konec et al., 2015). Genetic mapping analyses have revealed that pigmentation (Figure 2) and eye loss are under the control of a few genes of large effect, with closely related traits (e.g., eye loss and eye reduction) mapping to distinct regions (Protas et al., 2011). Interestingly, the same genomic regions (and even same genes) underlie the convergent loss of pigmentation in two different cave populations of A. aquaticus (Pivka and Rak Channels in Slovenia; Re et al., 2018), potentially indicating that evolutionary change may have happened via fixation of standing genetic variation from an ancestral surface lineage (Re et al., 2018).
In contrast to their surface water ancestors, cave-living A. aquaticus show physiological differences involving energy saving mechanisms, such as lower locomotor and metabolic activity (Jemec et al., 2017), as well as differences in energy biomarkers (Zidar et al., 2018). Similar physiological specializations have been described for other cave crustaceans (Hervant et al., 1997, 1999; Simcic et al., 2005). The reduced locomotion of cave-dwelling A. aquaticus has been taken as an indication that cave adaptation is primarily driven by the increased stability of cave environments (Jemec et al., 2017; Verovnik and Konec, 2019). However, phenotypic divergence in morphometric traits, which appears to have happened independently of ecological parameters (e.g., nutrient availability or habitat type; Konec et al., 2015), has been taken as evidence for a role of darkness as a main selective agent (Culver et al., 2010). Further work on A. aquaticus could help understanding how different environmental factors (and their interactions) influence phenotypic divergence in cave animals (Culver et al., 2010; Pipan and Culver, 2012; Soares and Niemiller, 2013; Gross et al., 2014).
Many of the morphological differences (e.g., pigmentation, eye size, and antennal article number) between cave and surface forms of A. aquaticus arise during embryonic development (Mojaddidi et al., 2018) and involve the differential expression of genes with diverse putative functions, such as phototransduction, photoreception, and eye development (Gross et al., 2019). Transcriptomic analyses of cave (versus surface) individuals also revealed allele-specific gene expression (Stahl et al., 2015; Gross et al., 2019), suggesting that cis-regulatory changes in gene expression may be responsible for phenotypic differences. Since cis-regulatory changes are known to constitute an important part of the genetic basis of adaptation (see Wray, 2007), A. aquaticus could provide insight into the contribution of cis-regulatory changes to cave adaptation. Moreover, studies using this species in comparison with other taxa, could help to elucidate the extent to which cave related traits are under the control of the same or of different genes in independent phylogenetic lineages.
Ecotype Divergence Within Lakes
Among the best-studied cases of phenotypic and ecological differentiation in A. aquaticus is the intralacustrine divergence into distinct ecotypes, which provides an excellent opportunity to study microevolutionary responses in both space and time. In several different lakes in Sweden, A. aquaticus has – over the last two decades – diverged phenotypically into two different ecotypes that associate with different vegetation types (Eroukhmanoff and Svensson, 2009, 2011; Harris et al., 2011): the ancestral reed (Phragmites australis) habitat and the novel stonewort (Chara tomentosum) habitat (Hargeby et al., 2004, 2005; Eroukhmanoff et al., 2009a). Phenotypic divergence in morphology and behavior in A. aquaticus is thought to have happened as the result of differences in visual predation pressure; invertebrate predators are most prevalent in reed habitats, while fish dominate the stonewort habitat (Hargeby et al., 2004; Eroukhmanoff and Svensson, 2009, 2011). These ecological conditions have favored crypsis in stoneworts, with isopods having smaller bodies, lighter pigmentation, and being behaviorally more cautious than those inhabiting reed habitats (Hargeby et al., 2004; Eroukhmanoff and Svensson, 2009, 2011).
Furthermore, mate choice experiments between individuals from the two ecotypes indicate that the combined effects of natural selection and size-assortative mating may have promoted morphological differentiation (Hargeby and Erlandsson, 2006). In addition to color polymorphism and anti-predator traits, demographic parameters also differ between the reed and stonewort ecotypes, with population densities being generally lower in the reed than in the stonewort habitat (Karlsson et al., 2010). These differences in demography have been associated with the differences in sexual behavior whereby the stonewort ecotype shows lower propensity to initiate precopula than the ancestral reed ecotype (Karlsson et al., 2010). At the same time, mating propensity is plastic (i.e., dependent on sex ratio) in the novel stonewort ecotype but not in the ancestral reed ecotype (Karlsson et al., 2010), suggesting that variation in mate choice (and its plasticity) may have contributed to ecotype divergence (Karlsson et al., 2010).
Anti-predator specializations have been found in several geographically separate and genetically independent stonewort-inhabiting populations in Sweden, indicative of parallel evolution (Hargeby et al., 2005; Eroukhmanoff and Svensson, 2009; Eroukhmanoff et al., 2009a). This divergence is, at least partly, under genetic control, such as seen for divergence in pigmentation between ecotypes (Hargeby et al., 2004). Molecular data suggests that A. aquaticus populations adapted to stonewort habitats have emerged independently and repeatedly, rather than emerged in one of the lakes and then subsequently dispersed to the others (Eroukhmanoff et al., 2009a).
Similar to the threespine stickleback model of ecological speciation (reviewed in Hendry et al., 2009) and other freshwater fish (Seehausen and Wagner, 2014; Skúlason et al., 2019), A. aquaticus occurs at wide geographic scales and in areas with different degrees of connectivity (e.g., across lakes, lake-stream, surface water-cave, and within lakes) providing the opportunity for future avenues of research on selection-gene flow balance and the speciation continuum (Nosil, 2012). In this vain, A. aquaticus constitutes an excellent invertebrate model system to study the mechanisms underlying parallel evolution in multiple independently evolved populations of a single species (Losos et al., 1998; Schluter et al., 2004; Boughman et al., 2005). Intralacustrine divergence further provides potential for understanding the interplay between natural selection (e.g., via vegetation and predation) and gene flow (e.g., effect of geographic distance and habitat suitability on potential for dispersal within ecosystems) (Richardson et al., 2014).
Sexual Selection and Assortative Mating
Assortative mating, whereby individuals with particular phenotypes mate with one another in a non-random fashion, is a main contributor to sexual selection (Jiang et al., 2013; Kopp et al., 2018), and has the potential to drive reproductive isolation (Lande, 1981; Panhuis et al., 2001; Ritchie, 2007). One of the most prevalent forms of assortative mating in many taxa, including A. aquaticus, is that with respect to size (i.e., size-assortative mating) (Harari et al., 1999; Shine et al., 2001; Jiang et al., 2013; Green, 2019).
Asellus aquaticus shows sexual dimorphism in body size: for any given age, males are larger than females (Box 1). Moreover, within populations, mating is size assortative in that larger males tend to pair with larger females (Ridley and Thompson, 1979). Mating in A. aquaticus is assortative also with respect to fluorescence (produced by Zenker cells), but the relevance of this is little studied so far (but see Zimmer et al., 2002b; Figure 2). Several lines of evidence suggest that sexual selection is important in A. aquaticus. Mating is preceded by a precopulatory stage (called amplexus or mate guarding), whereby males grasp onto the females with specialized legs (i.e., specialized fourth pair of pereopods) and wait for days until the female molts into a fertile state (see Box 1). Inter-male competition is likely to influence patterns of non-random mating as larger males are more likely to pair, and to displace small males from pairs (Verspoor, 1982; Adams et al., 1985; Hargeby and Erlandsson, 2006). In line with this, larger males seem to have a mating advantage (Crespi, 1989), which might determine the observed patterns of assortative mating in A. aquaticus (Ridley and Thompson, 1979).
The precopulatory mate guarding behavior in A. aquaticus imposes an intimate association between body size (and assortative mating) and reproductive success. In other species, precopulatory mate guarding can be subject to sexual conflict (Cameron et al., 2003; Arnqvist and Rowe, 2005; Wedell et al., 2006), mainly because the optimal duration of mate guarding can differ between males and females (Jormalainen et al., 1994; Jormalainen, 1998). In A. aquaticus, the probability of successful mate guarding increases with male size (Ridley and Thompson, 1979; Verspoor, 1982). However, males also tend to choose females that are closer to being fecund (Ridley and Thompson, 1979; Verspoor, 1982). This could potentially represent a compromise between pairing with a larger, more fecund female, and pairing with a smaller, but easier to carry female. All together, the relationship between pre-copulatory mate guarding and assortative mating in A. aquaticus offers an excellent opportunity to test empirically theories on life history evolution (Stearns, 1992; Roff, 1993; Wedell et al., 2006).
Integration and Future Perspectives
As stated at the onset of our review, understanding how organisms and ecosystems respond to environmental change requires integrative efforts that explore organism-environment interactions at different hierarchical levels (Lewontin, 1983; Kokko and López-Sepulcre, 2007; Post and Palkovacs, 2009; Matthews et al., 2014). Here, we have presented A. aquaticus, a species that is broadly distributed, ecologically relevant, experimentally tractable, and that has a well-studied natural history, as a suitable study system for integrative studies across different temporal, spatial, and biological scales. Building on the knowledge that we have synthesized in this review, we next suggest some specific avenues of integrative research using A. aquaticus. We particularly highlight two areas: first, the study of relationships between spatio-temporal environmental variability and phenotypic plasticity, and second, the study of host-microbiome interactions in relation to ecosystem function.
Spatio-Temporal Environmental Variability and Phenotypic Plasticity
Understanding how organisms cope with environmental variation has been a central theme in evolutionary biology and in ecology, and is becoming more relevant in the context of climate change (Charmantier et al., 2008; Chevin et al., 2010; Moritz and Agudo, 2013). Organismal traits can respond to environmental variation in different manners, and the type of adaptive responses depends on the predictability and the timescale of the environmental fluctuations (Leimar et al., 2006; Reed et al., 2010; Botero et al., 2015). Reversible phenotypic changes (via plasticity) are thought to be more likely under rapid or fluctuating environmental change, while genetic adaptation is considered more likely in response to long-term (and often gradual) environmental changes (Rando and Verstrepen, 2007; Stomp et al., 2008). Moreover, phenotypic plasticity is thought to be favored when environmental fluctuations are predictable (Lande, 2009; Botero et al., 2015; Tufto, 2015) and in the presence of gene flow (Sultan and Spencer, 2002; Crispo, 2008). Plasticity is often (but not always) adaptive (i.e., environmentally induced phenotypic change leads to a better match between phenotype and selective environment; e.g., Gotthard and Nylin, 1995; Ghalambor et al., 2007). In such cases, adaptive plasticity can allow populations to persist under heterogeneous environments, including those changing as a consequence of human activity (Charmantier et al., 2008; Merilä and Hendry, 2014; Sgrò et al., 2016).
Asellus aquaticus is well-suited for empirically testing the relationship between environmental variability and the evolution of plasticity (Tufto, 2000; Leimar et al., 2006; Lande, 2009). Given that the species occurs in temporally stable cave and deep water environments (e.g., Turk et al., 1996; Protas et al., 2011), as well as in spatio-temporally variable lakes and streams (e.g., Murphy and Learner, 1982; Hargeby, 1990b; Hargeby et al., 2007), genetic trait polymorphism or plasticity may be favored in different contexts (Sultan and Spencer, 2002; Richardson et al., 2014). The available evidence for ecogeographical variation in phenotypic traits in A. aquaticus provides a solid foundation for future studies aiming to understand genetic and phenotypic variation in fitness related traits.
One trait of particular interest in this context is body pigmentation. As stated above (section “Surface-Cave Divergence” and “Ecotype Divergence Within Lakes”; Figure 2), pigmentation in A. aquaticus is highly variable, may influence predation risk in lakes (Eroukhmanoff and Svensson, 2009; Eroukhmanoff et al., 2009a) and can be dramatically reduced in caves (Verovnik and Konec, 2019). Both surface water and cave studies in A. aquaticus suggest that color polymorphism is, at least in part, genetically determined (Hargeby et al., 2004; Protas et al., 2011). On the other hand, two recent studies also show extensive diet-based plasticity in A. aquaticus pigmentation (Lürig et al., 2019; Lürig and Matthews, 2021). This nutritional plasticity in body color has been hypothesized to help reduce the negative effects of high protein diets. Tryptophan, an amino acid whose concentration can vary strongly across diets, is toxic at high intracellular levels, but by forming inert pigments from soluble tryptophan, arthropods may avoid the negative effects of high protein diets (Linzen, 1974). Along these lines, variation in A. aquaticus pigmentation within lakes could also be explained by an alternative, but not mutually exclusive, hypothesis to predator-mediated selection: variation in pigmentation between habitats could reflect avoidance of cell toxicity when feeding on different dietary resources (e.g., reed versus stonewort).
Cave adapted A. aquaticus provide another intriguing case of evolution of pigmentation – likely in part via developmental plasticity of biochemical pathways (e.g., Bilandžija et al., 2020). The aforementioned different lines of evidence provide the foundations to explore how genes and environmental conditions co-determine the production and evolution of pigmentation and, in a broader sense, of phenotypic variation.
Host-Microbiome Interactions and Ecosystem Function
In the quest of predicting the impact of anthropogenic activity on nature, researchers are trying to understand the relationship between biodiversity and ecosystem functioning (Loreau et al., 2001; Hooper et al., 2005; Hillebrand and Matthiessen, 2009). In the past, this has been mostly studied from the perspective of the effects of environmental stressors on either individuals, populations, or ecosystems (Hoffmann and Parsons, 1997; Steinberg, 2012). However, it is becoming increasingly clear that an isolated perspective on the different scales of ecological organization may be overly simplistic and could lead to imprecise predictions of biodiversity–ecosystem function (Isbell et al., 2018). It is therefore important to take a more integrative perspective investigating biodiversity–ecosystem function across different spatial and temporal scales (Gonzalez et al., 2020).
Asellus aquaticus seems particularly well-suited to explore the inter-connectedness and reciprocal interactions across levels of ecological organization. This is, in particular, because of the potentially tight relationships between host microbiome (see section “Microbial Associations”), host performance, and ecosystem function. For instance, the accumulation and decomposition rate of organic material can be influenced by both environmentally acquired (Bärlocher and Porter, 1986; Bärlocher, 1992) and endosymbiotic microbes in the isopod digestive system (Robson, 1979; Zimmer and Bartholmé, 2003; Hasu et al., 2008). Gut microbial endosymbionts are likely to allow A. aquaticus to exploit a range of different diets (Robson, 1979; Zimmer and Bartholmé, 2003; Hasu et al., 2008; Bredon et al., 2020) (see section “Detritivory and Competition”). Furthermore, gut bacteria can provide protection against secondary infections by intestinal parasites (e.g., Buffie and Pamer, 2013; Knutie, 2018), further strengthening the potential role of the microbiome in host fitness. How variation in microbiome composition influences the processing of different nutritional sources, and the extent to which this affects rates of decomposition and defenses against parasites are, in general, not well understood. Further investigations of microbiome assembly mechanisms in A. aquaticus can help to elucidate whether and how dietary and/or parasitic symbiotic associations cascade to ecosystem function.
Concluding Remarks
Here we have summarized key aspects of knowledge from 150 years of research on A. aquaticus to highlight its potential as a broadly usable model system for the biological realm. The quest to better understand the evolutionary significance and ecological relevance of organism-environment interactions will benefit from integrative efforts that combine concepts and approaches across multiple disciplines. This integrative effort should include research across the interfaces between different disciplines and, importantly, include more animal systems, such as A. aquaticus, that permit inquiries from individual to ecosystem scale (Lewontin, 1983; Kokko and López-Sepulcre, 2007; Post and Palkovacs, 2009; Matthews et al., 2014). Increasing the diversity of study systems in ecology and evolution can also help to unravel the wondrous diversity of evolutionary processes in natural populations and how this diversity influences ecological processes (Bolker, 2014; Duffy et al., 2021).
Author Contributions
EL, ML, BM, and KR conceived the idea for the review. EL wrote the first draft of the manuscript, with contributions from ML and MR. MR carried out research on distribution patterns presented in Box 2. ML conducted bibliographic research presented in Figure 1, both with contributions from EL. EL produced the remaining figures. All authors contributed to the development and discussion of ideas, writing and editing of the manuscript, and approved the submitted version.
Funding
Financial support was provided by Eawag Discretionary Funds to KR (PSP 5221.00492.013.03). ML received financial support from the Swiss National Science Foundation through an Early Postdoc. Mobility Grant with the No. P2EZP3_191804 and from the European Union’s Horizon 2020 Research and Innovation Program under the Marie Skłodowska-Curie grant agreement No. 898932.
Conflict of Interest
The authors declare that the research was conducted in the absence of any commercial or financial relationships that could be construed as a potential conflict of interest.
Publisher’s Note
All claims expressed in this article are solely those of the authors and do not necessarily represent those of their affiliated organizations, or those of the publisher, the editors and the reviewers. Any product that may be evaluated in this article, or claim that may be made by its manufacturer, is not guaranteed or endorsed by the publisher.
Supplementary Material
The Supplementary Material for this article can be found online at: https://www.frontiersin.org/articles/10.3389/fevo.2021.748212/full#supplementary-material
References
Åbjörnsson, K., Dahl, J., Nyström, P., and Brönmark, C. (2000). Influence of predator and dietary chemical cues on the behaviour and shredding efficiency of Gammarus pulex. Aquat. Ecol. 34, 379–387. doi: 10.1023/A:1011442331229
Abrams, P. A. (2000). The evolution of predator-prey interactions: theory and evidence. Annu. Rev. Ecol. Syst. 31, 79–105. doi: 10.1146/annurev.ecolsys.31.1.79
Adams, J., Greenwood, P., Richard, P., and Tania, Y. (1985). Loading constraints and sexual size dimorphism in Asellus aquaticus. Behaviour 92, 277–287.
Adcock, J. A. (1979). Energetics of a population of the isopod Asellus aquaticus: life history and production. Freshw. Biol. 9, 343–355. doi: 10.1111/j.1365-2427.1979.tb01519.x
Adey, W. H., and Loveland, K. (2007). “Detritus and detritivores: the dynamics of muddy bottoms,” in Dynamic Aquaria (Third Edition), eds W. H. Adey and K. Loveland (London: Academic Press), 303–327. doi: 10.1016/B978-0-12-370641-6.50027-3
Alfred, J., and Baldwin, I. T. (2015). New opportunities at the wild frontier. eLife 4:e06956. doi: 10.7554/eLife.06956
Alves, A. M., Leonardo, M. G., Souza, G. T. R., Takemoto, R. M., de Lima, F. S., Tavares, L. E. R., et al. (2019). Occurrence of isopods in two species of snappers (Lutjanidae) from Northeast Brazil. J. Parasitol. Res. 2019:8176283. doi: 10.1155/2019/8176283
Amin, O. M., Heckmann, R. A., Fiser, Z., Zaksek, V., Herlyn, H., and Kostanjsek, R. (2019). Description of Acanthocephalus anguillae balkanicus subsp. n. (Acanthocephala: Echinorhynchidae) from Proteus anguinus Laurenti (Amphibia: Proteidae) and the cave ecomorph of Asellus aquaticus (Crustacea: Asellidae) in Slovenia. Folia Parasitol. 66:15. doi: 10.14411/fp.2019.015
Anderson, E. (1969). Life-cycle and growth of Asellus aquaticus (L.). With special reference to the effects of temperature. Instit. Freshw. Res. Drottningholm 49, 5–26.
Anderson, R. M., and May, R. M. (1982). Coevolution of hosts and parasites. Parasitology 85, 411–426. doi: 10.1017/S0031182000055360
Arakelova, K. S. (2001). The evaluation of individual production and scope for growth in aquatic sow bugs (Asellus aquaticus). Aquat. Ecol. 35, 31–42. doi: 10.1023/A:1011446224456
Arnqvist, G., and Rowe, L. (2005). Sexual Conflict. Princeton, NJ: Princeton University Press. doi: 10.1515/9781400850600
Aston, R. J., and Milner, A. G. P. (1980). A comparison of populations of the isopod Asellus aquaticus above and below power stations in organically polluted reaches of the River Trent. Freshw. Biol. 10, 1–14. doi: 10.1111/j.1365-2427.1980.tb01175.x
Bakovic, V., Martin Cerezo, M. L., Höglund, A., Fogelholm, J., Henriksen, R., Hargeby, A., et al. (2021). The genomics of phenotypically differentiated Asellus aquaticus cave, surface stream and lake ecotypes. Mol. Ecol. 30, 3530–3547. doi: 10.1111/mec.15987
Balian, E. V., Lévêque, C., Segers, H., and Martens, K. (2008). Freshwater Animal Diversity Assessment. Berlin: Springer Science & Business Media. doi: 10.1007/978-1-4020-8259-7
Bärlocher F. (ed.) (1992). The Ecology of Aquatic Hyphomycetes. Berlin: Springer. doi: 10.1007/978-3-642-76855-2
Bärlocher, F., and Porter, C. W. (1986). Digestive enzymes and feeding strategies of three stream invertebrates. J. N. Am. Benthol. Soc. 5, 58–66. doi: 10.2307/1467747
Barnett, J. A. (2007). A history of research on yeasts 10: foundations of yeast genetics. Yeast 24, 799–845. doi: 10.1002/yea.1513
Beauchamp, P. M. D. (1932). Deux nouveaux cas de phoresie chez les Rotiferes. Arch. Zool. Exp. Gén. 14, 385–398.
Becking, T., Chebbi, M. A., Giraud, I., Moumen, B., Laverré, T., Caubet, Y., et al. (2019). Sex chromosomes control vertical transmission of feminizing Wolbachia symbionts in an isopod. PLoS Biol. 17:e3000438. doi: 10.1371/journal.pbio.3000438
Bedford, N. L., and Hoekstra, H. E. (2015). The natural history of model organisms: Peromyscus mice as a model for studying natural variation. eLife 4:e06813. doi: 10.7554/eLife.06813
Benesh, D. P., Seppälä, O., and Valtonen, E. T. (2009). Acanthocephalan size and sex affect the modification of intermediate host colouration. Parasitology 136, 847–854. doi: 10.1017/S0031182009006180
Benesh, D. P., Valtonen, E. T., and Seppälä, O. (2008). Multidimensionality and intra-individual variation in host manipulation by an acanthocephalan. Parasitology 135, 617–626. doi: 10.1017/S0031182008004216
Bergland, A. O., Behrman, E. L., O’Brien, K. R., Schmidt, P. S., and Petrov, D. A. (2014). Genomic evidence of rapid and stable adaptive oscillations over seasonal time scales in Drosophila. PLoS Genet. 10:e1004775. doi: 10.1371/journal.pgen.1004775
Berryman, A. A. (1992). The origins and evolution of predator-prey theory. Ecology 73, 1530–1535. doi: 10.2307/1940005
Bertin, A., David, B., Cézilly, F., and Alibert, P. (2002). Quantification of sexual dimorphism in Asellus aquaticus (Crustacea: Isopoda) using outline approaches. Biol. J. Linn. Soc. 77, 523–533. doi: 10.1046/j.1095-8312.2002.00125.x
Best, R. J., and Stachowicz, J. J. (2012). Trophic cascades in seagrass meadows depend on mesograzer variation in feeding rates, predation susceptibility, and abundance. Mar. Ecol. Prog. Ser. 456, 29–42. doi: 10.3354/meps09678
Bianchi, T. S. (2020). The evolution of biogeochemistry: revisited. Biogeochemistry 154, 141–181. doi: 10.1007/s10533-020-00708-0
Bilandžija, H., Hollifield, B., Steck, M., Meng, G., and Ng, M. (2020). Phenotypic plasticity as a mechanism of cave colonization and adaptation. eLife 9:e51830. doi: 10.7554/eLife.51830.sa2
Bjelke, U. (2005). Processing of leaf matter by lake-dwelling shredders at low oxygen concentrations. Hydrobiologia 539, 93–98. doi: 10.1007/s10750-004-3369-6
Bjelke, U., and Herrmann, J. (2005). Processing of two detritus types by lake-dwelling shredders: species-specific impacts and effects of species richness. J. Anim. Ecol. 74, 92–98. doi: 10.1111/j.1365-2656.2004.00901.x
Bloor, M. (2010). Animal standardisation for mixed species ecotoxicological studies: establishing a laboratory breeding programme for Gammarus pulex and Asellus aquaticus. Zool. Baetica 21, 179–190.
Bloor, M. C. (2011). Dietary preference of Gammarus pulex and Asellus aquaticus during a laboratory breeding programme for ecotoxicological studies. Int. J. Zool. 2011:294394. doi: 10.1155/2011/294394
Bohmann, I. (2005). Coarse Detritus in Oligotrophic Lake Littoral Zones – Utilization by Invertebrates and Contribution to Carbon Flow. Småland: University of Kalmar.
Bolker, J. A. (2014). Model species in evo-devo: a philosophical perspective. Evol. Dev. 16, 49–56. doi: 10.1111/ede.12056
Botero, C. A., Weissing, F. J., Wright, J., and Rubenstein, D. R. (2015). Evolutionary tipping points in the capacity to adapt to environmental change. Proc. Natl. Acad. Sci. U.S.A. 112, 184–189. doi: 10.1073/pnas.1408589111
Bouchon, D., Rigaud, T., and Juchault, P. (1998). Evidence for widespread Wolbachia infection in isopod crustaceans: molecular identification and host feminization. Proc. Biol. Sci. R. Soc. 265, 1081–1090. doi: 10.1098/rspb.1998.0402
Bouchon, D., Zimmer, M., and Dittmer, J. (2016). The terrestrial isopod microbiome: an all-in-one toolbox for animal–microbe interactions of ecological relevance. Front. Microbiol. 7:252. doi: 10.3389/fmicb.2016.01472
Boughman, J. W., Rundle, H. D., and Schluter, D. (2005). Parallel evolution of sexual isolation in sticklebacks. Evol. Int. J. Org. Evol. 59, 361–373. doi: 10.1111/j.0014-3820.2005.tb00995.x
Bradic, M., Teotónio, H., and Borowsky, R. L. (2013). The population genomics of repeated evolution in the blind cavefish Astyanax mexicanus. Mol. Biol. Evol. 30, 2383–2400. doi: 10.1093/molbev/mst136
Brasiello, A. R., and Tadini, G. V. (1969). Autoradiographic study of spermatogenesis in Asellus aquaticus during reproductive stasis. Caryologia 22, 323–330. doi: 10.1080/00087114.1969.10796351
Brattey, J. (1983). The effects of larval Acanthocephalus lucii on the pigmentation, reproduction, and susceptibility to predation of the isopod Asellus aquaticus. J. Parasitol. 69, 1172–1173. doi: 10.2307/3280892
Bredon, M., Herran, B., Bertaux, J., Grève, P., Moumen, B., and Bouchon, D. (2020). Isopod holobionts as promising models for lignocellulose degradation. Biotechnol. Biofuels 13:49. doi: 10.1186/s13068-020-01683-2
Bredon, M., Herran, B., Lheraud, B., Bertaux, J., Grève, P., Moumen, B., et al. (2019). Lignocellulose degradation in isopods: new insights into the adaptation to terrestrial life. BMC Genomics 20:462. doi: 10.1186/s12864-019-5825-8
Breeuwer, J. A., and Jacobs, G. (1996). Wolbachia: intracellular manipulators of mite reproduction. Exp. Appl. Acarol. 20, 421–434. doi: 10.1007/BF00053306
Broly, P., Deville, P., and Maillet, S. (2013). The origin of terrestrial isopods (Crustacea: Isopoda: Oniscidea). Evol. Ecol. 27, 461–476. doi: 10.1007/s10682-012-9625-8
Brooks, A. C., Gaskell, P. N., and Maltby, L. L. (2009). Sublethal effects and predator-prey interactions: implications for ecological risk assessment. Environ. Toxicol. Chem. 28, 2449–2457. doi: 10.1897/09-108.1
Broom, M., and Ruxton, G. D. (2005). You can run—or you can hide: optimal strategies for cryptic prey against pursuit predators. Behav. Ecol. 16, 534–540. doi: 10.1093/beheco/ari024
Brownlie, J. C., Cass, B. N., Riegler, M., Witsenburg, J. J., Iturbe-Ormaetxe, I., McGraw, E. A., et al. (2009). Evidence for metabolic provisioning by a common invertebrate endosymbiont, Wolbachia pipientis, during periods of nutritional stress. PLoS Pathog. 5:e1000368. doi: 10.1371/journal.ppat.1000368
Brunet, M., Arnaud, J., and Mazza, J. (1994). Gut structure and digestive cellular processes in marine Crustacea. Oceanogr. Mar. Biol. Annu. Rev. 32, 335–367.
Brusca, R. C., and Iverson, E. W. (1985). A guide to the marine isopod crustacea of pacific Costa Rica. Rev. Biol. Trop. 33, 1–77.
Brusca, R. C., and Wilson, G. D. F. (1991). A phylogenetic analysis of the Isopoda with some classificatory recommendations. Mem. Queensl. Museum 31, 143–204.
Buffie, C. G., and Pamer, E. G. (2013). Microbiota-mediated colonization resistance against intestinal pathogens. Nat. Rev. Immunol. 13, 790–801. doi: 10.1038/nri3535
Burdon, F. J., Reyes, M., Alder, A. C., Joss, A., Ort, C., Räsänen, K., et al. (2016). Environmental context and magnitude of disturbance influence trait-mediated community responses to wastewater in streams. Ecol. Evol. 6, 3923–3939. doi: 10.1002/ece3.2165
Calizza, E., Rossi, L., and Costantini, M. L. (2013). Predators and resources influence phosphorus transfer along an invertebrate food web through changes in prey behaviour. PLoS One 8:e65186. doi: 10.1371/journal.pone.0065186
Cameron, E., Day, T., and Rowes, L. (2003). Sexual conflict and indirect benefits. J. Evol. Biol. 16, 1055–1060. doi: 10.1046/j.1420-9101.2003.00584.x
Cao, L.-J., Jiang, W., and Hoffmann, A. A. (2019). Life history effects linked to an advantage for wAu Wolbachia in Drosophila. Insects 10:126. doi: 10.3390/insects10050126
Carefoot, T. H., and Taylor, B. E. (1995). “Ligia: a prototypal terrestrial isopod,” in Terrestrial Isopod Biology, ed. A. M. Alikhan (Rotterdam: Balkema), 47–60.
Carlisle, D. B. (1956). Studies on the endocrinology of isopod crustaceans. Moulting in Ligia oceanica (L.). J. Mar. Biol. Assoc. U.K. 35, 515–520. doi: 10.1017/S0025315400010377
Carpenter, S. R., and Lodge, D. M. (1986). Effects of submersed macrophytes on ecosystem processes. Aquat. Bot. 26, 341–370. doi: 10.1016/0304-3770(86)90031-8
Chang, E. S. (1986). “Integument, pigments, and hormonal processes,” in The Biology of Crustacea, Volume 9. The Quarterly Review of Biology, ed. D. Bliss (Amsterdam: Elsevier). doi: 10.1086/414981
Chang, E. S., and Mykles, D. L. (2011). Regulation of crustacean molting: a review and our perspectives. Gen. Comp. Endocrinol. 172, 323–330. doi: 10.1016/j.ygcen.2011.04.003
Charlat, S., Hurst, G. D. D., and Merçot, H. (2003). Evolutionary consequences of Wolbachia infections. Trends Genet. 19, 217–223. doi: 10.1016/S0168-9525(03)00024-6
Charlesworth, D., Barton, N. H., and Charlesworth, B. (2017). The sources of adaptive variation. Proc. Biol. Sci. 284:20162864. doi: 10.1098/rspb.2016.2864
Charmantier, A., McCleery, R. H., Cole, L. R., Perrins, C., Kruuk, L. E. B., and Sheldon, B. C. (2008). Adaptive phenotypic plasticity in response to climate change in a wild bird population. Science 320, 800–803. doi: 10.1126/science.1157174
Chebbi, M. A., Becking, T., Moumen, B., Giraud, I., Gilbert, C., Peccoud, J., et al. (2019). The Genome of Armadillidium vulgare (Crustacea, Isopoda) provides insights into sex chromosome evolution in the context of cytoplasmic sex determination. Mol. Biol. Evol. 36, 727–741. doi: 10.1093/molbev/msz010
Chevin, L.-M., Lande, R., and Mace, G. M. (2010). Adaptation, plasticity, and extinction in a changing environment: towards a predictive theory. PLoS Biol. 8:e1000357. doi: 10.1371/journal.pbio.1000357
Christensen, S. C. B., Arvin, E., Nissen, E., and Albrechtsen, H.-J. (2013). Asellus aquaticus as a potential carrier of Escherichia coli and other coliform bacteria into drinking water distribution systems. Int. J. Environ. Res. Public Health 10, 845–855. doi: 10.3390/ijerph10030845
Cockrell, B. J. (1984). Effects of temperature and oxygenation on predator-prey overlap and prey choice of Notonecta glauca. J. Anim. Ecol. 53, 519–532. doi: 10.2307/4531
Cook, J. A., Chubb, J. C., and Veltkamp, E. (1998). Epibionts of Asellus aquaticus (L.) (Crustacea, Isopoda): an SEM study. Freshw. Biol. 39, 423–438. doi: 10.1046/j.1365-2427.1998.00286.x
Cordaux, R., Pichon, S., Hatira, H. B. A., Doublet, V., Grève, P., Marcadé, I., et al. (2012). Widespread Wolbachia infection in terrestrial isopods and other crustaceans. ZooKeys 176, 123–131. doi: 10.3897/zookeys.176.2284
Costantini, M. L., Mancinelli, G., Mandrone, S., and Rossi, L. (2005). Combined effects of acidification and competition on the feeding preference of a freshwater macroinvertebrate, Asellus aquaticus (Crustacea: Isopoda): a laboratory experiment. Mar. Freshw. Res. 56, 997–1004. doi: 10.1071/MF04272
Cowdry, E. V. (1923). The distribution of rickettsia in the tissues of insects and Arachnids. J. Exp. Med. 37, 431–456. doi: 10.1084/jem.37.4.431
Cresko, W. A., McGuigan, K. L., Phillips, P. C., and Postlethwait, J. H. (2007). Studies of threespine stickleback developmental evolution: progress and promise. Genetica 129, 105–126. doi: 10.1007/s10709-006-0036-z
Crespi, B. J. (1989). Causes of assortative mating in arthropods. Anim. Behav. 38, 980–1000. doi: 10.1016/S0003-3472(89)80138-1
Crispo, E. (2008). Modifying effects of phenotypic plasticity on interactions among natural selection, adaptation and gene flow. J. Evol. Biol. 21, 1460–1469. doi: 10.1111/j.1420-9101.2008.01592.x
Cross, W. F., Benstead, J. P., Frost, P. C., and Thomas, S. A. (2005). Ecological stoichiometry in freshwater benthic systems: recent progress and perspectives. Freshw. Biol. 50, 1895–1912. doi: 10.1111/j.1365-2427.2005.01458.x
Crotty, S. M., Minh, B. Q., Bean, N. G., Holland, B. R., Tuke, J., Jermiin, L. S., et al. (2019). GHOST: recovering historical signal from heterotachously-evolved sequence alignments. bioRxiv [Preprint]. doi: 10.1101/174789
Culver, D. C., Holsinger, J. R., Christman, M. C., and Pipan, T. (2010). Morphological differences among eyeless amphipods in the genus stygobromus dwelling in different subterranean habitats. J. Crustacean Biol. 30, 68–74. doi: 10.1651/09-3156.1
de Araújo, C. B., Marcondes-Machado, L. O., and Costa, G. C. (2014). The importance of biotic interactions in species distribution models: a test of the Eltonian noise hypothesis using parrots. J. Biogeogr. 41, 513–523. doi: 10.1111/jbi.12234
De Meester, L., Brans, K. I., Govaert, L., Souffreau, C., Mukherjee, S., Vanvelk, H., et al. (2019). Analysing eco-evolutionary dynamics—The challenging complexity of the real world. Function. Ecol. 33, 43–59. doi: 10.1111/1365-2435.13261
DeWitt, T. J., and Scheiner, S. M. (2004). Phenotypic Plasticity: Functional and Conceptual Approaches. Oxford: Oxford University Press.
Dezfuli, B. S. (2000). Host-parasite interface between Asellus aquaticus (Isopoda) and larvae of Acanthocephalus anguillae (Acanthocephala). Folia Parasitol. 47, 154–156. doi: 10.14411/fp.2000.030
Dezfuli, B. S., Rossetti, E., Rossi, R., and Fano, E. A. (1994). Occurrence of larval acanthocephalus Anguillae (acanthocephala) in the Asellus aquaticus (Crustacea, Isopoda) from the river Brenta. Boll. Zool. 61, 77–81. doi: 10.1080/11250009409355862
Dhanasekaran, D., Paul, D., Amaresan, N., Sankaranarayanan, A., and Shouche, Y. S. (2021). Microbiome-Host Interactions. Boca Raton, FL: CRC Press. doi: 10.1201/9781003037521
Dobson, A. P., and Hudson, P. J. (1986). Parasites, disease and the structure of ecological communities. Trends Ecol. Evol. 1, 11–15. doi: 10.1016/0169-5347(86)90060-1
Dobson, S. L., Marsland, E. J., and Rattanadechakul, W. (2002). Mutualistic Wolbachia infection in Aedes albopictus: accelerating cytoplasmic drive. Genetics 160, 1087–1094. doi: 10.1093/genetics/160.3.1087
Dohrn, A. (1867). Die embryonale entwicklung des Asellus aquaticus. Z. Wissenschaftliche Zool. 17, 221–278.
Dooley, K., and Zon, L. I. (2000). Zebrafish: a model system for the study of human disease. Curr. Opin. Genet. Dev. 10, 252–256. doi: 10.1016/S0959-437X(00)00074-5
Douglas, A. E. (1998). Host benefit and the evolution of specialization in symbiosis. Heredity 81, 599–603. doi: 10.1046/j.1365-2540.1998.00455.x
Douglas, A. E. (2014). Symbiosis as a general principle in eukaryotic evolution. Cold Spring Harb. Perspect. Biol. 6:a016113. doi: 10.1101/cshperspect.a016113
Duffy, M. A., García-Robledo, C., Gordon, S. P., Grant, N. A., Green, D. A. II, Kamath, A., et al. (2021). Model systems in ecology, evolution, and behavior: a call for diversity in our model systems and discipline. Am. Nat. 198, 53–68. doi: 10.1086/714574
Duvick, D. N. (2001). Biotechnology in the 1930s: the development of hybrid maize. Nat. Rev. Genet. 2, 69–74. doi: 10.1038/35047587
Edelaar, P., Jovani, R., and Gomez-Mestre, I. (2017). Should I change or should I go? Phenotypic plasticity and matching habitat choice in the adaptation to environmental heterogeneity. Am. Nat. 190, 506–520. doi: 10.1086/693345
Edelaar, P., Siepielski, A. M., and Clobert, J. (2008). Matching habitat choice causes directed gene flow: a neglected dimension in evolution and ecology. Evol. Int. J. Org. Evol. 62, 2462–2472. doi: 10.1111/j.1558-5646.2008.00459.x
Edwards, R. W., and Learner, M. A. (1960). Some factors affecting the oxygen consumption of Asellus. J. Exp. Biol. 37, 706–718. doi: 10.1242/jeb.37.4.706
Elangovan, R., Ballance, S., White, K. N., McCrohan, C. R., and Powell, J. J. (1999). Accumulation of aluminium by the freshwater crustacean Asellus aquaticus in neutral water. Environ. Pollut. 106, 257–263. doi: 10.1016/S0269-7491(99)00117-7
Elser, J. J., Fagan, W. F., Denno, R. F., Dobberfuhl, D. R., Folarin, A., Huberty, A., et al. (2000). Nutritional constraints in terrestrial and freshwater food webs. Nature 408, 578–580. doi: 10.1038/35046058
Eroukhmanoff, F., Hargeby, A., Arnberg, N. N., Hellgren, O., Bensch, S., and Svensson, E. I. (2009a). Parallelism and historical contingency during rapid ecotype divergence in an isopod. J. Evol. Biol. 22, 1098–1110. doi: 10.1111/j.1420-9101.2009.01723.x
Eroukhmanoff, F., Hargeby, A., and Svensson, E. I. (2009b). Rapid adaptive divergence between ecotypes of an aquatic isopod inferred from FST-QST analyses. Mol. Ecol. 18, 4912–4923. doi: 10.1111/j.1365-294X.2009.04408.x
Eroukhmanoff, F., and Svensson, E. I. (2009). Contemporary parallel diversification, antipredator adaptations and phenotypic integration in an aquatic isopod. PLoS One 4:e6173. doi: 10.1371/journal.pone.0006173
Eroukhmanoff, F., and Svensson, E. I. (2011). Evolution and stability of the G-matrix during the colonization of a novel environment. J. Evol. Biol. 24, 1363–1373. doi: 10.1111/j.1420-9101.2011.02270.x
Fano, E. A., Valentino, F., and Vitagliano-Tadini, G. (1976). Different rates of development in two geographical races of Asellus aquaticus, their genetic determination and adaptive significance. Proc. Accad. Nazl. Lincei Class Phys. Math. Nat. Sci. Rep. 8, 297–302.
Feckler, A., Goedkoop, W., Zubrod, J. P., Schulz, R., and Bundschuh, M. (2016). Exposure pathway-dependent effects of the fungicide epoxiconazole on a decomposer-detritivore system. Sci. Tot. Environ. 571, 992–1000. doi: 10.1016/j.scitotenv.2016.07.088
Fielenbach, N., and Antebi, A. (2008). C. elegans dauer formation and the molecular basis of plasticity. Genes Dev. 22, 2149–2165. doi: 10.1101/gad.1701508
Fisher, R. M., Henry, L. M., Cornwallis, C. K., Kiers, E. T., and West, S. A. (2017). The evolution of host-symbiont dependence. Nat. Commun. 8:15973. doi: 10.1038/ncomms15973
Flemming, H.-C., Neu, T. R., and Wozniak, D. J. (2007). The EPS matrix: the ‘house of biofilm cells’. J. Bacteriol. 189, 7945–7947. doi: 10.1128/JB.00858-07
Fontaneto, D., and Ambrosini, R. (2010). Spatial niche partitioning in epibiont rotifers on the waterlouse Asellus aquaticus. Limnol. Oceanogr. 55, 1327–1337. doi: 10.4319/lo.2010.55.3.1327
Foster, K. R., Schluter, J., Coyte, K. Z., and Rakoff-Nahoum, S. (2017). The evolution of the host microbiome as an ecosystem on a leash. Nature 548, 43–51. doi: 10.1038/nature23292
Fraune, S., and Bosch, T. C. G. (2010). Why bacteria matter in animal development and evolution. BioEssays 32, 571–580. doi: 10.1002/bies.200900192
Fuller, N., Ford, A. T., Nagorskaya, L. L., Gudkov, D. I., and Smith, J. T. (2018). Reproduction in the freshwater crustacean Asellus aquaticus along a gradient of radionuclide contamination at Chernobyl. Sci. Tot. Environ. 628, 11–17. doi: 10.1016/j.scitotenv.2018.01.309
Gandon, S., and Van Zandt, P. A. (1998). Local adaptation and host–parasite interactions. Trends Ecol. Evol. 13, 214–216. doi: 10.1016/S0169-5347(98)01358-5
Garant, D., Forde, S. E., and Hendry, A. P. (2007). The multifarious effects of dispersal and gene flow on contemporary adaptation. Funct. Ecol. 21, 434–443. doi: 10.1111/j.1365-2435.2006.01228.x
Garland, T. Jr., and Losos, J. B. (1994). “Ecological morphology of locomotor performance in squamate reptiles,” in Ecological Morphology: Integrative Organismal Biology, eds P. C. Wainwright and S. Reilly (Chicago, IL: University of Chicago Press), 240–302.
George, R. Y. (1972). Biphasic moulting in Isopod Crustacea and the finding of an unusual mode of moulting in the antarctic genus Glyptonotus. J. Nat. Hist. 6, 651–656. doi: 10.1080/00222937200770591
Gessner, M. O., Chauvet, E., and Dobson, M. (1999). A perspective on leaf litter breakdown in streams. Oikos 85, 377–384. doi: 10.2307/3546505
Ghalambor, C. K., McKAY, J. K., Carroll, S. P., and Reznick, D. N. (2007). Adaptive versus non-adaptive phenotypic plasticity and the potential for contemporary adaptation in new environments. Funct. Ecol. 21, 394–407. doi: 10.1111/j.1365-2435.2007.01283.x
Gilbert, S. F., Sapp, J., and Tauber, A. I. (2012). A symbiotic view of life: we have never been individuals. Q. Rev. Biol. 87, 325–341. doi: 10.1086/668166
Gonzalez, A., Germain, R. M., Srivastava, D. S., Filotas, E., Dee, L. E., Gravel, D., et al. (2020). Scaling-up biodiversity-ecosystem functioning research. Ecol. Lett. 23, 757–776. doi: 10.1111/ele.13456
Gorvett, H. (2009). Tegumental glands and terrestrial life in woodlice. Proc. Zool. Soc. Lond. 126, 291–314. doi: 10.1111/j.1096-3642.1956.tb00439.x
Gossiaux, A., Rollin, M., Guérold, F., Felten, V., Laviale, M., Bachelet, Q., et al. (2020). Temperature and nutrient effects on the relative importance of brown and green pathways for stream ecosystem functioning: a mesocosm approach. Freshw. Biol. 65, 1239–1255. doi: 10.1111/fwb.13474
Gotthard, K., and Nylin, S. (1995). Adaptive plasticity and plasticity as an adaptation: a selective review of plasticity in animal morphology and life history. Oikos 74, 3–17. doi: 10.2307/3545669
Govaert, L., Fronhofer, E. A., Lion, S., Eizaguirre, C., Bonte, D., Egas, M., et al. (2019). Eco-evolutionary feedbacks—Theoretical models and perspectives. Funct. Ecol. 33, 13–30. doi: 10.1111/1365-2435.13241
Graça, M. A., Maltby, L., and Calow, P. (1993a). Importance of fungi in the diet of Gammarus pulex and Asellus aquaticus. I. Feeding strategies. Oecologia 93, 139–144. doi: 10.1007/BF00321203
Graça, M. A. S., Maltby, L., and Calow, P. (1993b). Importance of fungi in the diet of Gammarus pulex and Asellus aquaticus. II. Effects on growth, reproduction and physiology. Oecologia 96, 304–309. doi: 10.1007/BF00317498
Graça, M. A. S., Maltby, L., and Calow, P. (1994). Comparative ecology of Gammarus pulex (L.) and Asellus aquaticus (L.) I: population dynamics and microdistribution. Hydrobiologia 281, 155–162. doi: 10.1007/BF00028678
Green, D. M. (2019). Rarity of size-assortative mating in animals: assessing the evidence with anuran amphibians. Am. Nat. 193, 279–295. doi: 10.1086/701124
Green, J. (1974). Parasites and epibionts of Cladocera. Trans. Zool. Soc. Lond. 32, 417–515. doi: 10.1111/j.1096-3642.1974.tb00031.x
Grieve, A., and Lau, D. C. P. (2018). Do autochthonous resources enhance trophic transfer of allochthonous organic matter to aquatic consumers, or vice versa? Ecosphere 9:e02307. doi: 10.1002/ecs2.2307
Groenenboom, M. A. C., and Hogeweg, P. (2002). Space and the persistence of male–killing endosymbionts in insect populations. Proc. R. Soc. Lond. Ser. B Biol. Sci. 269, 2509–2518. doi: 10.1098/rspb.2002.2197
Gross, J. B., Krutzler, A. J., and Carlson, B. M. (2014). Complex craniofacial changes in blind cave-dwelling fish are mediated by genetically symmetric and asymmetric loci. Genetics 196, 1303–1319. doi: 10.1534/genetics.114.161661
Gross, J. B., Sun, D. A., Carlson, B. M., Brodo-Abo, S., and Protas, M. E. (2019). Developmental transcriptomic analysis of the cave-dwelling crustacean, Asellus aquaticus. Genes 11:42. doi: 10.3390/genes11010042
Hairston, N. G. Jr., Ellner, S. P., Geber, M. A., Yoshida, T., and Fox, J. A. (2005). Rapid evolution and the convergence of ecological and evolutionary time. Ecol. Lett. 8, 1114–1127. doi: 10.1111/j.1461-0248.2005.00812.x
Hake, S., and Ross-Ibarra, J. (2015). The natural history of model organisms: genetic, evolutionary and plant breeding insights from the domestication of maize. eLife 4:e05861. doi: 10.7554/eLife.05861
Harari, A. R., Handler, A. M., and Landolt, P. J. (1999). Size-assortative mating, male choice and female choice in the curculionid beetle Diaprepes abbreviatus. Anim. Behav. 58, 1191–1200. doi: 10.1006/anbe.1999.1257
Hargeby, A. (1990a). Effects of pH, humic substances and animal interactions on survival and physiological status of Asellus aquaticus L. and Gammarus pulex (L.). Oecologia 82, 348–354. doi: 10.1007/BF00317482
Hargeby, A. (1990b). Macrophyte associated invertebrates and the effect of habitat permanence. Oikos 57, 338–346. doi: 10.2307/3565963
Hargeby, A., Blindow, I., and Andersson, G. (2007). Long-term patterns of shifts between clear and turbid states in Lake Krankesjön and Lake Tåkern. Ecosystems 10, 29–36. doi: 10.1007/s10021-006-9008-5
Hargeby, A., and Erlandsson, J. (2006). Is size-assortative mating important for rapid pigment differentiation in a freshwater isopod? J. Evol. Biol. 19, 1911–1919. doi: 10.1111/j.1420-9101.2006.01170.x
Hargeby, A., Johansson, J., and Ahnesjö, J. (2004). Habitat-specific pigmentation in a freshwater isopod: adaptive evolution over a small spatiotemporal scale. Evol. Int. J. Org. Evol. 58, 81–94. doi: 10.1111/j.0014-3820.2004.tb01575.x
Hargeby, A., Stoltz, J., and Johansson, J. (2005). Locally differentiated cryptic pigmentation in the freshwater isopod Asellus aquaticus. J. Evol. Biol. 18, 713–721. doi: 10.1111/j.1420-9101.2004.00837.x
Harris, S., Eroukhmanoff, F., Green, K. K., Svensson, E. I., and Pettersson, L. B. (2011). Changes in behavioural trait integration following rapid ecotype divergence in an aquatic isopod. J. Evol. Biol. 24, 1887–1896. doi: 10.1111/j.1420-9101.2011.02322.x
Hart, P. J., and Ison, S. (1991). The influence of prey size and abundance, and individual phenotype on prey choice by the three-spined stickleback, Gasterosteus aculeatm L. J. Fish Biol. 38, 359–372. doi: 10.1111/j.1095-8649.1991.tb03126.x
Hart, P. J. B., and Gill, A. B. (1992). Constraints on prey size selection by the three-spined stickleback: energy requirements and the capacity and fullness of the gut. J. Fish Biol. 40, 205–218. doi: 10.1111/j.1095-8649.1992.tb02567.x
Hasu, T., Holmes, J. C., and Valtonen, E. T. (2007). Isopod (Asellus aquaticus) Size and Acanthocephalan (Acanthocephalan lucil) Infections. J. Parasitol. 93, 450–457. doi: 10.1645/GE-1032R.1
Hasu, T., Jokela, J., and Valtonen, E. T. (2008). Effects of growth factors and water source on laboratory cultures of a northern Asellus aquaticus (Isopoda) population. Aquat. Ecol. 42, 141–150. doi: 10.1007/s10452-007-9089-z
Hendry, A. P. (2017). Eco-Evolutionary Dynamics. Princeton, NJ: Princeton University Press. doi: 10.1515/9781400883080
Hendry, A. P., Bolnick, D. I., Berner, D., and Peichel, C. L. (2009). Along the speciation continuum in sticklebacks. J. Fish Biol. 75, 2000–2036. doi: 10.1111/j.1095-8649.2009.02419.x
Henry, L. P., Bruijning, M., Forsberg, S. K. G., and Ayroles, J. F. (2021). The microbiome extends host evolutionary potential. Nat. Commun. 12:5141. doi: 10.1038/s41467-021-25315-x
Hereford, J. (2009). A quantitative survey of local adaptation and fitness trade-offs. Am. Nat. 173, 579–588. doi: 10.1086/597611
Herrmann, J. (1984). Factors affecting the occurrence of Dendrocoelum lacteum (Turbellaria) in Swedish lakes. Hydrobiologia 114, 3–8. doi: 10.1007/BF00016595
Hervant, F., Mathieu, J., Barré, H., Simon, K., and Pinon, C. (1997). Comparative study on the behavioral, ventilatory, and respiratory responses of hypogean and epigean crustaceans to long-term starvation and subsequent feeding. Comp. Biochem. Physiol. A Physiol. 118, 1277–1283. doi: 10.1016/S0300-9629(97)00047-9
Hervant, F., Mathieu, J., and Culver, D. C. (1999). Comparative responses to severe hypoxia and subsequent recovery in closely related amphipod populations (Gammarus minus) from cave and surface habitats. Hydrobiologia 392, 197–204. doi: 10.1023/A:1003511416509
Hessler, R. R., Wilson, G. D., and Thistle, D. (1979). The deep-sea isopods: a biogeographic and phylogenetic overview. Sarsia N. Atlantic Mar. Sci. 64, 67–75. doi: 10.1080/00364827.1979.10411365
Hewitt, G. (2000). The genetic legacy of the Quaternary ice ages. Nature 405, 907–913. doi: 10.1038/35016000
Hewitt, G. M. (1999). Post-glacial re-colonization of European biota. Biol. J. Linn. Soc. 68, 87–112. doi: 10.1111/j.1095-8312.1999.tb01160.x
Hilgenboecker, K., Hammerstein, P., Schlattmann, P., Telschow, A., and Werren, J. H. (2008). How many species are infected with Wolbachia?–A statistical analysis of current data. FEMS Microbiol. Lett. 281, 215–220. doi: 10.1111/j.1574-6968.2008.01110.x
Hillebrand, H., and Matthiessen, B. (2009). Biodiversity in a complex world: consolidation and progress in functional biodiversity research. Ecol. Lett. 12, 1405–1419. doi: 10.1111/j.1461-0248.2009.01388.x
Hoffmann, A. A., and Hercus, M. J. (2000). Environmental stress as an evolutionary force. Bioscience 50, 217–226. doi: 10.1641/0006-3568(2000)050[0217:ESAAEF]2.3.CO;2
Hoffmann, A. A., and Parsons, P. A. (1997). Extreme Environmental Change and Evolution. Cambridge, MA: Cambridge University Press.
Hooper, D. U., Chapin, F. S. III, Ewel, J. J., Hector, A., Inchausti, P., Lavorel, S., et al. (2005). Effects of biodiversity on ecosystem functioning: a consensus of current knowledge. Ecol. Monogr. 75, 3–35. doi: 10.1890/04-0922
Hornung, E. (2011). Evolutionary adaptation of oniscidean isopods to terrestrial life: structure, physiology and behavior. Terrestrial Arthropod. Rev. 4, 95–130. doi: 10.1163/187498311X576262
Horváthová, T., Antoł, A., Czarnoleski, M., Kozłowski, J., and Bauchinger, U. (2017). An evolutionary solution of terrestrial isopods to cope with low atmospheric oxygen levels. J. Exp. Biol. 220, 1563–1567. doi: 10.1242/jeb.156661
Hunt, S. E., McLaren, W., Gil, L., Thormann, A., Schuilenburg, H., Sheppard, D., et al. (2018). Ensembl variation resources. Database 2018:bay119. doi: 10.1093/database/bay119
Hurst, G. D. D. (2017). Extended genomes: symbiosis and evolution. Interface Focus 7:20170001. doi: 10.1098/rsfs.2017.0001
Hynes, H. B. N., and Williams, W. D. (1965). Experiments on competition between two Asellus species (Isopoda Crustacea). Hydrobiologia 26, 203–210. doi: 10.1007/BF00142268
Isbell, F., Cowles, J., Dee, L. E., Loreau, M., Reich, P. B., Gonzalez, A., et al. (2018). Quantifying effects of biodiversity on ecosystem functioning across times and places. Ecol. Lett. 21, 763–778. doi: 10.1111/ele.12928
Jeffery, W. R. (2009). Regressive evolution in Astyanax cavefish. Annu. Rev. Genet. 43, 25–47. doi: 10.1146/annurev-genet-102108-134216
Jemec, A., Škufca, D., Prevorčnik, S., Fišer, Ž, and Zidar, P. (2017). Comparative study of acetylcholinesterase and glutathione S-transferase activities of closely related cave and surface Asellus aquaticus (Isopoda: Crustacea). PLoS One 12:e0176746. doi: 10.1371/journal.pone.0176746
Jeppesen, E., Sondergaard, M., Sondergaard, M., and Christoffersen, K. (1998). The Structuring Role of Submerged Macrophytes in Lakes. New York, NY: Springer. doi: 10.1007/978-1-4612-0695-8
Jiang, Y., Bolnick, D. I., and Kirkpatrick, M. (2013). Assortative mating in animals. Am. Nat. 181, E125–E138. doi: 10.1086/670160
Joca, L. K., Leray, V. L., Zigler, K. S., and Brusca, R. C. (2015). A new host and reproduction at a small size for the ‘snapper-choking isopod’ cymothoa excisa (Isopoda: Cymothoidae). J. Crustacean Biol. 35, 292–294. doi: 10.1163/1937240X-00002312
Johnson, J. B., and Belk, M. C. (2020). Predators as agents of selection and diversification. Diversity 12:415. doi: 10.3390/d12110415
Jormalainen, V. (1998). Precopulatory mate guarding in crustaceans: male competitive strategy and intersexual conflict. Q. Rev. Biol. 73, 275–304. doi: 10.1086/420306
Jormalainen, V., and Merilaita, S. (1995). Female resistance and duration of mate-guarding in three aquatic peracarids (Crustacea). Behav. Ecol. Sociobiol. 36, 43–48. doi: 10.1007/BF00175727
Jormalainen, V., Tuomi, J., and Yamamura, N. (1994). Intersexual conflict over precopula duration in mate guarding crustacea. Behav. Process. 32, 265–283. doi: 10.1016/0376-6357(94)90047-7
Karlsson, K., Eroukhmanoff, F., and Svensson, E. I. (2010). Phenotypic plasticity in response to the social environment: effects of density and sex ratio on mating behaviour following ecotype divergence. PLoS One 5:e12755. doi: 10.1371/journal.pone.0012755
Kawecki, T. J., and Ebert, D. (2004). Conceptual issues in local adaptation. Ecol. Lett. 7, 1225–1241. doi: 10.1111/j.1461-0248.2004.00684.x
Kenna, D., Fincham, W. N. W., Dunn, A. M., Brown, L. E., and Hassall, C. (2017). Antagonistic effects of biological invasion and environmental warming on detritus processing in freshwater ecosystems. Oecologia 183, 875–886. doi: 10.1007/s00442-016-3796-x
Kennish, R., and Williams, G. A. (1997). Feeding preferences of the herbivorous crab Grapsus albolineatus:the differential influence of algal nutrient content and morphology. Mar. Ecol. Prog. Ser. 147, 87–95. doi: 10.3354/meps147087
Kilpert, F., Held, C., and Podsiadlowski, L. (2012). Multiple rearrangements in mitochondrial genomes of Isopoda and phylogenetic implications. Mol. Phylogenet. Evol. 64, 106–117. doi: 10.1016/j.ympev.2012.03.013
Knutie, S. A. (2018). Relationships among introduced parasites, host defenses, and gut microbiota of Galapagos birds. Ecosphere 9:e02286. doi: 10.1002/ecs2.2286
Kohler, R. E. (1994). Lords of the Fly: Drosophila Genetics and the Experimental Life. Chicago, IL: University of Chicago Press.
Kokko, H., and López-Sepulcre, A. (2007). The ecogenetic link between demography and evolution: can we bridge the gap between theory and data? Ecol. Lett. 10, 773–782. doi: 10.1111/j.1461-0248.2007.01086.x
Konec, M., Delić, T., and Trontelj, P. (2016). DNA barcoding sheds light on hidden subterranean boundary between Adriatic and Danubian drainage basins. Ecohydrology 9, 1304–1312. doi: 10.1002/eco.1727
Konec, M., Prevorčnik, S., Sarbu, S. M., Verovnik, R., and Trontelj, P. (2015). Parallels between two geographically and ecologically disparate cave invasions by the same species, Asellus aquaticus (Isopoda, Crustacea). J. Evol. Biol. 28, 864–875. doi: 10.1111/jeb.12610
Kopp, M., Servedio, M. R., Mendelson, T. C., Safran, R. J., Rodríguez, R. L., Hauber, M. E., et al. (2018). Mechanisms of assortative mating in speciation with gene flow: connecting theory and empirical research. Am. Nat. 191, 1–20. doi: 10.1086/694889
Koskella, B., Hall, L. J., and Metcalf, C. J. E. (2017). The microbiome beyond the horizon of ecological and evolutionary theory. Nat. Ecol. Evol. 1, 1606–1615. doi: 10.1038/s41559-017-0340-2
Kosswig, C., and Kosswig, L. (1940). Die Variabilität bei Asellus aquaticus, unter besonderer Berücksichtigung der Variabilität in isolierten unter- und oberirdischen Populationen. Rev. Facultie Sci. B 5, 1–55.
Kostanjšek, R., Milatovič, M., and Strus, J. (2010). Endogenous origin of endo-β-1,4-glucanase in common woodlouse Porcellio scaber (Crustacea, Isopoda). J. Comp. Physiol. B Biochem. Syst. Environ. Physiol. 180, 1143–1153. doi: 10.1007/s00360-010-0485-7
Kovalenko, K. E., Thomaz, S. M., and Warfe, D. M. (2012). Habitat complexity: approaches and future directions. Hydrobiologia 685, 1–17. doi: 10.1007/s10750-011-0974-z
Kritzberg, E. S., Cole, J. J., Pace, M. L., Granéli, W., and Bade, D. L. (2004). Autochthonous versus allochthonous carbon sources of bacteria: results from whole-lake 13 C addition experiments. Limnol. Oceanogr. 49, 588–596. doi: 10.4319/lo.2004.49.2.0588
Lafuente, E., and Beldade, P. (2019). Genomics of developmental plasticity in animals. Front. Genet. 10:720. doi: 10.3389/fgene.2019.00720
Laland, K., Matthews, B., and Feldman, M. W. (2016). An introduction to niche construction theory. Evol. Ecol. 30, 191–202. doi: 10.1007/s10682-016-9821-z
Lande, R. (1981). Models of speciation by sexual selection on polygenic traits. Proc. Natl. Acad. Sci. U.S.A. 78, 3721–3725. doi: 10.1073/pnas.78.6.3721
Lande, R. (2009). Adaptation to an extraordinary environment by evolution of phenotypic plasticity and genetic assimilation. J. Evol. Biol. 22, 1435–1446. doi: 10.1111/j.1420-9101.2009.01754.x
Lartillot, N., Brinkmann, H., and Philippe, H. (2007). Suppression of long-branch attraction artefacts in the animal phylogeny using a site-heterogeneous model. BMC Evol. Biol. 7:S4. doi: 10.1186/1471-2148-7-S1-S4
Leimar, O., Hammerstein, P., and Van Dooren, T. J. M. (2006). A new perspective on developmental plasticity and the principles of adaptive morph determination. Am. Nat. 167, 367–376. doi: 10.1086/499566
Lenski, R. E., Rose, M. R., Simpson, S. C., and Tadler, S. C. (1991). Long-term experimental evolution in Escherichia coli. I. Adaptation and divergence during 2,000 generations. Am. Nat. 138, 1315–1341. doi: 10.1086/285289
Levine, S. H. (1976). Competitive interactions in ecosystems. Am. Nat. 110, 903–910. doi: 10.1086/283116
Levins, R., and Lewontin, R. (1985). The Dialectical Biologist. Cambridge, MA: Harvard University Press.
Lewontin, R. C. (1983). The organism as the subject and object of evolution. Sci. Riv. Sci. 118, 65–95.
Leys, M., Keller, I., Robinson, C. T., and Räsänen, K. (2017). Cryptic lineages of a common alpine mayfly show strong life-history divergence. Mol. Ecol. 26, 1670–1686. doi: 10.1111/mec.14026
Liancourt, P., Callaway, R. M., and Michalet, R. (2005). Stress tolerance and competitive-response ability determine the outcome of biotic interactions. Ecology 86, 1611–1618. doi: 10.1890/04-1398
Lindeman, R. L. (1991). The trophic-dynamic aspect of ecology. Bull. Math. Biol. 53, 167–191. doi: 10.1016/S0092-8240(05)80045-X
Linnaeus, C. (1758). Systema Naturae Per Regna Tria Naturae, Secundum Classes, Ordines, Genera, Species, Cum Characteribus, Differentiis, Synonymis, Locis. Editio Decima, Reformata. Holmiae: Laurentius Salvius. doi: 10.5962/bhl.title.542
Lins, L. S. F., Ho, S. Y. W., and Lo, N. (2017). An evolutionary timescale for terrestrial isopods and a lack of molecular support for the monophyly of Oniscidea (Crustacea: Isopoda). Organ. Divers. Evol. 17, 813–820. doi: 10.1007/s13127-017-0346-2
Linzen, B. (1974). “The Tryptophan → Ommochrome pathway in insects,” in Advances in Insect Physiology, eds J. E. Treherne, M. J. Berridge, and V. B. Wigglesworth (Cambridge, MA: Academic Press), 117–246. doi: 10.1016/S0065-2806(08)60130-7
Lion, S. (2018). Theoretical approaches in evolutionary ecology: environmental feedback as a unifying perspective. Am. Nat. 191, 21–44. doi: 10.1086/694865
Little, C. J., Fronhofer, E. A., and Altermatt, F. (2019). Dispersal syndromes can impact ecosystem functioning in spatially structured freshwater populations. Biol. Lett. 15:20180865. doi: 10.1098/rsbl.2018.0865
Liu, W., Golovatch, S., Wesener, T., and Tian, M. (2017). Convergent evolution of unique morphological adaptations to a subterranean environment in cave millipedes (Diplopoda). PLoS One 12:e0170717. doi: 10.1371/journal.pone.0170717
Lockwood, A. P. M. (1959). The extra-haemolymph sodium of Asellus aquaticus (L.). J. Exp. Biol. 36, 562–565. doi: 10.1242/jeb.36.3.562
López-García, P., Eme, L., and Moreira, D. (2017). Symbiosis in eukaryotic evolution. J. Theor. Biol. 434, 20–33. doi: 10.1016/j.jtbi.2017.02.031
Loreau, M., Naeem, S., Inchausti, P., Bengtsson, J., Grime, J. P., Hector, A., et al. (2001). Biodiversity and ecosystem functioning: current knowledge and future challenges. Science 294, 804–808. doi: 10.1126/science.1064088
Losos, J. B., Jackman, T. R., Larson, A., Queiroz, K., and Rodriguez-Schettino, L. (1998). Contingency and determinism in replicated adaptive radiations of island lizards. Science 279, 2115–2118. doi: 10.1126/science.279.5359.2115
Lürig, M. D. (2021). Phenopype: a phenotyping pipeline for python. bioRxiv doi: 10.1101/2021.03.17.435781
Lürig, M. D., Best, R. J., and Stachowicz, J. J. (2016). Microhabitat partitioning in seagrass mesograzers is driven by consistent species choices across multiple predator and competitor contexts. Oikos 125, 1324–1333. doi: 10.1111/oik.02932
Lürig, M. D., Best, R. J., Svitok, M., Jokela, J., and Matthews, B. (2019). The role of plasticity in the evolution of cryptic pigmentation in a freshwater isopod. J. Anim. Ecol. 88, 612–623. doi: 10.1111/1365-2656.12950
Lürig, M. D., and Matthews, B. (2021). Dietary-based developmental plasticity affects juvenile survival in an aquatic detritivore. Proc. Biol. Sci. 288:20203136. doi: 10.1098/rspb.2020.3136
Lyndon, A. R., and Kennedy, C. R. (2001). Colonisation and extinction in relation to competition and resource partitioning in acanthocephalans of freshwater fishes of the British Isles. Folia Parasitol. 48, 37–46. doi: 10.14411/fp.2001.007
MacColl, A. D. C. (2011). The ecological causes of evolution. Trends Ecol. Evol. 26, 514–522. doi: 10.1016/j.tree.2011.06.009
MacNeil, C., Dick, J. T. A., Bigsby, E., Elwood, R. W., Montgomery, W. I., Gibbins, C. N., et al. (2002). The validity of the Gammarus:Asellus ratio as an index of organic pollution: abiotic and biotic influences. Water Res. 36, 75–84. doi: 10.1016/S0043-1354(01)00218-4
Maltby, L. (1995). Sensitivity of the crustaceans Gammarus pulex (L.) and Asellus aquaticus (L.) to short-term exposure to hypoxia and unionized ammonia: observations and possible mechanisms. Water Res. 29, 781–787. doi: 10.1016/0043-1354(94)00231-U
Marchetti, E., and Montalenti, G. (1990). La imperfetta fitness del maschio di Asellus aquaticus (L.) nel riconoscimento delle femmine recettive. Rendiconti Lincei. Sci. Fisiche Nat. 1, 327–333.
Marcus, J. H. (1990). Moulting as an indicator of growth in Asellus aquaticus (L., 1758) and A. meridianus Racovitza, 1919 (Isopoda). Crustaceana 58, 136–143. doi: 10.1163/156854090X00048
Marcus, J. H., Sutcliffe, D. W., and Willoughby, L. G. (1978). Feeding and growth of Asellus aquaticus (Isopoda) on food items from the littoral of Windermere, including green leaves of Elodea canadensis. Freshw. Biol. 8, 505–519. doi: 10.1111/j.1365-2427.1978.tb01473.x
Martínez, G., and Defeo, O. (2006). Reproductive biology of the isopod Excirolana braziliensis at the southern edge of its geographical range. Helgoland Mar. Res. 60:273. doi: 10.1007/s10152-006-0042-7
Matthews, B., De Meester, L., and Jones, C. G. (2014). Under niche construction: an operational bridge between ecology, evolution, and ecosystem science. Ecol. Monogr. 84, 245–263. doi: 10.1890/13-0953.1
Matthews, B., Narwani, A., Hausch, S., Nonaka, E., Peter, H., Yamamichi, M., et al. (2011). Toward an integration of evolutionary biology and ecosystem science. Ecol. Lett. 14, 690–701. doi: 10.1111/j.1461-0248.2011.01627.x
May, L. (1989). “Epizoic and parasitic rotifers,” in Proceedings of the Rotifer Symposium V, (Netherlands: Springer), 59–67. doi: 10.1007/978-94-009-0465-1_8
Merilä, J., and Hendry, A. P. (2014). Climate change, adaptation, and phenotypic plasticity: the problem and the evidence. Evol. Appl. 7, 1–14. doi: 10.1111/eva.12137
Migliore, L., Paluzzi, R., and Vitagliano-Tadini, G. (1982). Reproductive activity in Asellus aquaticus L. (Crustacea, Isopoda) from southern Italy. Int. J. Invertebr. Reprod. 4, 359–367. doi: 10.1080/01651269.1982.10553444
Minchella, D. J., and Scott, M. E. (1991). Parasitism: a cryptic determinant of animal community structure. Trends Ecol. Evol. 6, 250–254. doi: 10.1016/0169-5347(91)90071-5
Miner, B. E., De Meester, L., Pfrender, M. E., Lampert, W., and Hairston, N. G. Jr. (2012). Linking genes to communities and ecosystems: Daphnia as an ecogenomic model. Proc. Biol. Sci. R. Soc. 279, 1873–1882. doi: 10.1098/rspb.2011.2404
Misof, B., Liu, S., Meusemann, K., Peters, R. S., Donath, A., Mayer, C., et al. (2014). Phylogenomics resolves the timing and pattern of insect evolution. Science 346, 763–767. doi: 10.1126/science.1257570
Mojaddidi, H., Fernandez, F. E., Erickson, P. A., and Protas, M. E. (2018). Embryonic origin and genetic basis of cave associated phenotypes in the isopod crustacean Asellus aquaticus. Sci. Rep. 8:16589. doi: 10.1038/s41598-018-34405-8
Montalenti, G., and Rocchi, A. (1964). Note cariologiche sul genere Asellus. Boll. Zool. 31, 341–349. doi: 10.1080/11250006409441068
Montalentl, S. (1990). The imperfect fitness of the male Asellus aquaticus (L.) for the identification of the receptive female. Evol. Biol. 333, 327–333. doi: 10.1007/BF03001768
Moritz, C., and Agudo, R. (2013). The future of species under climate change: resilience or decline? Science 341, 504–508. doi: 10.1126/science.1237190
Murphy, P. M., and Learner, M. A. (1982). The life history and production of Asellus aquaticus (Crustacea: Isopoda) in the River Ely, South Wales. Freshw. Biol. 12, 435–444. doi: 10.1111/j.1365-2427.1982.tb00638.x
Needham, A. E. (1942). Micro-anatomical studies on Asellus. J. Cell Sci. 2, 49–72. doi: 10.1242/jcs.s2-84.333.49
Needham, A. E. (1950). Determination of the form of regenerating limbs in Asellus aquaticus. Q. J. Microsc. Sci. 91, 401–418. doi: 10.1242/jcs.s3-91.16.401
Needham, A. E. (1970). The integumental pigments of some isopod crustacea. Comp. Biochem. Physiol. 35, 509–534. doi: 10.1016/0010-406X(70)90970-9
Needham, A. E. (1974). The Significance of Zoochromes. Berlin: Springer Science & Business Media. doi: 10.1007/978-3-642-80766-4
Nosil, P. (2012). Ecological Speciation (Oxford Series in Ecology and Evolution). Oxford: Oxford University Press. doi: 10.1093/acprof:osobl/9780199587100.001.0001
O’Callaghan, I., Harrison, S., Fitzpatrick, D., and Sullivan, T. (2019). The freshwater isopod Asellus aquaticus as a model biomonitor of environmental pollution: a review. Chemosphere 235, 498–509. doi: 10.1016/j.chemosphere.2019.06.217
Odling-Smee, F. J., Laland, K. N., and Feldman, M. W. (1996). Niche construction. Am. Nat. 147, 641–648. doi: 10.1086/285870
Oetinger, D. F., and Nickol, B. B. (1981). Effects of acanthocephalans on pigmentation of freshwater isopods. J. Parasitol. 67, 672–684. doi: 10.2307/3280441
Økland, K. A. (1978). Life history and growth of Asellus aquaticus (L.) in relation to environment in a eutrophic lake in Norway. Hydrobiologia 59, 243–259. doi: 10.1007/BF00036504
O’Leary, N. A., Wright, M. W., Brister, J. R., Ciufo, S., Haddad, D., McVeigh, R., et al. (2016). Reference sequence (RefSeq) database at NCBI: current status, taxonomic expansion, and functional annotation. Nucleic Acids Res. 44, D733–D745. doi: 10.1093/nar/gkv1189
O’Neill, S. L., Hoffmann, A., and Werren, J. (1997). Influential Passengers: Inherited Microorganisms and Arthropod Reproduction. Oxford: Oxford University Press.
Panhuis, T. M., Butlin, R., Zuk, M., and Tregenza, T. (2001). Sexual selection and speciation. Trends Ecol. Evol. 16, 364–371. doi: 10.1016/S0169-5347(01)02160-7
Penczykowski, R. M., Laine, A.-L., and Koskella, B. (2016). Understanding the ecology and evolution of host–parasite interactions across scales. Evol. Appl. 9, 37–52. doi: 10.1111/eva.12294
Persson, L., and Eklöv, P. (1995). Prey refuges affecting interactions between piscivorous perch and juvenile perch and roach. Ecology 76, 70–81. doi: 10.2307/1940632
Pimentel, D. (1961). Animal population regulation by the genetic feed-back mechanism. Am. Nat. 95, 65–79. doi: 10.1086/282160
Pipan, T., and Culver, D. C. (2012). Convergence and divergence in the subterranean realm: a reassessment. Biol. J. Linn. Soc. 107, 1–14. doi: 10.1111/j.1095-8312.2012.01964.x
Post, D. M., and Palkovacs, E. P. (2009). Eco-evolutionary feedbacks in community and ecosystem ecology: interactions between the ecological theatre and the evolutionary play. Philos. Trans. R. Soc. Lond. B Biol. Sci. 364, 1629–1640. doi: 10.1098/rstb.2009.0012
Poulin, R. (1995). Evolutionary influences on body size in free-living and parasitic isopods. Biol. J. Linn. Soc. 54, 231–244. doi: 10.1016/0024-4066(95)90019-5
Prevorcnik, S., Blejec, A., and Sket, B. (2004). Racial differentiation in Asellus aquaticus (L.) (Crustacea: Isopoda: Asellidae). Arch. Hydrobiol. 160, 193–214. doi: 10.1127/0003-9136/2004/0160-0193
Protas, M. E., Trontelj, P., and Patel, N. H. (2011). Genetic basis of eye and pigment loss in the cave crustacean, Asellus aquaticus. Proc. Natl. Acad. Sci. U.S.A. 108, 5702–5707. doi: 10.1073/pnas.1013850108
Ramos, E. M., Hoffman, D., Junkins, H. A., Maglott, D., Phan, L., Sherry, S. T., et al. (2014). Phenotype–Genotype Integrator (PheGenI): synthesizing genome-wide association study (GWAS) data with existing genomic resources. Eur. J. Hum. Genet. 22, 144–147. doi: 10.1038/ejhg.2013.96
Rando, O. J., and Verstrepen, K. J. (2007). Timescales of genetic and epigenetic inheritance. Cell 128, 655–668. doi: 10.1016/j.cell.2007.01.023
Räsänen, K., and Hendry, A. P. (2008). Disentangling interactions between adaptive divergence and gene flow when ecology drives diversification. Ecol. Lett. 11, 624–636. doi: 10.1111/j.1461-0248.2008.01176.x
Rask, M., and Hiisivuori, C. (1985). The predation on Asellus aquaticus (L.) by perch, Perca fluviatilis (L.), in a small forest lake. Hydrobiologia 121, 27–33. doi: 10.1007/BF00035226
Rauch, S., and Morrison, G. M. (1999). Platinum uptake by the freshwater isopod Asellus aquaticus in urban rivers. Sci. Tot. Environ. 235, 261–268. doi: 10.1016/S0048-9697(99)00203-X
Ray, D. L., and Julian, J. R. (1952). Occurrence of cellulase in Limnoria. Nature 169, 32–33. doi: 10.1038/169032a0
Re, C., Fišer, Ž, Perez, J., Tacdol, A., Trontelj, P., and Protas, M. E. (2018). Common genetic basis of eye and pigment loss in two distinct cave populations of the isopod crustacean Asellus aquaticus. Integr. Comp. Biol. 58, 421–430. doi: 10.1093/icb/icy028
Reed, T. E., Waples, R. S., Schindler, D. E., Hard, J. J., and Kinnison, M. T. (2010). Phenotypic plasticity and population viability: the importance of environmental predictability. Proc. Biol. Sci. R. Soc. 277, 3391–3400. doi: 10.1098/rspb.2010.0771
Reznick, D. N., Bassar, R. D., Handelsman, C. A., Ghalambor, C. K., Arendt, J., Coulson, T., et al. (2019). Eco-evolutionary feedbacks predict the time course of rapid life-history evolution. Am. Nat. 194, 671–692. doi: 10.1086/705380
Richardson, B. A., Kitchen, S. G., Pendleton, R. L., Pendleton, B. K., Germino, M. J., Rehfeldt, G. E., et al. (2014). Adaptive responses reveal contemporary and future ecotypes in a desert shrub. Ecol. Appl. 24, 413–427. doi: 10.1890/13-0587.1
Ridley, M., and Thompson, D. J. (1979). Size and mating in Asellus aquaticus (Crustacea: Isopoda). Z. Tierpsychol. 51, 380–397. doi: 10.1111/j.1439-0310.1979.tb00697.x
Ritchie, M. G. (2007). Sexual selection and speciation. Annu. Rev. Ecol. Evol. Syst. 38, 79–102. doi: 10.1146/annurev.ecolsys.38.091206.095733
Robson, C. M. (1979). Purification and properties of the digestive amylase of Asellus aquaticus (L.) (crustacea, isopoda). Comp. Biochem. Physiol. B Comp. Biochem. 62, 501–505. doi: 10.1016/0305-0491(79)90124-X
Rocchi, A., Prantera, G., Lanza, V., and Di Castro, M. (1984). Incipient sex chromosome differentiation in an isopod crustacean species, Asellus aquaticus. Chromosoma 89, 193–196. doi: 10.1007/BF00294998
Roff, D. (1993). Evolution Of Life Histories: Theory and Analysis. Berlin: Springer Science & Business Media.
Rossi, L. (1985). Interactions between invertebrates and microfungi in freshwater ecosystems. Oikos 44, 175–184. doi: 10.2307/3544059
Rossi, L., and Fano, A. E. (1979). Role of fungi in the trophic niche of the congeneric detritivorous Asellus aquaticus and A. coxalis (Isopoda). Oikos 32, 380–385. doi: 10.2307/3544749
Rota-Stabelli, O., Daley, A. C., and Pisani, D. (2013). Molecular timetrees reveal a Cambrian colonization of land and a new scenario for ecdysozoan evolution. Curr. Biol. 23, 392–398. doi: 10.1016/j.cub.2013.01.026
Rudy, J., Rendoš, M., L’uptáčik, P., and Mock, A. (2018). Terrestrial isopods associated with shallow underground of forested scree slopes in the Western Carpathians (Slovakia). ZooKeys 801, 323–335. doi: 10.3897/zookeys.801.24113
Ruxton, G. D., Allen, W. L., Sherratt, T. N., and Speed, M. P. (2019). Avoiding Attack: The Evolutionary Ecology of Crypsis, Aposematism, and Mimicry. Oxford: Oxford University Press. doi: 10.1093/oso/9780199688678.001.0001
Salemaa, H. (1979). The chromosomes of Asellus aquaticus (l.): a technique for isopod karyology. Crustaceana 36, 316–318. doi: 10.1163/156854079X00799
Salvanes, A., and Hart, P. J. B. (1998). Individual variability in state-dependent feeding behaviour in three-spined sticklebacks. Anim. Behav. 55, 1349–1359. doi: 10.1006/anbe.1997.0707
Santschi, F., Gounand, I., Harvey, E., and Altermatt, F. (2018). Leaf litter diversity and structure of microbial decomposer communities modulate litter decomposition in aquatic systems. Funct. Ecol. 32, 522–532. doi: 10.1111/1365-2435.12980
Schädel, M., van Eldijk, T. M., St, H. W., Reu, J. W., and Haug, J. M. T. (2020). Triassic Isopoda – three new species from Central Europe shed light on the early diversity of the group. Bull. Geosci. 95, 145–166. doi: 10.3140/bull.geosci.1773
Schlichting, C. D., and Pigliucci, M. (1998). Phenotypic Evolution: A Reaction Norm Perspective. Sunderland, MA: Sinauer.
Schluter, D., Clifford, E. A., Nemethy, M., and McKinnon, J. S. (2004). Parallel evolution and inheritance of quantitative traits. Am. Nat. 163, 809–822. doi: 10.1086/383621
Schmidt, C. (2008). Phylogeny of the terrestrial Isopoda (Oniscidea): a review. Arthropod. Syst. Phylog. 66, 191–226.
Schmitz, O. J., Wilmers, C. C., Leroux, S. J., Doughty, C. E., Atwood, T. B., Galetti, M., et al. (2018). Animals and the zoogeochemistry of the carbon cycle. Science 362:eaar3213. doi: 10.1126/science.aar3213
Schoener, T. W. (2011). The newest synthesis: understanding the interplay of evolutionary and ecological dynamics. Science 331, 426–429. doi: 10.1126/science.1193954
Schwab, D. B., Casasa, S., and Moczek, A. P. (2018). On the reciprocally causal and constructive nature of developmental plasticity and robustness. Front. Genet. 9:735. doi: 10.3389/fgene.2018.00735
Seehausen, O., and Wagner, C. E. (2014). Speciation in Freshwater Fishes. London: Annual Reviews. doi: 10.1146/annurev-ecolsys-120213-091818
Selden, P. A., Wilson, G. D. F., Simonetto, L., and Dalla Vecchia, F. M. (2016). First fossil asellote (Isopoda: Asellota), from the Upper Triassic (Norian) of the Carnic Prealps (Friuli, Northeastern Italy). J. Crustacean Biol. 36, 68–86. doi: 10.1163/1937240X-00002387
Seppälä, O., Valtonen, E. T., and Benesh, D. P. (2008). Host manipulation by parasites in the world of dead-end predators: adaptation to enhance transmission? Proc. Biol. Sci. R. Soc. 275, 1611–1615. doi: 10.1098/rspb.2008.0152
Sgrò, C. M., Terblanche, J. S., and Hoffmann, A. A. (2016). What can plasticity contribute to insect responses to climate change? Annu. Rev. Entomol. 61, 433–451. doi: 10.1146/annurev-ento-010715-023859
Shen, Y., Kou, Q., Zhong, Z., Li, X., He, L., He, S., et al. (2017). The first complete mitogenome of the South China deep-sea giant isopod Bathynomus sp. (Crustacea: Isopoda: Cirolanidae) allows insights into the early mitogenomic evolution of isopods. Ecol. Evol. 7, 1869–1881. doi: 10.1002/ece3.2737
Shine, R., O’connor, D., Lemaster, M. P., and Mason, R. T. (2001). Pick on someone your own size: ontogenetic shifts in mate choice by male garter snakes result in size-assortative mating. Anim. Behav. 61, 1133–1141. doi: 10.1006/anbe.2001.1712
Simcic, T., Lukancic, S., and Brancelj, A. (2005). Comparative study of electron transport system activity and oxygen consumption of amphipods from caves and surface habitats. Freshw. Biol. 50, 494–501. doi: 10.1111/j.1365-2427.2005.01339.x
Sket, B. (1994). Distribution of Asellus aquaticus (Crustacea: Isopoda: Asellidae) and its hypogean populations at different geographic scales, with a note on Proasellus istrianus. Hydrobiologia 287, 39–47. doi: 10.1007/BF00006895
Skúlason, S., Parsons, K. J., Svanbäck, R., Räsänen, K., Ferguson, M. M., Adams, C. E., et al. (2019). A way forward with eco evo devo: an extended theory of resource polymorphism with postglacial fishes as model systems. Biol. Rev. Camb. Philos. Soc. 94, 1786–1808. doi: 10.1111/brv.12534
Soares, D., and Niemiller, M. L. (2013). Sensory adaptations of fishes to subterranean environments. Bioscience 63, 274–283. doi: 10.1525/bio.2013.63.4.7
Solomon, C. T., Carpenter, S. R., Cole, J. J., and Pace, M. L. (2008). Support of benthic invertebrates by detrital resources and current autochthonous primary production: results from a whole-lake 13C addition. Freshw. Biol. 53, 42–54. doi: 10.1111/j.1365-2427.2007.01866.x
Stahl, B. A., Gross, J. B., Speiser, D. I., Oakley, T. H., Patel, N. H., Gould, D. B., et al. (2015). A transcriptomic analysis of cave, surface, and hybrid isopod crustaceans of the species Asellus aquaticus. PLoS One 10:e0140484. doi: 10.1371/journal.pone.0140484
Stamm, C., Räsänen, K., Burdon, F. J., Altermatt, F., Jokela, J., Joss, A., et al. (2016). “Chapter four - unravelling the impacts of micropollutants in aquatic ecosystems: interdisciplinary studies at the interface of large-scale ecology,” in Advances in Ecological Research, eds A. J. Dumbrell, R. L. Kordas, and G. Woodward (Cambridge, MA: Academic Press), 183–223. doi: 10.1016/bs.aecr.2016.07.002
Steel, C. G. H. (1982). Stages of the intermoult cycle in the terrestrial isopod Oniscus asellus and their relation to biphasic cuticle secretion. Can. J. Zool. 60, 429–437. doi: 10.1139/z82-058
Steel, E. A. (2009). Some observations on the life history of Asellus aquaticus (L.) and Asellus meridianus racovitza (Crustacea: Isopoda). Proc. Zool. Soc. Lond. 137, 71–87. doi: 10.1111/j.1469-7998.1961.tb06162.x
Steffan, A. W. (1967). Ectosymbiosis in Aquatic Insects. Amsterdam: Elsevier, 207–289. doi: 10.1016/B978-1-4832-2758-0.50011-4
Stomp, M., van Dijk, M. A., van Overzee, H. M. J., Wortel, M. T., Sigon, C. A. M., Egas, M., et al. (2008). The timescale of phenotypic plasticity and its impact on competition in fluctuating environments. Am. Nat. 172, 169–185. doi: 10.1086/591680
Stouthamer, R., Breeuwer, J. A., and Hurst, G. D. (1999). Wolbachia pipientis: microbial manipulator of arthropod reproduction. Annu. Rev. Microbiol. 53, 71–102. doi: 10.1146/annurev.micro.53.1.71
Stouthamer, R., Luck, R. F., and Hamilton, W. D. (1990). Antibiotics cause parthenogenetic Trichogramma (Hymenoptera/Trichogrammatidae) to revert to sex. Proc. Natl. Acad. Sci. U.S.A. 87, 2424–2427. doi: 10.1073/pnas.87.7.2424
Sultan, S. E. (2015). Organism and Environment: Ecological Development, Niche Construction, and Adaption. Oxford: Oxford University Press. doi: 10.1093/acprof:oso/9780199587070.001.0001
Sultan, S. E. (2021). “Phenotypic Plasticity as an Intrinsic Property of Organisms,” in Phenotypic Plasticity & Evolution, ed. D. W. Pfennig (Boca Raton, FL: CRC Press), 3–24. doi: 10.1201/9780429343001-2
Sultan, S. E., and Spencer, H. G. (2002). Metapopulation structure favors plasticity over local adaptation. Am. Nat. 160:271. doi: 10.1086/341015
Svensson, E. I. (2018). On reciprocal causation in the evolutionary process. Evol. Biol. 45, 1–14. doi: 10.1007/s11692-017-9431-x
Sworobowicz, L., Grabowski, M., Mamos, T., Burzyński, A., Kilikowska, A., Sell, J., et al. (2015). Revisiting the phylogeography of Asellus aquaticus in Europe: insights into cryptic diversity and spatiotemporal diversification. Freshw. Biol. 60, 1824–1840. doi: 10.1111/fwb.12613
Sworobowicz, L., Mamos, T., Grabowski, M., and Wysocka, A. (2020). Lasting through the ice age: the role of the proglacial refugia in the maintenance of genetic diversity, population growth, and high dispersal rate in a widespread freshwater crustacean. Freshw. Biol. 65, 1028–1046. doi: 10.1111/fwb.13487
Teixeira, L., Ferreira, A., and Ashburner, M. (2008). The bacterial symbiont Wolbachia induces resistance to RNA viral infections in Drosophila melanogaster. PLoS Biol. 6:e2. doi: 10.1371/journal.pbio.1000002
Ter-Poghossian, A. (1909). Beitrage zur Kenntnis der Exkretionsorgane der Isopoden. Leipzig: Universitat Leipzig.
Thiery, A., and Cazaubon, A. (1992). Epizootic algae and Protozoa on fresh water branchiopods (Anostraca, Notostraca and Spinicaudata) in Moroccan temporary ponds. Hydrobiologia 239, 85–91. doi: 10.1007/BF00012574
Thomas, G. W. C., Dohmen, E., Hughes, D. S. T., Murali, S. C., Poelchau, M., Glastad, K., et al. (2020). Gene content evolution in the arthropods. Genome Biol. 21:15. doi: 10.1186/s13059-019-1925-7
Thompson, D. J. (1978). Prey size selection by larvae of the damselfly, Ischnura elegans (Odonata). J. Anim. Ecol. 47, 769–785. doi: 10.2307/3670
Thompson, J. N. (1989). Concepts of coevolution. Trends Ecol. Evol. 4, 179–183. doi: 10.1016/0169-5347(89)90125-0
Thompson, J. N. (1998). Rapid evolution as an ecological process. Trends Ecol. Evol. 13, 329–332. doi: 10.1016/S0169-5347(98)01378-0
Thurmond, J., Goodman, J. L., Strelets, V. B., Attrill, H., Gramates, L. S., Marygold, S. J., et al. (2019). FlyBase 2.0: the next generation. Nucleic Acids Res. 47, D759–D765. doi: 10.1093/nar/gky1003
Travis, J. (2020). Where is natural history in ecological, evolutionary, and behavioral science? Am. Nat. 196, 1–8. doi: 10.1086/708765
Tufto, J. (2000). The evolution of plasticity and nonplastic spatial and temporal adaptations in the presence of imperfect environmental cues. Am. Nat. 156:121. doi: 10.2307/3079213
Tufto, J. (2015). Genetic evolution, plasticity, and bet-hedging as adaptive responses to temporally autocorrelated fluctuating selection: a quantitative genetic model. Evol. Int. J. Org. Evol. 69, 2034–2049. doi: 10.1111/evo.12716
Turk, S., Sket, B., and Sarbu, Ş (1996). Comparison between some epigean and hypogean populations of Asellus aquaticus (Crustacea: Isopoda: Asellidae). Hydrobiologia 337, 161–170. doi: 10.1007/BF00028517
Turk-Prevorčnik, S., and Blejec, A. (1998). Asellus aquaticus infernus, new subspecies (Isopoda: Asellota: Asellidae), from romanian hypogean waters. J. Crustacean Biol. 18, 763–773. doi: 10.2307/1549153
Unwin, E. E., and Stebbing, T. R. R. (1920). Notes upon the reproduction of Asellus aquaticus. Zool. J. Linn. Soc. 34, 335–343. doi: 10.1111/j.1096-3642.1920.tb01795.x
Valentino, F., Del Marco, A., and Rocchi, A. (1983). Nuclear DNA content of Asellus aquaticus and Proasellus coxalis. Mitteilungen Zool. Museum Univ. Kiel 1, 19–23.
van Bergen, E., and Beldade, P. (2019). Seasonal plasticity in anti-predatory strategies: matching of color and color preference for effective crypsis. Evol. Lett. 3, 313–320. doi: 10.1002/evl3.113
Van den Brink, P. J., Klein, S. L., and Rico, A. (2017). Interaction between stress induced by competition, predation, and an insecticide on the response of aquatic invertebrates. Environ. Toxicol. Chem. 36, 2485–2492. doi: 10.1002/etc.3788
Van Ginneken, M., Blust, R., and Bervoets, L. (2017). How lethal concentration changes over time: toxicity of cadmium, copper, and lead to the freshwater isopod Asellus aquaticus. Environ. Toxicol. Chem. 36, 2849–2854. doi: 10.1002/etc.3847
Van Ginneken, M., Blust, R., and Bervoets, L. (2019). The impact of temperature on metal mixture stress: sublethal effects on the freshwater isopod Asellus aquaticus. Environ. Res. 169, 52–61. doi: 10.1016/j.envres.2018.10.025
Verovnik, R., and Konec, M. (2019). “Chapter 11 - Asellus aquaticus: a model system for historical biogeography,” in Encyclopedia of Caves (Third Edition), eds W. B. White, D. C. Culver, and T. Pipan (Cambridge, MA: Academic Press), 76–84. doi: 10.1016/B978-0-12-814124-3.00011-X
Verovnik, R., Prevorčnik, S., and Jugovic, J. (2009). Description of a neotype for Asellus aquaticus Linné, 1758 (Crustacea: Isopoda: Asellidae), with description of a new subterranean Asellus species from Europe. Zool. Anzeiger 248, 101–118. doi: 10.1016/j.jcz.2009.03.001
Verovnik, R., Sket, B., and Trontelj, P. (2004). Phylogeography of subterranean and surface populations of water lice Asellus aquaticus (Crustacea: Isopoda). Mol. Ecol. 13, 1519–1532. doi: 10.1111/j.1365-294X.2004.02171.x
Verovnik, R., Sket, B., and Trontelj, P. (2005). The colonization of Europe by the freshwater crustacean Asellus aquaticus (Crustacea: Isopoda) proceeded from ancient refugia and was directed by habitat connectivity. Mol. Ecol. 14, 4355–4369. doi: 10.1111/j.1365-294X.2005.02745.x
Verspoor, E. (1982). Assortative mating for size in Asellus aquaticus (L.) (Isopoda). Crustaceana 43, 216–219. doi: 10.1163/156854082X00579
Via, S., and Lande, R. (1985). Genotype-environment interaction and the evolution of phenotypic plasticity. Evol. Int. J. Org. Evol. 39, 505–522. doi: 10.1111/j.1558-5646.1985.tb00391.x
Vick, P., and Blum, M. (2010). The isopod Asellus aquaticus: a novel arthropod model organism to study evolution of segment identity and patterning. Palaeodiversity 3, 89–97.
Visser, S., Thébault, E., and Ruiter, P. C. (2012). “Ecosystem engineers, keystone species,” in Encyclopedia of Sustainability Science and Technology, ed. R. A. Meyers (New York, NY: Springer), 3299–3306. doi: 10.1007/978-1-4419-0851-3_569
Vitagliano, E., Marchetti, E., and Maccauro, P. (1994). Correlation among skewed sex-ratio, monogeny and maternal sex determination in Asellus aquaticus. Rendiconti Lincei. Sci. Fisiche Nat. 5, 289–297. doi: 10.1007/BF03001628
Volpi, E. V., Pelliccia, F., Lanza, V., Di Castro, M., and Rocchi, A. (1992). Morphological differentiation of a sex chromosome and ribosomal genes in Asellus aquaticus (Crust. Isop.). Heredity 69, 478–482. doi: 10.1038/hdy.1992.153
von Reumont, B. M., Jenner, R. A., Wills, M. A., Dell’ampio, E., Pass, G., Ebersberger, I., et al. (2012). Pancrustacean phylogeny in the light of new phylogenomic data: support for Remipedia as the possible sister group of Hexapoda. Mol. Biol. Evol. 29, 1031–1045. doi: 10.1093/molbev/msr270
Wahlström, N., Edlund, U., Pavia, H., Toth, G., Jaworski, A., Pell, A. J., et al. (2020). Cellulose from the green macroalgae Ulva lactuca: isolation, characterization, optotracing, and production of cellulose nanofibrils. Cellulose 27, 3707–3725. doi: 10.1007/s10570-020-03029-5
Wallace, J. B., and Webster, J. R. (1996). The role of macroinvertebrates in stream ecosystem function. Annu. Rev. Entomol. 41, 115–139. doi: 10.1146/annurev.en.41.010196.000555
Walsh, J. R., Carpenter, S. R., and Vander Zanden, M. J. (2016). Invasive species triggers a massive loss of ecosystem services through a trophic cascade. Proc. Natl. Acad. Sci. U.S.A. 113, 4081–4085. doi: 10.1073/pnas.1600366113
Wang, Y., Brune, A., and Zimmer, M. (2007). Bacterial symbionts in the hepatopancreas of isopods: diversity and environmental transmission. FEMS Microbiol. Ecol. 61, 141–152. doi: 10.1111/j.1574-6941.2007.00329.x
Warfe, D. M., Barmuta, L. A., and Wotherspoon, S. (2008). Quantifying habitat structure: surface convolution and living space for species in complex environments. Oikos 117, 1764–1773. doi: 10.1111/j.1600-0706.2008.16836.x
Watling, L. (1981). An alternative phylogeny of peracarid crustaceans. J. Crustacean Biol. 1, 201–210. doi: 10.2307/1548159
Webster, J. R., and Benfield, E. F. (1986). Vascular plant breakdown in freshwater ecosystems. Annu. Rev. Ecol. Syst. 17, 567–594. doi: 10.1146/annurev.es.17.110186.003031
Wedell, N., Kvarnemo, C., Lessells, C. M., and Tregenza, T. (2006). Sexual conflict and life histories. Anim. Behav. 71, 999–1011. doi: 10.1016/j.anbehav.2005.06.023
Wege, W. (1909). Über die Insertionsweise der Arthropodenmuskeln nach Beobachtungen an Asellus aquaticus. Zool. Anzeiger Leipzig 35, 124–129.
Weinert, L. A., Araujo-Jnr, E. V., Ahmed, M. Z., and Welch, J. J. (2015). The incidence of bacterial endosymbionts in terrestrial arthropods. Proc. Biol. Sci. 282:20150249. doi: 10.1098/rspb.2015.0249
Weltje, L. O. J. (2006). Effects of endocrine disrupting compounds and temperature on the moulting frequency of the freshwater isopod Asellus aquaticus. (Isopoda: Asellota). Acta Biol. Benrodis 13, 105–115.
Werren, J. H. (1997). Biology of Wolbachia. Annu. Rev. Entomol. 42, 587–609. doi: 10.1146/annurev.ento.42.1.587
Werren, J. H., Baldo, L., and Clark, M. E. (2008). Wolbachia: master manipulators of invertebrate biology. Nat. Rev. Microbiol. 6, 741–751. doi: 10.1038/nrmicro1969
West-Eberhard, M. J. (2003). Developmental Plasticity and Evolution. Oxford: Oxford University Press. doi: 10.1093/oso/9780195122343.001.0001
Wetzer, R. (2002). Mitochondrial genes and isopod phylogeny (Peracarida: Isopoda). J. Crustacean Biol. 22, 1–14. doi: 10.1163/20021975-99990204
Whitehurst, I. T. (1991). The Gammarus: Asellus ratio as an index of organic pollution. Water Res. 25, 333–339. doi: 10.1016/0043-1354(91)90014-H
Whitehurst, I. T., and Lindsey, B. I. (1990). The impact of organic enrichment on the benthic macroinvertebrate communities of a lowland river. Water Res. 24, 625–630. doi: 10.1016/0043-1354(90)90195-C
Williams, W. D. (1962a). Some remarks on phenotypic variation and genetic isolation in Asellus (Isopoda, Asellota). Crustaceana 3, 279–284. doi: 10.1163/156854062X00517
Williams, W. D. (1962b). The geographical distribution of the isopods Asellus aquaticus (L.) and A. meridianus Rac. Proc. Zool. Soc. Lond. 139, 75–96.
Willoughby, L. G., and Marcus, J. H. (1979). Feeding and growth of the isopod Asellus aquaticus on actinomycetes, considered as model filamentous bacteria. Freshw. Biol. 9, 441–449. doi: 10.1111/j.1365-2427.1979.tb01528.x
Wilson, G. D. F. (1986). Evolution of the female cuticular organ in the Asellota (crustacea, isopoda). J. Morphol. 190, 297–305. doi: 10.1002/jmor.1051900306
Wilson, G. D. F. (1991). “Functional morphology and evolution of isopod genitalia,” in Crustacean Sexual Biology, ed. J. W. M. R. T. Bauer (New York, NY: Columbia University Press), 228–245. doi: 10.7312/baue90796-014
Wilson, G. D. F. (2008). Global diversity of Isopod crustaceans (Crustacea; Isopoda) in freshwater. Hydrobiologia 595, 231–240. doi: 10.1007/s10750-007-9019-z
Wilson, G. D. F. (2009). The phylogenetic position of the isopoda in the peracarida (Crustacea: Malacostraca). Arthropod. Syst. Phylog. 67, 159–198.
Wimsatt, W. C., and Wimsatt, W. K. (2007). Re-engineering Philosophy for Limited Beings: Piecewise Approximations to Reality. Cambridge, MA: Harvard University Press. doi: 10.2307/j.ctv1pncnrh
Wolff, C. (2009). The embryonic development of the malacostracan crustacean Porcellio scaber (Isopoda, Oniscidea). Dev. Genes Evol. 219, 545–564. doi: 10.1007/s00427-010-0316-6
Wolff, W. J. (1973). The distribution of Asellus aquaticus (L.) and Proasellus meridianus (Rac.) in the southwestern part of the Netherlands. Hydrobiologia 42, 381–392. doi: 10.1007/BF00047014
Wray, G. A. (2007). The evolutionary significance of cis-regulatory mutations. Nat. Rev. Genet. 8, 206–216. doi: 10.1038/nrg2063
Yoshizawa, M., Yamamoto, Y., O’Quin, K. E., and Jeffery, W. R. (2012). Evolution of an adaptive behavior and its sensory receptors promotes eye regression in blind cavefish. BMC Biol. 10:108. doi: 10.1186/1741-7007-10-108
Yu, J., An, J., Li, Y., and Boyko, C. B. (2018). The first complete mitochondrial genome of a parasitic isopod supports Epicaridea Latreille, 1825 as a suborder and reveals the less conservative genome of isopods. Syst. Parasitol. 95, 465–478. doi: 10.1007/s11230-018-9792-2
Zhang, D., Zou, H., Hua, C.-J., Li, W.-X., Mahboob, S., Al-Ghanim, K. A., et al. (2019). Mitochondrial architecture rearrangements produce asymmetrical nonadaptive mutational pressures that subvert the phylogenetic reconstruction in isopoda. Genome Biol. Evol. 11, 1797–1812. doi: 10.1093/gbe/evz121
Zidar, P., Škufca, D., Prevorčnik, S., Kalčikova, G., and Kokalj, A. J. (2018). Energy reserves in the water louse Asellus aquaticus (Isopoda, Crustacea) from surface and cave populations: seasonal and spatial dynamics. Fundam. Appl. Limnol. 191, 253–265. doi: 10.1127/fal/2018/1118
Zimmer, M., and Bartholmé, S. (2003). Bacterial endosymbionts in Asellus aquaticus (Isopoda) and Gammarus pulex (Amphipoda) and their contribution to digestion. Limnol. Oceanogr. 48, 2208–2213. doi: 10.4319/lo.2003.48.6.2208
Zimmer, M., Danko, J., Pennings, S., Danford, A., Carefoot, T., Ziegler, A., et al. (2002a). Cellulose digestion and phenol oxidation in coastal isopods (Crustacea: Isopoda). Mar. Biol. 140, 1207–1213. doi: 10.1007/s00227-002-0800-2
Zimmer, M., Geisler, S., Walter, S., and Brendelberger, H. (2002b). Fluorescence in Asellus aquaticus (Isopoda: Asellota): a first approach. Evol. Ecol. Res. 4, 181–187.
Zimmer, M., Danko, J. P., Pennings, S. C., Danford, A. R., Ziegler, A., Uglow, R. F., et al. (2001). Hepatopancreatic endosymbionts in coastal isopods (Crustacea: Isopoda), and their contribution to digestion. Mar. Biol. 138, 955–963. doi: 10.1007/s002270000519
Keywords: animal model system, ecosystem function, evolutionary ecology, freshwater ecosystems, integrative biology, organism-environment interactions, microbiome
Citation: Lafuente E, Lürig MD, Rövekamp M, Matthews B, Buser C, Vorburger C and Räsänen K (2021) Building on 150 Years of Knowledge: The Freshwater Isopod Asellus aquaticus as an Integrative Eco-Evolutionary Model System. Front. Ecol. Evol. 9:748212. doi: 10.3389/fevo.2021.748212
Received: 27 July 2021; Accepted: 21 September 2021;
Published: 04 November 2021.
Edited by:
Ivan Gomez-Mestre, Estación Biológica de Doñana (EBD), SpainReviewed by:
Peter Trontelj, University of Ljubljana, SloveniaRebecca Nagel, Bielefeld University, Germany
Copyright © 2021 Lafuente, Lürig, Rövekamp, Matthews, Buser, Vorburger and Räsänen. This is an open-access article distributed under the terms of the Creative Commons Attribution License (CC BY). The use, distribution or reproduction in other forums is permitted, provided the original author(s) and the copyright owner(s) are credited and that the original publication in this journal is cited, in accordance with accepted academic practice. No use, distribution or reproduction is permitted which does not comply with these terms.
*Correspondence: Elvira Lafuente, ZWxhZnVlbnRlbWF6QGdtYWlsLmNvbQ==