- 1Mitrani Department of Desert Ecology, Blaustein Institutes for Desert Research, Ben-Gurion University of the Negev, Beersheba, Israel
- 2Shraga Segal Department of Microbiology, Immunology, and Genetics, Faculty of Health Sciences, Ben-Gurion University of the Negev, Beersheba, Israel
- 3Department of Integrated Mathematical Oncology, Moffitt Cancer Center, Tampa, FL, United States
As cancer progresses, its impact should manifest in the foraging behavior of its host much like the effects of endo-parasites that hinder foraging aptitudes and risk management abilities. Furthermore, the lifestyle of the host can impact tumor growth and quality of life. To approach these questions, we conducted novel experiments by letting C57BL/6 laboratory mice, with or without oral squamous cell carcinoma, free range in a large outdoor vivarium. Our goals were to: (1) determine whether one could conduct experiments with a mouse model under free range conditions, (2) measure effects of cancer burden on foraging metrics, (3) compare tumor growth rates with laboratory housed mice, and (4) begin to sort out confounding factors such as diet. With or without cancer, the C57BL/6 laboratory mice dealt with natural climatic conditions and illumination, found shelter or dug burrows, sought out food from experimental food patches, and responded to risk factors associated with microhabitat by foraging more thoroughly in food patches under bush (safe) than in the open (risky). We quantified foraging using giving-up densities of food left behind in the food patches. The mice’s patch use changed over time, and was affected by disease status, sex, and microhabitat. Males, which were larger, consumed more food and had lower giving-up densities than females. Relative to cancer-free mice, mice with growing tumors lost weight, harvested more food, and increasingly relied on patches in the bush microhabitat. The tumors of free-ranging mice in the vivarium grew slower than those of their cohort that were housed in mouse cages in animal facilities. Numerous interesting factors could explain the difference in tumor growth rates: activity levels, stress, weather, food intake, diet, and more. To tease apart one of these intertwined factors, we found that tumors grew faster when mice in the laboratory were fed on millet rather than laboratory mouse chow. While just a start, these novel experiments and framework show how free-ranging mice provide a model that can test a broader range of hypotheses and use a broader range of metrics regarding cancer progression and its consequences for the host.
Introduction
Cancer experiments with mice invariably involve very small spaces (laboratory cages), ad lib food, the simplest of lifestyles, and little to no habitat heterogeneity. Here we explore the potential for using mouse model experiments in large outdoor enclosures or vivaria using the inbred laboratory mouse strain C57BL/6. This strain dates from 1921 and may be the most widely used mouse model in research (Festing, 1979; Rao et al., 1988; Song and Hwang, 2017). We offered each of four groups of C57BL/6 laboratory mice (male vs. female; and cancer vs. cancer-free) an outdoor space measuring 8.5 m × 17 m. The results are promising and instructive with respect to insights into foraging behaviors, tumor growth rates, and diet.
Experiments in large outdoor enclosures have a long history in ecological studies. Enclosures have ranged in size from a few square meters in an indoor setting (e.g., Dice, 1945; Clarke, 1983; Morris et al., 2017; Mowry et al., 2017), somewhat larger semi-natural outdoor enclosures of one to several hundred square meters (e.g., Brown et al., 1988; Kotler et al., 1991; Meagher et al., 2000; Bär et al., 2020), on up to large natural outdoor enclosures of one to many hectares (e.g., Abramsky et al., 1991, 1997, Rohner and Krebs, 1996). The subject matters of these studies are diverse and range from ecological questions such as food-safety trade-offs, locomotion, and territoriality (Morris et al., 2017) to evolutionary issues such as body mass and sexual selection (Meagher et al., 2000).
More recently, semi-natural enclosures have been used to study both wild-caught and laboratory-bred house mice (e.g., Ruff et al., 2017; Phifer-Rixey et al., 2018), including various “rewilding” experiments with C57BL/6 laboratory mice (Leung et al., 2018; Cope et al., 2019; Bär et al., 2020; Graham, 2021). Medically related questions with laboratory mice have included the role of fungi in immune function (Yeung et al., 2020), the effects of high levels of sugar consumption on mortality (Ruff et al., 2013), the role of sex and social organization on rates of pathogen transmission (Cornwall et al., 2021), and the magnitude of inbreeding effects (Meagher et al., 2000). Such settings can measure the performance of mice as an assay for the safety of pharmaceuticals (Gaukler et al., 2016a,b).
Large outdoor vivaria have shown particular utility in foraging ecology (e.g., Brown et al., 1988; Kotler et al., 1991, 2010; Embar et al., 2011). In such experiments, rodents respond to predators (e.g., owls, snakes, and foxes) by foraging less and shifting foraging toward safer microhabitats. The vivarium used in the current study also provided the setting for experiments with wild gerbils and other desert rodents to address issues ranging from the interplay of state, time allocation, and vigilance in optimal foraging decisions over the lunar cycle (Kotler et al., 2010), the role of sight lines (Embar et al., 2011), and the consequences of compromise-breaking adaptations in understanding limited convergence between rodents from different continents (Kotler et al., 2016). With respect to disease burden, wild-caught gerbils infected with an endoparasitic bacteria (Mycoplasma haemomuris-like bacteria) harvested less food, exhibited less effective anti-predator responses, and were more susceptible to predation by owls (Makin et al., 2020). Cancer burdens in mouse models might elicit similar responses. Free-range experiments in large enclosures represent a scaling down of space when using wild-caught rodents, whereas they represent a scaling up for laboratory mice that may not have seen a space larger than a laboratory cage in 100 or more generations.
In the vivarium, as in many field studies of rodents, foraging behaviors can be measured using experimental food patches in which a known amount of seeds is mixed into a substrate that requires the rodent to dig, search for, and harvest seeds. This creates diminishing returns: as the patch becomes depleted, the animal’s harvest rate declines. Eventually, the forager abandons the patch. The seeds remaining in the patch, the giving-up density (GUD), can be collected and measured to provide data on the amount of seeds harvested and the willingness of the forager to work for additional food. The less food left, the more the animal was willing to work. The giving-up density declines (more food harvested) in patches perceived as safer from predation risk, and for animals that have a higher need for food (Brown, 1988, 1992). Giving-up densities in such food patches can provide both behavioral enrichments and measures of foraging behavior in the free-ranging C57BL/6 mice.
C57BL/6 mice free-ranging in the vivarium need to contend with natural fluctuations in temperature and the need to thermoregulate. There, they are no longer housed under sterile or near-sterile conditions. They must spend several hours each night visiting seed trays and digging through the sand to find and harvest seeds. The mice in the vivarium must seek shelter and avoid risky locations. In general, studies reveal that wild-caught rodents perceive greater predation risk away from cover vs. under cover (open vs. bush microhabitat) (Brown et al., 1988; Kotler et al., 1991), have lower giving-up densities in the bush microhabitat (Brown et al., 1988; Kotler et al., 1991; Kotler and Blaustein, 1995), and have lower giving-up densities when in a lower body condition or when experiencing a greater need for food (Kotler, 1997; Kotler et al., 2004; Berger-Tal and Kotler, 2010; Berger-Tal et al., 2010). Under this more complex lifestyle, we can test hypotheses for how cancer and its progression influence food harvest, giving-up densities, and use of open and bush microhabitats.
Exercise (Zielinski et al., 2004; Jones et al., 2012; Betof et al., 2015; Idorn and Thor Straten, 2017), food limitation (Lee et al., 2012; Nencioni et al., 2018), and stress (Grimm et al., 1996; Thaker et al., 2006; Kokolus et al., 2013) all have been shown to affect tumor growth rates, generally resulting in slower tumor growth rates. Free-ranging C57BL/6 mice as compared to their counterparts remaining in the laboratory will likely exercise more, contend with food limitation, and experience more and different forms of stress. To explore this, we compared tumor growth rates of a cohort of mice that were inoculated with the same batch of cancer cells on the same day, and then split into those remaining in the laboratory and those transferred to the vivarium.
Diet is known to influence tumor growth rates (e.g., Wang et al., 1995; Rose et al., 1999; O’Neill et al., 2016). To measure patch use and for consistency with other studies with wild-caught rodents, we used millet seeds. To initiate this novel and unusual experiment, the animal care committee allowed us to use millet for the free-range mice, but not for those housed in the laboratory (thus confounding free-range with diet regarding tumor growth rates). With the successful application of this approach to the free-range mice, we subsequently received permission to compare tumor growth rates of laboratory mice fed on mouse chow pellets or fed on just millet. Millet is lower in protein than the mouse chow pellets, and high protein diets have been shown to slow tumor growth in mouse models (Ho et al., 2011) and associated ketogenic diets (Chung and Park, 2017; Weber et al., 2020). Alternatively, millet is known to be high in antioxidants, often seen as anti-tumorigenic (Parohan et al., 2019). In addition to these alternative hypotheses, millet provides a compelling food source. Millet is one of the ancient grains associated with the Fertile Crescent. It is likely associated with the original evolution and dispersal of the ancestor of C57BL/6 laboratory mice, the house mouse, Mus musculus domesticus (Cucchi and Vigne, 2006; Cucchi et al., 2020), as well as the house sparrow, Passer domesticus (Boursot et al., 1993; Whelan et al., 2015). These animals and their laboratory descendants may be specialists at consuming cereal grains such as millet.
Methods
For all experiments, we used C57BL/6 laboratory mice. For cancer research, they are favored for being permissive of many injected cancer cell lines while remaining immuno-competent.
Effects of Microhabitat, Sex, and Cancer on Foraging Behaviors
C57BL/6 mice are being used more frequently within the free-range contexts of outdoor enclosures to address ecological, evolutionary, and even medical questions (e.g., Gaukler et al., 2016a, b; Cope et al., 2019; Bär et al., 2020; Yeung et al., 2020). With Experiment 1A, we tested whether free ranging, C57BL/6 laboratory mice forage in a manner typical of house mice and other seed-eating rodents in nature; and we tested for the effects of cancer on foraging behavior.
Experiment 1A took place in a large, outdoor vivarium (Figure 1) on the Sede Boker Campus of Ben-Gurion University of the Negev in Midreshet Ben-Gurion, Israel (30.857274°N, 34.780942°E) and Experiment 1B in laboratories located there and on the Marcus Family Campus of Ben-Gurion University in Beer Sheva, Israel. Sede Boker is a small rural town of less than 1,000 inhabitants located on the 90 mm rainfall isopleth in the Negev Desert. The vivarium is located there on the desert’s edge. The vivarium is exposed to ambient conditions, including illumination, humidity, and temperature. Natural predators in the vicinity include barn owls (Tyto alba), red foxes (Vulpes vulpes), and Clifford’s desert diadem snakes (Spalerosophis cliffordi). During the vivarium experiments, daytime highs averaged 33.56 ± 0.244 (s.e.) °C and nighttime lows 19.59 ± 0.463 (s.e.) °C. No precipitation fell then, but dew occurred on most nights. The vivarium is a rectangular outdoor enclosure (17 × 34 × 4.5 m high) enclosed with chicken wire sides and roof. It is also bounded with a rodent-proof wall that extends 1 m below ground and another 1 m above ground. Inside the vivarium, two 1 m tall and 1 m deep walls run east to west and north to south, respectively. These walls divide the vivarium into four equally sized 17 × 8.5 m quadrants. We placed 5 water dishes and three nest boxes in each quadrant. Mice were free to occupy nest boxes or burrows that they could either find or dig for themselves. We provided seed resources to rodents using experimental food patches, and quantified rodent foraging by measuring GUDs (giving-up densities: the amount of food left behind in a resource patch after an animal has stopped feeding from the patch) in these patches. Except for the seeds that we provided in the food patches, there was little other food to be found.
Food patches consisted of plastic trays (25 × 25 × 10 cm) each filled with 3 l of sifted sand. Before each night of the experiment, we provisioned each tray with 6 g of millet seeds (11% crude protein, 4% crude fat, 8.5% crude fiber, total carbohydrates 73%) mixed thoroughly and randomly into the sand. Each quadrant received four pairs of trays for a total of 32 patches in the vivarium. Each pair of trays was separated from the adjacent pair by approximately 2–3.5 m. The trays within each pair were placed 1.5 m apart. One tray of each pair was covered by a low-lying wooden trellis (76 × 60 × 16 cm) covered in black shade cloth. This tray simulated the bush microhabitat typical of natural systems. The other tray of the pair was left uncovered and simulated the open microhabitat.
Most rodent species tested to date, whether in enclosures or free-living in nature, find the bush microhabitat to be safer than the open (e.g., Brown et al., 1988; Kotler et al., 1991; Longland and Price, 1991). They demonstrate this preference by harvesting more seeds from and having lower GUDs in trays placed in the bush microhabitat relative to those placed in the open microhabitat (e.g., Kotler et al., 1991, but see Brown, 1989 for a reversal of this pattern in the kangaroo rat, Dipodomys merriami). Prior researchers have shown that both laboratory rats and laboratory mice have lower GUDs when patches are covered (Arcis and Desor, 2003; Troxell-Smith et al., 2016). We expected laboratory mice in the vivarium to exhibit lower GUDs in the bush than in the open.
Prior to the vivarium experiment, 10 mice each marked with a uniquely numbered PIT (passive induction transponder) tag were placed in each quadrant (40 in total) of the vivarium. In two of the quadrants, all the mice were males; in two of the quadrants, all were females. This allowed us to test for sex differences in GUDs. The sex that finds energy more valuable or that feels safer will have the lower GUD.
To begin the experiment, all mice in two of the quadrants (one with 10 males; one with 10 females) were injected subcutaneously with a mouse-derived cancer cell line. The 20 mice in the remaining two quadrants were injected with saline. The cell line is derived from 4-Nitroquinoline N-oxide (4NQO) induced oral squamous cell carcinoma from a male C57BL/6 mouse (Hawkins et al., 1994; Badarni et al., 2019). We have sequenced and characterized these cell lines (Elkabets and Prasad, unpublished data). Prior to injection in the mice, the cell line was grown and maintained at 37°C in a humidified atmosphere at 5% CO2 in DMEM media supplemented with 1% l-glutamine 200 mM, 100 units each of penicillin and streptomycin, and 10% FBS. Cells were routinely tested for Mycoplasma infection and treated with appropriate antibiotics as needed (De-Plasma, TOKU-E, D022). For injection, cells were trypsinized and resuspended in 1X sterile PBS (c. 1 Million cells /injection). We made two subcutaneous injections per mouse, one in the left and one in the right flank (100 μl for each injection). In this way, each mouse developed two localized non-metastatic tumors. The control group was injected with sterile 1xPBS (100 μl in each side). All mice in both the experimental group and the control group were anesthetized for the procedures. Anesthesia was performed using an intraperitoneal injection of ketamine (80–100 mg/kg) and xylazine (10–12.5 mg/kg), following which mice were placed on a heating pad for recovery. Relative to the cancer-free mice, the cancer mice over time should see an increase in GUDs if their capacity to forage becomes impaired, or they may show a decrease in GUDs if they require more seeds to meet metabolic demands.
We quantified tumor volume twice a week by measuring length (mm) and width (mm) using a caliper and fitting them to the formula Volume (mm3) = (Length2 × Width ×π)/6. At the conclusion of the experiment, we also weighed final tumor mass. All animals were weighed weekly. For measurements, we either captured animals by hand from nest boxes or used Sherman live traps baited with millet seeds on nights without food patches. We injected all mice of Experiment 1 with cancer cells or with saline on 30 July 2020. Following this, we released mice into the vivarium a day after injection.
For mice in the vivarium, we gave them 2 days after release to acclimate, after which we began collecting GUD data from the seed trays on 3 August 2020. Our experience with other species of rodents indicates that 2 days is sufficient acclimation to yield reliable data. Each week, for the next 4 weeks, we collected GUD data on four consecutive days centered on the moon phase and starting with the full moon. Prior to each night of data collection, we provisioned each seed tray with millet seeds (6 g). The following morning, we collected the remaining seeds from each tray and replenished seeds and sand to their original levels. We then returned the seeds to the laboratory where they were dried, cleaned of sand and debris, and weighed to obtain the GUD for each tray.
We analyzed the GUD data using an ANCOVA, with day as a covariate and GUD in a patch as the dependent variable (in grams). The rationale is that the tumors are growing with time and should change the disposition of the mice with cancer relative to those without. This will manifest as a day by cancer treatment interaction. Changes in risk management with time will manifest as a day by cancer treatment by microhabitat interaction. Hence, our interest is primarily in the interactions of group variables with day. The group variables were cancer treatment (cancer and cancer-free), sex (male and female), and microhabitat (bush and open). We included in our model the covariate of day and main effects of sex and microhabitat; the interaction of sex by microhabitat; the interaction of treatment by day; the interaction of treatment by sex by day; the interaction of treatment by microhabitat by day; the interaction of treatment by sex by microhabitat by day. Interactions of group variables (and their interactions) with day indicate differences in slopes, or rates at which GUDs changed per day. The data collection included the entire lunar cycle, but we did not include moon phase in our analysis as moon phases are associated with day, which in turn are associated with tumor growth in the cancer mice. As such, the effect of moon phase is subsumed in the covariate of day.
Effect of Free-Ranging on Tumor Growth Rates
Experiment 1B involved measuring the tumor growth rates of mice housed under standard laboratory conditions. Drawing randomly from the same set of mice as Experiment 1A and using the same batch of cancer cells on the same day (30 July 2020), we injected mice that were then kept in two laboratory locations: Sede Boker (3 males and 3 females) and Beer Sheva (5 males and 6 females). The Beer Sheva facility maintains SPF (Specific Pathogen Free) conditions, the Sede Boker facility does not. Because of the animal care regulations for the SPF conditions at the Beer Sheva facilities, mice in Beer Sheva could only be fed sterilized mouse chow pellets. Accordingly, mice in both the Sede Boker and Beer Sheva laboratories received mouse chow pellets (Ssnif, Mouse Breeding V1154-300, 22.5% crude protein, 5.5% crude fat, 4.0% crude fiber, 6.0% crude ash, 1.0% calcium, 0.7% calcium). As in Experiment 1A, we quantified tumor volume twice a week, weighed animals weekly, and, at the conclusion of the experiment, weighed final tumor masses.
We analyzed the tumor growth rates in a similar manner as the GUD data. We summed the tumor volume from both sides of a mouse and then under the assumption of exponential tumor growth, we took the natural logarithm of this value as our dependent variable. For the ANCOVA of the tumor growth rates measured during Experiment 1 (the vivarium and the concurrent laboratory trials), day was the covariate, with location (free-range in the vivarium, Beer Sheva lab, and Sede Boker lab) and sex (male and female) as group variables.
Effect of Diet on Tumor Growth Rates
In comparing tumor growth rates between mice of Experiments 1A and 1B there is a confounding of diet and location. To test for effects of a diet of millet or mouse chow pellets on tumor growth rates, we ran Experiment 2 in the laboratory in Sede Boker where both millet and mouse chow pellets were permitted. We began by giving each individual in two groups of mice cancer as described above. One group then received millet ad lib, the other mouse chow ad lib. Each group consisted of 6 males and 6 females for a total of 24 mice. We injected mice with cancer cells as described above on November 30, 2020. We weighed mice weekly and measured tumor volume twice a week. We ended the experiment on January 4, 2021, euthanized individual mice, and then resected, weighed, and preserved tumors.
For tumor growth rates in response to diet, we used the same ANCOVA as with tumor growth in the three locations, but with the adjustment that diet (millet and mouse chow pellets) substituted for location as a group variable. While seemingly appealing, we did not analyze tumor growth rates from both experiments together, as each of the two experiments used a different batch of cultured cancer cells, and batch effects can be significant (e.g., Karp et al., 2020). The same batch was used for all mice within an experiment.
For all experiments, we also used ANCOVA (one for each experiment) to track changes in body mass and the effects of the group variables, including housing conditions, cancer vs. cancer-free, and diet, as appropriate.
Results
Effects of Microhabitat, Sex, and Cancer on Foraging Behaviors
The ANCOVA of the GUDs in Experiment 1A provided a good fit to the data (r2 = 0.73). As expected, mouse GUDs were significantly and substantially higher in the open [4.23 ± 0.08 (s.e.) g] than bush [1.72 ± 0.06 (s.e.) g] microhabitat [MS = 802.1, F(1, 496) = 1075.2, p < 0.001]. Furthermore, males had significantly lower GUDs than females: 2.48 ± 0.08 (s.e.) g vs. 3.48 ± 0.11 (s.e.) g [MS = 135.3, F(1, 496) = 181.4, p < 0.001]. Note that males are larger in body mass than females. Males might also be more territorial than females (more often found alone in nest boxes rather than in groups), although we did not quantify this. Overall, males harvested 5.63 g of millet per day per individual, and females harvested 4.63 g per day per individual. Rather than consuming all of this, the mice can be expected to have cached some. Most notably, there is a significant interaction effect between microhabitat and sex (Figure 2) showing that the higher GUDs of females relative to males was much more pronounced in the open than bush microhabitat [MS = 48.5, F(1, 496) = 65.0, p < 0.001]. This result suggests that females are warier of the risky open areas than males (see Kotler et al., 1988, 1991 for experimental evidence showing that differences in GUDs across microhabitat is caused by predation risk).
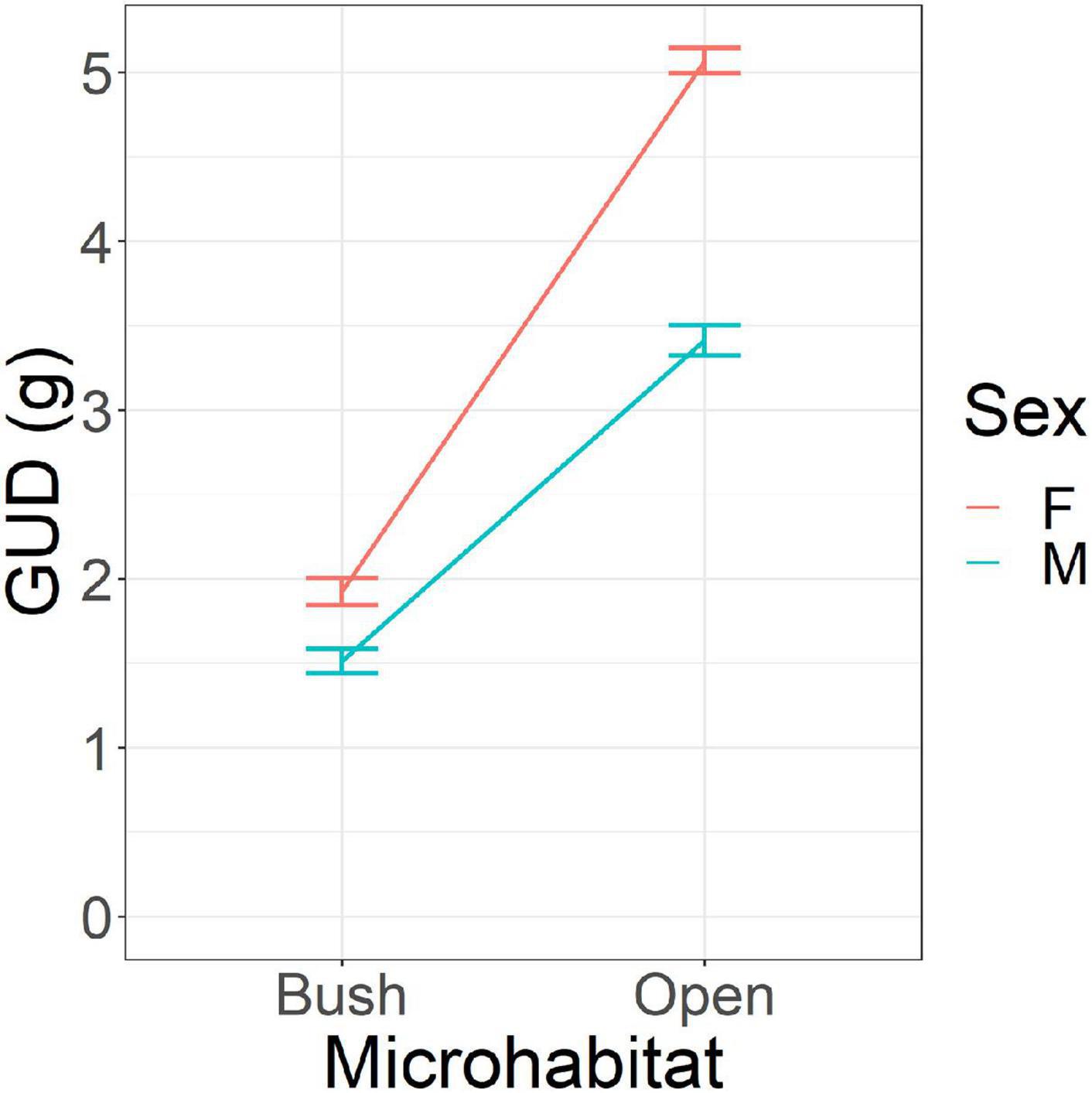
Figure 2. Giving-up densities (GUD) of mice in the vivarium according to microhabitat (bush and open) and sex. Females (F) have lower GUDs than males (M). All mice had lower GUDs in the bush than the open. Error bars represent standard errors of the means.
There were significant temporal trends in GUDs during the experiment, during which time the tumors were growing in the mice with cancer. Overall, GUDs tended to decline with time at a rate of −0.017 g per day [MS = 10.28, F(1, 496) = 13.78, p < 0.001]. There was a significant microhabitat by cancer treatment by day effect [MS = 4.12, F(1, 496) = 5.53, p < 0.02], showing that a growing tumor burden influenced foraging behavior. In the bush microhabitat, the GUDs of cancer mice changed little with time (overall rate of increase of 0.001 g per day) while that of cancer-free mice increased substantially with time (0.035 g per day). In the open microhabitat, the GUDs of both cancer and cancer-free mice declined similarly with time (−0.050 and −0.057 g per day, respectively), with cancer mice tending to have higher GUDs than cancer-free mice. While all mice began to shift more of their foraging toward the open microhabitat with time (suggesting perhaps a growing sense of safety), this trend was stronger for the cancer-free mice than for the cancer mice. This suggests possibly greater divergence in wariness over the course of the experiment by the cancer mice as their tumor burdens increased.
There was a significant cancer treatment by sex by day effect on GUDs [MS = 5.17, F(1, 496) = 6.925, p < 0.01; Figures 3A,B). For females, cancer and cancer-free mice saw a decline in GUDs with time, though more strikingly for females with cancer than those without (−0.034 and −0.013 g per day, respectively). For males, declines in GUDs with time were less pronounced than for females, with less difference between cancer and cancer-free males (−0.016 and −0.009 g per day, respectively). There was a significant cancer treatment by sex by microhabitat by day effect [MS = 4.09, F(1, 496) = 5.485, p < 0.02]. It shows that in the open microhabitat all four groups of mice (cancer treatment by sex) exhibited nearly identical daily declines in GUDs (Figure 3B), with GUDs for cancer mice being higher than cancer-free mice. This is suggestive of higher foraging costs for cancer mice. But in the bush microhabitat, there were striking divergences in daily trends, with cancer-free males and females showing sharp increases in GUDs with time, cancer males exhibiting a smaller increase in GUDs with time, and cancer females showing a temporal decline in daily GUDs (Figure 3A). By the end of the experiment, cancer males achieved lower GUDs in the bush than cancer-free males. This suggests increasing energy demands for cancer mice relative to cancer free mice.
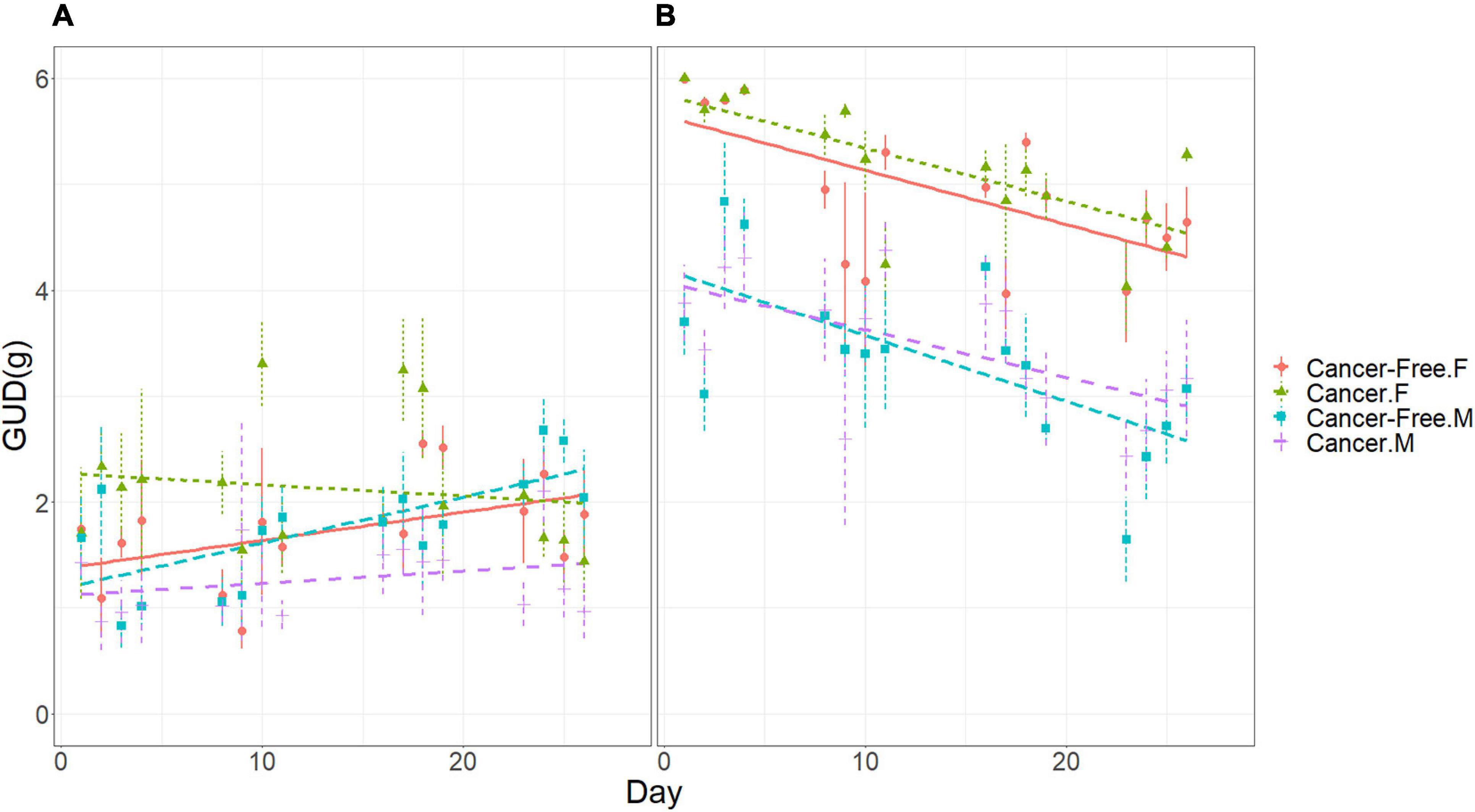
Figure 3. Giving-up densities (GUDs) of mice in the vivarium according to cancer treatment, sex, and date. (A) Bush microhabitat. (B) Open microhabitat. Error bars represent standard errors of the means.
Effect of Free-Ranging on Tumor Growth Rates
In Experiment 1 (A and B), both sex and housing conditions (free-range, Sede Boker facility, and Beer Sheva facility) influenced tumor growth rates. Mice at all locations started the experiment with similar tumor volumes (Figure 4B). Furthermore, the initial tumor volume was larger for males than females and remained so throughout the experiment [Figure 4A; M.S. = 9.483, F(1, 256) = 55.6, p < 0.001]. Tumor volume increased at a rate of 9.4% per day during the experiment [M.S. = 127.7, F(1, 256) = 748.7, p < 0.001], with similar rates for males and females. Finally, tumor growth rates differed in the three locations, with similarly high rates in the laboratory of 10.5% per day in Beer Sheva and 9.7% per day in Sede Boker, and a much lower rate of 8.0% per day in the free-range vivarium mice [Figure 4B; M.S. = 1.165, F(1, 256) = 6.83, p = 0.001]. The growth rate differences between caged facilities and the free-range vivarium matters. Exponential growth rates of 10% vs. 8% per day will result in a tumor that is 80% larger after 30 days (20-fold vs. 11-fold increase).
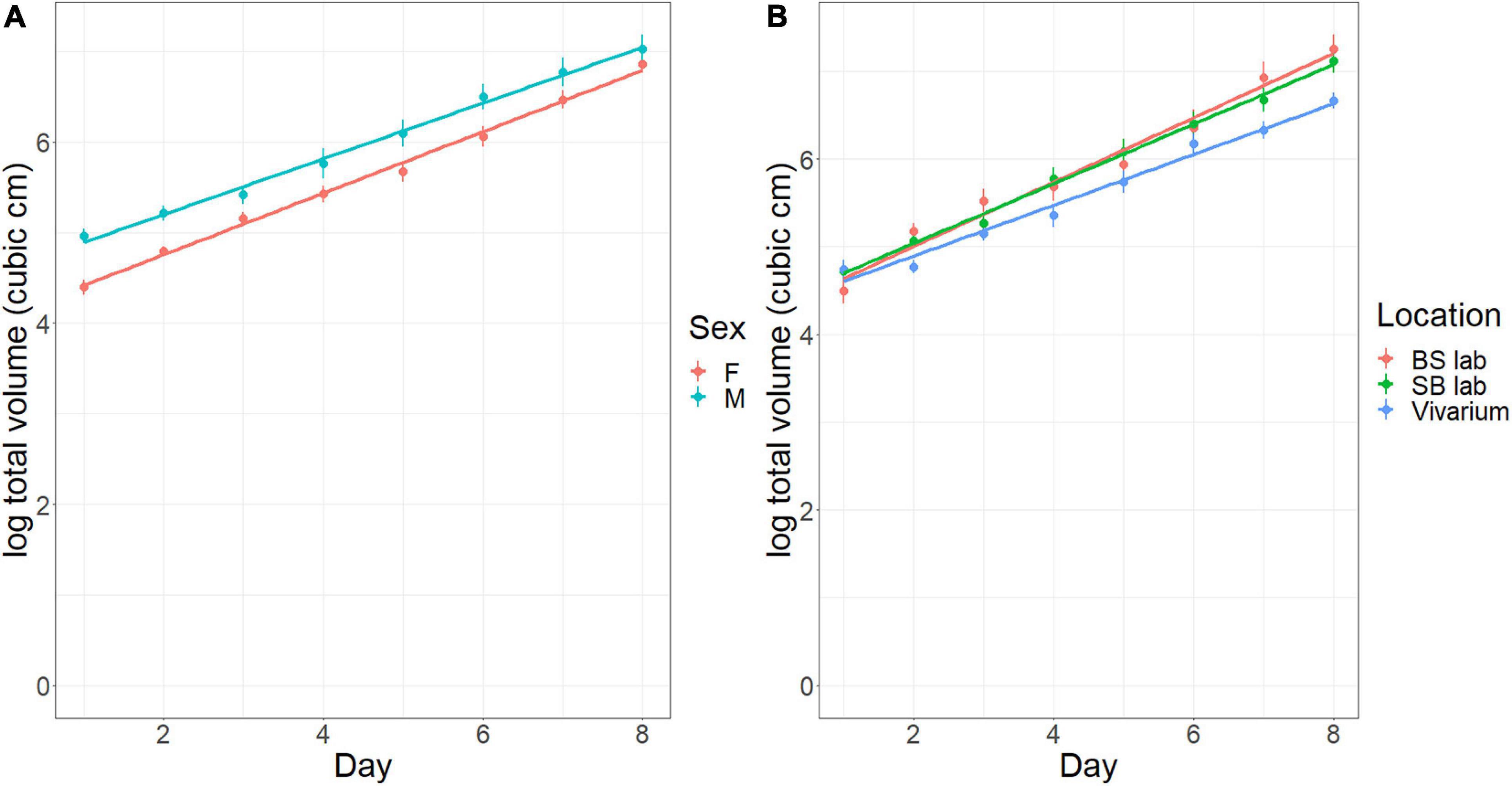
Figure 4. Tumor size (cm3) in the vivarium experiment and concurrent laboratory experiment over time (days). (A) Effect of sex (F, female; M, male). (B) Effect of Location (BS laboratory, laboratory in Beer Sheva with SPF conditions; SB laboratory, laboratory in Sede Boker; Vivarium, large, outdoor vivarium in Sede Boker). Error bars represent standard errors of the means.
In terms of body mass, males were significantly larger than females in Experiment 1 (A and B) (23.7 vs. 19.0 g, respectively, at day zero). For free-ranging mice in the vivarium, those with cancer declined in body mass over time (−0.024 grams per day) while those without saw little or no change (−0.006 g per day) [ANCOVA: significant interaction of cancer treatment with day, M.S. = 30.89, F(1, 198) = 6.73, p < 0.01)] (Figure 5A). This occurred despite the mice with cancer increasing their daily food harvest relative to the cancer-free mice over time. In a separate analysis, for mice with cancer, those in the laboratory increased in body mass over the 31 days of the experiment (0.06 g per day), while as noted, those in the vivarium declined in mass with time [ANCOVA: significant interaction of location by day, M.S. = 30.89, F(1, 198) = 6.73, p < 0.01] (Figure 5B). Although males are heavier than females, the location specific trends were similar for both sexes (Figure 5A).
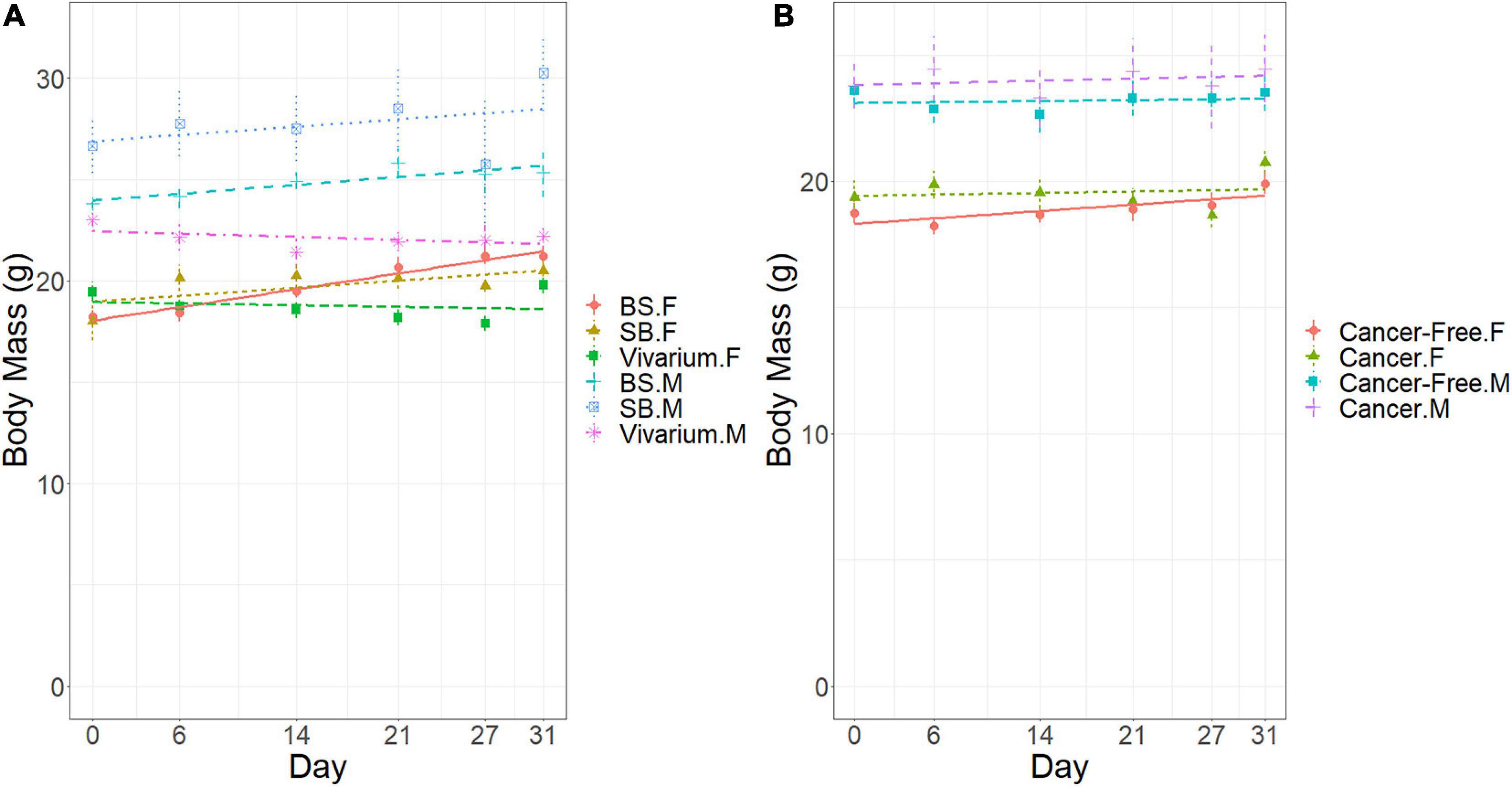
Figure 5. Body mass (g) of the mice in the vivarium experiment over time (days). (A) Effects of sex (F, female; M, male) and location (Vivarium, Laboratory). (B) Effects of sex (F, female; M, male) and cancer treatment (Yes, cancer; cancer-free). Error bars represent standard errors of the means.
During Experiment 1A, one cancer mouse male was found dead in the vivarium on 17 August and another cancer mouse male on 20 August. Each was replaced the following day by a cancer mouse male kept in the laboratory for such purposes. In addition, a cancer mouse female and a cancer-free female were killed on 13 August by a snake that managed to enter the vivarium. The snake was removed and released, and the mice were replaced on August 14th.
Effect of Diet on Tumor Growth Rates
In Experiment 2 conducted in the Sede Boker facility, diet significantly affected tumor growth rates. As before, the initial tumor volume was larger for males than females [M.S. = 0.932, F(1,210) = 6.058, p < 0.02], and thereafter remained larger as tumors in males and females grew at the same rate (Figure 6A). Tumors grew at a rate of 9.3% per day [M.S. = 156.14, F(1,210) = 1015.7, p < 0.001]. Diet did not affect initial mass, but did influence tumor growth rates. Mice fed on millet had significantly higher tumor growth rates than mice eating mouse chow pellets [M.S. = 1.385, F(1, 210) = 9.007, p = 0.003] at 9.8% per day and 8.8% per day, respectively (Figure 6B). After 30 days, this difference leads to tumors that are 35% larger in mice fed on millet than those fed on mouse chow.
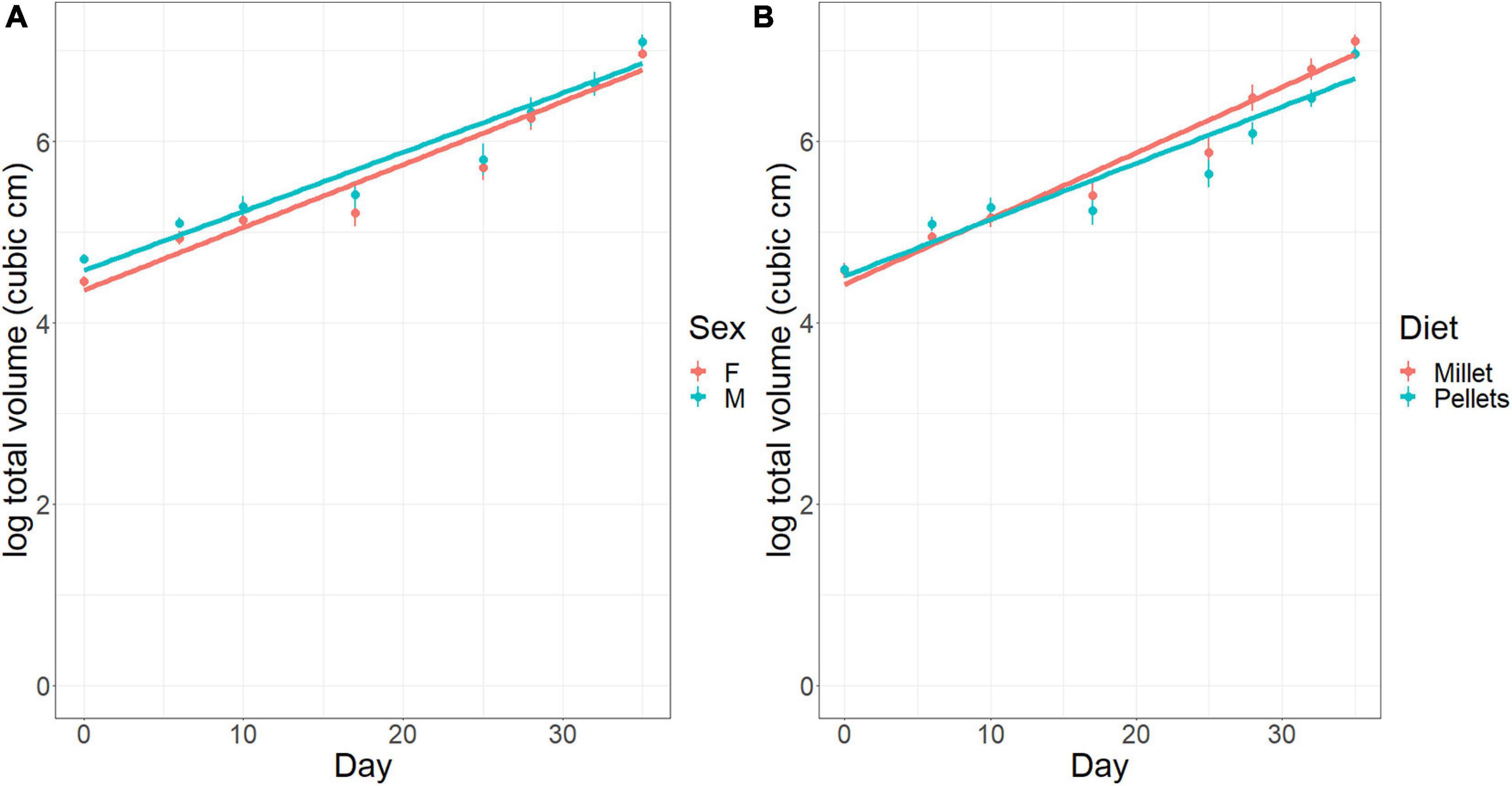
Figure 6. Tumor size (cm3) in the laboratory diet experiment over time (days). (A) Effect of sex (F, female; M, male). (B) Effect of diet (Millet, mice fed on millet seeds; Pellets, mice fed on mouse chow pellets). Error bars represent standard errors of the means.
In terms of body mass in Experiment 2, like the first experiment, males were larger than females [23.7 ± 0.4 (s.e.) g vs. 18.9 ± 0.4 (s.e.) g, respectively, at day zero], and body mass increased over time for both diet treatments (0.089 g per day), with a strong trend of mice fed on millet gaining mass at a faster rate than those fed on mouse chow pellets (0.122 g per day vs. 0.055 g per day, respectively) [ANCOVA: interaction of diet with day, M.S. = 11.67, F(1, 140) = 3.45, p = 0.065] (Figure 7).
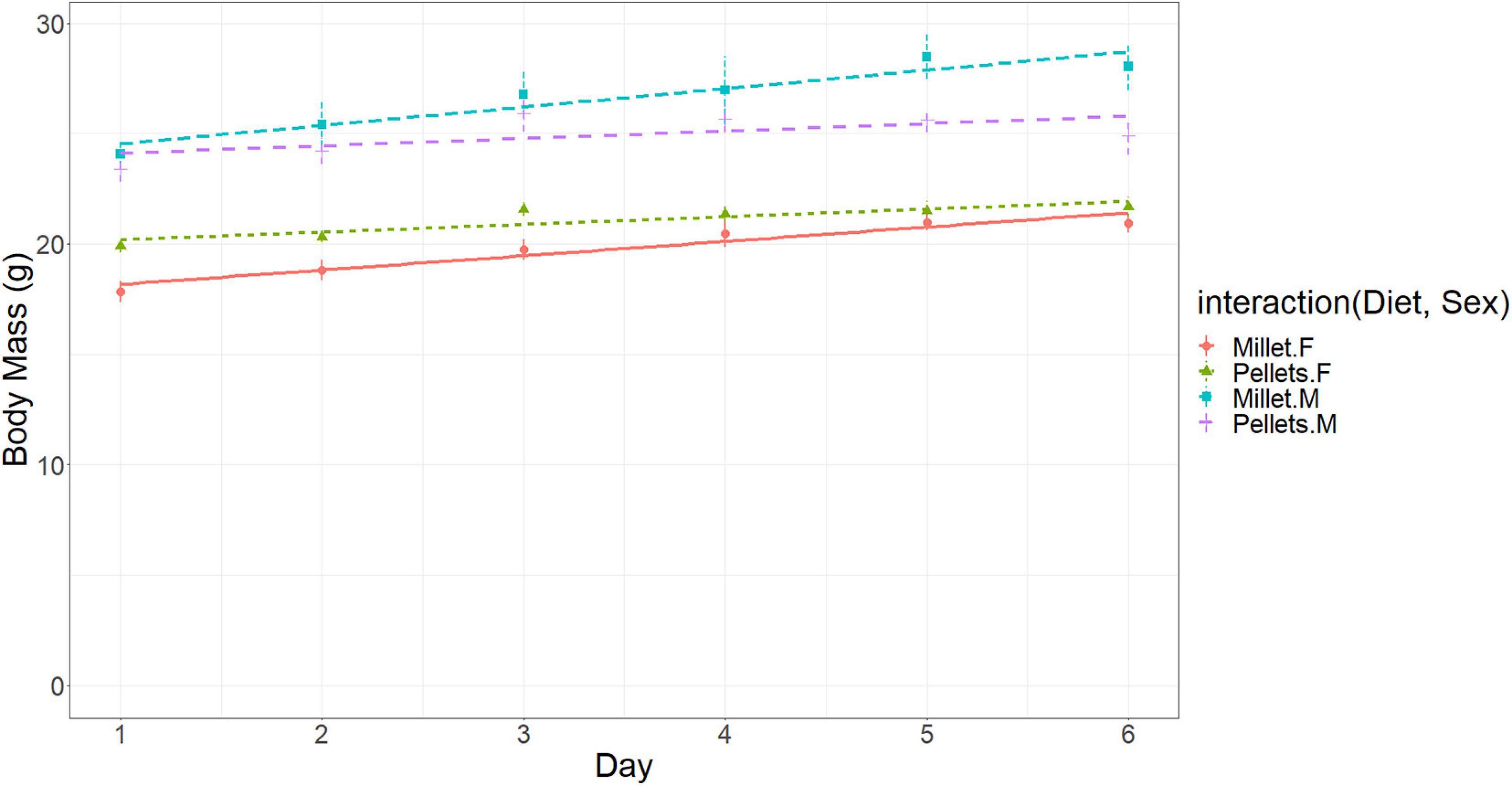
Figure 7. Body mass (g) of the mice in the diet experiment for cancer mice fed a diet of either Millet or Mouse Chow Pellets. Error bars represent standard errors of the means.
For both Experiments, the final mass of a tumor correlated tightly with the final measurement of tumor volume [Pearson’s correlation of 0.932 and 0.882 for the first (n = 67) and second (n = 48) experiments, respectively]. Recall that each cancer mouse had two tumors, one on its left flank and one on its right. At the end of the experiment there was some to no correlation across mice between the tumors on the right and left flanks [Pearson’s correlation of 0.526 and −0.017 for the first (n = 34) and second (n = 24) experiments, respectively].
Discussion
Our goals were to: (1) determine whether one could conduct experiments with a mouse model under free range conditions, (2) measure effects of cancer burden on foraging metrics, (3) compare tumor growth rates with laboratory housed mice, and (4) begin to sort out confounding factors such as diet.
Effects of Microhabitat, Sex, and Cancer on Foraging Behaviors
With respect to (1), laboratory mice, despite their pedigree, when released into the vivarium behaved as expected of wild rodents (e.g., Kotler et al., 1991). They sought shelter, they explored their environment, they searched for food and water, they dug through sandy substrates to harvest the millet seeds, and they traded off food and safety when making foraging decisions. They responded strongly to the risk factor of microhabitat and recognized the open microhabitat as intrinsically more dangerous than sheltered areas under bushes as reflected in their GUDs. Thus C57BL/6 mice free-ranging in the vivarium show reasonable behavior that is similar to wild rodents and so offer a potential mouse model for more complex and complete lifestyles.
With respect to (2), cancer in the C57BL/6 laboratory mice gave rise to measurable changes in foraging patterns. Over time, free-range mice with cancer harvested more food yet saw a loss in body mass relative to those without cancer. Based on models of patch use and foraging economics, we interpret this as the cancer mice having a greater need for energy (higher marginal value of energy) or perceiving less to live for (lower survivor’s fitness) as their tumors grew (Brown, 1992; Brown et al., 1997). Furthermore, mice with cancer became warier relative to the cancer-free mice. With time, mice with cancer harvested a greater and greater fraction of their food from the bush (safe) than open (risky) microhabitat relative to the cancer-free mice (note: both groups showed a general temporal tendency of harvesting more from the open).
Upon approaching a threshold tumor size, the mice were euthanized according to standard animal care protocols (for consistency, we euthanized all mice in Experiment 1 at the same time even though tumors were substantially smaller in the free-range than facility-housed mice). At that 30-day point, the free-range cancer mice continued to be highly active and forage extensively. They were not symptomatic to the extent that their foraging aptitudes were impaired or that they were debilitated with cachexia (body wasting). Presumably, had the tumors continued to grow, at some point the cancer mice’s GUDs would have increased, either through an increase in foraging time or from the symptomatic effects of the tumor burden.
Regarding cancer in natural populations, the increased foraging effort and weight loss seen in the free-range cancer mice might render such animals less successful at reproducing and competing for food, and more susceptible to predators. Overall, we would thus expect cancerous animals to disappear more rapidly from the population. Thus, we might expect cancer to be rarely observed in natural populations (Ewald and Ewald, 2017; Madsen et al., 2017) even beyond the direct mortality caused by the disease itself.
Because cancer induces changes in foraging metrics (GUDs and habitat selection) and because cancer induces changes in body condition, this may allow for an animal model for evaluating quality of life. With the free-ranging mice, one can objectively define critical points such as when GUDs or habitat choices begin to change mice with growing tumor burden relative to mice without. At what level of cancer progression do GUDs begin to increase and effective foraging cease? How quickly do these change manifest with cancer cell lines that vary in metastatic potential and potential to induce cachexia? Finally, how does therapy alter foraging behavior? We suggest that the vivarium-based system described here when coupled with various types of cancer and targeted therapies can provide quantitative metrics of quality of life.
We envisage that we can investigate quality of life issues quantitatively using behavioral indicators (i.e., GUDs) by giving laboratory mice the appropriate cancer and the appropriate therapy drug regime in a full factorial design. The experimental design would consist of: the baseline for healthy mice (cancer-free, no therapy drugs; expectation of low GUDs); the negative effects of cancer on quality of life (plus cancer, no therapy drugs — this experiment; expectation of first lower, and then higher GUDs that track the energetic demands and the deleterious impact of cancer); the negative effects of the therapy on quality of life (cancer-free, plus therapy drugs; expectation of higher GUDs reflecting the adverse effect of the therapy on quality of life); and the combined effect of both cancer and therapy drugs in potentially increasing the quality of life (plus cancer, plus therapy drugs; therapy worthwhile so long as GUDs are lower than for cancer alone or therapy alone). The behavioral indicators can then be linked to physiological indicators as well. The mice without cancer reveal in their GUDs how they would like to go about their foraging (among the most significant activities for a non-human mammal) vs. how they do go about their business when burdened with cancer and/or therapy.
Effect of Free-Ranging on Tumor Growth Rates
With respect to (3), we were able to demonstrate significantly lower tumor growth rates in the free-range mice relative to those housed in laboratory facilities. This is an intriguing result, that while not definitive in itself, shows the opportunity to track the growth of tumors in animals experiencing different ecological settings (Loizides et al., 2015). Differences could have been due to a multitude of factors including activity levels, stress, food intake, diet, and daily vicissitudes of weather and other stimuli, all factors known to or likely to influence tumor growth rates. While beyond our scope and resources, the present experiments show promise. Additional experiments could include mice housed in cages and placed in the vivarium so as to be exposed to the daily and nightly changes in climate and soundscape. Mice could be allowed to free-range in the vivarium, but have effort-free, ad lib access to food and water as is typical in the laboratory and some vivarium experiments, while mice in laboratory cages (sufficiently large, but still much smaller than a quadrant of the vivarium) would obtain their food from the seed trays used in our vivarium experiments. These experiments and more become intriguing and relevant in light of our results.
Regardless of husbandry, tumors were larger in males than females (Clocchiatti et al., 2016; Kim et al., 2018). The 4NQO cancer cell line was derived from a male mouse (Badarni et al., 2019). Its establishment at a larger size in males than females may reflect a boost from androgens (e.g., Birrell et al., 1995; Trigunaite et al., 2015). Beyond that, in humans, males and females often show different incidences of cancer, different outcomes from therapy, and different mortality rates (Clocchiatti et al., 2016). These may be due to different sensitivities of the cancer cells to estrogens and androgens, the role of sex chromosomes (Birrell et al., 1995), or the manner in which androgens suppress the immune system (Trigunaite et al., 2015), among other possible causes.
Effect of Diet on Tumor Growth Rates
With respect to (4), we began to untangle the effects of diet and free-ranging on tumor growth rates. In the laboratory, we showed that tumor growth rates were higher for mice fed on millet than those fed on laboratory chow. This result does begin to rule out the role of diet in explaining why the free-range mice (fed millet) exhibited slower tumor growth rates than the mice housed in the laboratory facilities (fed on chow). As future directions, seeds or pelletized food can be varied or experimentally manipulated to have different nutritional compositions (Nersesian et al., 2011). One could then observe the effects of different foods on the foraging behaviors (e.g., Schmidt et al., 1998; Shrader et al., 2008) and tumor growth rates of the free-ranging C57BL/6 or even other mice strains relevant to cancer research.
Data Availability Statement
The raw data supporting the conclusions of this article will be made available by the authors, without undue reservation.
Ethics Statement
The animal study was reviewed and approved by the Animal care and Ethics Committee of Ben-Gurion University.
Author Contributions
BK, DM, and JB conceived the project and designed the vivarium experiments. DM and EA conducted the vivarium experiments. ME and MP developed the cancer cell line and with EA and FS managed the laboratory experiments. DM, JM, EA, and JB managed data analyses. BK oversaw the entire project and wrote the initial manuscript draft. All authors contributed to edits and revisions.
Funding
This work was funded by the Israel Science Foundation (Grants 259-19 to BK and 700/16 to ME). JB acknowledges funding from NIH/NCI 1U54CA193489-01A1, “Cancer as a Complex Adaptive System,” NIH/NCI U54 Supplement, “The Tumor-host Evolutionary Arms Race.”
Conflict of Interest
The authors declare that the research was conducted in the absence of any commercial or financial relationships that could be construed as a potential conflict of interest.
Publisher’s Note
All claims expressed in this article are solely those of the authors and do not necessarily represent those of their affiliated organizations, or those of the publisher, the editors and the reviewers. Any product that may be evaluated in this article, or claim that may be made by its manufacturer, is not guaranteed or endorsed by the publisher.
Acknowledgments
We thank Liran Sagi for assistance with the initial data analysis and for writing codes that helped track the mice during the experiment. We also thank Orna Herling for helping record data. This work was carried out with the approval of the Ben-Gurion University Animal Care Committee, permit IL-08-01-2020(E).
References
Abramsky, Z., Rosenzweig, M. L., and Pinshow, B. (1991). The shape of a gerbil isocline measured using principles of optimal habitat selection. Ecology 72, 329–340. doi: 10.2307/1938926
Abramsky, Z., Rosenzweig, M. L., and Subach, A. (1997). Gerbils under threat of owl predation: isoclines and isodars. Oikos 78, 81–90. doi: 10.2307/3545803
Arcis, V., and Desor, D. (2003). Influence of environment structure and food availability on the foraging behaviour of the laboratory rat. Behav. Processes 60, 191–198. doi: 10.1016/s0376-6357(02)00122-5
Badarni, M., Prasad, M., Balaban, N., Zorea, J., Yegodayev, K. M., Ben-Zion, J., et al. (2019). Repression of AXL expression by AP-1/JNK blockage overcomes resistance to PI3Ka therapy. JCI Insight 4, e125341.
Bär, J., Leung, J. M., Hansen, C., Loke, P. N., Hall, A. R., Conour, L., et al. (2020). Strong effects of lab-to-field environmental transitions on the bacterial intestinal microbiota of Mus musculus are modulated by Trichuris muris infection. FEMS Microbiol. Ecol. 96:fiaa167.
Berger-Tal, O., and Kotler, B. P. (2010). State of emergency: gerbil behavior is affected by the hunger state of their predators. Ecology 93, 593–600.
Berger-Tal, O., Kotler, B. P., Mukherjee, S., and Brown, J. S. (2010). Complex state-dependent games between owls and gerbils. Ecol. Lett. 13, 302–310. doi: 10.1111/j.1461-0248.2010.01447.x
Betof, A. S., Lascola, C. D., Weitzel, D., Landon, C., Scarbrough, P. M., Devi, G. R., et al. (2015). Modulation of murine breast tumor vascularity, hypoxia, and chemotherapeutic response by exercise. J. Nat. Cancer Inst. 107:djv040.
Birrell, S. N., Bente, J. M., Hickey, T. E., Ricciardelli, C., Weger, M. A., Horsfall, D. J., et al. (1995). Androgens induce divergent proliferative responses in human breast cancer cell lines. J. Steroid Biochem. Mol. Biol. 52, 459–467. doi: 10.1016/0960-0760(95)00005-k
Boursot, P., Auffray, J. C., Britton-Davidian, J., and Bonhomme, F. (1993). The evolution of house mice. Ann. Rev. Ecol. Syst. 24, 119–152.
Brown, J. S. (1989). Desert rodent community structure: a test of four mechanisms of coexistence. Ecol. Monogr. 59, 1–20.
Brown, J. S. (1988). Patch use as an indicator of habitat preference, predation risk, and competition. Behav. Ecol. Sociobiol. 22,37–47.
Brown, J. S. (1992). Patch use under predation risk: I. Models and predictions. Annal. Zool. Fenni. 29, 301–309.
Brown, J. S., Kotler, B. P., and Mitchell, W. A. (1997). Competition between birds and mammals: a comparison of giving-up densities between crested larks and gerbils. Evol. Ecol. 11, 757–772. doi: 10.1023/a:1018442503955
Brown, J. S., Kotler, B. P., Smith, R. J., and Wirtz, W. O. II (1988). The effects of owl predation on the foraging behavior of heteromyid rodents. Oecologia 76, 408–415. doi: 10.1007/bf00377036
Chung, H. Y., and Park, Y. K. (2017). Rationale, feasibility and acceptability of ketogenic diet for cancer treatment. J. Cancer Prevent. 22:127.
Clarke, J. A. (1983). Moonlight’s influence on predator/prey interactions between short-eared owls (Asio flammeus) and deermice (Peromyscus maniculatus). Behav. Ecol. Sociobiol. 13, 205–209. doi: 10.1007/bf00299924
Clocchiatti, A., Cora, E., Zhang, Y., and Paolo Dotto, G. (2016). Sexual dimorphism in cancer. Nat. Rev. Cancer 16, 330–339.
Cope, E. C., Opendak, M., LaMarca, E. A., Murthy, S., Park, C. Y., Olson, L. B., et al. (2019). The effects of living in an outdoor enclosure on hippocampal plasticity and anxiety-like behavior in response to nematode infection. Hippocampus 29, 366–377. doi: 10.1002/hipo.23033
Cornwall, D. H., Ruff, J. S., Zachary, E. R., Young, C. P., Maguire, K. M., Painter, R. J., et al. (2021). Horizontal transmission of a murine retrovirus is driven by males within semi-natural enclosures. Funct. Ecol. 35, 884–893. doi: 10.1111/1365-2435.13766
Cucchi, T., and Vigne, J. D. (2006). Origin and diffusion of the house mouse in the Mediterranean. Hum. Evol. 21, 95–106. doi: 10.1007/s11598-006-9011-z
Cucchi, T., Papayianni, K., Cersoy, S., Aznar-Cormano, L., Zazzo, A., Debruyne, R., et al. (2020). Tracking the Near Eastern origins and European dispersal of the western house mouse. Sci. Rep. 10, 1–12.
Dice, L. R. (1945). Minimum intensities of illumination un-der which owls can find dead prey by sight. Am. Nat. 79, 385–416. doi: 10.1086/281276
Embar, K., Kotler, B. P., Mukherjee, S., and Brown, J. S. (2011). Risk management in optimal foragers: the effect of sightlines and predators on patch use, time allocation, vigilance, and apprehension in gerbils. Oikos 120, 1657–1666. doi: 10.1111/j.1600-0706.2011.19278.x
Ewald, P. W., and Ewald, H. A. S. (2017). “Infection and cancer in nature,” in Ecology And Evolution Of Cancer, eds B. Ujvari, B. Roche, and F. Thomas (London: Academic Press), 47–56. doi: 10.1016/b978-0-12-804310-3.00003-x
Festing, M. F. (1979). Properties of inbred strains and outbred stocks, with special reference to toxicity testing. J. Toxicol. Environ. Health 5, 53–68. doi: 10.1080/15287397909529725
Gaukler, S. M., Ruff, J. S., Galland, T., Underwood, T. K., Kandaris, K. A., Liu, N. M., et al. (2016a). Quantification of cerivastatin toxicity supports organismal performance assays as an effective tool during pharmaceutical safety assessment. Evol. Appl. 9, 685–696. doi: 10.1111/eva.12365
Gaukler, S. M., Ruff, J. S., and Potts, W. K. (2016b). Paroxetine exposure skews litter sex ratios in mice suggesting a Trivers–Willard process. Behav. Ecol. 27, 1113–1121. doi: 10.1093/beheco/arw017
Graham, A. L. (2021). Naturalizing mouse models for immunology. Nat. Immunol. 22, 111–117. doi: 10.1038/s41590-020-00857-2
Grimm, M. S., Emerman, J. T., and Weinberg, J. (1996). Effects of social housing condition and behavior on growth of the Shionogi mouse mammary carcinoma. Physiol. Behav. 59, 633–642. doi: 10.1016/0031-9384(95)02126-4
Hawkins, B. L., Heniford, B. L., Ackermann, D. M., Leonberger, M., Martinez, S. A., and Hendler, F. J. (1994). 4NQO carcinogenesis: a mouse model of oral cavity squamous cell carcinoma. Head Neck 16, 424–432. doi: 10.1002/hed.2880160506
Ho, V. W., Leung, K., Hsu, A., Luk, B., Lai, J., Shen, S. Y., et al. (2011). A low carbohydrate, high protein diet slows tumor growth and prevents cancer initiation. Cancer Res. 71, 4484–4493. doi: 10.1158/0008-5472.can-10-3973
Idorn, M., and Thor Straten, P. (2017). Exercise and cancer: from “healthy” to “therapeutic”? Cancer Immunol., Immunother. 66, 667–671.
Jones, L. W., Antonelli, J., Masko, E. M., Broadwater, G., Lascola, C. D., Fels, D., et al. (2012). Exercise modulation of the host-tumor interaction in an orthotopic model of murine prostate cancer. J. Appl. Physiol. 113, 263–272. doi: 10.1152/japplphysiol.01575.2011
Karp, N. A., Wilson, Z., Stalker, E., Mooney, L., Lazic, S. E., Zhang, B., et al. (2020). A multi-batch design to deliver robust estimates of efficacy and reduce animal use–a syngeneic tumour case study. Sci. Rep. 10, 1–10.
Kim, H., Lim, H., and Moon, A. (2018). Sex differences in cancer: epidemiology, genetics and therapy. Biomol. Ther. 26, 335–342. doi: 10.4062/biomolther.2018.103
Kokolus, K. M., Capitano, M. L., Lee, C. T., Eng, J. W. L., Waight, J. D., Hylander, B. L., et al. (2013). Baseline tumor growth and immune control in laboratory mice are significantly influenced by subthermoneutral housing temperature. Proc. Natl. Acad. Sci. U.S.A. 110, 20176–20181. doi: 10.1073/pnas.1304291110
Kotler, B. P. (1997). Patch use by gerbils in a risky environment: manipulating food and safety to test four models. Oikos 78, 274–282.
Kotler, B. P., and Blaustein, L. (1995). Titrating food and safety in a heterogeneous environment: when is the risky patch of equal value? Oikos 74, 251–258.
Kotler, B. P., Brown, J. S., and Bouskila, A. (2004). Apprehension and time allocation in gerbils: the effects of predatory risk and energetic state. Ecology 85, 917–922. doi: 10.1890/03-3002
Kotler, B. P., Brown, J. S., and Hasson, O. (1991). Factors affecting gerbil foraging behavior and rates of owl predation. Ecology 72, 2249–2260.
Kotler, B. P., Brown, J. S., Bleicher, S. S., and Embar, K. (2016). Intercontinental-wide consequences of compromise-breaking adaptations: the case of desert rodents. Israel J. Ecol. Evol. 62, 186–195. doi: 10.1080/15659801.2015.1125832
Kotler, B. P., Brown, J. S., Mukherjee, S., Berger-Tal, O., and Bouskila, A. (2010). Moonlight avoidance in gerbils reveals a sophisticated interplay among time allocation, vigilance, and state-dependent foraging. Proc. R. Soc. Lond. B. 277, 1469–1474. doi: 10.1098/rspb.2009.2036
Kotler, B. P., Brown, J. S., Smith, R. J., and Wirtz, W. O. I. I. (1988). The effects of morphology and body size on the rates of predation in desert rodents. Oikos 53, 145–152. doi: 10.2307/3566056
Lee, C., Raffaghello, L., Brandhorst, S., Safdie, F. M., Bianchi, G., Martin-Montalvo, A., et al. (2012). Fasting cycles retard growth of tumors and sensitize a range of cancer cell types to chemotherapy. Sci. Translat. Med. 4:124ra27. doi: 10.1126/scitranslmed.3003293
Leung, J. M., Budischak, S. A., Chung The, H., Hansen, C., Bowcutt, R., Neill, R., et al. (2018). Rapid environmental effects on gut nematode susceptibility in rewilded mice. PLoS Biol. 16:e2004108. doi: 10.1371/journal.pbio.2004108
Loizides, C., Iacovides, D., Hadjiandreou, M. M., Rizki, G., Achilleos, A., Strati, K., et al. (2015). Model-based tumor growth dynamics and therapy response in a mouse model of de novo carcinogenesis. PLoS One 10:e0143840. doi: 10.1371/journal.pone.0143840
Longland, W. S., and Price, M. V. (1991). Direct observations of owls and heteromyid rodents: can predation risk explain microhabitat use? Ecology 72, 2261–2273.
Madsen, T., Arnal, A., Vittecoq, M., Bernex, F., Abadie, J., Labrut, S., et al. (2017). “Cancer prevalence and etiology in wild and captive animals,” in Ecology And Evolution Of Cancer, eds B. Ujvari, B. Roche, and F. Thomas (London: Academic Press), 9–46.
Makin, D. F., Kotler, B. P., Brown, J. S., Garrido, M., and Menezes, J. F. S. (2020). The enemy within: how does a bacterium inhibit the foraging aptitude and risk management behavior of Allenby’s gerbils? Am. Nat. 196, 717–729. doi: 10.1086/711397
Meagher, S., Penn, D. J., and Potts, W. K. (2000). Male–male competition magnifies inbreeding depression in wild house mice. Proc. Natl. Acad. Sci. U.S.A. 97, 3324–3329.
Morris, J. S., Ruff, J. S., Potts, W. K., and Carrier, D. R. (2017). A disparity between locomotor economy and territory-holding ability in male house mice. J. Exp. Biol. 220, 2521–2528.
Mowry, A. V., Donoviel, Z. S., Kavazis, A. N., and Hood, W. R. (2017). Mitochondrial function and bioenergetic trade-offs during lactation in the house mouse (Mus musculus). Ecol. Evol. 7, 2994–3005.
Nencioni, A., Caffa, I., Cortellino, S., and Longo, V. D. (2018). Fasting and cancer: molecular mechanisms and clinical application. Nat. Rev. Cancer 18, 707–719. doi: 10.1038/s41568-018-0061-0
Nersesian, C. L., Banks, P. B., and McArthur, C. (2011). Titrating the cost of plant toxins against predators: determining the tipping point for foraging herbivores. J. Anim. Ecol. 80, 753–760. doi: 10.1111/j.1365-2656.2011.01822.x
O’Neill, A. M., Burrington, C. M., Gillaspie, E. A., Lynch, D. T., Horsman, M. J., and Greene, M. W. (2016). High-fat Western diet–induced obesity contributes to increased tumor growth in mouse models of human colon cancer. Nutr. Res. 36, 1325–1334.
Parohan, M., Sadeghi, A., Khatibi, S. R., Nasiri, M., Milajerdi, A., Khodadost, M., et al. (2019). Dietary total antioxidant capacity and risk of cancer: a systematic review and meta-analysis on observational studies. Crit. Rev. Oncol. Hemat. 138, 70–86.
Phifer-Rixey, M., Bi, K., Ferris, K. G., Sheehan, M. J., Lin, D., Mack, K. L., et al. (2018). The genomic basis of environmental adaptation in house mice. PLoS Genet. 14:e1007672. doi: 10.1371/journal.pgen.1007672
Rao, G. N., Birnbaum, L. S., Collins, J. J., Tennant, R. W., and Skow, L. C. (1988). Mouse strains for chemical carcinogenicity studies: overview of a workshop. Toxicol. Sci. 10, 385–394. doi: 10.1016/0272-0590(88)90285-0
Rohner, C., and Krebs, C. J. (1996). Owl predation on snowshoe hares: consequences of antipredator behaviour. Oecologia 108, 303–310. doi: 10.1007/bf00334655
Rose, M. L., Madren, J., Bunzendahl, H., and Thurman, R. G. (1999). Dietary glycine inhibits the growth of B16 melanoma tumors in mice. Carcinogenesis 20, 793–798. doi: 10.1093/carcin/20.5.793
Ruff, J. S., Cornwall, D. H., Morrison, L. C., Cauceglia, J. W., Nelson, A. C., Gaukler, S. M., et al. (2017). Sexual selection constrains the body mass of male but not female mice. Ecol. Evol. 7, 1271–1275. doi: 10.1002/ece3.2753
Ruff, J. S., Suchy, A. K., Hugentobler, S. A., Sosa, M. M., Schwartz, B. L., Morrison, L. C., et al. (2013). Human-relevant levels of added sugar consumption increase female mortality and lower male fitness in mice. Nat. Commun. 4, 1–7.
Schmidt, K. A., Brown, J. S., and Morgan, R. A. (1998). Plant defenses as complementary resources: a test with squirrels. Oikos 81, 130–142. doi: 10.2307/3546475
Shrader, A. M., Kotler, B. P., Brown, J. S., and Kerley, G. I. H. (2008). Providing water for goats in arid landscapes: effects on feeding effort with regard to time period, herd size and secondary compounds. Oikos 117, 466–472.
Song, H. K., and Hwang, D. Y. (2017). Use of C57BL/6N mice on the variety of immunological researches. Lab. Anim. Res. 33, 119–123. doi: 10.5625/lar.2017.33.2.119
Thaker, P. H., Han, L. Y., Kamat, A. A., Arevalo, J. M., Takahashi, R., Lu, C., et al. (2006). Chronic stress promotes tumor growth and angiogenesis in a mouse model of ovarian carcinoma. Nat. Med. 12, 939–944.
Trigunaite, A., Dimo, J., and Jørgensen, T. N. (2015). Suppressive effects of androgens on the immune system. Cell Immun. 294, 87–94. doi: 10.1016/j.cellimm.2015.02.004
Troxell-Smith, S. M., Tutka, M. J., Albergo, J. M., Balu, D., Brown, J. S., and Leonard, J. P. (2016). Foraging decisions in wild versus domestic Mus musculus: what does life in the laboratoryselect for? Behav. Proc. 122, 43–50. doi: 10.1016/j.beproc.2015.10.020
Wang, Y., Corr, J. G., Thaler, H. T., Tao, Y., Fair, W. R., and Heston, W. D. (1995). Decreased growth of established human prostate LNCaP tumors in nude mice fed a low-fat diet. J. Nat. Cancet Inst. 87, 1456–1462. doi: 10.1093/jnci/87.19.1456
Weber, D. D., Aminzadeh-Gohari, S., Tulipan, J., Catalano, L., Feichtinger, R. G., and Kofler, B. (2020). Ketogenic diet in the treatment of cancer–where do we stand? Mol. Metab. 33, 102–121. doi: 10.1016/j.molmet.2019.06.026
Whelan, C. J., Brown, J. S., and Hank, A. E. (2015). Diet preference in the house sparrow asser domesticus: hooked on millet? Bird Study 62, 569–573.
Yeung, F., Chen, Y. H., Lin, J. D., Leung, J. M., McCauley, C., Devlin, J. C., et al. (2020). Altered immunity of laboratory mice in the natural environment is associated with fungal colonization. Cell Host Microbe 27, 809–822.
Keywords: disease ecology, foraging ecology, foraging aptitudes, risk management, cancer, tradeoffs of food and safety, tumor growth rates, environmental effects
Citation: Makin DF, Agra E, Prasad M, Brown JS, Elkabets M, Menezes JFS, Sargunaraj F and Kotler BP (2021) Using Free-Range Laboratory Mice to Explore Foraging, Lifestyle, and Diet Issues in Cancer. Front. Ecol. Evol. 9:741389. doi: 10.3389/fevo.2021.741389
Received: 14 July 2021; Accepted: 06 September 2021;
Published: 08 October 2021.
Edited by:
Frederick R. Adler, The University of Utah, United StatesReviewed by:
James Ruff, The University of Utah, United StatesWendy Hood, Auburn University, United States
KayLene Yamada, Auburn University, United States, in collaboration with reviewer WH
Copyright © 2021 Makin, Agra, Prasad, Brown, Elkabets, Menezes, Sargunaraj and Kotler. This is an open-access article distributed under the terms of the Creative Commons Attribution License (CC BY). The use, distribution or reproduction in other forums is permitted, provided the original author(s) and the copyright owner(s) are credited and that the original publication in this journal is cited, in accordance with accepted academic practice. No use, distribution or reproduction is permitted which does not comply with these terms.
*Correspondence: Burt P. Kotler, a290bGVyQGJndS5hYy5pbA==
†These authors share first authorship