- 1Departamento de Biología Molecular e Ingeniería Bioquímica, Universidad Pablo de Olavide, Seville, Spain
- 2Departamento de Biología Vegetal y Ecología, Facultad de Biología, Universidad de Sevilla, Seville, Spain
Flower colour is mainly due to the presence and type of pigments. Pollinator preferences impose selection on flower colour that ultimately acts on flower pigments. Knowing how pollinators perceive flowers with different pigments becomes crucial for a comprehensive understanding of plant-pollinator communication and flower colour evolution. Based on colour space models, we studied whether main groups of pollinators, specifically hymenopterans, dipterans, lepidopterans and birds, differentially perceive flower colours generated by major pigment groups. We obtain reflectance data and conspicuousness to pollinators of flowers containing one of the pigment groups more frequent in flowers: chlorophylls, carotenoids and flavonoids. Flavonoids were subsequently classified in UV-absorbing flavonoids, aurones-chalcones and the anthocyanins cyanidin, pelargonidin, delphinidin, and malvidin derivatives. We found that flower colour loci of chlorophylls, carotenoids, UV-absorbing flavonoids, aurones-chalcones, and anthocyanins occupied different regions of the colour space models of these pollinators. The four groups of anthocyanins produced a unique cluster of colour loci. Interestingly, differences in colour conspicuousness among the pigment groups were almost similar in the bee, fly, butterfly, and bird visual space models. Aurones-chalcones showed the highest chromatic contrast values, carotenoids displayed intermediate values, and chlorophylls, UV-absorbing flavonoids and anthocyanins presented the lowest values. In the visual model of bees, flowers with UV-absorbing flavonoids (i.e., white flowers) generated the highest achromatic contrasts. Ours findings suggest that in spite of the almost omnipresence of floral anthocyanins in angiosperms, carotenoids and aurones-chalcones generates higher colour conspicuousness for main functional groups of pollinators.
Introduction
The colours of flowers, usually those of petals, mainly act as a signal to attract pollinators by making flowers highly conspicuous against the vegetative background. Thus, flower colour largely affects floral advertising to pollinators, which has subsequent implications in plant reproduction (Fenster et al., 2004; Phillips et al., 2020). Although abiotic agents of selection may be involved in driving flower colour evolution, this trait is mostly influenced by selection pressures exerted by biotic agents, specifically antagonistic florivores and most importantly mutualistic pollinators (Strauss and Whittall, 2006; Dalrymple et al., 2020; Sullivan and Koski, 2021). Hence, a deeper insight into how flowers produce their colours and how animals perceive them becomes crucial for a comprehensive understanding of both plant-pollinator interactions and flower colour evolution.
Flower colour is mainly produced through pigmentation, in which chemical compounds, i.e., pigments, can absorb certain wavelengths of light and reflect the remaining (van der Kooi et al., 2019). The vast range of flower colours relies on four major pigment classes: chlorophylls, carotenoids, flavonoids, and betalains (Lee, 2007; Narbona et al., 2021). Each pigment class has a distinctive chemical structure, which ultimately affects the specific wavelengths it absorbs and thereby the colour it generates (Grotewold, 2006; Glover, 2007; Tanaka et al., 2008). Chlorophylls absorb in the blue and red regions of the spectrum, generating green colourations for humans; carotenoids mainly absorb in the blue region, giving rise to yellow-orange colourations; and betalains absorb in either the blue or green regions, generating yellow or pink colourations, respectively (Grotewold, 2006; Narbona et al., 2021). Flavonoids are the most widespread and diverse class of pigments in angiosperms (Iwashina, 2015). Flavonoids include important groups such as aurones and chalcones absorbing in the blue region (hereafter, aurones-chalcones; yellow colouration), and flavonols, flavones and flavanones absorbing in the ultraviolet spectrum (hereafter, UV-absorbing flavonoids; white and pale-yellow colourations) (Harborne, 1984; Tanaka et al., 2008; Narbona et al., 2021). Yet, anthocyanins are the flavonoids that generate the most varied colouration to flowers; they absorb in different parts of the green region of the spectrum and produce shades of blue-pink-orange-red floral colours (Grotewold, 2006). Anthocyanins are classified depending on the hydroxylation level of the basic structures, the anthocyanidins, being the six more common in angiosperms (in this order): cyanidin (pink colourations), delphinidin (blue), pelargonidin (orange-red), peonidin (red), petunidin (purple), and malvidin (blue) (Castañeda-Ovando et al., 2009). Furthermore, each of the major classes of pigments and subcategories contains hundreds or thousands of different compounds that vary in the configuration of the core molecular structure (Davies, 2009), which generally affect the absorption of light and thereby the resulting colour (i.e., hue).
Petals may advertise with simple homogenous colours in some species, through to extremely complex colour patterns in other plant species. Our ability to interpret such complex signals is just starting to emerge (Lunau et al., 2021; Tunes et al., 2021). Even in petals or petal parts with homogeneous colour, one or several types of major pigment classes may be accumulated to generate a unique colour (Kay et al., 1981; Grotewold, 2006; Davies, 2009). For example, it is common to find flowers with different types of anthocyanins or with the presence of anthocyanins and carotenoids coexisting (Glover, 2007; Ng and Smith, 2016; Narbona et al., 2021). Only the accumulations of betalains and anthocyanins are mutually exclusive, being the production of the former restricted to some families of the order Caryophyllales (Moghe and Smith, 2018; Timoneda et al., 2019). Pigments are usually located in the epidermal and mesophyll cell layers of petals, and each single cell may accumulate one or several major pigment classes (Kay et al., 1981). In this way, the production of each major class of pigments within the cell is completely independent from each other due to the biosynthetic pathways are unrelated (Tanaka et al., 2008; Fattorini and Glover, 2020; Li et al., 2020). The evolutionary gain and loss of one or various major flower pigment classes in species of the same linage is common across the angiosperms (Rausher, 2008; Smith and Goldberg, 2015; Ng and Smith, 2016; Landis et al., 2018; Wessinger et al., 2019; Roguz et al., 2020; Berardi et al., 2021). Those shifts are often reversible, suggesting that the functionality of the underlying biochemical pathways is conserved (Ng et al., 2018). Evolutionary changes in major floral pigment groups are ubiquitous, and there are attempts in some lineages to assess how different pigment groups are perceived by their main pollinators (Muchhala et al., 2014; Ng and Smith, 2016; Kellenberger et al., 2019; Wessinger et al., 2019; Ogutcen et al., 2020). However, broad comparative studies directly linking pigment biochemistry with pollinator visual system models need to be done (see An et al., 2018; Stavenga et al., 2021).
The pollinator’s visual system clearly determines how colour is perceived (Garcia et al., 2020; Dyer et al., 2021), differing strikingly among higher-level taxonomical groups but showing greater similarity within more closely related animal groups (Price et al., 2019). In this regards, insect colour vision has been thoroughly studied, namely in the honeybee Apis mellifera, and several butterflies, hawkmoths, flies and beetles, and marked differences have been found among orders and even among families (van der Kooi et al., 2021). Among insect pollinators, Lepidoptera species present receptor’s visual systems particularly diverse with up to 15 different photoreceptors, but not all are equally involved in colour perception (van der Kooi et al., 2021). In contrast, the visual system of main hymenopteran pollinators (bees) is rather constant, and most species have three photoreceptors sensitive to UV, blue and green light (Goldsmith, 1990; Briscoe and Chittka, 2001); however, an additional receptor sensitive to red is found in some few species (Peitsch et al., 1992; van der Kooi et al., 2021). Likewise, main dipteran pollinators (Syrphidae, Muscoidae, and Bombyliidae) have similar visual systems with four types of photoreceptors involved in colour processing, with sensitivity peaks at approximately 330, 340, 460, and 540 nm (Lunau, 2014; An et al., 2018; Hannah et al., 2019). Birds, the main pollinator group aside insects, have four kinds of photoreceptors, but two types of visual systems occur depending on the sensitivity of receptor with peak at lowest wavelengths (UVS or VS types, that is ultraviolet or violet sensitive), the remaining three being sensitive to blue, green, and red wavelengths (Goldsmith, 1990; Vorobyev and Osorio, 1998; Hart and Hunt, 2007).
In order to represent colour stimuli into perceptual spaces of different pollinator groups, different colour vision models have been developed taking into consideration their photoreceptor spectral sensitivities and their mechanisms of neuronal processing. The colour vision system of bees has been extensively studied and several vision models have been proposed (Backhaus, 1991; Chittka, 1992; Vorobyev and Brandt, 1997; Vorobyev and Osorio, 1998). The colour hexagon (Chittka, 1992) is the most widely used vision model for bees and is applicable to a wide range of bee species; it allows measurement of perceived chromatic differences and categorisation of bee colours, but it is unclear if and how bees might form colour categories (Renoult et al., 2017). Our understanding of colour vision system of flies is considerably lesser; yet, Troje (1993) developed a vision model for blowflies and found strong evidence of a categorical colour vision, and blowflies were unable to distinguish stimuli falling within a colour category. However, it has been proved that hoverflies can discriminate colours throughout a continuous variation, although neural mechanisms underlying their colour vision are similar to those proposed by Troje for blowflies; thus, Troje’s model can also be used to measure chromatic differences as perceived by hoverflies (An et al., 2018; Hannah et al., 2019). Generating a colour space model for butterflies is more complex due to the diversity of their visual systems and their high number of photoreceptors (Arikawa, 2017; van der Kooi et al., 2021). Yet, in Papilio xuthus it has been proved that not all eight photoreceptors are involved in colour vision and a tetrachromatic vision systems has been proved (Koshitaka et al., 2008; Arikawa, 2017); from this, a tetrahedral colour space, similar to that of birds (see below), has been proposed to represent colours under Papilio vision and measure the perceived chromatic differences (Ohashi et al., 2015; Kantsa et al., 2017). To represent colour stimuli under bird vision, a tetrahedral colour space is used, this space being a representation of the stimulation of the four photoreceptors involved in avian colour perception (Endler and Mielke, 2005; Stoddard and Prum, 2008; Burd et al., 2014). As the previous ones, this model also allows to measure perceived chromatic differences among stimuli (Endler and Mielke, 2005; Stoddard and Prum, 2008; Camargo et al., 2019). A considerable number of studies show that functional groups of pollinators can perceive differently the same flower colour (e.g., Ohashi et al., 2015; Bergamo et al., 2018; Whitney et al., 2020). In fact, it has been demonstrated that flower colours have evolved in different regions of the world to match the visual capabilities and preferences of local pollinator fauna (Burd et al., 2014; Shrestha et al., 2016; Camargo et al., 2019; Coimbra et al., 2020). Recently, it has been found in species of the Gesneriaceae that the production of certain groups of anthocyanins may generate flower reflectance spectra that are adapted to the specific pollinator groups (Ogutcen et al., 2020). Thus, it is particularly relevant to assess if major pigment groups generate visual signals which conspicuousness differs for the main pollinator groups.
In this study, we analysed how flowers containing different pigments classes are perceived by the main functional groups of pollinators, specifically hymenopterans, dipterans, lepidopterans, and birds. We focused our research on flowers with petals homogenously coloured containing only one group of flower pigments. We used the flower reflectance spectra of 123 species with known pigment composition to address the following questions: How do main groups of pollinators perceive the colours generated by different major pigment classes? Is there any pigment class that is more conspicuous for a particular pollinator group? Theory of pollination syndromes hypothesised that some groups of pollinators such as bees, flies, butterflies or birds show preference for certain flower colours (Faegri and Van der Pijl, 1979). However, further studies showed that these predictions are only met in certain pollinator groups (Reverté et al., 2016). Flowers visited by bees are expected to be blue or violet, which is supported in laboratory and field studies (Giurfa et al., 1995; Dyer et al., 2016; Reverté et al., 2016). In addition, honeybees and bumblebees show inmate flower colour preferences for these two colours (Chittka and Wells, 2004). Therefore, we predict that flowers containing blue-violet pigments, i.e., anthocyanin derivatives (Harborne, 2014), may show higher conspicuousness for hymenopterans than those containing other pigment groups. In contrast, flowers visited by dipterans are mostly yellow and white (Reverté et al., 2016) and hoverflies show innate preferences to these colours (Lunau, 2014; Dunn et al., 2020); thus, we expect that flowers containing yellow carotenoids or aurones-chalcones and those containing white UV-absorbing flavonoids may show higher conspicuousness for dipterans. Lastly, reported colour preferences for butterflies are diverse, varying among species or even between sexes (Yoshida et al., 2015; Arikawa, 2017; Arikawa et al., 2021) and no clear preferences have been reported for birds (Lunau et al., 2011). However, given that both birds and many butterflies have red photoreceptors (Peitsch et al., 1992; van der Kooi et al., 2021), one would expect that pelargonidin-containing flowers would be more conspicuous to both groups of pollinators.
Materials and Methods
Groups of Flower Pigments Considered
We chose the pigment classes or groups more frequent in flowers: chlorophylls, carotenoids, and flavonoids (Figure 1A). We intentionally excluded betalains because the number of species with biochemical information on the presence of these pigments in their flowers is limited (Strack et al., 1981, 2003; Sakuta, 2014; Polturak and Aharoni, 2018), and no reflectance data is available for these species. The details of biochemical information of flowers containing flavonoids are much more comprehensive than those for the chlorophylls and carotenoids (e.g., Harborne et al., 1975; Harborne and Williams, 2000; Andersen and Markham, 2006); thus, for this reason we only considered pigment subcategories in flavonoids. In addition, in most studied species containing carotenoids, flowers frequently show a mix of several subcategories of carotenoids (Ohmiya, 2011, 2013; Yuan et al., 2015; Wang et al., 2018). On the basis of their molecular and absorption characteristics, we chose the following groups of flavonoids: UV-absorbing flavonoids (mostly flavonols and flavones), aurones-chalcones, and anthocyanins (Mabry et al., 1970; Harborne, 1984; Andersen and Markham, 2006). Furthermore, we subsequently divided anthocyanins according to the type of anthocyanidins, namely, cyanidin, pelargonidin, delphinidin, and malvidin derivatives (Figure 1A). We excluded other anthocyanins, such as peonidin or petunidin derivatives, from the study because of the low number of known species containing these pigments (Harborne and Williams, 2000; Andersen and Markham, 2006).
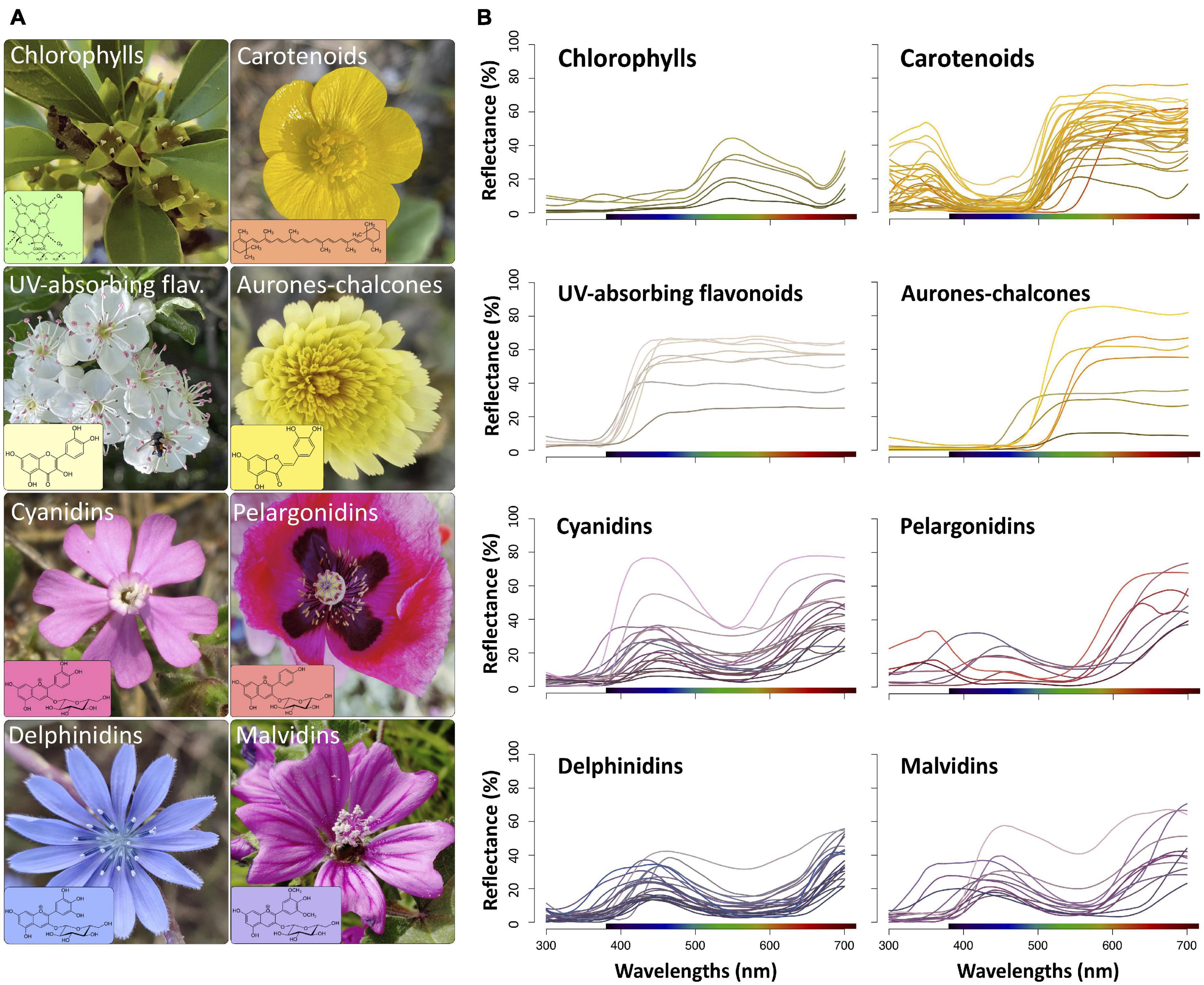
Figure 1. (A) Examples of species with flowers containing one of the main groups of pigments considered in this study and its molecular structures (in the boxes). Rhamnus lycioides (Rhamnaceae), chlorophylls; Ranunculus acris (Ranunculaceae), carotenoids; Crataegus monogyna (Rosaceae), UV-absorbing flavonoids; Andryala integrifolia (Asteraceae), aurones-chalcones; Silene littorea (Caryophyllaceae), cyanidins; P. somniferum (Papaveraceae), pelargonidins; Cichorium intybus (Asteraceae), delphinidins; Malva sylvestris (Malvaceae), malvidins. The colour of the box represents the approximate colours producing in flowers based on human vision model. (B) Reflectance spectra of all species used in this study grouped by types of flower pigments. The colour of each spectral line represents the flower colour based on human vision model.
Flower Pigment Composition
We mainly obtained biochemical data of flowers by literature review (N = 78), and additionally performed our own analyses (N = 45) to complete the sample of some pigment groups that are poorly represented in the bibliography, such as chlorophylls, carotenoids, and UV-absorbing flavonoids (see below). Regarding literature review, we mainly used the series “A survey of anthocyanins” published by G. M. Robison and R. Robison and their collaborator (Lawrence et al., 1938 and references therein) to obtain data of species with floral anthocyanins, although we also used other studies (Supplementary Table 1). For species with flowers containing either carotenoids or aurones-chalcones, we mainly used those listed in Camara et al. (1995) and Boucherle et al. (2017), respectively. In total, we obtained biochemical data of seven species containing chlorophylls, 33 species showing carotenoids and 83 species containing flavonoids (seven with aurones-chalcones, nine with UV-absorbing flavonoids, eight with pelargonidins, 13 with malvidins, 22 with cyanidins, and 24 with delphinidins; Supplementary Table 1). Species belong to 41 families of angiosperms, with a mean of three species per family and a maximum of 15 in Asteraceae and Fabaceae. We discarded species which flowers contain traces or mixtures of more than one group of anthocyanins or even mixtures with other pigments groups (mainly carotenoids).
For analytical identifications of major pigment groups, we performed differential extraction method followed by an analysis of absorbance spectra (Schoefs, 2004; Thrane et al., 2015). Briefly, we used two solvents to extract and separate the mayor pigments classes in each sample: methanol with 1% HCl and pure acetone. Methanol solution is particularly effective to extract flavonoids, whereas acetone mainly extracts carotenoids and chlorophylls (Harborne, 1984; Schoefs, 2004). We placed the same quantity of floral tissue (6–25 mg of fresh weight) in two microtubes containing 1.5 mL of each solvent, which overnighted at 4°C in the dark and kept in the freezer at −80°C until further analysis. We measured absorbance spectra of the samples in each solvent using a Multiskan GO microplate spectrophotometer (Thermo Fisher Scientific Inc., MA, United States) with polypropylene 96-well microplates. We set the scan mode from 280 to 700 nm with 1 nm steps at 22°C constant temperature. We performed identification of mayor pigment classes by means of spectrophotometric analysis. The fact that each pigment group have a particular absorbance spectrum with distinguishable peaks (Mabry et al., 1970; Ritchie, 2006; Narbona et al., 2021) allowed us to identify such pigments over the absorbance spectra of floral extracts (Harborne, 1984; Thrane et al., 2015).
Reflectance Data and Spectral Analysis
For 80 out of the total 123 species used in this study we downloaded floral reflectance data from the Floral Reflectance Database (hereafter “FReD”) (Arnold et al., 2010), whereas for the remaining 43 we obtained them from direct measurements performed from wild flowers (Supplementary Table 1). For direct measurements, we used a Jaz portable spectrometer equipped with a deuterium–tungsten light source (200–800 nm; Ocean Optics, Dunedin, FL, United States), calibrated with a white standard (WS-1-SL, Ocean Optics; see more details in Del Valle et al., 2018). During measurements, light was incident on the adaxial surface of the petals at an angle of 45°. For species with multiple floral colours, we considered only the predominant colour occupying most of the petal area. Similarly, for species with UV or visible petal colour patterns (i.e., bull’s-eyes), we studied the outer part of the petal (see specific methods in Heuschen et al., 2005 and Ortiz et al., 2021).
For the spectral analyses, we considered wavelengths between 300 and 700 nm (Briscoe and Chittka, 2001; Endler and Mielke, 2005). We processed reflectance curves prior to conduct further analysis and plotting. We first used the “procspec” function of the pavo R-package (Maia et al., 2019) to handle negative values, setting the minimum value to zero, but scaling other values accordingly. Then, for noise removal, we used the same function to smooth reflectance curves with a smoothness parameter of 0.20.
To describe the colour produced by each pigment, we calculated hue from its reflectance spectrum (Renoult et al., 2017; van der Kooi et al., 2019). Hue, is usually defined as the wavelength of maximum reflectance, which raises problems when processing reflectance spectra with more than one maximum (Grill and Rush, 2000; Maia et al., 2019), as is common in flowers (Chittka et al., 1994). Thus, we used hue from segment classification analysis (Endler and Mielke, 2005), which is particularly suitable for analysis of all data distributions and for detecting broad trends (Kemp et al., 2015), and we used the modification of Smith (2014) to express hue in degrees. Although more accurate methods exist to calculate hue as specifically perceived by some pollinators like bees (Chittka and Wells, 2004; Dyer et al., 2016) the method used here is suitable when considering a broader pollinator range.
Flower Colour Conspicuousness to Pollinators
To assess colour perception of flowers by hymenopterans, dipterans, lepidopterans and birds, we translated the reflectance spectra to a position in four widely used colour space models. We used the colour hexagon model for the trichromatic vision of bees (Chittka et al., 1992), the categorical space model for the tetravariant visual system of flies (Troje, 1993) and tetrahedral colour space models for the tetrachromatic visual systems of butterflies (Ohashi et al., 2015) and birds (Goldsmith, 1990; Briscoe and Chittka, 2001). We plotted the processed reflectance curves as loci in these colour spaces using the function “colspace” in the pavo R-package (Maia et al., 2019). For bee vision model, we used photoreceptor sensitivities of the honeybee A. mellifera (Chittka et al., 1992), for fly vision model those of housefly Musca domestica (Troje, 1993), for butterfly vision model those of swallowtail P. xuthus (Koshitaka et al., 2008), and for bird vision model we used the average photoreceptor sensitivities of the VS vision system of Trochilidae and Meliphagidae (Ödeen and Håstad, 2010; Burd et al., 2014), the two largest groups of bird pollinators (Krauss et al., 2017). All four models incorporate von Kries adaptation, which assumes that receptors adapt to light environment (Renoult et al., 2017). In all cases, we used the standard daylight function (D65 irradiance function) as illuminant, and the average spectrum of green foliage proposed by Chittka (1992) as background. We calculated the chromatic contrast against the background (i.e., the Euclidean distance between the colour loci of the flower and the achromatic centre; Rohde et al., 2013) according to the photoreceptor spectral sensitivities of the four colour space models. The stronger is flower contrast against the green foliage background, the easier will be the flower detection by pollinators. We also calculated the achromatic contrast for bees (i.e., green contrast) as the difference between the excitation value generated by the stimulus in the green photoreceptor and 0.5, which is the excitation value generated by the background in that receptor (Spaethe et al., 2001). This parameter is important because it is known that bees use this contrast to detect distant flowers under small visual angles (Spaethe et al., 2001). We did not calculate the achromatic contrast for flies, butterflies and birds because no behavioural information was available in this regard (Ohashi et al., 2015).
Statistical Analysis
We used phylogenetic ANOVAs (phylANOVAs) to control the potential influence of phylogeny when analysing differences among flowers containing main groups of pigments in hue, chromatic and achromatic contrasts. To perform phylANOVAs, we used the “phytools” R-package (Revell, 2012) with 10,000 simulations for each test and Holm-adjusted P-values for post hoc comparisons. To construct the phylogenetic tree used in the analyses, we used the “phylo.maker” function implemented in the “V.PhyloMaker” R-package (Jin and Qian, 2019), which uses a mega-tree GBOTB.extended derived from the combination of GBOTB for seed plants (Smith and Brown, 2018) and the phylogeny for pteridophytes published in Zanne et al. (2014) as a backbone. We standardised species names according to The Plant List1 since these are the ones used by V.PhyloMaker. We set the options “nodes = nodes.info.1” (the genus- and family-level node information was extracted from the mega-tree) and “scenarios = S3” (species tips absent from the mega-tree are bound to genus- or family-level following specific rules described in Jin and Qian, 2019). All statistical analyses were conducted in R 4.0.3 (R Core Team, 2021).
Results
Spectral Properties of Flowers Containing Main Groups of Pigments
Chlorophyll-containing flowers were characterised by a low reflection, a peak in the green region and a shoulder in yellow and red regions (Figure 1B). Most flowers with carotenoids displayed a steep slope before the green region of the spectrum (∼560–600 nm) and a secondary peak in the UV region (300–400 nm). Flowers containing either UV-absorbing flavonoids or aurones-chalcones lacked reflectance in the UV wavelengths, but differed in the range they reflect: while UV-absorbing flavonoids reflected all visible light (400–700 nm), aurones-chalcones mostly reflected at wavelengths higher than 500–550 nm. Flowers with the four groups of anthocyanins showed reflectance spectra with a generalised low reflectance in the UV and green regions, but displayed some differences that may affect the resulting flower colour (Figure 1B). Three species with flowers containing pelargonidins (Papaver somniferum, P. rhoeas, and Lysimachia arvensis orange morph) had spectra with low reflection in the blue region producing human orange or red colourations. In most flowers containing delphinidins, reflection was also low in the yellow region, which confers the distinctive blue colourations, but this characteristic is shared with other flowers containing cyanidins, pelargonidins, or malvidins. Flowers containing anthocyanins showed the highest variability in reflectance spectra within each group. On the other hand, the groups of pigments showed significant differences in hue (F = 1484.0, P < 0.001), calculated following the segment classification method. Hue values were structured in three significantly different groups: chlorophylls; carotenoids, UV-absorbing flavonoids and aurones-chalcones; and anthocyanins (Supplementary Figure 1 and Supplementary Table 2).
Flower Pigment Groups as Perceived by Pollinators
In general, floral colour loci of main pigment groups occupied different regions in the bee, fly, butterfly, and bird colour space models (Figure 2; see Supplementary Figures 2–5 for individual representations of each pigment group in each colour space). In the hexagonal colour model for bee vision, colour loci of flowers containing chlorophylls were located in the green region. Most flowers with carotenoids were concentrated in the UV-green zone, whereas aurones-chalcones were mainly located in the green region; only a few flowers containing carotenoids also occupied the green region, indeed these flowers showed spectra with no reflectance in the UV region (Figure 1B). Flowers with UV-absorbing flavonoids mostly occupied the blue-green region of the hexagon, and those with anthocyanins are mainly perceived as blue or UV-blue, with the exception of three species containing orange-red pelargonidins previously described, which are perceived as UV (Figure 2A and Supplementary Figure 2).
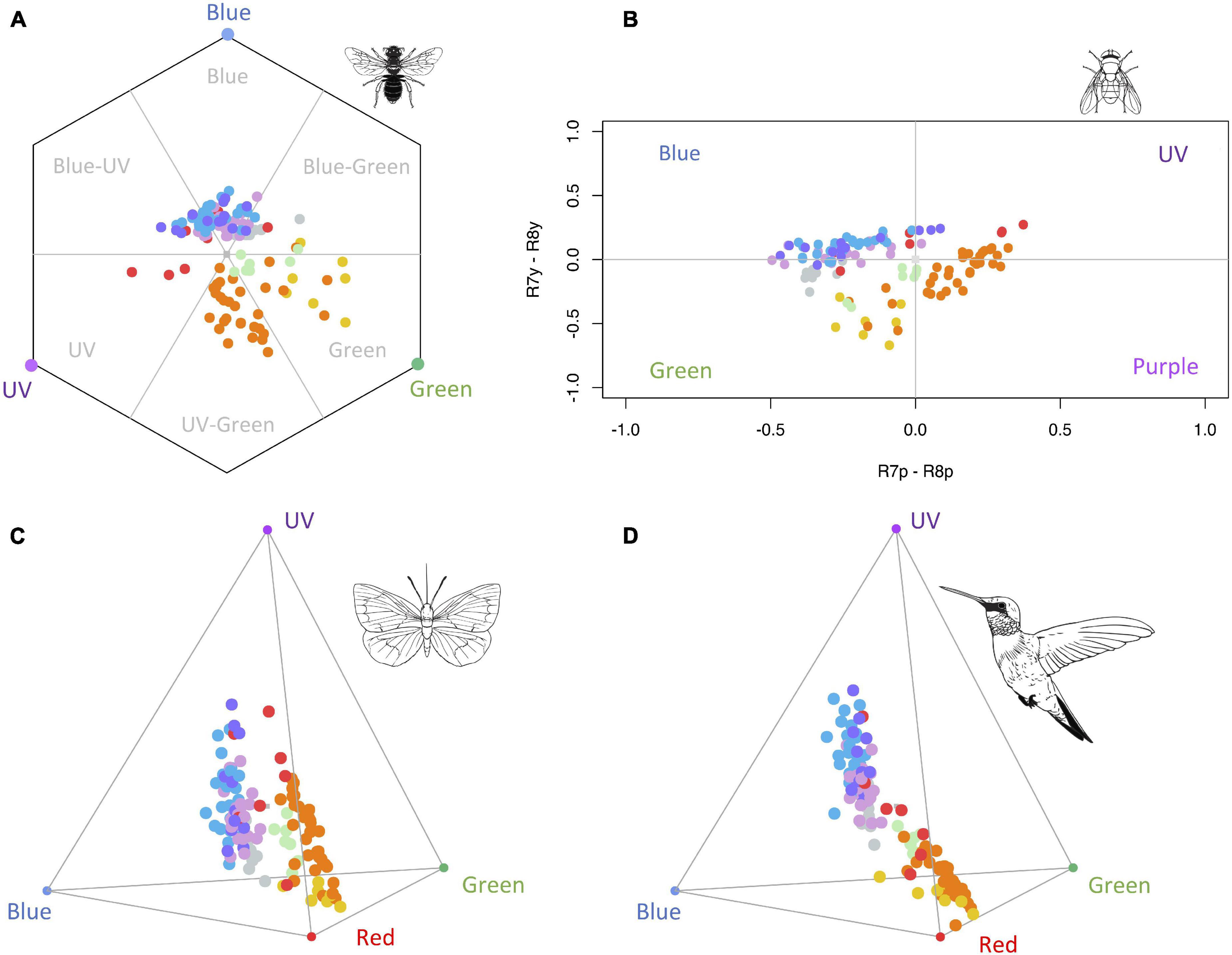
Figure 2. Colour loci of species used in the study according to different colour vision models. The 123 colour loci are represented in the hexagon colour space for the trichromatic vision of bees (A), the categorical colour space for the tetravariant visual system of flies (B), the tetrachromatic colour space model for butterflies (C), and the tetrahedral colour space model for birds with VS vision system (D). Coloured blue, green, purple, and red circles in each vertex represent the maximum signals in the blue, green, UV, and red photoreceptors. Colour loci correspond to each pigment group: green (chlorophylls), orange (carotenoids), grey (UV-absorbing flavonoids), yellow (aurones-chalcones), pink (cyanidins), red (pelargonidins), blue (delphinidins), and purple (malvidins). See Supplementary Figures 2–5 for individual representations of each pigment group in the four colour vision models. Animal silhouettes taken from Divulgare (www.divulgare.net) under a Creative Common licence.
In the fly model, colour loci of pigment groups occupied the four quadrants of the colour space (Figure 2B and Supplementary Figure 3). Flowers containing chlorophylls, UV-absorbing flavonoids, or aurones-chalcones were mostly located in the green category. Carotenoids were widespread across the green, purple, and UV categories of the colour space, being more predominant in the purple region. Anthocyanins predominantly occupied the blue category of the colour space, except for the same three pelargonidin loci previously mentioned, as well as one cyanidin and three malvidin loci, which occupy the UV region.
In the tetrahedron colour space of butterflies, flowers containing aurones-chalcones were mainly located towards the red region, whereas those containing carotenoids showed a more dispersed distribution between the red and UV regions (Figure 2C and Supplementary Figure 4). Anthocyanins were dispersed between the UV and blue regions, apart from some pelargonidins located towards the UV-red edge. UV-absorbing flavonoids occupied positions between blue, green, and red regions of butterfly colour space. Finally, chlorophylls were located between carotenoids and anthocyanins, near the centre of tetrahedron.
In the tetrahedron colour space of birds, flowers containing different groups of pigment showed a similar distribution pattern that in the butterfly colour space (Figure 2D and Supplementary Figure 5). Exceptions occurred with carotenoids that were more clustered towards the red region, and with some pelargonidins appearing near carotenoids.
Colour Conspicuousness of Pigment Groups to Pollinators
We found a significant effect of main pigment groups in the chromatic contrast using the four colour space models (F = 11.72, P < 0.001 for the bee model, F = 8.08, P < 0.001 for the fly model, F = 11.90, P < 0.001 for the butterfly model, and F = 14.31, P < 0.001 for the bird model). In general, flowers containing chlorophylls showed the lowest values of chromatic contrast, and UV-absorbing flavonoids and the four groups of anthocyanins also showed low values of chromatic contrast statistically similar to those of chlorophylls (Figure 3 and Supplementary Table 3). In contrast, flowers containing aurones-chalcones showed the highest chromatic contrast, and carotenoids showed intermediate values. An exception of this general pattern occurred in the fly colour space, where UV-absorbing flavonoids reached intermediate values, similar to those of carotenoids. Moreover, in all visual models, pelargonidins tended to show higher values than the other groups of anthocyanins, especially in bird model and relative to cyanidins, but differences were only marginally significant due to the high variation within pelargonidin.
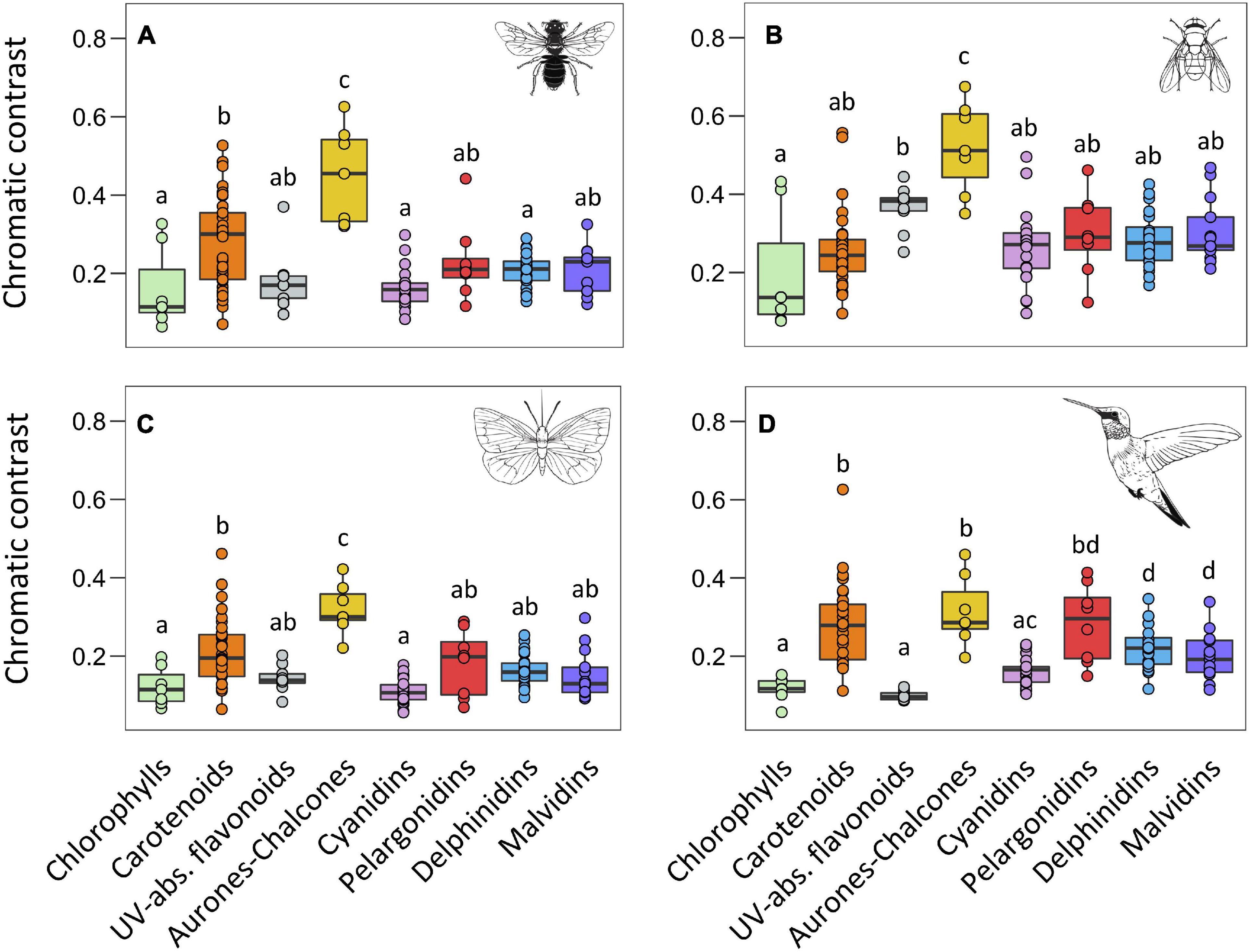
Figure 3. Boxplots representing the distribution of chromatic contrast values for each type of pigment obtained from the vision models of bees (A), flies (B), butterflies (C), and birds (D). The central line displays the median, the bottom and top of the box are the first and third quartiles, and dots represent sample values. We performed phylANOVAs with 10,000 simulations and Holm-adjusted P-value. Different letters represent significant differences at 0.05 level. Animal silhouettes taken from Divulgare (www.divulgare.net) under a Creative Common licence.
With respect the achromatic contrast using the bee colour space model (i.e., green contrast), we found a significant effect of main pigment groups as well (F = 16.59, P < 0.001; Figure 4 and Supplementary Table 4). The four groups of anthocyanins and chlorophylls produced the lowest values while UV-absorbing flavonoids produced the highest ones. Carotenoids and aurones-chalcones showed intermediate values that were statistically similar to those of UV-absorbing flavonoids.
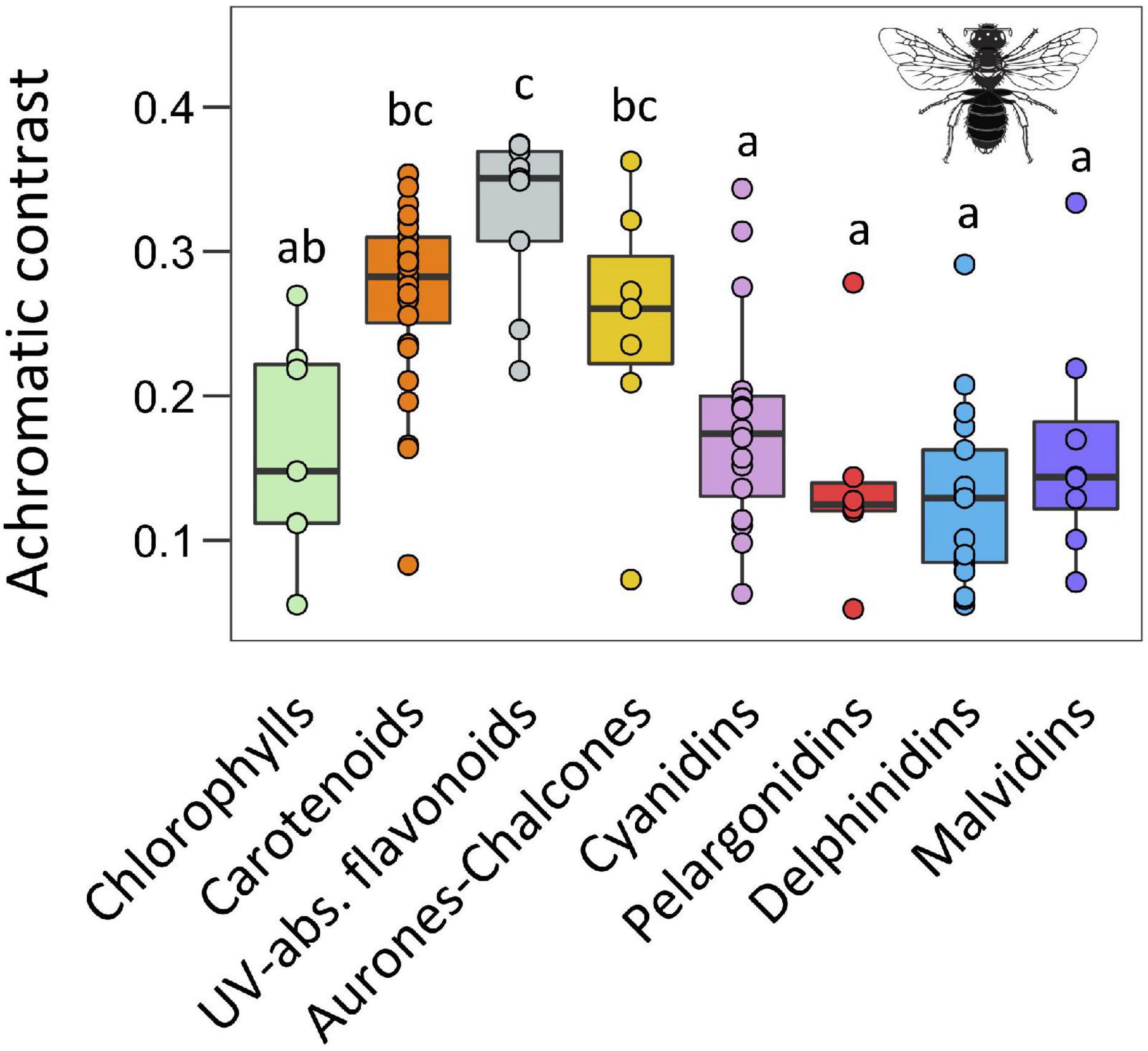
Figure 4. Boxplots representing the distribution of achromatic contrast values for each type of pigment calculated in the hexagonal colour model for bee vision. The central line displays the median, the bottom and top of the box are the first and third quartiles, and dots represent sample values. We performed phylANOVAs with 10,000 simulations and Holm-adjusted P-value. Different letters represent significant differences at 0.05 level. Bee silhouette taken from Divulgare (www.divulgare.net) under a Creative Common licence.
Discussion
The overall goal of this study was to know whether flowers containing different major groups of pigments generate different colour signals for pollinators. Our study demonstrated that flowers containing chlorophylls, carotenoids, UV-absorbing flavonoids, aurones-chalcones and anthocyanins showed distinctive reflectance spectra, which differences and similarities were mostly maintained when the spectral information was translated into the pollinator’s visual system models. In general, flower colour loci of these pigment groups occupied different regions of the bee, fly, butterfly, and bird colour space models. Within the flavonoids, the four groups of anthocyanins produced a unique cluster of colour loci in all the visual colour spaces, UV-absorbing flavonoids were located within or close to anthocyanins, but aurones-chalcones were located in an independent region sometimes shared with some carotenoids samples. The different pigment groups generated differences in colour conspicuousness in all the four visual space models of pollinators. Most of these differences showed high similarity among colour space models, as we found that aurones-chalcones always showed the highest chromatic contrast values, carotenoids displayed intermediate values, and chlorophylls, UV-absorbing flavonoids and anthocyanins presented the lowest values. Yet, some differences among visual models emerged. The implications of these findings for the evolution of flower pigmentation and the ecological consequences for plant-pollinator interaction are discussed.
In general, the clustering patterns of loci observed in the colour space of bees, flies, butterflies and birds were similar. Loci of flowers with chlorophylls occupied central position in all models and showed low chromatic contrast, which suggest that these flowers are difficult to perceive by the four groups of pollinators (Chittka et al., 1994; Endler and Mielke, 2005; Hannah et al., 2019), at least in environments where dominant background is green foliage (Endler, 2012; Bukovac et al., 2017; Martins et al., 2021). This fact may explain the low frequency of green flowers in local floras (Weevers, 1952; Dyer, 1996; Warren and Mackenzie, 2001). However, the distinct colour signal and low chromatic contrast of chlorophylls may help to explain why these pigments are frequently combined with UV-absorbing flavonoids, anthocyanins, or carotenoids to form visible floral guides and patterned flowers (Lunau, 1992; An et al., 2018; Koski, 2020a; Narbona et al., 2021) and thus, to generate highly perceptible colour patterns for pollinators (van den Berg et al., 2020; Roguz et al., 2021).
Flowers with aurones-chalcones generated the highest chromatic contrast in the fly model, thus matching our predictions, but those with carotenoids did not so. In fact, flowers with aurones-chalcones exhibited high contrast in the three colour space models for insects, higher than that of flowers with carotenoids. The higher colour contrast of aurones-chalcones in flowers could lead to a higher attraction for bees, flies and butterflies and, consequently, represent a selective advantage for this group of pigments over carotenoids. Nevertheless, the occurrence of aurones-chalcones in flowers is anecdotic in comparison with carotenoids, being found in certain lineages of Asteraceae, Fabaceae, Plantaginaceae, Oxalidaceae, Gesneriaceae, Rosaceae, and Hamamelidaceae (Bohm, 1988; Iwashina, 2015; Boucherle et al., 2017). The biosynthesis of aurones requires complex enzymatic machinery, particularly the enzyme aurone synthase, with an unusual polyphenol oxidase activity (Molitor et al., 2016; Boucherle et al., 2017), which would explain the rarity of aurones in flowers. It is worth mentioning that in flowers, aurones mostly appear in combination with carotenoids, and to a lesser extend with anthocyanins or UV-absorbing flavonoids, to form floral guides; only a few species are known to contain aurones alone (Valdés, 1970; Iwashina, 2015; Boucherle et al., 2017). Our results support that colour properties of aurones-chalcones are suitable for shaping spatial patterns in flowers (see below).
The conspicuousness of white flowers with UV-absorbing flavonoids was intriguing for two reasons. On the one hand, they showed low chromatic contrast values in all visual models, excepting the fly model where contrast values were intermediate and only lower than those of flowers with aurones-chalcones, which matches our predictions of both groups of flowers generating the highest chromatic contrasts for dipterans. On the other hand, these flowers showed the highest achromatic contrast in the bee visual model, as reported in previous studies with white flowers (Chittka et al., 1994; Lunau et al., 2011; Coimbra et al., 2020). This fact might be relevant because honeybees use such achromatic contrast to find small flowers and flowers at long distance (Giurfa et al., 1997; Spaethe et al., 2001). Besides that, flowers with UV-absorbing flavonoids are defined by reflectance spectra with a steep slope around 400 nm (Chittka et al., 1994; Kevan et al., 2001; Reverté et al., 2016). Insects, and particularly hymenopterans show a maximum of discrimination capacity at 400 nm (Chittka, 1996) and can act as selective agents on UV-absorbing white flowers as has been proposed (Dyer et al., 2012). This suggests selective pressures for accumulating specific types or concentrations of these compounds to generate such UV-absorbing white flowers.
A clear trend is observed for carotenoids, UV-absorbing flavonoids and aurones-chalcones to be separately clustered in all four colour space models, which means that these pigment groups generate contrasting colour signals for all these pollinators and has important consequences in flowers with floral guides. A common pattern in insect-pollinated yellow flowers is an UV-absorbing centre and UV-reflecting periphery (Papiorek et al., 2016; Lunau et al., 2021). This pattern may be produced in two ways: by accumulating aurones-chalcones in the centre and carotenoids in the periphery (e.g., species of the Asteraceae and Plantaginaceae; Harborne and Smith, 1978; Boucherle et al., 2017), or by accumulating carotenoids plus UV-absorbing flavonoids in the centre and only carotenoids in the periphery (e.g., species of the Asteraceae and Fabaceae; Harbone and Grayer, 1994; Bohm and Stuessy, 2001). This intrafloral pattern in yellow flowers could have behavioural consequences for flies and bees. Hoverflies accept UV-reflecting as well as UV-absorbing yellow for landing, but they extend their proboscis preferably to UV-absorbing yellow colours (An et al., 2018). A similar behaviour is found in bees, which made the first antennal contact preferably in UV-absorbing areas (Papiorek et al., 2013). Our results suggest that spatial segregation of aurones-chalcones and carotenoids may produce an UV pattern in yellow flowers that would be highly different in colour category but also in chromatic contrast. It has been found that flowers displaying multiple colours that maximise contrast are more attractive for bees than flowers with homogeneous colours (Heuschen et al., 2005; Leonard et al., 2011; Ma et al., 2016).
Finally, it is worth noting that most flowers containing any of the four groups of anthocyanins here studied clustered close together in all four colour spaces, and in general showed no differentiation among them in terms of chromatic and achromatic contrasts. The case of flowers containing delphinidins or malvidins deserves special mention. They produce human blue colourations, which agree with previous studies (Harborne and Williams, 2000; Yoshida et al., 2009), and also generated loci that mostly occupied the blue category in both bee and fly colour spaces. This colour is the preferred for bees and bumblebees (reviewed in Dyer et al., 2021) but, contrary to our predictions, flowers with delphinidins or malvidins did not generate the highest chromatic contrasts in the bee colour space model. To produce delphinidins or malvidins it is necessary the enzyme flavonoid 3′5′hydroxylase (F3′5′H), which is proposed as the most recent addition to the anthocyanin biosynthetic pathway (Campanella et al., 2014). Our results show that similar blue loci in both bee and fly colour space may result from flowers with cyanidin and pelargonidins through a variety of biochemical or cellular modifications (Harborne and Williams, 2000; Okitsu et al., 2018). Thus, blue colour in flowers can be produced through the three main biosynthetic branches of the anthocyanin pathway (i.e., cyanidin, delphinidin, pelargonidin branches; Grotewold, 2006; Tanaka et al., 2008), which originates an interesting case of phenotypic convergence (Larter et al., 2018). It is also worth noting that the highest chromatic contracts for flowers with pelargonidins were generated in the bird colour space, which matches our predictions. In fact, flowers with pelargonidins were the most contrasting to birds along with those with carotenoids and aurones-chalcones. The role of pelargonidins in bird-pollinated flowers was previously reported in species of the Solanaceae and Gesnerioideae (Ng and Smith, 2016, 2018; Ogutcen et al., 2020). Although birds do not show preferences for a particular colour, they have an excellent colour discrimination (Lunau et al., 2011; Stoddard et al., 2020; Whitney et al., 2020). Our results suggest that both carotenoids and pelargonidins pigments generate a higher conspicuousness to birds than other pigment groups, which would favour their accumulation in flowers of bird-pollinated species. In fact, an important characteristic of this pollination syndrome is the presence of red flowers (Chen et al., 2020), which is typically produced by pelargonidins or by other pigment combinations (Sakuta, 2014; Ng and Smith, 2016; Ogutcen et al., 2020).
In conclusion, we have found that main groups of flower pigments generate distinct flower colours with differential conspicuousness, and each group of pigment showed nearly similar chromatic contrast values in the four visual models of pollinators. As expected, yellow aurones-chalcones showed a high chromatic contrast in the fly visual model, but white UV-absorbing flavonoids showed intermediate contrast and carotenoids showed lower values, similar to anthocyanins. As predicted, pelargonidins generated a higher chromatic contrast in the bird model. Yet, in contrast to our predictions, anthocyanins showed low chromatic and achromatic contrasts in the visual model of bees. Our results suggest that to explain the success of anthocyanins as the most frequent floral pigment in angiosperms (Warren and Mackenzie, 2001; Narbona et al., 2018), other causes than their role in attracting pollinators should be taken into account. Indeed, an increased body of knowledge indicate a protective function of anthocyanin against environmental stressors, which may play an important role in the success and distribution of anthocyanins in flowers (Strauss and Whittall, 2006; Del Valle et al., 2019; Dalrymple et al., 2020). Our results must be taken with caution because the visual systems of the main groups of pollinators are only approximations to their colour vision and further validation with behavioural tests will be needed (Renoult et al., 2017; Lunau and Gerten, 2020; Garcia et al., 2021). Furthermore, our study used specific illumination and background conditions, and colour signals may notably vary in different habitats or environmental conditions (Endler, 2012; Bukovac et al., 2017; Koski, 2020b).
Data Availability Statement
The original contributions presented in the study are included in the article/Supplementary Material, further inquiries can be directed to the corresponding author. The raw data supporting the conclusions of this study are included as Supplementary Table 5.
Author Contributions
EN and JV obtained the data. JV analysed the data and performed the statistical analyses. EN, JV, and PO drafted the manuscript. All authors conceived the study, contributed to the final manuscript, and approved the submitted version.
Funding
This work was supported by Research by the European Regional Development Fund (ERDF) and grants from the Spanish Government (PID2020-116222GB-I00 and CGL2015-63827) and the Andalusian Regional Ministry of Economy, Knowledge, Business and University (PY18-3651, US-1265280, and UPO-1261687).
Conflict of Interest
The authors declare that the research was conducted in the absence of any commercial or financial relationships that could be construed as a potential conflict of interest.
Publisher’s Note
All claims expressed in this article are solely those of the authors and do not necessarily represent those of their affiliated organizations, or those of the publisher, the editors and the reviewers. Any product that may be evaluated in this article, or claim that may be made by its manufacturer, is not guaranteed or endorsed by the publisher.
Supplementary Material
The Supplementary Material for this article can be found online at: https://www.frontiersin.org/articles/10.3389/fevo.2021.743850/full#supplementary-material
Supplementary Figure 1 | Boxplot representing the distribution of hue values for each group of pigments. The central line displays the median, the bottom, and top of the box are the first and third quartiles, and dots represent sample values. We performed phylANOVAs with 10,000 simulations and Holm-adjusted P-value. Different letters represent significant differences at 0.05 level.
Supplementary Figure 2 | Individual representation of each pigment type in the hexagon colour space for the trichromatic vision of bees. Coloured blue, green, and purple circles in each vertex represent the maximum signals in the blue, green, and UV photoreceptors. Colour loci correspond to each pigment group: green (chlorophylls), orange (carotenoids), grey (UV-absorbing flavonoids), yellow (aurones-chalcones), pink (cyanidins), red (pelargonidins), blue (delphinidins), and purple (malvidins).
Supplementary Figure 3 | Individual representation of each pigment type in the categorical colour space for the tetravariant visual system of flies. Colour loci correspond to each pigment group: green (chlorophylls), orange (carotenoids), grey (UV-absorbing flavonoids), yellow (aurones-chalcones), pink (cyanidins), red (pelargonidins), blue (delphinidins), and purple (malvidins).
Supplementary Figure 4 | Individual representation of each pigment type in the tetrahedral colour space for butterflies. Coloured blue, green, purple, and red circles in each vertex represent the maximum signals in the blue, green, UV, and red photoreceptors. Colour loci correspond to each pigment group: green (chlorophylls), orange (carotenoids), grey (UV-absorbing flavonoids), yellow (aurones-chalcones), pink (cyanidins), red (pelargonidins), blue (delphinidins), and purple (malvidins).
Supplementary Figure 5 | Individual representation of each pigment type in the tetrahedral colour space for birds with VS vision system. Coloured blue, green, purple, and red circles in each vertex represent the maximum signals in the blue, green, UV, and red photoreceptors. Colour loci correspond to each pigment group: green (chlorophylls), orange (carotenoids), grey (UV-absorbing flavonoids), yellow (aurones-chalcones), pink (cyanidins), red (pelargonidins), blue (delphinidins), and purple (malvidins).
Footnotes
References
An, L., Neimann, A., Eberling, E., Algora, H., Brings, S., and Lunau, K. (2018). The yellow specialist: Dronefly Eristalis tenax prefers different yellow colours for landing and proboscis extension. J. Exp. Biol. 221:jeb184788. doi: 10.1242/jeb.184788
Andersen, ØM., and Markham, K. R. (2006). Flavonoids: chemistry, biochemistry and applications. Boca Raton, FL: CRC Press. doi: 10.1201/9781420039443
Arikawa, K. (2017). The eyes and vision of butterflies. J. Physiol. 595, 5457–5464. doi: 10.1113/jp273917
Arikawa, K., Nakatani, Y., Koshitaka, H., and Kinoshita, M. (2021). Foraging small white butterflies, Pieris rapae, search flowers using color vision. Front. Ecol. Evol. 9:650069. doi: 10.3389/fevo.2021.650069
Arnold, S. E. J., Faruq, S., Savolainen, V., McOwan, P. W., and Chittka, L. (2010). FReD: The floral reflectance database – A web portal for analyses of flower colour. PLoS ONE 5:e14287. doi: 10.1371/journal.pone.0014287
Backhaus, W. (1991). Color opponent coding in the visual system of the honeybee. Vision Res. 31, 1381–1397. doi: 10.1016/0042-6989(91)90059-E
Berardi, A. E., Esfeld, K., Jäggi, L. V., Mandel, T., Cannarozzi, G. M., and Kuhlemeier, C. (2021). Complex evolution of novel red floral color in Petunia. Plant Cell 33, 2273–2295. doi: 10.1093/plcell/koab114
Bergamo, P. J., Telles, F. J., Arnold, S. E. J., and Brito, V. L. G. (2018). Flower colour within communities shifts from overdispersed to clustered along an alpine altitudinal gradient. Oecologia 188, 223–235. doi: 10.1007/s00442-018-4204-5
Bohm, B. A. (1988). “The minor flavonoids,” in The Flavonoids: Advances in Research since 1980, ed. J. B. Harborne (Dordrecht: Springer Science), 329–388. doi: 10.1007/978-1-4899-2913-6_9
Bohm, B. A., and Stuessy, T. F. (2001). Flavonoids of the sunflower family (Asteraceae). New York, NY: Springer-Verlag Wien.
Boucherle, B., Peuchmaur, M., Boumendjel, A., and Haudecoeur, R. (2017). Occurrences, biosynthesis and properties of aurones as high-end evolutionary products. Phytochemistry 142, 92–111. doi: 10.1016/j.phytochem.2017.06.017
Briscoe, A. D., and Chittka, L. (2001). The evolution of color vision in insects. Annu. Rev. Entomol. 46, 471–510. doi: 10.1146/annurev.ento.46.1.471
Bukovac, Z., Shrestha, M., Garcia, J. E., Burd, M., Dorin, A., and Dyer, A. G. (2017). Why background colour matters to bees and flowers. J. Comp. Physiol. A 203, 369–380. doi: 10.1007/s00359-017-1175-7
Burd, M., Tristan Stayton, C., Shrestha, M., and Dyer, A. G. (2014). Distinctive convergence in Australian floral colours seen through the eyes of Australian birds. Proc. R. Soc. B Biol. Sci. 281:20132862. doi: 10.1098/rspb.2013.2862
Camara, B., Hugueney, P., Bouvier, F., Kuntz, M., and Monéger, R. (1995). Biochemistry and molecular biology of chromoplast development. Int. Rev. Cytol. 163, 175–247. doi: 10.1016/S0074-7696(08)62211-1
Camargo, M. G. G., Lunau, K., Batalha, M. A., Brings, S., Brito, V. L. G., and Morellato, L. P. C. (2019). How flower colour signals allure bees and hummingbirds: a community-level test of the bee avoidance hypothesis. New Phytol. 222, 1112–1122. doi: 10.1111/nph.15594
Campanella, J. J., Smalley, J. V., and Dempsey, M. E. (2014). A phylogenetic examination of the primary anthocyanin production pathway of the Plantae. Bot. Stud. 55, 1–10. doi: 10.1186/1999-3110-55-10
Castañeda-Ovando, A., Pacheco-Hernández, M., de, L., Páez-Hernández, M. E., Rodríguez, J. A., and Galán-Vidal, C. A. (2009). Chemical studies of anthocyanins: a review. Food Chem. 113, 859–871. doi: 10.1016/j.foodchem.2008.09.001
Chen, Z., Niu, Y., Liu, C. Q., and Sun, H. (2020). Red flowers differ in shades between pollination systems and across continents. Ann. Bot. 126, 837–848. doi: 10.1093/AOB/MCAA103
Chittka, L. (1992). The colour hexagon: a chromaticity diagram based on photoreceptor excitations as a generalized representation of colour opponency. J. Comp. Physiol. A 170, 533–543. doi: 10.1007/BF00199331
Chittka, L. (1996). Optimal sets of color receptors and color opponent systems for coding of natural objects in insect vision. J. Theor. Biol. 181, 179–196. doi: 10.1006/jtbi.1996.0124
Chittka, L., and Wells, H. (2004). “Color vision in bees: mechanisms, ecology and evolution,” in How simple nervous systems create complex perceptual worlds, ed. F. Prete (Boston: MIT Press), 165–191.
Chittka, L., Beier, W., Hertel, H., Steinmann, E., and Menzel, R. (1992). Opponent colour coding is a universal strategy to evaluate the photoreceptor inputs in Hymenoptera. J. Comp. Physiol. A 170, 545–563. doi: 10.1007/BF00199332
Chittka, L., Troje, N., and Menzel, R. (1994). Ultraviolet as a component of flower reflections, and the color perception of Hymenoptera. Vision Res. 34, 1489–1508. doi: 10.1016/0042-6989(94)90151-1
Coimbra, G., Araujo, C., Bergamo, P. J., Freitas, L., and Rodríguez-Gironés, M. A. (2020). Flower conspicuousness to bees across pollination systems: A generalized test of the bee-avoidance hypothesis. Front. Plant Sci. 11:558684. doi: 10.3389/fpls.2020.558684
Dalrymple, R. L., Kemp, D. J., Flores-Moreno, H., Laffan, S. W., White, T. E., Hemmings, F. A., et al. (2020). Macroecological patterns in flower colour are shaped by both biotic and abiotic factors. New Phytol. 228, 1972–1985. doi: 10.1111/nph.16737
Davies, K. M. (2009). “Modifying anthocyanin production in flowers,” in Anthocyanins, eds K. S. Gould, K. M. Davies, and C. Winefield (New York, NY: Springer), 49–83. doi: 10.1007/978-0-387-77335-3_3
Del Valle, J. C., Alcalde-Eon, C., Escribano-Bailón, M. T., Buide, M. L., Whittall, J. B., and Narbona, E. (2019). Stability of petal color polymorphism: the significance of anthocyanin accumulation in photosynthetic tissues. BMC Plant Biol. 19:496. doi: 10.1186/s12870-019-2082-6
Del Valle, J. C., Gallardo-López, A., Buide, M. L., Whittall, J. B., and Narbona, E. (2018). Digital photography provides a fast, reliable, and noninvasive method to estimate anthocyanin pigment concentration in reproductive and vegetative plant tissues. Ecol. Evol. 8, 3064–3076. doi: 10.1002/ece3.3804
Dunn, L., Lequerica, M., Reid, C. R., and Latty, T. (2020). Dual ecosystem services of syrphid flies (Diptera: Syrphidae): pollinators and biological control agents. Pest Manag. Sci. 76, 1973–1979. doi: 10.1002/ps.5807
Dyer, A. G. (1996). Reflection of near-ultraviolet radiation from flowers of Australian native plants. Aust. J. Bot. 44, 473–488. doi: 10.1071/BT9960473
Dyer, A. G., Boyd-Gerny, S., Mcloughlin, S., Rosa, M. G. P., Simonov, V., and Wong, B. B. M. (2012). Parallel evolution of angiosperm colour signals: Common evolutionary pressures linked to hymenopteran vision. Proc. R. Soc. B Biol. Sci. 279, 3606–3615. doi: 10.1098/rspb.2012.0827
Dyer, A. G., Boyd-Gerny, S., Shrestha, M., Lunau, K., Garcia, J. E., Koethe, S., et al. (2016). Innate colour preferences of the Australian native stingless bee Tetragonula carbonaria Sm. J. Comp. Physiol. A 202, 603–613. doi: 10.1007/s00359-016-1101-4
Dyer, A. G., Jentsch, A., Burd, M., Garcia, J. E., Giejsztowt, J., Camargo, M. G. G., et al. (2021). Fragmentary blue: Resolving the rarity paradox in flower colors. Front. Plant Sci. 11:618203. doi: 10.3389/fpls.2020.618203
Endler, J. A. (2012). A framework for analysing colour pattern geometry: adjacent colours. Biol. J. Linn. Soc. 107, 233–253. doi: 10.1111/j.1095-8312.2012.01937.x
Endler, J. A., and Mielke, P. W. (2005). Comparing entire colour patterns as birds see them. Biol. J. Linn. Soc. 86, 405–431. doi: 10.1111/j.1095-8312.2005.00540.x
Faegri, K., and Van der Pijl, L. (1979). The principles of pollination ecology. Oxford: Pergamon Press.
Fattorini, R., and Glover, B. J. (2020). Molecular mechanisms of pollination biology. Annu. Rev. Plant Biol. 71, 487–515. doi: 10.1146/annurev-arplant-081519-040003
Fenster, C. B., Armbruster, W. S., Wilson, P., Dudash, M. R., and Thomson, J. D. (2004). Pollination syndromes and floral specialization. Annu. Rev. Ecol. Evol. Syst. 35, 375–403. doi: 10.1146/annurev.ecolsys.34.011802.132347
Garcia, J. E., Phillips, R. D., Peter, C. I., and Dyer, A. G. (2020). Changing how biologists view flowers—color as a perception not a trait. Front. Plant Sci. 11:601700. doi: 10.3389/fpls.2020.601700
Garcia, J. E., Rohr, D. H., and Dyer, A. G. (2021). Colour discrimination from perceived differences by birds. Front. Ecol. Evol. 9:639513. doi: 10.3389/fevo.2021.639513
Giurfa, M., Nunez, J., Chittka, L., and Menzel, R. (1995). Colour preferences of flower-naive honeybees. J. Comp. Physiol. A 177, 247–259.
Giurfa, M., Vorobyev, M., Brandt, R., Posner, B., and Menzel, R. (1997). Discrimination of coloured stimuli by honeybees: Alternative use of achromatic and chromatic signals. J. Comp. Physiol. A 180, 235–243. doi: 10.1007/s003590050044
Glover, B. (2007). Understanding flowers and flowering: an integrated approach. New York, NY: Oxford University Press.
Goldsmith, T. H. (1990). Optimization, constraint, and history in the evolution of eyes. Q. Rev. Biol. 65, 281–322. doi: 10.1086/416840
Grill, C. P., and Rush, V. N. (2000). Analysing spectral data: Comparison and application of two techniques. Biol. J. Linn. Soc. 69, 121–138. doi: 10.1006/bijl.1999.0360
Grotewold, E. (2006). The genetics and biochemistry of floral pigments. Annu. Rev. Plant Biol. 57, 761–780. doi: 10.1146/annurev.arplant.57.032905.105248
Hannah, L., Dyer, A. G., Garcia, J. E., Dorin, A., and Burd, M. (2019). Psychophysics of the hoverfly: Categorical or continuous color discrimination? Curr. Zool. 65, 483–492. doi: 10.1093/cz/zoz008
Harbone, J. B., and Grayer, R. J. (1994). “Flavonoids and insects,” in The flavonoids. Advances in research since 1986, ed. J. B. Harborne (London, UK: Chapman & Hall), 589–618. doi: 10.1007/978-1-4899-2911-2_14
Harborne, J. B. (1984). Phytochemical Methods. A guide to modern techniques of plant analysis. London, UK: Chapman & Hall.
Harborne, J. B., and Smith, D. M. (1978). Anthochlors and other flavonoids as honey guides in the Compositae. Biochem. Syst. Ecol. 6, 287–291. doi: 10.1016/0305-1978(78)90047-9
Harborne, J. B., and Williams, C. A. (2000). Advances in flavonoid research since 1992. Phytochemistry 55, 481–504. doi: 10.1016/S0031-9422(00)00235-1
Harborne, J. B., Marby, H., and Marby, T. J. (1975). The flavonoids. Advances in research. London, UK: Chapman & Hall.
Hart, N. S., and Hunt, D. M. (2007). Avian visual pigments: Characteristics, spectral tuning, and evolution. Am. Nat. 169, S7–S26. doi: 10.1086/510141
Heuschen, B., Gumbert, A., and Lunau, K. (2005). A generalised mimicry system involving angiosperm flower colour, pollen and bumblebees’ innate colour preferences. Plant Syst. Evol. 252, 121–137. doi: 10.1007/s00606-004-0249-5
Iwashina, T. (2015). Contribution to flower colors of flavonoids including anthocyanins: A review. Nat. Prod. Commun. 10, 529–544. doi: 10.1177/1934578x1501000335
Jin, Y., and Qian, H. (2019). V.PhyloMaker: an R package that can generate very large phylogenies for vascular plants. Ecography (Cop.). 42, 1353–1359. doi: 10.1111/ecog.04434
Kantsa, A., Raguso, R. A., Dyer, A. G., Sgardelis, S. P., Olesen, J. M., and Petanidou, T. (2017). Community-wide integration of floral colour and scent in a Mediterranean scrubland. Nat. Ecol. Evol. 1, 1502–1510. doi: 10.1038/s41559-017-0298-0
Kay, Q. O. N., Daoud, H. S., and Stirton, C. H. (1981). Pigment distribution, light reflection and cell structure in petals. Bot. J. Linn. Soc. 83, 57–83. doi: 10.1111/j.1095-8339.1981.tb00129.x
Kellenberger, R. T., Byers, K. J., Francisco, R. M. D. B., Staedler, Y. M., LaFountain, A. M., Schönenberger, J., et al. (2019). Emergence of a floral colour polymorphism by pollinator-mediated overdominance. Nat. Commun. 10:63.
Kemp, D. J., Herberstein, M. E., Fleishman, L. J., Endler, J. A., Bennett, A. T. D., Dyer, A. G., et al. (2015). An integrative framework for the appraisal of coloration in nature. Am. Nat. 185, 705–724. doi: 10.1086/681021
Kevan, P. G., Chittka, L., and Dyer, A. G. (2001). Limits to the salience of ultraviolet: Lessons from colour vision in bees and birds. J. Exp. Biol. 204, 2571–2580. doi: 10.1242/jeb.204.14.2571
Koshitaka, H., Kinoshita, M., Vorobyev, M., and Arikawa, K. (2008). Tetrachromacy in a butterfly that has eight varieties of spectral receptors. Proc. R. Soc. B 275, 947–954. doi: 10.1098/rspb.2007.1614
Koski, M. H. (2020a). Macroevolution of flower color patterning: biased transition rates and correlated evolution with flower size. Front. Plant Sci. 11:945. doi: 10.3389/fpls.2020.00945
Koski, M. H. (2020b). The role of sensory drive in floral evolution. New Phytol. 227, 1012–1024. doi: 10.1111/nph.16510
Krauss, S. L., Phillips, R. D., Karron, J. D., Johnson, S. D., Roberts, D. G., and Hopper, S. D. (2017). Novel consequences of bird pollination for plant mating. Trends Plant Sci. 22, 395–410. doi: 10.1016/j.tplants.2017.03.005
Landis, J. B., Bell, C. D., Hernandez, M., Zenil-Ferguson, R., McCarthy, E. W., Soltis, D. E., et al. (2018). Evolution of floral traits and impact of reproductive mode on diversification in the Phlox family (Polemoniaceae). Mol. Phylogenet. Evol. 127, 878–890. doi: 10.1016/j.ympev.2018.06.035
Larter, M., Dunbar-Wallis, A., Berardi, A. E., and Smith, S. D. (2018). Convergent evolution at the pathway level: Predictable regulatory changes during flower color transitions. Mol. Biol. Evol. 35, 2159–2169. doi: 10.1093/molbev/msy117
Lawrence, W. J. C., Price, J. R., Robinson, G. M., and Robinson, R. (1938). A survey of anthocyanins. V. Biochem. J. 32, 1661–1667. doi: 10.1042/bj0321661
Leonard, A. S., Dornhaus, A., and Papaj, D. R. (2011). Flowers help bees cope with uncertainty: signal detection and the function of floral complexity. J. Exp. Biol. 214, 113–121. doi: 10.1242/jeb.047407
Li, B. J., Zheng, B. Q., Wang, J. Y., Tsai, W. C., Lu, H. C., Zou, L. H., et al. (2020). New insight into the molecular mechanism of colour differentiation among floral segments in orchids. Commun. Biol. 3:89. doi: 10.1038/s42003-020-0821-8
Lunau, K. (1992). A new interpretation of flower guide colouration: Absorption of ultraviolet light enhances colour saturation. Plant Syst. Evol. 183, 51–65. doi: 10.1007/BF00937735
Lunau, K. (2014). Visual ecology of flies with particular reference to colour vision and colour preferences. J. Comp. Physiol. A 200, 497–512. doi: 10.1007/s00359-014-0895-1
Lunau, K., and Gerten, S. (2020). Selective attraction in bird-pollinated flowers. A commentary on:‘Red flowers differ in shades between pollination systems and across continents’. Ann. Bot. 126, iv–v. doi: 10.1093/aob/mcaa144
Lunau, K., Papiorek, S., Eltz, T., and Sazima, M. (2011). Avoidance of achromatic colours by bees provides a private niche for hummingbirds. J. Exp. Biol. 214, 1607–1612. doi: 10.1242/jeb.052688
Lunau, K., Scaccabarozzi, D., Willing, L., and Dixon, K. (2021). A bee’s eye view of remarkable floral colour patterns in the south-west Australian biodiversity hotspot revealed by false colour photography. Ann. Bot. 2021:mcab088. doi: 10.1093/aob/mcab088
Ma, X., Shi, J., Bänziger, H., Sun, Y., Guo, Y., Liu, Z., et al. (2016). The functional significance of complex floral colour pattern in a food-deceptive orchid. Funct. Ecol. 30, 721–732. doi: 10.1111/1365-2435.12571
Mabry, T. J., Markham, K. R., and Thomas, M. B. (1970). The systematic identification of flavonoids. New York, NY: Springer.
Maia, R., Gruson, H., Endler, J. A., and White, T. E. (2019). pavo 2: New tools for the spectral and spatial analysis of colour in R. Methods Ecol. Evol. 10, 1097–1107. doi: 10.1111/2041-210X.13174
Martins, A. E., Camargo, M. G. G., and Morellato, L. P. C. (2021). Flowering phenology and the influence of seasonality in flower conspicuousness for bees. Front. Plant Sci. 11:594538. doi: 10.3389/fpls.2020.594538
Moghe, G. D., and Smith, S. D. (2018). The push and pull of plant specialized metabolism underlies a long-standing, colorful mystery. New Phytol. 217, 471–473. doi: 10.1111/nph.14914
Molitor, C., Mauracher, S. G., and Rompel, A. (2016). Aurone synthase is a catechol oxidase with hydroxylase activity and provides insights into the mechanism of plant polyphenol oxidases. Proc. Nat. Acad. Sci. 113, E1806–E1815.
Muchhala, N., Johnsen, S., and Smith, S. D. (2014). Competition for hummingbird pollination shapes flower color variation in Andean Solanaceae. Evolution, 68, 2275–2286.
Narbona, E., Del Valle, J. C., and Whittall, J. B. (2021). Painting the green canvas: how pigments produce flower colours. Biochemistry 43, 6–12. doi: 10.1042/bio_2021_137
Narbona, E., Wang, H., Ortiz, P. L., Arista, M., and Imbert, E. (2018). Flower colour polymorphism in the Mediterranean Basin: occurrence, maintenance and implications for speciation. Plant Biol. 20, 8–20. doi: 10.1111/plb.12575
Ng, J., and Smith, S. D. (2016). How to make a red flower: the combinatorial effect of pigments. AoB Plants 8:lw013. doi: 10.1093/aobpla/plw013
Ng, J., and Smith, S. D. (2018). Why are red flowers so rare? Testing the macroevolutionary causes of tippiness. J. Evol. Biol. 31, 1863–1875. doi: 10.1111/jeb.13381
Ng, J., Freitas, L. B., and Smith, S. D. (2018). Stepwise evolution of floral pigmentation predicted by biochemical pathway structure. Evolution (N. Y). 72, 2792–2802. doi: 10.1111/evo.13589
Ödeen, A., and Håstad, O. (2010). Pollinating birds differ in spectral sensitivity. J. Comp. Physiol. A 196, 91–96. doi: 10.1007/s00359-009-0474-z
Ogutcen, E., Durand, K., Wolowski, M., Clavijo, L., Graham, C., Glauser, G., et al. (2020). Chemical basis of floral color signals in gesneriaceae: The effect of alternative anthocyanin pathways. Front. Plant Sci. 11:604389. doi: 10.3389/fpls.2020.604389
Ohashi, K., Makino, T. T., and Arikawa, K. (2015). Floral colour change in the eyes of pollinators: Testing possible constraints and correlated evolution. Funct. Ecol. 29, 1144–1155. doi: 10.1111/1365-2435.12420
Ohmiya, A. (2011). Diversity of carotenoid composition in flower petals. Japan Agric. Res. Q. 45, 163–171. doi: 10.6090/jarq.45.163
Ohmiya, A. (2013). Qualitative and quantitative control of carotenoid accumulation in flower petals. Sci. Hortic. (Amsterdam). 163, 10–19. doi: 10.1016/j.scienta.2013.06.018
Okitsu, N., Noda, N., Chandler, S., and Tanaka, Y. (2018). “Flower color and its engineering by genetic modification,” in Ornamental Crops, ed. J. Van Huylenbroeck (Cham: Springer), 29–62. doi: 10.1007/978-3-319-90698-0_3
Ortiz, P. L., Fernández-Díaz, P., Pareja, D., Escudero, M., and Arista, M. (2021). Do visual traits honestly signal floral rewards at community level? Funct. Ecol. 35, 369–383. doi: 10.1111/1365-2435.13709
Papiorek, S., Junker, R. R., Alves-dos-Santos, I., Melo, G. A. R., Amaral-Neto, L. P., Sazima, M., et al. (2016). Bees, birds and yellow flowers: Pollinator-dependent convergent evolution of UV patterns. Plant Biol. 18, 46–55. doi: 10.1111/plb.12322
Papiorek, S., Rohde, K., and Lunau, K. (2013). Bees’ subtle colour preferences: How bees respond to small changes in pigment concentration. Naturwissenschaften 100, 633–643. doi: 10.1007/s00114-013-1060-3
Peitsch, D., Fietz, A., Hertel, H., de Souza, J., Ventura, D. F., and Menzel, R. (1992). The spectral input systems of hymenopteran insects and their receptor-based colour vision. J. Comp. Physiol. A 170, 23–40. doi: 10.1007/bf00190398
Phillips, R. D., Peakall, R., van der Niet, T., and Johnson, S. D. (2020). Niche perspectives on plant–pollinator interactions. Trends Plant Sci. 25, 779–793. doi: 10.1016/j.tplants.2020.03.009
Polturak, G., and Aharoni, A. (2018). “La Vie en Rose”: Biosynthesis, sources, and applications of betalain pigments. Mol. Plant 11, 7–22. doi: 10.1016/j.molp.2017.10.008
Price, T. D., Stoddard, M. C., Shevell, S. K., and Bloch, N. I. (2019). Understanding how neural responses contribute to the diversity of avian colour vision. Anim. Behav. 155, 297–305. doi: 10.1016/j.anbehav.2019.05.009
R Core Team (2021). R: A Language and Environment for Statistical Computing. Available online at: http://www.R-project.org
Rausher, M. D. (2008). Evolutionary transitions in floral color. Inter. J. Plant Sci. 169, 7–21. doi: 10.1086/523358
Renoult, J. P., Kelber, A., and Schaefer, H. M. (2017). Colour spaces in ecology and evolutionary biology. Biol. Rev. 92, 292–315. doi: 10.1111/brv.12230
Revell, L. J. (2012). phytools: An R package for phylogenetic comparative biology (and other things). Methods Ecol. Evol. 3, 217–223. doi: 10.1111/j.2041-210X.2011.00169.x
Reverté, S., Retana, J., Gómez, J. M., and Bosch, J. (2016). Pollinators show flower colour preferences but flowers with similar colours do not attract similar pollinators. Ann. Bot. 118, 249–257. doi: 10.1093/aob/mcw103
Ritchie, R. J. (2006). Consistent sets of spectrophotometric chlorophyll equations for acetone, methanol and ethanol solvents. Photosynth. Res. 89, 27–41. doi: 10.1007/s11120-006-9065-9
Roguz, K., Gallagher, M. K., Senden, E., Bar-Lev, Y., Lebel, M., Heliczer, R., et al. (2020). All the colors of the rainbow: Diversification of Flower color and intraspecific color variation in the genus Iris. Front. Plant Sci. 11:569811. doi: 10.3389/fpls.2020.569811
Roguz, K., Hill, L., Koethe, S., Lunau, K., Roguz, A., and Zych, M. (2021). Visibility and attractiveness of Fritillaria (Liliaceae) flowers to potential pollinators. Sci. Rep. 11:11006.
Rohde, K., Papiorek, S., and Lunau, K. (2013). Bumblebees (Bombus terrestris) and honeybees (Apis mellifera) prefer similar colours of higher spectral purity over trained colours. J. Comp. Physiol. A 199, 197–210. doi: 10.1007/s00359-012-0783-5
Sakuta, M. (2014). Diversity in plant red pigments: anthocyanins and betacyanins. Plant Biotechnol. Rep. 8, 37–48. doi: 10.1007/s11816-013-0294-z
Schoefs, B. (2004). Determination of pigments in vegetables. J. Chromatogr. A 1054, 217–226. doi: 10.1016/j.chroma.2004.05.105
Shrestha, M., Lunau, K., Dorin, A., Schulze, B., Bischoff, M., Burd, M., et al. (2016). Floral colours in a world without birds and bees: the plants of Macquarie Island. Plant Biol. (Stuttg). 18, 842–850. doi: 10.1111/plb.12456
Smith, S. A., and Brown, J. W. (2018). Constructing a broadly inclusive seed plant phylogeny. Am. J. Bot. 105, 302–314. doi: 10.1002/ajb2.1019
Smith, S. D. (2014). Quantifying color variation: Improved formulas for calculating hue with segment classification. Appl. Plant Sci. 2:1300088. doi: 10.3732/apps.1300088
Smith, S. D., and Goldberg, E. E. (2015). Tempo and mode of flower color evolution. Am. J. Bot. 102, 1014–1025. doi: 10.3732/ajb.1500163
Spaethe, J., Tautz, J., and Chittka, L. (2001). Visual constraints in foraging bumblebees: Flower size and color affect search time and flight behavior. Proc. Natl. Acad. Sci. 98, 3898–3903. doi: 10.1073/pnas.071053098
Stavenga, D. G., Leertouwer, H. L., Dudek, B., and van der Kooi, C. J. (2021). Coloration of flowers by flavonoids and consequences of pH dependent absorption. Front. Plant Sci. 11:600124. doi: 10.3389/fpls.2020.600124
Stoddard, M. C., and Prum, R. O. (2008). Evolution of avian plumage color in a tetrahedral color space: A phylogenetic analysis of new world buntings. Am. Nat. 171, 755–776. doi: 10.1086/587526
Stoddard, M. C., Eyster, H. N., Hogan, B. G., Morris, D. H., Soucy, E. R., and Inouye, D. W. (2020). Wild hummingbirds discriminate nonspectral colors. Proc. Natl. Acad. Sci. 117, 15112–15122.
Strack, D., Engel, U., and Reznik, H. (1981). High performance liquid chromatography of betalains and its application to pigment analysis in Aizoaceae and Cactaceae. Zeitschrift für Pflanzenphysiol. 101, 215–222. doi: 10.1016/s0044-328x(81)80027-x
Strack, D., Vogt, T., and Schliemann, W. (2003). Recent advances in betalain research. Phytochemistry 62, 247–269. doi: 10.1016/S0031-9422(02)00564-2
Strauss, S. Y., and Whittall, J. B. (2006). “Non-pollinator agents of selection on floral traits,” in Ecology and evolution of flowers, eds L. D. Harder and S. C. H. Barret (Oxford, U.K: Oxford University Press), 120–138.
Sullivan, C. N., and Koski, M. H. (2021). The effects of climate change on floral anthocyanin polymorphisms. Proc. R. Soc. B Biol. Sci. 288:20202693. doi: 10.1098/rspb.2020.2693
Tanaka, Y., Sasaki, N., and Ohmiya, A. (2008). Biosynthesis of plant pigments: anthocyanins, betalains and carotenoids. Plant J. 54, 733–749. doi: 10.1111/j.1365-313X.2008.03447.x
Thrane, J. E., Kyle, M., Striebel, M., Haande, S., Grung, M., Rohrlack, T., et al. (2015). Spectrophotometric analysis of pigments: A critical assessment of a high-throughput method for analysis of algal pigment mixtures by spectral deconvolution. PLoS One 10:e0137645. doi: 10.1371/journal.pone.0137645
Timoneda, A., Feng, T., Sheehan, H., Walker-Hale, N., Pucker, B., Lopez-Nieves, S., et al. (2019). The evolution of betalain biosynthesis in Caryophyllales. New Phytol. 224, 71–85. doi: 10.1111/nph.15980
Troje, N. (1993). Spectral categories in the learning behaviour of blowflies. Zeitschrift fur Naturforsch. C J. Biosci. 48, 96–104. doi: 10.1515/znc-1993-1-218
Tunes, P., Camargo, M. G. G., and Guimarães, E. (2021). Floral UV features of plant species from a Neotropical savanna. Front. Plant Sci. 12:618028. doi: 10.3389/fpls.2021.618028
Valdés, B. (1970). Flavonoid pigments in flower and leaf of the genus Linaria (Scrophulariaceae). Phytochemistry 9, 1253–1260.
van den Berg, C. P., Troscianko, J., Endler, J. A., Marshall, N. J., and Cheney, K. L. (2020). Quantitative Colour Pattern Analysis (QCPA): A comprehensive framework for the analysis of colour patterns in nature. Methods Ecol. Evol. 11, 316–332. doi: 10.1111/2041-210X.13328
van der Kooi, C. J., Dyer, A. G., Kevan, P. G., and Lunau, K. (2019). Functional significance of the optical properties of flowers for visual signalling. Ann. Bot. 123, 263–276. doi: 10.1093/aob/mcy119
van der Kooi, C. J., Stavenga, D. G., Arikawa, K., Belušič, G., and Kelber, A. (2021). Evolution of insect color vision: From spectral sensitivity to visual ecology. Annu. Rev. Entomol. 66, 435–461. doi: 10.1146/annurev-ento-061720-071644
Vorobyev, M., and Brandt, R. (1997). How do insect pollinators discriminate colors? Israel J. Plant Sci. 45, 103–113.
Vorobyev, M., and Osorio, D. (1998). Receptor noise as a determinant of colour threshoIds. Proc. R. Soc. B Biol. Sci. 265, 351–358. doi: 10.1098/rspb.1998.0302
Wang, Y., Zhang, C., Dong, B., Fu, J., Hu, S., and Zhao, H. (2018). Carotenoid accumulation and its contribution to flower coloration of Osmanthus fragrans. Front. Plant Sci. 871:1499. doi: 10.3389/fpls.2018.01499
Warren, J., and Mackenzie, S. (2001). Why are all colour combinations not equally represented as flower-colour polymorphisms? New Phytol. 151, 237–241. doi: 10.1046/j.1469-8137.2001.00159.x
Wessinger, C. A., Rausher, M. D., and Hileman, L. C. (2019). Adaptation to hummingbird pollination is associated with reduced diversification in Penstemon. Evol. Lett. 3, 521–533.
Whitney, K. D., Smith, A. K., White, T. E., and Williams, C. F. (2020). Birds perceive more intraspecific color variation in bird-pollinated than bee-pollinated flowers. Front. Plant Sci. 11:590347. doi: 10.3389/fpls.2020.590347
Yoshida, K., Mori, M., and Kondo, T. (2009). Blue flower color development by anthocyanins: From chemical structure to cell physiology. Nat. Prod. Rep. 26, 884–915. doi: 10.1039/b800165k
Yoshida, M., Itoh, Y., Ômura, H., Arikawa, K., and Kinoshita, M. (2015). Plant scents modify innate colour preference in foraging swallowtail butterflies. Biol. Lett. 11:20150390.
Yuan, H., Zhang, J., Nageswaran, D., and Li, L. (2015). Carotenoid metabolism and regulation in horticultural crops. Hortic. Res. 2:15036. doi: 10.1038/hortres.2015.36
Keywords: anthocyanins, carotenoids, chlorophylls, colour space models, flavonoids, flower colour, flower pigments, pollinators
Citation: Narbona E, del Valle JC, Arista M, Buide ML and Ortiz PL (2021) Major Flower Pigments Originate Different Colour Signals to Pollinators. Front. Ecol. Evol. 9:743850. doi: 10.3389/fevo.2021.743850
Received: 19 July 2021; Accepted: 15 September 2021;
Published: 05 October 2021.
Edited by:
Casper J. Van Der Kooi, Groningen Institute for Evolutionary Life Sciences, University of Groningen, NetherlandsReviewed by:
Doekele G. Stavenga, University of Groningen, NetherlandsAdrian G. Dyer, RMIT University, Australia
Matthew Koski, Clemson University, United States
Copyright © 2021 Narbona, del Valle, Arista, Buide and Ortiz. This is an open-access article distributed under the terms of the Creative Commons Attribution License (CC BY). The use, distribution or reproduction in other forums is permitted, provided the original author(s) and the copyright owner(s) are credited and that the original publication in this journal is cited, in accordance with accepted academic practice. No use, distribution or reproduction is permitted which does not comply with these terms.
*Correspondence: Eduardo Narbona, ZW5hcmZlckB1cG8uZXM=
†These authors have contributed equally to this work and share first authorship